- 1Plant Conservation Unit (PCU), Department of Biological Sciences, University of Cape Town, Rondebosch, South Africa
- 2African Climate and Development Initiative (ACDI), Department of Environmental and Geographical Science, University of Cape Town, Rondebosch, South Africa
- 3Institute for Water Research (IWR), Rhodes University, Makhanda, South Africa
- 4Institute for Coastal and Marine Research (CMR), Nelson Mandela University, Gqeberha, South Africa
Landscapes are social–ecological systems (SESs) that produce ecosystem services, which change over time in response to environmental, biotic, and social drivers. Failure to consider this variability, and the feedbacks that can stabilize or destabilize systems, can have consequences for sustainable ecosystem services provision. This study applies a conceptual meta-framework, past–present–future lens, to interpret changes in land cover and ecosystem services within the Cape Floristic Region (CFR) of South Africa. Paleoecology (fossil pollen, spores, and charcoal) and participatory system dynamics modeling were used to explore long-term variability in provisioning ecosystem services (plant biodiversity) and the drivers of this variability (fire and herbivory) at Elandsberg Private Nature Reserve (Elandsberg PNR). From ca. 1800s, the paleoecological record suggests that environmental changes, particularly a transition to unpalatable Elytropappus-dominated vegetation, were driven by grazing and that an ecological threshold was crossed in ca. 1950s due to agricultural intensification. Participatory system dynamics was used to identify feedbacks in the dynamic SES structure. The ecological model replicates the paleoecological results and, furthermore, suggests that in the future, returning the system to within historical ranges variability may require sustained reductions in both grazing and fire over decades. This innovative approach blends paleoecology and participatory system dynamics to provide an evidence-based understanding of temporal variability and feedbacks for policymakers and land-use managers to inform sustainable land management.
1 Introduction
Ecosystem services are the benefits that people obtain from the environment. They are broadly categorized into supporting, provisioning, regulating, and cultural services and include biodiversity, food, clean air and water, and recreational opportunities (Millennium Ecosystem Assessment, 2005). Ecosystem services are derived from ecological infrastructure, e.g., healthy mountain catchments, wetlands, soils, and corridors of natural habitat, which together form a network of interconnected structural elements in the landscape (Cumming et al., 2014). Effective management, conservation, and restoration practices toward healthy and productive ecosystems are essential, given the critical links between ecosystem services, human wellbeing, and sustainable development (Olen and Audouin, 2007; Midgley et al., 2014; Pasquini and Cowling, 2015; Holden et al., 2021). Internal and external environmental drivers, such as climate change, fire, and land-use change, as well as biotic drivers, such as grazing, can have a stabilizing or destabilizing effect on ecosystems. Consequently, this will cause a shift in the benefits that ecosystems provide to people (Dearing et al., 2012). Furthermore, it is essential to understand how these drivers interact to maintain or regain production and the utilization of ecosystem services.
A past–present–future lens (Dawson, 2011; Birks, 2012; Gillson and Marchant, 2014; Marchant and Lane, 2014; Gillson, 2015) of environmental change can be used as a meta-framework to help in the understanding of complexity-based social–ecological systems (SESs), including variability and non-conformity with a system (Figure 1). In our study, we use paleoecology (past), stakeholder participation (present), and a simulation experiment (future) to develop a process-based perspective that can help in the understanding of SES resilience. The resilience perspective is core to systems thinking as it emphasizes non-linear dynamics, thresholds, uncertainty, and surprise (Meadows, 2008). SES resilience is the capacity and ability of a SES to change and respond to impacts by sustaining, restoring, or adapting its function, structure, and feedback. It determines how periods of gradual change interplay with periods of rapid change and how such dynamics interact across temporal and spatial scales (Folke, 2006), and how those interactions affect ecosystem services (Chapin et al., 2010; Lew et al., 2016; Marchese et al., 2018). An interdisciplinary approach is needed to understand the interacting processes that drive the dynamics of ecosystem services provision. This study applies a conceptual meta-framework, past–present–future lens, to interpret changes in land cover and ecosystem services, with the aim of identifying a “safe operating space” (Rockström et al., 2009; Dearing et al., 2014; Hossain et al., 2017) for sustainable land management within the CFR, a globally recognized biodiversity hotspot. A safe operating space is a value-based description that is important for grounding the scientific information in the SES context and encourages stakeholder ownership and buy-in for sustainable land management.
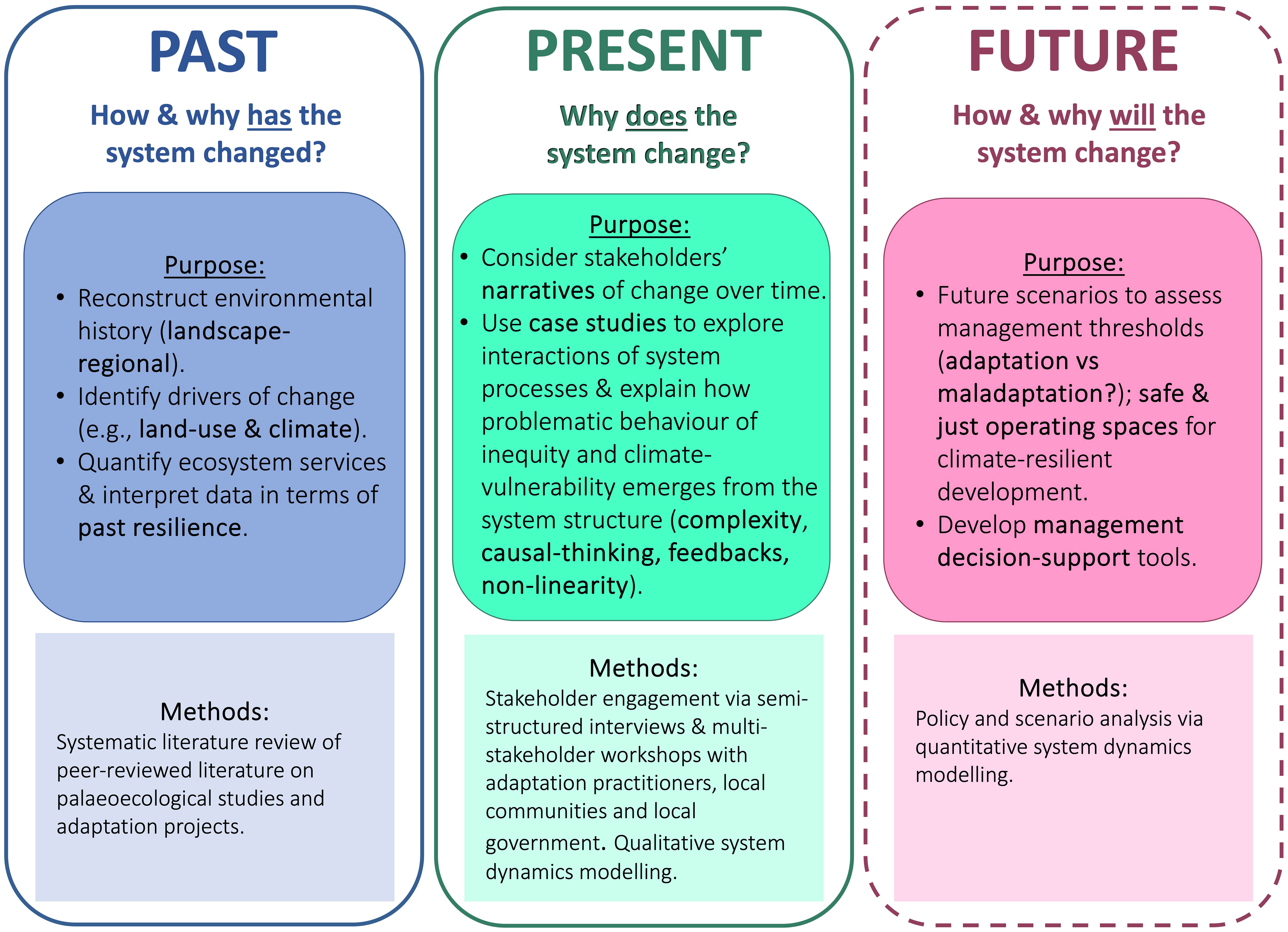
Figure 1 The past–present–future lens of environmental change as a conceptual meta-framework to explore long-term changes in ecosystem services and drivers for understanding the resilience of complexity-based social–ecological systems (SESs). Figure adapted from Dirk (2022).
Systems thinking offers a broad perspective that can be used to understand sustainability. It allows for a process-based understanding of SESs and opens the door to understanding how systems change over time. SESs do not respond in an incremental and predictable way to increasing or decreasing external pressures, an effect is rarely proportional to the cause, and what happens locally in a system often does not apply in distant regions (other states of the system or patches in the landscape) (Sterman, 2002; Reyers et al., 2018). Therefore, complexity-based SESs are non-linear in their dynamics. To achieve sustainable ecosystem management, stakeholder participation and mutual learning are required (Knight et al., 2008; Wheeler et al., 2019). Stakeholder engagement is seen as a best practice, particularly in supporting problem scoping and policy analyses (Langsdale, 2007; Videira et al., 2010; Voinov and Bousquet, 2010; Inam et al., 2015; Bou Nassar et al., 2020), and has been found to be effective in conservation science in the region (Balmford, 2003; Cowling and Pressey, 2003; Gelderblom et al., 2003; Rouget et al., 2014). Although many ecosystem services are produced independently of human intervention, research shows that people can influence the coproduction of ecosystem services (Bengtsson, 2015; Fischer and Eastwood, 2016). Therefore, this study seeks to understand how land-use managers perceive current policies and management measures, and how multiple stakeholders value the insights derived from the interdisciplinary study that incorporates paleoecology data into ecosystem assessments (Dearing et al., 2012; Gillson and Marchant, 2014; Gillson, 2015; Jeffers et al., 2015) and system dynamics data into environmental management (Ford, 2000). By obtaining these insights, the study can shed light on the intersection between human decision-making and the processes that underpin ecosystem services and resilience at the Elandsberg Private Nature Reserve (Elandsberg PNR).
System dynamics (SD) modeling, as a subset of systems thinking, is one of the many approaches and tools used to understand the complexities of social–ecological dynamics. Participatory system dynamics (PSD) is a form of SD that applies system diagrams and computer simulations in group settings (Voinov and Bousquet, 2010; Kopainsky et al., 2017). SD has been applied to environmental management interventions (Ford, 2000) for resilience (Bennett et al., 2005; Ciobanu and Saysel, 2020) and scenario planning (Turner K. G. et al., 2016; Miller et al., 2017; Allington et al., 2018), water resource management (Stave, 2003; Tidwell et al., 2004; Simonovic, 2009; Winz et al., 2009; Beall et al., 2011; Rehan et al., 2011; Wang et al., 2011; Clifford-Holmes et al., 2017b, a), marine ecosystems (Weller et al., 2016; Vermeulen et al., 2022), fire management (Collins et al., 2013), ecological restoration (Crookes et al., 2013; Turner B. L. et al., 2016; Menendez et al., 2020), and agro-ecological systems (Stave and Kopainsky, 2015; Brzezina et al., 2016; Turner B. L. et al., 2016; Von Loeper et al., 2016; Bennich et al., 2018; Herrera de Leon and Kopainsky, 2019; Turner and Kodali, 2020). Investigating the future is important as it improves our understanding of complexity-based SES by modeling dynamic behavior and non-linearity and behavior such as thresholds and regime shifts.
Furthermore, some regime shifts may show evidence for non-linear-reversible fold bifurcations with major time lags or hysteresis (Dearing et al., 2015; Jackson and Wood, 2018; González Sagrario et al., 2020). This is an important factor for land-use managers to contemplate for future resilience and sustainable development planning. Paleoecology and modeling can be used to create boundary objects such as behavior over time graphs and a visual user interface, respectively, that can facilitate dialogue between multiple stakeholders (Star and Griesemer, 1989; Black, 2013; Fischer and Riechers, 2019). These boundary objects have enough content to be interpreted differently by different knowledge holders, but maintain their integrity. The learning that comes with interpreting the paleoecological data, assessing current systemic structures and values systems, can be used to help plan for future scenarios. There are meaningful SD applications in climate change adaptation and decision support tools in South Africa (Griggs, 2013; Brent et al., 2017a; Brent et al., 2017b; Aronson et al., 2019; Carnohan et al., 2021), which provide motivation for the present study to add to this sum of knowledge. This approach of combining paleoecological data on plant biodiversity, herbivory, and fire history with qualitative data on the connections between changes in ecosystem services and land-use and climatic drivers as obtained through stakeholder engagement and quantitative SD modeling is rare. Although this meta-conceptual framework was only applied to a small geographical region with very specific conditions and vegetation types, the methodology of combining paleoecology and PSD itself is novel in the Cape Floristic Region (CFR), South Africa, and the broader African continent, with potential implications for sustainable land management and biodiversity conservation.
In this study, Elandsberg Private Nature Reserve (Elandsberg PNR) in the Berg River Catchment was chosen as a lowland conservation site with high biodiversity value and a notable history of land-use change, ranging from a transition from little human intervention prior to European settlement, to a period of intensifying agricultural use and finally to a period primarily focused on nature conservation since its proclamation as a nature reserve in 1973 when large indigenous herbivores were reintroduced. In the Elandsberg PNR case, ecosystem services are linked to human wellbeing and provide ES such as plant biodiversity, wildmeat/wool/dairy, and fuelwood, which provide economic opportunities such as ecotourism and biodiversity conservation. Integrating such evidence-, process-, and simulation-based data demonstrates the importance of landscape history as an important component of biodiversity conservation and the sustainable use of natural resources in multi-functional landscapes. The combination of techniques described above was used to address the following questions: 1) What variables/processes confer resilience and what processes stabilize or destabilize SES? 2) How can conservation and restoration practices and the sustainable management of ecosystem services be incorporated into the Elandsberg PNR?
This knowledge can serve as a basis for developing more effective management strategies and policies for future sustainability over decadal timescales similar to the timeframes of paleoecological records (Dearing et al., 2012; Gillson and Marchant, 2014; Gillson, 2015; Jeffers et al., 2015). It is necessary to undertake participatory efforts with stakeholders to establish management thresholds by correlating the pollen record with precise estimates of the land cover of the proposed indicator taxa (e.g., Gillson and Duffin, 2007). This can provide an indication of the probable efficacy of existing management strategies in restoring and safeguarding SES in the future. Thus, the mechanisms for SES resilience must be identified and the following research questions can assist in its exploration:
1) What are the main changes in plant biodiversity, fire, and herbivory over time? (past)
2) What are the feedbacks that drive these changes? (past–present)
3) Can the changes in ecosystem services, drivers, and interactions between system processes identified in the paleoecological record be simulated? (past–present)
4) How might these changes in ecosystem services, drivers, and interactions impact on ecosystem function/resilience for biodiversity conservation and ecotourism practices at Elandsberg PNR in the future? (future)
5) What sustainable land management practices can be implemented to increase ecosystem resilience (greater plant biodiversity and acceptable levels of grazing and fire) within a safe operating space? (future)
2 Materials and methods
2.1 Study site
The study site from which the paleoecological data originate (Forbes, 2014) is a lowland conservation site called Elandsberg Private Nature Reserve (Elandsberg PNR) (3,500 ha) (Figure 2). Elandsberg PNR is a Stewardship Contract Nature Reserve since 1973 situated on Farm Bartholomeus Klip (−33.45000S and 19.05000E) within the Middle Berg River Catchment, located in the Western Cape Province toward the south-west of South Africa. The Middle Berg River Catchment, which is a part of the winter rainfall zone (WRZ) of the CFR, is characterized by Mediterranean climatic conditions with warm dry summers and rainfall being at its maximum during the winter season, representing precipitation in the equatorward margin of the westerly wind belt (Chase and Meadows, 2007; Haensler et al., 2011; Chevalier and Chase, 2015). The CFR is a globally recognized biodiversity hotspot (Low and Rebelo, 1996; Mittermeier et al., 1998; Goldblatt and Manning, 2000; Myers et al., 2000; Manning and Goldblatt, 2012), and it has a long history of land use, currently supporting commercial agriculture, conservation, and urban areas. Elandsberg PNR was chosen as a proof of concept to apply a past–present–future lens and identify management targets to enhance social–ecological resilience. Located in an intermediate level of land-use disturbance and elevation gradient (151 m above sea level), it is a suitable illustrative example for capturing significant transitions in the landscape over centennial–millennial temporal scales (Dirk, 2022). Since the proclamation of Elandsberg PNR in 1973, large indigenous herbivores were reintroduced and are currently managed at the site: eland (Taurotragus oryx), blue wildebeest (Connochaetes taurinus), black wildebeest (Connochaetes gnou), zebra (Equus spp.), red hartebeest (Alcelaphus buselaphus), gemsbok (Oryx gazelle), bontebok (Damaliscus orcas orcas), and springbok (Antidorcas marsupialis). The Mediterranean climate of the WRZ and the geology at Elandsberg PNR support species-rich Renosterveld and Fynbos vegetation types. Renosterveld is an evergreen, fire-prone Mediterranean-type shrubland or asteraceous shrubland with mainly tussock (bunch) grasses and a high diversity of geophytes and typically occurs on fine-grained, nutrient-rich substrates (Mucina and Rutherford, 2006; Rebelo et al., 2006). Fynbos is classified as evergreen, hard-leaved, fire-adapted shrubland or heathland and commonly occurs on nutrient-poor soils (Moll and Jarman, 1984; Van Wilgen and Richardson, 1985; Bergh et al., 2014). The VANG core was extracted from a small-sized wetland (Forbes, 2014) on an ecotone between Swartland Shale Renosterveld and Swartland Alluvium Fynbos (Mucina and Rutherford, 2006) (Figure 3), which makes it useful in detecting vegetation change as species are at their biological and/or environmental limits at the between-biome scale (Ekblom and Gillson, 2017; MacPherson et al., 2019; Gillson et al., 2020). Pollen from the most abundant pollen types in the 1,300-year-old VANG paleoecological record is employed as a proxy for plant biodiversity, with Asteraceae long/high-spine type-1 pollen representing multiple Asteraceous plant species, yet being indistinguishable at the family and genus level, and Asteraceae Stoebe/Elytropappus-type pollen (hereafter, Renosterbos pollen) representing a single Asteraceous species, Renosterbos, which is unpalatable and, when in high abundances, is deemed less desirable for conservation. Paleo-proxies for drivers of change associated with land-use disturbance include macro-charcoal, serving as a proxy for local fire history, and coprophilous fungal spores, functioning as a proxy for herbivory/grazing. Therefore, the VANG core provided a precolonial benchmark that helped to determine whether a regime shift in fire, grazing, and Renosterbos abundance had taken place.
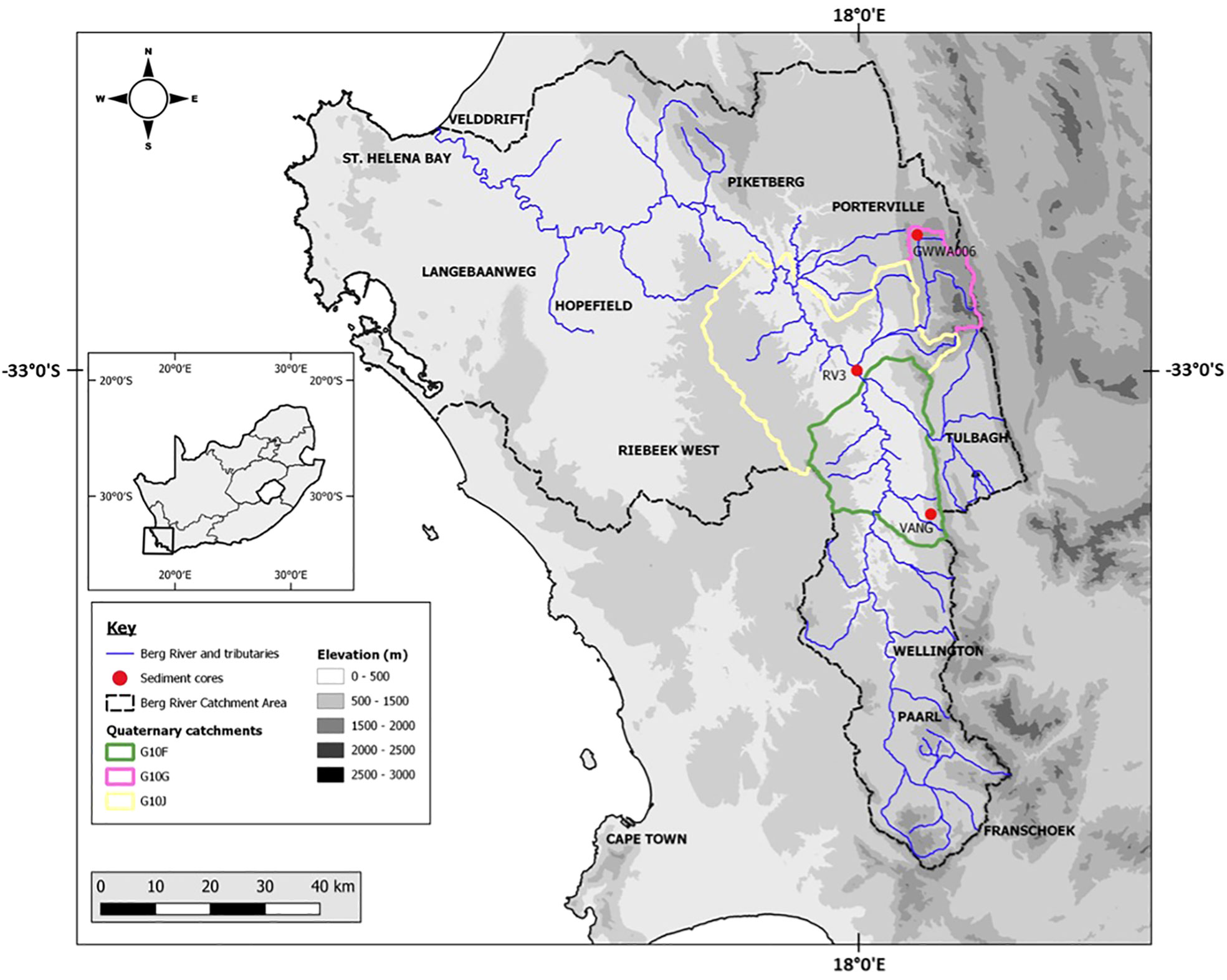
Figure 2 Map showing the general study focal area with three case study sites, with the respective quaternary catchments, covering an elevation gradient within the Middle Berg River Catchment in the Cape Floristic Region, South Africa. The lowland conservation site analyzed in this study is Elandsberg Private Nature Reserve (PNR) with the sedimentary core labeled as “VANG”.
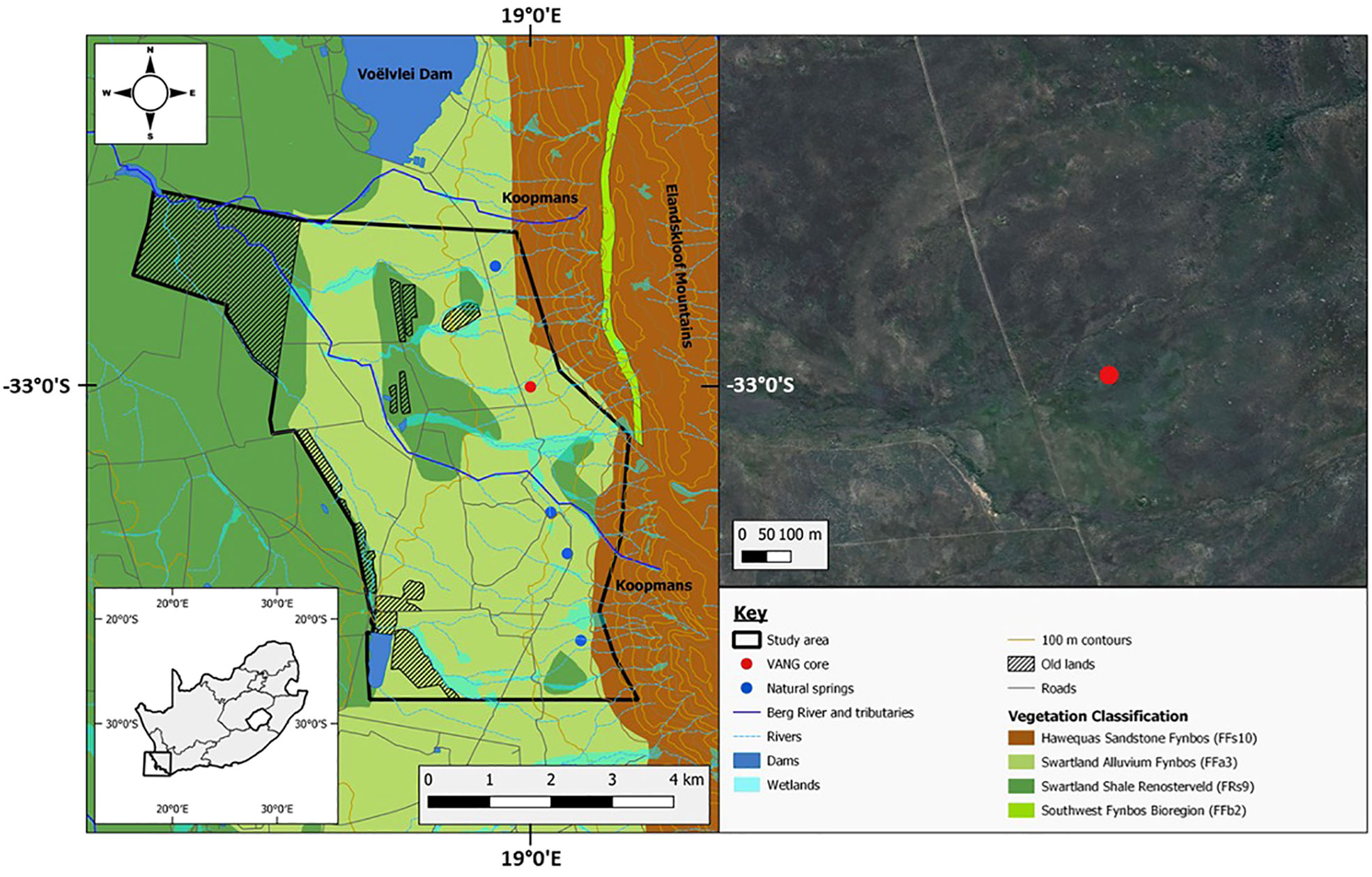
Figure 3 Map of the lowland conservation site, Elandsberg PNR, within the Lower Berg River Catchment, of the Cape Floristic Region, South Africa (Dirk, 2021: p. 65). The sediment core (VANG) was retrieved in October 2012. The paleoecological findings are published in Forbes (2014) and Forbes et al. (2018), and a subset of the paleoecological dataset was used for system dynamics analysis in the present study.
2.2 Methodological approach
There are selection of mixed methods and resulting data types that can be analyzed with a past–present–future lens (Kirsten et al., 2023), and therefore, a boundary crossing approach is required. Boundary crossing can be described as a generative process that involves the effective integration of diverse knowledge types and domains while creating new knowledge through collaborative networks and processes (Jean et al., 2018). The five-phase SD modeling process (Sterman, 2000) was used: problem articulation, formulation of a dynamic hypothesis, formulation of a simulation model, model testing, and policy design and evaluation. The modeling process provided an overarching methodological structure for integrating applied paleoecology, multistakeholder engagement, and system dynamics modeling in an iterative and scientifically rigorous manner. As Elandsberg PNR was chosen as a proof of concept, participating stakeholders (Supplementary Table 1) were aware that their contribution was part of an applied paleoecological research study and that the SD model development was exploratory in nature. The text below summarizes the methodological approach detailed in Dirk (2022), while Figure 4 illustrates the 12 process steps as they relate to the five phases of the SD modeling process and the conceptual meta-framework.
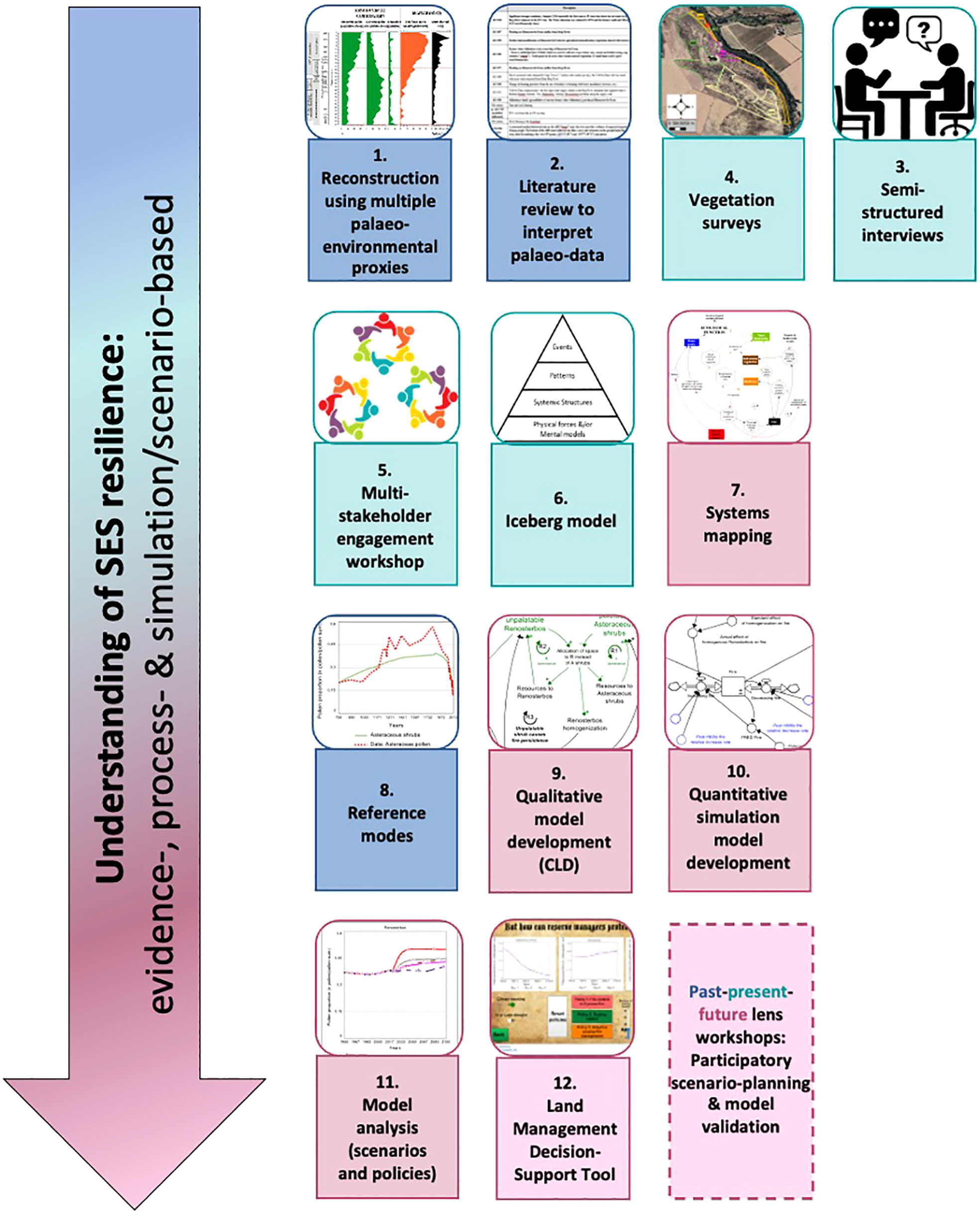
Figure 4 Summary of the methodological process steps used in this interdisciplinary study when following a past–present–future lens. The approach is moving toward a more evidence-, process-, and simulation/scenario-based understanding of social–ecological systems (SESs). Figure adapted from Dirk (2022).
2.2.1 Phase 1: problem articulation (past)
Process step 1: Past environmental change was explored using multi-proxy, high-temporal resolution quantitative paleoecological data of plant biodiversity (fossil pollen), fire history (macro-charcoal), and herbivory (coprophilous/dung fungal spores) from the VANG sedimentary record (Forbes et al., 2018). The utilization of multiple proxies offers the benefit of enabling a comprehensive examination of environmental changes, as each proxy will respond differently both temporally and spatially (Gillson and Willis, 2004; Gell et al., 2005; Pederson et al., 2006; Willis and Birks, 2006; Dearing, 2008; Gil-Romera et al., 2010; Birks, 2012; Dearing et al., 2012; Gell, 2012; Jeffers et al., 2015). Standard procedures were followed for the extraction and analysis of the paleoecological proxies and chronology (Appleby et al., 1979; Appleby, 2001; Bennett and Willis, 2002; Lowe and Walker, 2014) spanning the ca. 1,300-year VANG sedimentary record from Elandsberg PNR case study (Forbes, 2014; Forbes et al., 2018). Reconstructed environmental history was used to identify unprecedented trends within the sedimentary record at the site. These decadal–centennial–millennial time series data were used as reference modes in the development of the simulation model.
Process step 2: A historical timeline depicting past land-use and climate change events at the study site and within the CFR was composed using relevant paleoecological literature and records from historical sources including log books from Elandsberg PNR (see Supplementary Material in Forbes et al., 2018). This information defined the socioecological context and assisted with the interpretation of the long-term changes in paleoecological data from the VANG sedimentary record at Elandsberg PNR.
2.2.2 Phase 1 (continued): problem articulation (present)
Process step 3: A vegetation survey was conducted to assess the vegetation within 5 × 10 m plots located in each vegetation unit surrounding the Vangkraal Spring wetland where the VANG core was retrieved. The dominant species present in each plot were identified and their respective percentage abundance was documented. The data collected from the vegetation survey were used to evaluate the correlation between modern vegetation type and modern pollen rain. Results from the vegetation survey previously published in Forbes (2014) and Forbes et al. (2018) were used to understand the present-day landscape dynamics at the study site. It is assumed that the pollen profile from the VANG core reflects changes in vegetation, as well as the impacts of climate and land use over time at a local level, thus aided in interpreting the paleoecological data.
Process step 4: Over a 3-year period, several semistructured interviews (Supplementary Table 1) were conducted with multiple stakeholders—commercial farmers, conservation practitioners, and government authorities—to gain insights regarding the SES context and assist with paleoecological data interpretation and model development (Dirk, 2022). When it comes to modeling decision-making, there are two core principles that should be taken into consideration. First, the structure of the model should reflect the physical and institutional environment of the system in question. It is essential that it captures the structure of the SES to be effective. Second, the model needs to take into consideration the decision process of the key stakeholders involved in the real system, since their mental models may influence the overall systemic structure (Forrester, 1961; van den Belt, 2004; Maani and Cavana, 2007; Maani, 2013; Berkes, 2017).
Process step 5: The same categories of stakeholders participated in a multistakeholder engagement workshop held on 4 July 2019 (Supplementary Table 1). An adapted group model building script (Wilkerson et al., 2020) incorporating a general social learning process (Reed et al., 2010) and mediated modeling (van den Belt, 2004) was used to facilitate a consensus-based group discussion. Therefore, participatory tools such as the World Café method and sustainability dialogues (Carson, 2011; Currie, 2018) were used by the model facilitation team for variable elicitation and to encourage reflection and co-creation on how selected ecosystem services (plant biodiversity, water quality, and soil erosion regulation) are connected in the system and how the SES problems are related to land management and climate change within the Middle Berg River Catchment, CFR (Dirk, 2022).
Process step 6: The qualitative data on ecosystem services and drivers of change obtained from the semistructured interviews and multistakeholder engagement workshop were analyzed thematically using the multiple levels of a systems perspective as applied to a natural and human-designed system articulated by the iceberg model (Monat and Gannon, 2015).
Process step 7: Subsequently, the qualitative data were used to construct a visual diagram, commonly known as a “systems map,” that depicts the relationships between entities of a system and holistically considers different mental models (Mahajan et al., 2019). In-depth information collected from the semistructured interviews and workshop was not intended for making statistical inferences but rather informed the discussion of the paleoecological and computational model results. Therefore, connections between variables were recorded by the modeller using modelling software (Stella® Architect, 2019. isee systems inc.) after the multistakeholder engagement workshop, with no further active modes of engagement with stakeholders for the co-construction of the simulation model’s structures.
2.2.3 Phase 2: formulation of a dynamic hypothesis (past–present)
Process step 8: Based on the paleoecological data (time-series data used as reference modes) and systems map, an appropriate model boundary was identified for the SES problem at Elandsberg PNR.
Process step 9: Thereafter, a qualitative model was developed to illustrate the dynamic hypothesis. This qualitative model was represented as a causal loop diagram (CLD), which included both reinforcing and balancing/counteracting feedback loops. Using the systems map and CLDs to build theory diagrammatically provides a conceptual model of contemporary SES problems and how the problematic behavior emerged and changed due to the systemic structure of the SES, thus building a richer, process-based understanding (Langsdale, 2007; Videira et al., 2010; Voinov and Bousquet, 2010; Inam et al., 2015; Bou Nassar et al., 2020).
2.2.4 Phase 3: formulation of the simulation model and Phase 4: model testing (past–present–future)
Process step 10: A quantitative, simulation model was developed to focus on the effects of land-use disturbance (fire and grazing) on unpalatable Elytropappus rhinocerotis (Renosterbos) and Asteraceous shrub cover, excluding grasses. Although the paleoecological data were not calibrated according to measures of modern vegetation, fire, and grazing levels as this was not within the scope of this study, the ecological model was calibrated using paleoecological data as proxies for changes in plant biodiversity, fire, and grazing (dated ca. 750–2012 CE) (Forbes, 2014; Forbes et al., 2018). Furthermore, the ecological model formulation considered foundational literature related to resilience theory including alternative stable states and management thresholds, patch-mosaic landscapes, and disturbance and the competitive exclusion principle such as the success to the successful systems archetype (Kim and Anderson, 1998; Biggs and Rogers, 2003; Milton, 2007; Radloff et al., 2014; Bond, 2019; Gillson et al., 2019; Weiner et al., 2019; Wang and Liu, 2020). In addition to the literature, expert input provided invaluable context for perceptions of past/future drivers of change and values-based interpretation of paleoecological data, informing model development and analysis (Videira et al., 2010; Voinov and Bousquet, 2010; van den Belt and Blake, 2015; Roux et al., 2017; Helfgott, 2018). In the current model structure, a boundary decision was made to focus on the effects of land-use disturbance (fire and grazing) on Renosterbos and Asteraceous shrub cover, excluding grasses. Therefore, the ecological model represented the system as a set of stocks (Renosterbos pollen, Asteraceae pollen, macro-charcoal, and coprophilous fungal spores) and flows (e.g., increase and decrease in plant diversity, fire, and grazing in and out of the stocks). Variables such as micro-charcoal, exotic pollen, areas under protection/stewardship, “old lands” areas, and climate parameters were excluded from the stock and flow diagram (SFD) due to model boundary selection.
Climate change has an indirect effect on grazing (i.e., less precipitation has a direct effect on primary productivity and thus the vegetation cover as a grazing resource). Furthermore, climate change was indirectly included according to the way climate warming impacts the Fire Danger Index (FDI) days and consequently the likelihood of increased fire occurrence in the landscape (Forsyth and van Wilgen, 2008; Kraaij and Wilgen, 2014) (Table 1; Supplementary Table 3). It is to be noted that this model was formulated for exploratory purposes and not for predictive ones; therefore, the model structure directly associated with climate change variables, such as temperature and precipitation, was excluded as a further model boundary selection decision.
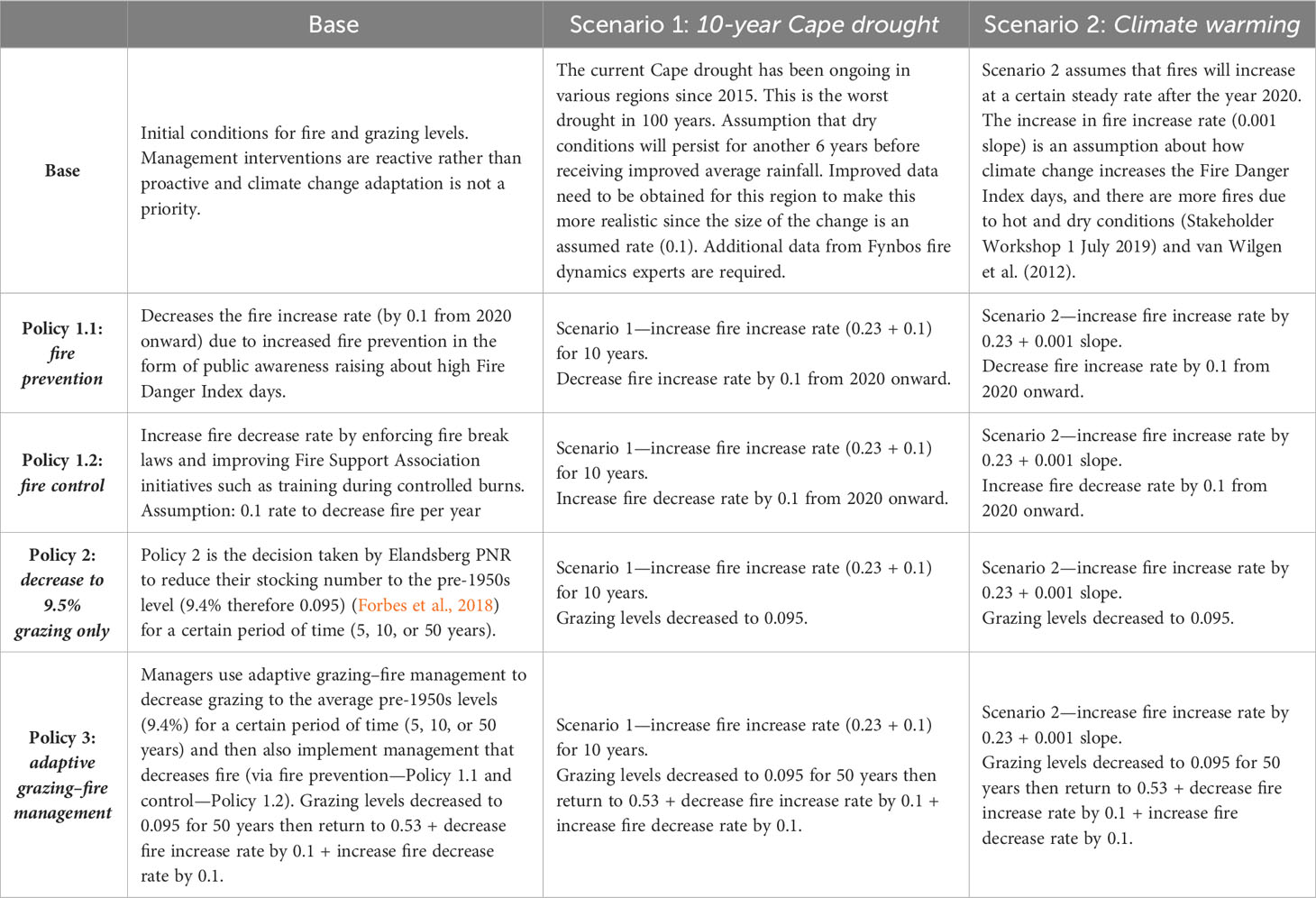
Table 1 Summary of parameters that define the policy space for land-use management at Elandsberg Private Nature Reserve used in the ecological model.
The reliability of the paleodata and the vegetation dynamics literature allowed for the optimization of rates that influenced the main flows (increasing and decreasing Asteraceous, Renosterbos, and fire). Different rates were used for pre- and post-1800 since this was when grazing began to increase with a delayed regime shift occurring in the ca. 1950s. Model validation testing was carried out throughout all five phases of the SD modeling process to increase confidence in the model structure and behavior and to provide an opportunity to improve the model iteratively. The suite of model validation tests used in this study (results shown in Supplementary Table 2) covers types/categories that have a good spread of varying degrees of complexity according to their validation hierarchy and model hierarchy (Schwaninger and Groesser, 2009; Groesser and Schwaninger, 2012). The ecological model formulation (decision rules, parameter estimation, initial values, and associated assumptions of key variables) is documented according to SD best practices in the Supplementary Material so that it can be reproduced since the SD approach advocates model transparency and the ease of communication with regard to all model elements (Martinez-Moyano, 2012). Such model documentation and transparency enable model users to identify and understand the assumptions, relationships, and data used in the model.
2.2.5 Phase 5: policy design and evaluation (present–future)
Process step 11: Various scenarios and policies linked to management thresholds were designed and evaluated via model simulation analyses (AD 750–2100), including future scenarios of changes in plant biodiversity and sensitivity analysis. Model results were discussed from 1950 to 2100 since this timescale can detect future regime shifts. This includes a relative conservation time horizon of the 2030 Sustainable Development Goals (United Nations Development Program (UNDP), 2016) and 2050 timescale used in climate change projections (Turpie et al., 2002; Meadows, 2006; Haensler et al., 2011; Hoffman et al., 2011). Furthermore, as changes in the VANG paleoecological record occurred over decadal–centennial timescales, extending the timescale to 2100 is appropriate to detect change.
Process step 12: Since the SD modeling process is iterative (Sterman, 2000), there is an opportunity to test the ecological model for model validation. Therefore, an interactive model interface referred to as a visual user interface, known as the Land Management Decision-Support Tool, was developed as a user-friendly end-product (Story Interface in Stella ® Architect, 2019) to provide decision support to Elandsberg PNR reserve managers when setting sustainable land-use guidelines/strategies on future grazing and fire that would protect local plant biodiversity, safeguard ecosystem services, and build resilience in the landscape. The interface allows for engagement with interactive data visualizations powered by the simulation model and can be used as a boundary object to facilitate dialogue (Star and Griesemer, 1989; Black, 2013; Fischer and Riechers, 2019) about any surprising simulation results at further stakeholder engagement such as past–present–future lens workshops dedicated to model validation and participatory scenario planning. Furthermore, the interface may be continually updated along with model development, aiming to provide end-users access to the model through the interface.
3 Results
3.1 A long-term paleoecological perspective
The central modeling problem of the Elandsberg PNR case study is how to increase plant biodiversity (decrease Renosterbos cover) and, therefore, improve ecosystem function for plant biodiversity conservation and possibly sustainable ecotourism practices in the future. Together with the long-term patterns from high-temporal resolution, multiproxy paleodata as reference modes (process steps 1 and 8), connections with systemic structure and mental models, as derived from stakeholder engagement insights, become more explicit. Mapping out the SES problem in this way is useful in the identification of potential leverage points as places to intervene in the system that influence the feedback loops of ecosystem services and drivers that cause the SES problems described by stakeholders and evidenced in the paleorecord. Figure 5 provides a synthesized depiction of key results relevant to the SES problem and used in model development. A further detailed account of the unabridged paleoecological record can be found in Forbes (2014) and Forbes et al. (2018).
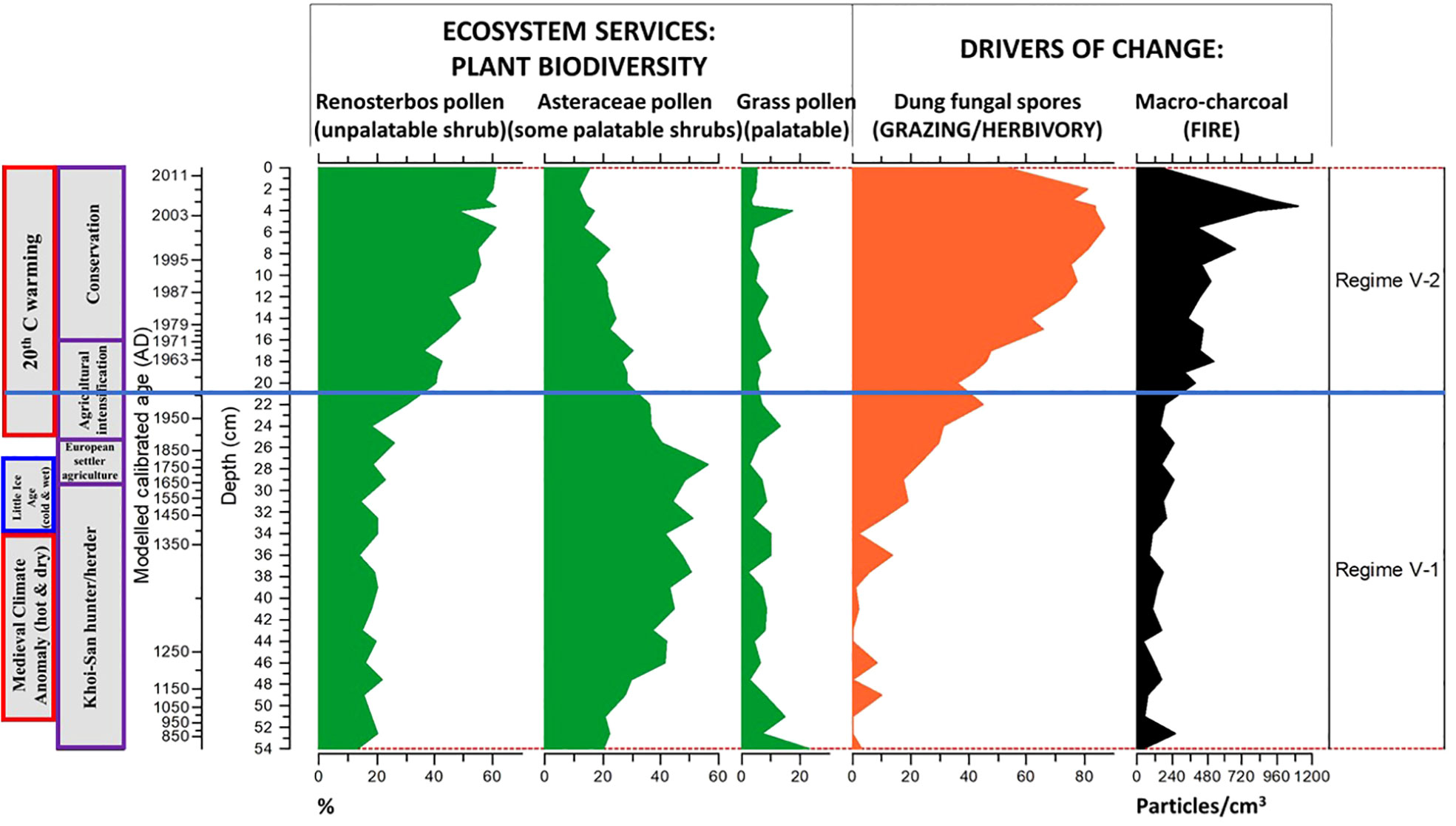
Figure 5 Synthesis diagram of key selected paleoecological proxy data from Elandsberg Private Nature Reserve, South Africa, used as reference modes that show problematic behavior over time: decreased plant biodiversity (pollen) and increased grazing (coprophilous/dung fungal spores) and local fire (macro-charcoal). Climate variation and land use during the ca. 1,300 years is summarized on the far left. Pollen zones depict a regime shift: Zone V-1 is <1950s and Zone V-2 is 1950s–2012. A further detailed account of the unabridged paleorecord can be found in Forbes (2014) and Forbes et al. (2018).
The ca. 1,300-year-old VANG sedimentary core (Forbes et al., 2018) data were used as reference modes (Figure 5) for the SD simulation model. Fossil pollen from the most abundant pollen types in the VANG paleorecord is used as a proxy for plant biodiversity. The Asteraceae family is characterized by the presence of two distinct pollen types in the paleorecord: Asteraceae long/high-spine type-1 pollen, which is indistinguishable at the family and genus levels, and Asteraceae Stoebe/Elytropappus-type pollen, which is from a single Asteraceous species called Renosterbos, that is unpalatable and, when present in high abundances, is considered less desirable for biodiversity conservation purposes. Paleoproxies for drivers of change associated with land-use disturbance include macro-charcoal as a proxy for local fire history and coprophilous fungal spores as a proxy for herbivory/grazing. Paleoecological results include the significant changes in land use at various time markers and show two ecological regimes (domains of attraction or alternative stable states) occurring over the past ca. 1,300 years—Regime V-1 from ca. AD 750–1950 and Regime V-2 from ca. AD 1950–2012. Thus, the regime shift occurred in the ca. AD 1950s during a period of agricultural intensification including grain and livestock (cattle and sheep) agriculture, and it is characterized by a decrease in plant biodiversity, evident by a decrease in Asteraceous pollen and an unprecedented increase in Renosterbos pollen in the time period in the sediment sequence from this site (Figure 5). Decreased plant biodiversity was gradually driven by increased grazing since ca. AD 1800s during a period when indigenous peoples, Khoi-San Hunter/Herders, no longer occupied the region and European settler agriculture became dominant. In the ca. AD 1950s, during agricultural intensification, the interacting effects between increased grazing and increased burning show an upward trend. Trends of increased grazing and burning continued during the ca. AD 1970s when Elandsberg PNR was proclaimed as a nature reserve and large indigenous herbivores were reintroduced in AD 1973 until the present (Figure 5).
3.2 Systems thinking and stakeholder engagement to understand complex SESs
The purpose of the multistakeholder engagement workshop was to help familiarize the stakeholders with the SD modeling process, elicit variables that explained the SES problems, and identify the model boundary (Figure 6). By using the stakeholder perceptions of the connections between ecosystem services and drivers results indicated that land-use managers with local contextual knowledge have a good understanding of the connections between ecosystem services and drivers. The thematic analysis conducted after the workshop illustrated the nuanced perspectives of changes in ecosystem services and connections to socioeconomic drivers, which are regarded as implicit mental models that are made more explicit by recording their perceptions in the visual diagram/systems map (see Supplementary Figure 1). Stakeholders recognized the importance of biodiversity and that protected areas act as a source of biodiversity needed for resilience and socioeconomic benefits. The following summarizes the perceptions that surfaced during the dialogues, which could be framed as possible problem definitions to be explored in further research in the CFR: a) plant biodiversity is threatened due to multiple interacting factors; b) land-use management decisions heavily influence the level of grazing and there is little long-term information on its impact; c) fire regimes are changing; and d) management does not have the adaptive capacity to evolve appropriately.
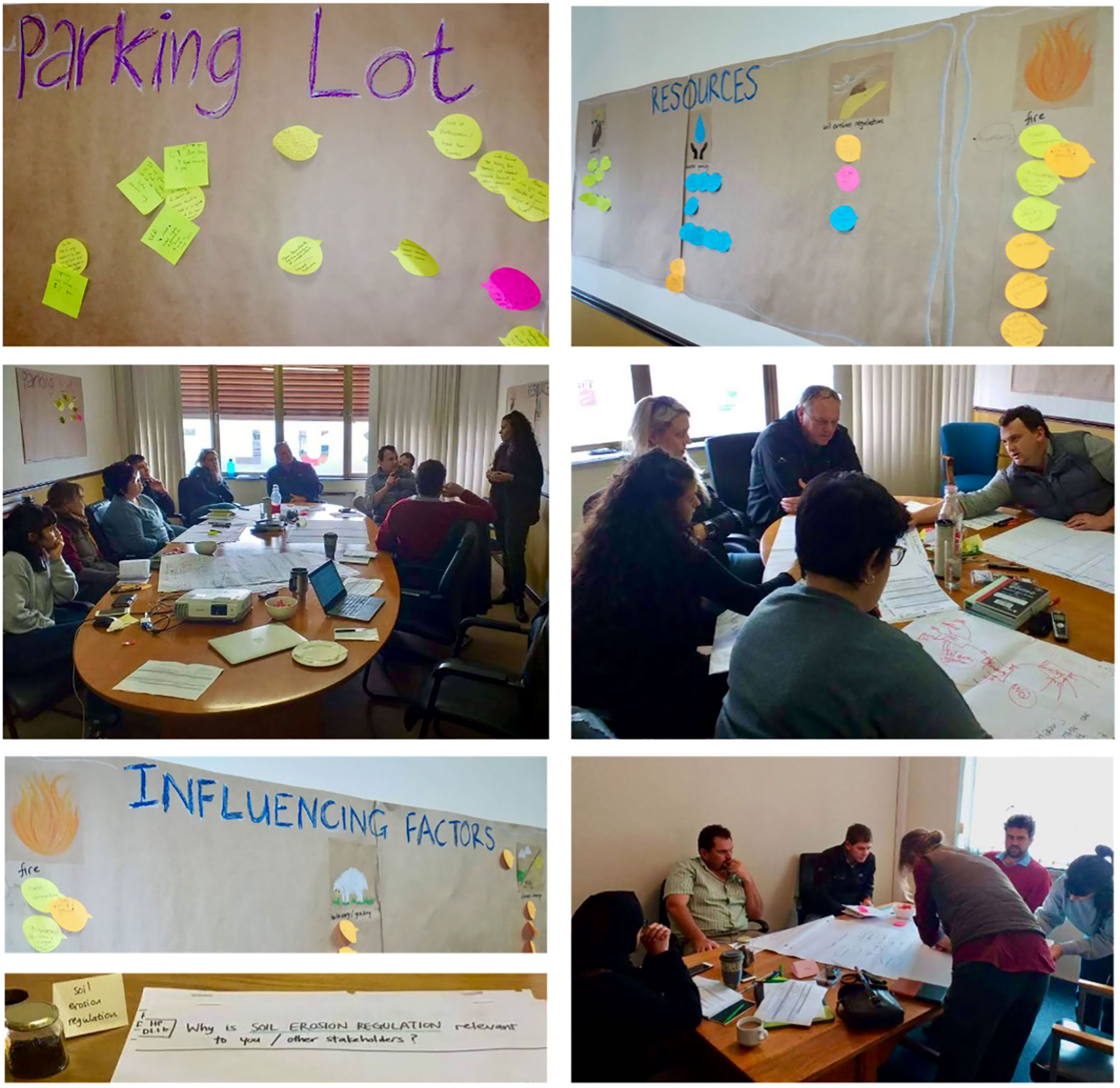
Figure 6 Photo grid of multistakeholder participation and materials used in the multistakeholder engagement workshop—designed for co-creation and reflection on sustainable land management in the Middle Berg River Catchment (Dirk, 2022: p. 214).
The systems map in Supplementary Figure 1 shows that there was a notable emphasis on systemic governance variable level that could be leverage points for achieving sustainable land management in the region. Connections between the systemic governance issues are captured in detail in the systems map (see hexagons in Supplementary Figure 1) but some examples include funding of the biodiversity section, illegal clearing of natural vegetation, tools and training to manage fire, and rehabilitation of the riparian zone. The systems map aided in zooming in on a particular SES problem (Supplementary Figure 1) and the case-specific CLD (Dirk, 2022: p. 214).
Figure 7 is important for model boundary selection, i.e., identifying key variables and stocks that would be modeled for Elandsberg PNR. The dynamic hypothesis articulated by the six feedback loops in the case-specific CLD are R1–R4, B1, and B2, which underpin the systemic structure of the SES problem. Factors mentioned in the Asteraceous shrubs dominance (R1) and Renosterbos dominance (R2) loops are the most central to the dynamic behavior over time. The reinforcing loop R1 is the loop that reinforces the dominance of Asteraceous shrubs, which is a result of the reinforcing loop R4 that reinforces the low levels of grazing and fire levels. It has been observed that there were low levels of grazing on palatable plants (grasses and some Asteraceous shrubs) by large indigenous herbivores during the period of Khoi-San Hunter/Herders inhabiting the region until AD 1600s. This was until increased grazing pressure from livestock during agricultural intensification during AD 1800s–1950s (as evidenced in Regime V-1 of Figure 5). This has resulted in the dominance of unpalatable Asteraceous shrubs which is reinforced by the feedback between resources, the space available for Asteraceous shrubs (reinforcing loop R1) and Renosterbos (reinforcing loop R2), and low grazing and fire levels (R4 and B1). A feedback between resources and the space available for Asteraceous shrubs (R1) is linked to a shift in loop dominance from Asteraceous shrubs dominance during the pre-1950s to Renosterbos dominance (R2) post-1950s and is explained by the threshold behavior observed in the paleorecord between ca. AD 1800 and 1950 (Figure 5) and how the drivers and feedbacks interacted non-linearly and caused the system to shift from one stable state (Regime V-1: Asteraceous shrub-dominated regime) to an alternative stable state (Regime V-2: unpalatable Renosterbos-dominated regime).
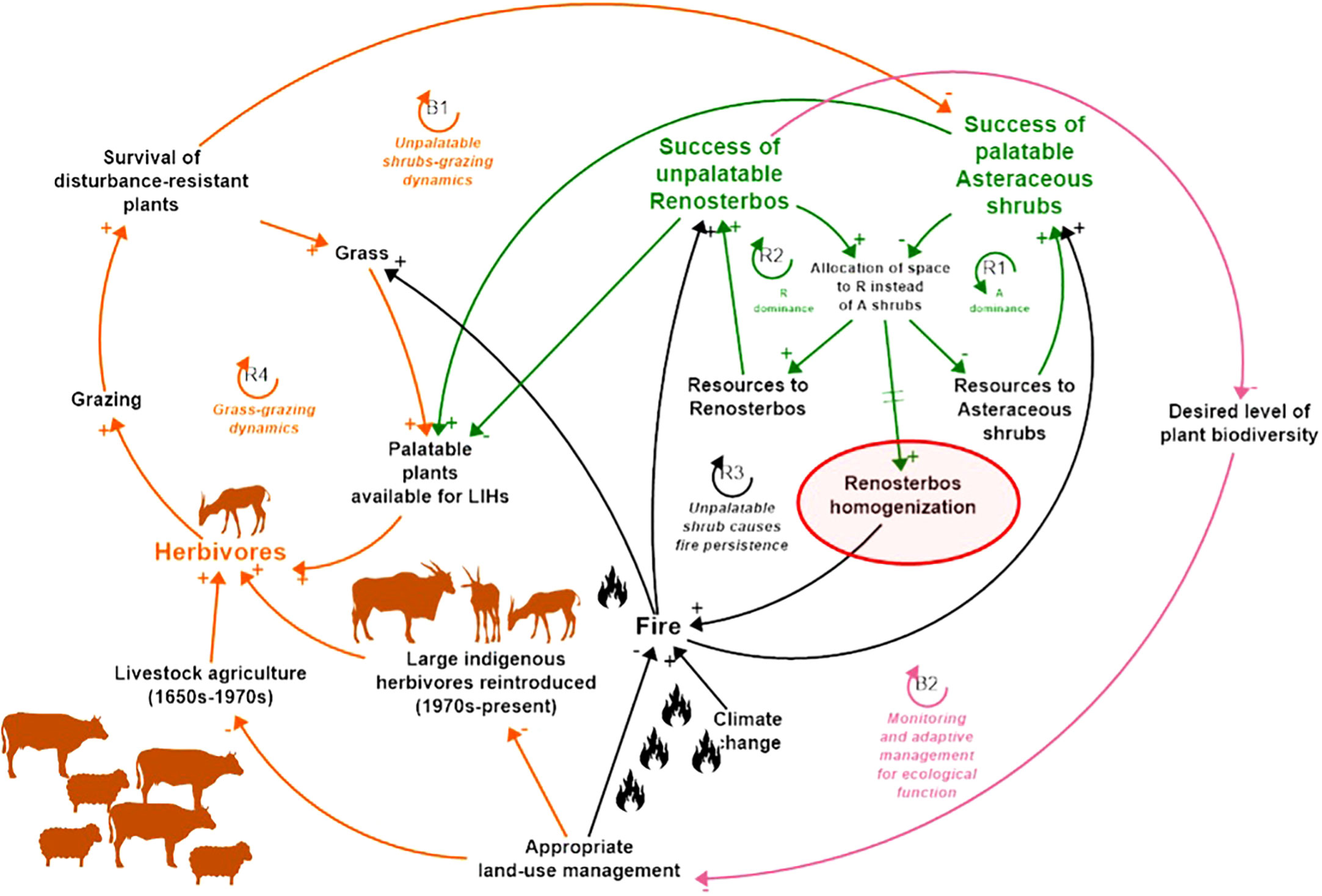
Figure 7 Causal loop diagram depicting the dynamic hypothesis (feedback loops R1–R4, B1, and B2) for the ecological model for biodiversity conservation based on Forbes et al. (2018) and Forbes (2014) and expert input resulting from the multistakeholder engagement. Connectors highlighted in pink influence the management policy and scenario analyses.
Regime V-1 in the pollen record is characterized by a heterogeneous, patch-mosaic landscape that includes patches of different vegetation units/elements (i.e., patches of Alluvium Fynbos, Renosterveld-Fynbos Ecotone, grassland, Shale Renosterveld, Grassland-Renosterveld matrix, and thicket) that result in less widespread fires, thus promoting further heterogeneity in vegetation units and varying postfire ages of vegetation patches (Forbes et al., 2018). However, once grazing levels reached a threshold, loop dominance favored Renosterbos competition. The feedbacks that maintained Regime V-2 are high grazing–fire dynamics (R3, R4, and B1), which reinforces competition that favors the unpalatable Renosterbos (R2) over other palatable Asteraceous shrubs. Despite Renosterbos dominance, at the present day, the site remains heterogeneous to a certain extent due to conservation practices including fire management and grazing management of large indigenous herbivores (Dirk, 2022). The heterogeneous landscape comprises patches of different vegetation types/units—Asteraceous shrubs, thicket, and indigenous grasses and varying postfire ages persist in the landscape though at lower levels than in precolonial times.
3.3 The interface between paleoecology and modeling
Development of the simulation model, called the ecological model, via an SFD and select model analysis results from the simulation model, the behavior of the preliminary scenario, and policy analyses are described here. The policy space for biodiversity conservation at Elandsberg PNR is defined in Table 1 and detailed in Supplementary Table 3. The ecological model sufficiently replicates the paleoecological record as reference modes and reproduces threshold behavior driven by grazing since the ca. AD 1800s resulting in an ecological threshold being crossed in ca. AD 1950s coupled with an unprecedently high local fire regime. A snapshot of the modeled plant biodiversity and fire levels in the future (AD 2050 and AD 2100) are shown in Table 2, whereas Figures 8, 9 show how trends in vegetation dynamics evolve from AD 2020 to 2100. Table 2 highlights significant findings for each simulated scenario. In the "base-case" scenario, which reflects business as usual assuming constant rates of change in the abundance of Asteraceous shrubs, Renosterbos, fire, and grazing levels, a decrease in plant biodiversity is observed, marked by an increase in Renosterbos and a decrease in Asteraceous shrubs. The shaded areas in Table 2 show a more favorable ratio (0.35 in 2050 and 0.19 in 2100) if managers choose to only control grazing (Policy 2, Table 1, Supplementary Table 3) and lack adequate resources for fire prevention and control. In this case, the Asteraceae pollen level would reach only 7.7% by 2100, and macro-charcoal levels would be 1.07 times higher (1,875 particles cm–3) compared to the base case (1,760 particles cm–3). If managers decrease fire levels only, there will be some recovery in Asteraceous shrubs but unpalatable Renosterbos still persists (Figure 8). Simulated fire control (Policy 1.1) resulted in fewer local fires (macro-charcoal 1,248 articles cm–3 (shown by red shading in Table 2)) compared with fire prevention (Policy 1.2) (macro-charcoal 1,732 particles cm–3 (shown by grey shading in Table 2)); however, fire prevention is not beneficial for increasing biodiversity (ratio 0.19 in AD 2050 and 0.09 in AD 2100—in Table 2) since Renosterveld and Fynbos vegetation is fire-prone and requires fire for regeneration.
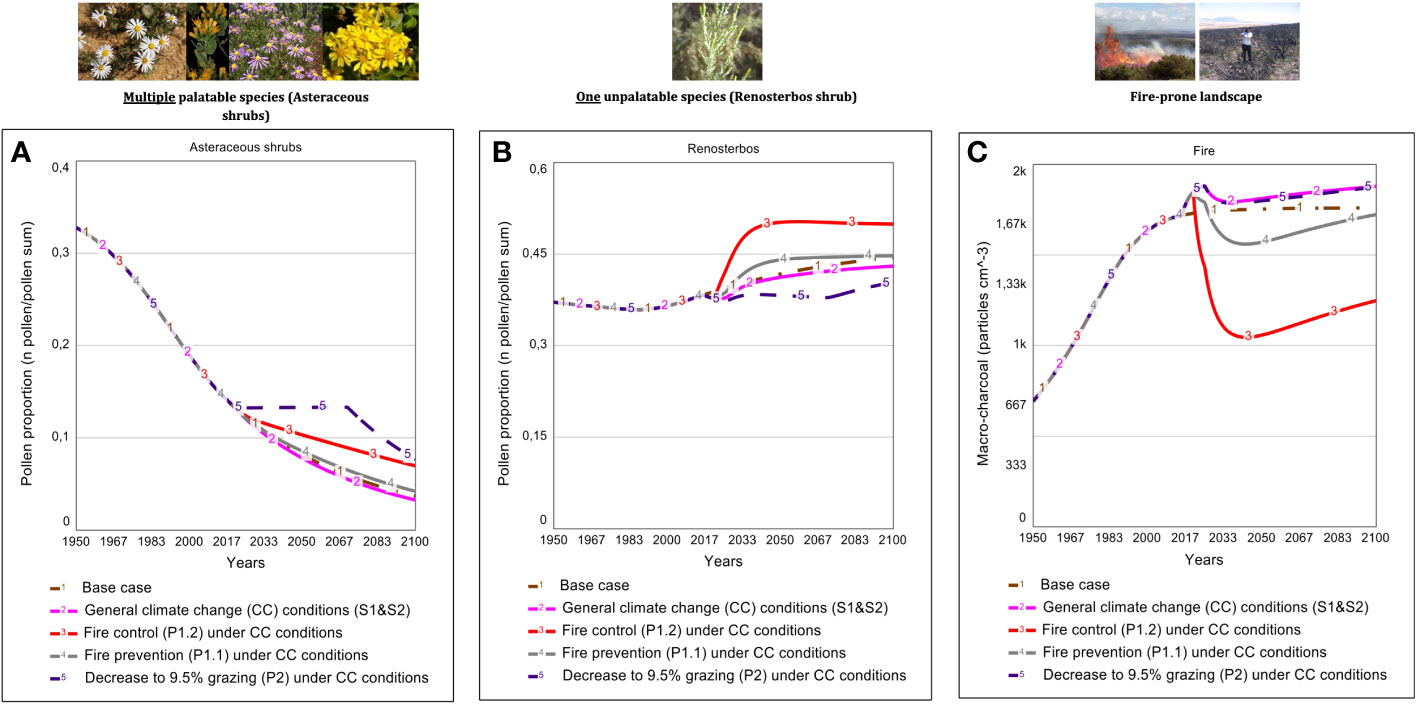
Figure 8 Five-scenario analysis outputs for the ecological model showing changes in plant biodiversity [pollen proportion data of (A) multiple Asteraceae species and (B) one unpalatable species, Renosterbos] and (C) fire (macro-charcoal) over time (1950–2100) for the Elandsberg PNR.
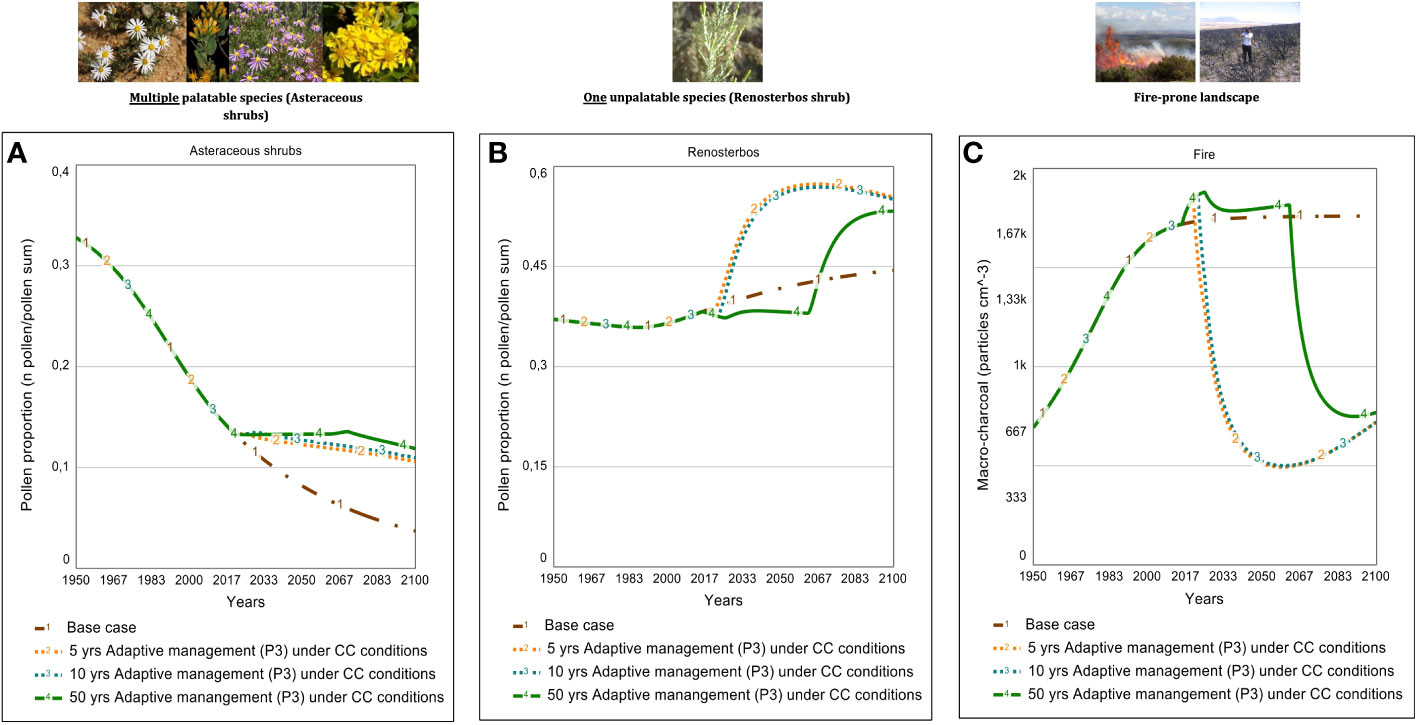
Figure 9 Expansion of Policy 3’s adaptive grazing–fire management analysis outputs (5, 10, and 50 years) compared with the base case for the ecological model showing changes in plant biodiversity [pollen proportion data of (A) multiple Asteraceae species and (B) one unpalatable species, Renosterbos] and (C) fire (macro-charcoal) over time (1950–2100) for the Elandsberg PNR.
Interestingly, the decision point variable “Duration of policy: Time to adjust grazing management” (Policy 3, Supplementary Table 3), an adaptive grazing–fire management, has varying consequences depending on the duration of implementation, which can range from 5 to 50 years. Here, the measure of equilibrium is expressed in terms of a ratio or fraction. The surface sample from ca. AD 75 to 2012 record is outside of the pre-1950s range for pollen (Forbes, 2014; Forbes et al., 2018). On average, the pre-1950s ratio of Asteraceous to Renosterbos pollen was 2.1, whereas the post-1950s ratio was low at 0.4 (Forbes et al., 2018). Ideally, policy and management would aim to return levels of grazing, fire, and plant biodiversity to that of a pre-1950s reference condition (i.e., an Asteraceous to Renosterbos pollen ratio of 2.1 and lower levels of fire and grazing). Simulation results suggest that it may not be possible to return vegetation to pre-1950s levels, so the policy should aim to decrease Renosterbos by lower grazing and control the high fire frequency problem. Therefore, Policy 3 may be the most desirable intervention to maintain this new current system state by not crossing a land cover change management threshold that is calibrated to an Asteraceae to Renosterbos pollen ratio of 0.22–0.35 (see green shading in Table 2).
However, considering the priorities of the current stakeholders managing Elandsberg PNR, it may be unfavorable for a thriving private nature reserve to decrease its large indigenous herbivore stocking rates for 50 years; thus, the duration could be decreased to 10 or 5 years. When only implementing the policy for 5–10 years before adjusting grazing back to its current levels, the simulation experiment suggests that Renosterbos pollen abundance at 2100 would be almost the same (55.0%) compared with implementing the policy adjusted after 50 years (53.0%); thus, the difference was negligible between the policy scenarios. Similarly, Asteraceous shrubs would be between 10.60% and 11.0% after adaptive management for 5–10 years, which is not much less than 11.9% after 50 years. Furthermore, the ratio of Asteraceous to Renosterbos pollen in 2050 and 2100 under various scenarios is shown in the last two columns of Table 2. Before the regime shift occurred around the ca. AD 1950s, Asteraceous shrubs and Renosterbos were in equilibrium (i.e., see Figure 5, Regime V-1 with no abrupt changes in ecosystem services and drivers) for at least 1,200 years (Forbes et al., 2018), with Asteraceous shrubs dominant in the system (see R1 of Figure 7).
4 Discussion
4.1 Insights for ecological restoration and safe operating space for the management of SES resilience
This study investigates how biodiversity, fire, and herbivory have changed over time; the feedback that drives these changes; how these changes can be simulated; and how changes in biodiversity, fire, and herbivory may impact ecosystems in the future. By simulating past change using paleoecology and participatory system dynamics modeling, we have a basis for interrogating how future management of fire and grazing might affect plant biodiversity results. In this process, it is essential to identify the drivers of a system regime shift and what variables maintain the system state in a SES; this is imperative for understanding what mechanism(s) enable conditions for the resilience of a system and can be explored through the conceptual past-present-future meta-framework (Figure 1), using Elandsberg PNR as a case study. This framework considers changes in ecosystem services and drivers of change in the context of sustainable land-use management within the CFR’s multifunctional landscape. The study contributes to a comprehensive exploration of how sustainable land management practices can be employed with the aim to manage ecosystem resilience, and the following paragraphs address the research questions outlined above.
4.1.1 What are the main changes in plant biodiversity, fire, and herbivory over time? (past)
The paleoecological record shows that the main changes that occurred were an increase in the dominance of Renosterbos post-1950 in response to increased grazing and fire (Forbes et al., 2018). The ecological processes changed slowly pre-1950s, particularly the slow increases in herbivory since the ca. AD 1800s coupled with low levels of fire, and these controlled the resilience of a patch-mosaic landscape dominated by Asteraceous shrubs. After the ca. 1950s regime shift, the rates of change increased for herbivory, and a slower increase in local fire controlled the less resilient patch-mosaic landscape that has increased Renosterbos dominance. In complexity terms, the slower processes can be viewed as controlling resilience (Biggs et al., 2009; Carpenter et al., 2009).
4.1.2 What are the feedbacks that drive these changes? (past–present)
Changes in biodiversity, fire, and herbivory over time are driven by feedbacks in the environment. For instance, a high grazing pressure can lower the availability of pasture, reducing the abundance of grasses and palatable Asteraceous shrubs. Similarly, if fire intensity and frequency increase, the abundance of Asteraceous shrubs can decrease due to their fire-prone and fire-adapted nature, and grazing pressure can increase as herbivores have more access to palatable plants. This dynamic feedback loop changes plant biodiversity (provisioning/supporting ecosystem service) over time and therefore illustrates how ecological integrity is impacted (Figure 7). As the rates of change in drivers increase, the landscape processes increase and resilience in a particular vegetation state is no longer maintained. The new vegetation state is therefore governed by new slow processes; for example, Figure 7 shows the slow process of Renosterbos homogenization by reinforcing loop R3 (unpalatable shrub causes fire persistence) which is currently maintaining the resilience of a more undesirable state.
The dynamics over time are supported conceptually by resilience theory (including alternative stable states and management thresholds), disturbance in patch-mosaic landscapes, and the competitive exclusion principle (the success to the successful systems archetype–feedback loop dominance changing from R1 to R2 over time) (Kim and Anderson, 1998; Biggs and Rogers, 2003; Milton, 2007; Radloff et al., 2014; Bond, 2019; Gillson et al., 2019; Weiner et al., 2019; Wang and Liu, 2020). Furthermore, even in the absence of precise calibration data regarding modern relationships between pollen, charcoal, and dung fungal spores, the paleoecological record from the past is an invaluable resource for understanding these changes over time (Figure 5; Supplementary Figure 2). Similar to the findings reported by Radloff et al. (2014) and Dirk (2022) in other Renosterveld landscapes within the CFR, the ca. 1,300-year-old VANG record showed that herbivory (not fire) was the main driver of change in plant biodiversity. Specifically, the paleoecological work revealed that more herbivory caused a regime shift from Asteraceous shrubs to unpalatable Renosterbos, and more local fires maintained the regime (Figure 7).
The changes in biodiversity, fire, and herbivory can have a major impact on the ecosystem function and resilience for biodiversity conservation and ecotourism practices at Elandsberg PNR. Fire and herbivory, if not managed properly, can have a negative effect on biodiversity. This can then lead to the ecosystem becoming more vulnerable to external factors, such as climate change, and having an effect on the plant biodiversity, which then has an impact on the ecosystem services, such as water quality and soil erosion. Stakeholders reported that the multiple interacting effects of fire include too frequent fires, damaged topsoil, and invasive alien plants (IAPs). As a result, plant biodiversity is likely to decrease sooner than expected as evident in the Elandsberg PNR regime shift in ca. AD 1950s. The most significant stakeholders’ perception was that the true impacts of fire are unknown due to the lack of systems that adequately monitor the effects of fire on biodiversity.
4.1.3 Can the changes in ES, drivers, and interactions between system processes identified in the paleoecological record be simulated? (past–present)
This study provides a proof of concept that it is possible to simulate the changes in ecosystem services (ES), drivers, and interactions between system processes identified in the paleoecological record. Research that combines long-term paleoecological data with system dynamics is rare (but see, e.g., Armstrong McKay et al., 2019). Therefore, the present study’s methodological approach to extend the temporal scale and by utilizing the reference modes provided by the paleoecological record during model development is unconventional in the SD field and SES research and is the first of its kind within the CFR, South Africa, and the African continent. This novel methodological approach (paleoecology, stakeholder engagement, and system dynamics) has the potential to provide management decision support for land-use managers as it considers the historical range of variability and, therefore, “paleo-safe operating space” insights based on historical range of variability into how the system may respond to different management interventions and under different future scenarios. This provides a better understanding of the complexities of the system and allows for more informed decisions about the system’s management and conservation to be made. By taking into account the physical and institutional environment and the underlying mental models of the stakeholders, we can create an effective decision-making model, which is tailored to the individual system. Therefore, PSD modeling (including future scenario techniques) can be used to simulate these processes and ultimately provide lessons from the past to improve our understanding of what interventions are needed (Gillson, 2015; Hossain et al., 2016; Armstrong McKay et al., 2019) to restore plant biodiversity and reduce degradation for sustainable land management at Elandsberg PNR.
4.1.4 How might these changes in ecosystem services, drivers, and interactions impact on ecosystem function/resilience for biodiversity conservation and ecotourism practices at Elandsberg PNR in the future? (future)
Variability in ecosystem services and the feedback affecting system stability are important to consider for sustainable ecosystem services provision. Therefore, the levels of fire, herbivory, Asteraceous shrubs, and unpalatable Renosterbos abundance (Forbes, 2014; Forbes et al., 2018) can be used as indicators to assess ecosystem function, alternative stable states and ecological thresholds, and SES resilience. Asteraceous shrubs and Renosterbos were stable for at least 1,200 years (Forbes et al., 2018), with Asteraceous shrubs dominating the system (see Regime V-1 of Figure 5). On average, the ratio of Asteraceous to Renosterbos pollen was 2.1 before 1950, but it decreased to a low 0.4 after 1950. This shift in the ratio reflects a regime shift in the ecosystem services and drivers of the system, which impacts resilience. Long-term data can provide insight into patterns associated with system structure, and modeling this SES problem related to land degradation and biodiversity loss can help to improve biodiversity conservation at this site.
The simulation model has shown that an undesirable Renosterbos-dominated alternative stable state may be approaching since ca. AD 1950s. This could variously be described as a tipping point, a crossing of an ecological threshold, a regime shift, or a transition to an alternative stable state (Holling, 1973; Ludwig et al., 1997; Folke et al., 2004; Walker and Meyers, 2004). The land-use disturbance regime shift of the ca. AD 1950s, however, was likely not a critical threshold, but the simulation results from 2022 to 2100 show a potentially new ecological regime or system state that would be crossing a critical threshold. This new state would consist of unpalatable Renosterbos together with a higher fire frequency and would be considered “negatively resilient” due to its resistance to change (Lake, 2013; Grace and Pope, 2015; Oliver et al., 2015). Adaptive grazing–fire management practices that decrease grazing pressure and the rate of fire (Figure 7) are recommended to maintain or improve current levels of plant biodiversity and prevent a future regime shift to an alternative stable state, “degraded Renosterveld” (Dirk, 2022).
4.1.5 What sustainable land management practices can be implemented to increase ecosystem resilience within a safe operating space?
The time-series data in Figure 5 show that the paleoecological record can be used to test assumptions and make informed management recommendations for the future. The VANG record from Elandsberg PNR, spanning ca. 1,300 years, has revealed a substantial shift from a pre-1950s to post-1950s regime in terms of the ecological character (Finlayson et al., 2005; Gell et al., 2013; Davidson, 2016; Gell et al., 2018). This shift means that setting biodiversity conservation targets based on the reference state of AD 1973 is unrealistic, as the landscape was already degraded when Elandsberg PNR was proclaimed in AD 1973. If the dynamic hypothesis shown in Dirk (2022: p. 214; Figure 7) is true, then the main impacts on ecosystem services are a decrease in plant biodiversity (supporting and provisioning services) with subsequent negative impacts on other ecosystem services such as water quality, soil erosion regulation, pollination (regulating services) and recreation, livelihoods, and economic activity due to social conflict between biodiversity conservation and ecotourism priorities (cultural services). Therefore, to increase ecosystem resilience and create a safe operating space, we recommend using adaptive grazing–fire management practices, such as a) reducing grazing to pre-1950s levels for a desirable period of time and b) implementing fire prevention and improved fire control. These measures should be implemented over decades and might be complemented by manual clearing of Renosterbos. Though potentially costly and challenging, manual clearing of Renosterbos and rehabilitation using various clearing techniques (e.g., loppers, slashers, dickers, and varying burning intensities) (Millar et al., 2007; Palmer, 2010), alongside reduced grazing and burning, will hopefully maintain heterogeneity and the current regime where the ratio of Asteraceae : Renosterbos pollen is between 0.22 and 0.35 (Table 2).
In addition, changes in biodiversity, fire, and herbivory can also affect the cultural services provided by the ecosystem, such as recreation, livelihoods, and economic activity. Therefore, it is important to consider how these changes may impact the ecosystem services and resilience of Elandsberg PNR in order to ensure its sustainability. Structural character resilience can be recognized by a loss of original ecological character due to instability of a vegetation type, with an ecological threshold being crossed. Simulation results show increased resilience of an undesirable degraded state (Figure 9). Therefore, functional redundancy may explain the structural character resilience (Dirk, 2022) at a between-biome scale of the ecosystem (see Figure 3) at the ecotone between Renosterveld and Fynbos vegetation) despite crossing a land-use disturbance threshold in ca. AD 1950s (Figure 5). Given the evidence for possible hysteresis in the future, we recommend that managers maintain and monitor current land-use practices or restore ecological character to avoid a critical ecological threshold being crossed. Reversibility of an even more degraded Renosterveld state in the future (Slingsby et al., 2014; Forbes et al., 2018) is highly unlikely. Hysteresis must be considered when setting thresholds for adaptive grazing–fire management (Holling, 1973; Ludwig et al., 1997; Folke et al., 2004; Walker and Meyers, 2004), as the ecological model’s future scenario analysis suggests that it may be difficult to return the ecological system to its pre-1950s historical range of variability even if grazing and fire intensity are reduced to pre-1950s levels. Therefore, manual clearing of unpalatable Renosterbos is recommended to decrease the likelihood of a future regime shift to a degraded alternative stable state that is undesirably resilient due to its resistance to change or reversibility to a desirable state. With these practices in place, managers can actively restore the ecosystem.
4.2 Significance, limitations, and future work
The study demonstrates the importance of a past–present–future perspective in understanding the dynamics of SES. It shows the power of combining paleoecological data with stakeholder participation and SD modeling, providing a powerful tool for defining management thresholds and safe operating spaces. While representing a proof of concept, further studies are needed to develop generic insights and decision support tools and develop possible scripts and adjustments to make it applicable in other contexts and at a wider scale. The interactive simulation model interface translates long-term data that can be used by policymakers and land-use managers. It helps to generate insights on how to maintain ecological integrity that preserves supporting/provisioning and regulating services which, in turn, benefit complex SESs. This interactive tool may be valuable during future multistakeholder engagement and participatory scenario planning workshops within and outside of paleoecology and biodiversity conservation networks in similar ways that other decision support tools have benefited sustainable land management (e.g., Bieber et al., 2018; Armstrong McKay et al., 2019; Rupnik et al., 2019).
When combining methods from different fields, it is important to consider the social implications of knowledge sharing. Consequently, to further augment SES resilience, it is necessary to heighten the target multistakeholder group’s cognizance of paleoecological data to contextually define the historical range of variability and explore the impacts on ecosystem services. Furthermore, familiarity with system dynamics modeling can assist stakeholders in exploring dynamic feedback and future scenarios (Turner K. G. et al., 2016; Miller et al., 2017; Allington et al., 2018), furnishing greater comprehension of how the ecosystem may react to distinct management interventions as demonstrated in other SD decision support tools developed (Brent et al., 2017a; Brent et al., 2017b; Carnohan et al., 2021). Paleoecological evidence can ground potential future scenarios and help stakeholders plan preventative measures. The Land Management Decision Support Tool captures tacit knowledge (see Figure 10 and openly available online: https://exchange.iseesystems.com/public/cherie-dirk/ecological-model-land-management-decision-support-tool) and can be used as a boundary object to facilitate discussion, explore scenarios, and support education and outreach. The SD modeling process is iterative, and through these iterations, the model can be improved and validated, which could improve the articulation of the dynamic SES problem so that the problem can be better simulated. All of these measures can help manage trade-offs to maintain or improve plant biodiversity and support socioeconomic benefits and prevent hysteretic reorganization.
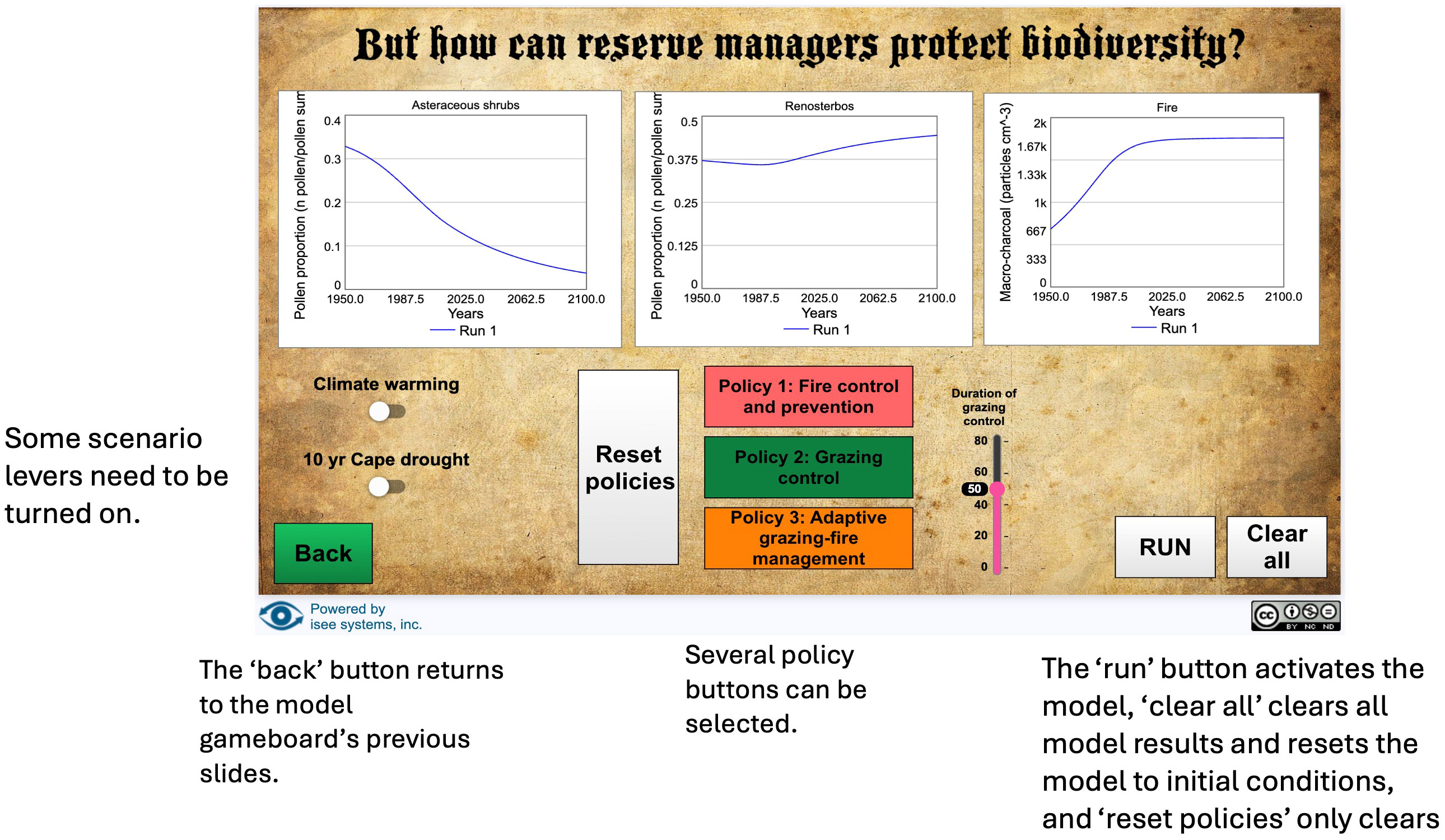
Figure 10 Screenshot of the ecological model Stella user interface to be used as a Land Management Decision Support Tool. Available via open access at the following link: https://exchange.iseesystems.com/public/cherie-dirk/ecological-model-land-management-decision-support-tool.
This exploratory study has its own limitations, such as the lack of calibration of paleoecological data with modern pollen and charcoal (e.g., Gillson and Duffin, 2007). It is essential to note the regional specificity of this study’s results, which may not be applicable across similar sites in the CFR, even though the methods might be transferable. Further research is necessary to ensure the same trends are observed in similar landscapes in the region (e.g., see high-resolution, multiproxy paleoecological insights on changes in plant biodiversity, water quality, soil erosion regulation, fire, and grazing at Rhenostervlei Farm—Dirk, 2022). Moreover, this exploratory study was limited by the model’s tight model boundary inability to account for all possible variables and interactions involved in the system. Furthermore, potential impacts of changes in biodiversity, fire, and herbivory may not be considered, thus not providing a complete picture of the system, especially because no direct paleoclimate proxies were explored in the VANG paleorecord. Thus, vectors related to climate change such as changes in temperature and rainfall and the decline in pan evaporation and wind run and extreme weather events such as floods and drought events (Turpie et al., 2002; Meadows, 2006; Haensler et al., 2011; Hoffman et al., 2011) can be included to enhance a submodel of the ecological model presented here. To improve this work in the future, the model must be developed further by incorporating more variables of a climate submodel and interactions, as the changes in temperature and precipitation will also have a strong direct impact on the distribution of plants and herbivores and the composition of communities.
Low levels of modeling experience and the lack of input from some stakeholder groups were further limitations. A more informed value-based description would require paleoproxy calibration and stakeholder consultation, especially around grazing–fire management regimes, in terms of what stakeholders perceive to be desirable and feasible and within what time period (Table 2; Figure 9). However, not all stakeholders may be willing to engage in the modeling process, making it uncertain to comment whether the current landscape state is within a safe and just operating space. This emphasizes the need for engaging stakeholders in the process of building and refining the model, as this can help to ensure that their expectations and needs are considered. Furthermore, it would be beneficial to explore the implications of including different types of stakeholders in the model building process, testing different levels of intensity of participation in the model building processes (Bots and Van Daalen, 2008; Voinov and Bousquet, 2010; Voinov et al., 2016; Clifford-Holmes et al., 2017a) and the analysis of outcomes.
At the multistakeholder engagement workshop, stakeholders expressed their concern about the current levels of fire and emphasized the need for greater fire prevention and control measures (Dirk, 2022). To better understand the dynamics of the system, more research is needed to explore how changes in biodiversity, fire, and herbivory may affect the system. The increase in fire was related to climate and was impacted by land-use management. While stakeholders are already taking fire prevention precautions by not using certain agricultural machines on high FDI days, the ratio of Asteraceae to Renosterbos pollen (0.19 in 2050 and 0.08 in 2100—Table 2) did not change significantly compared with the base case—see pink shading in Table 2. Stakeholders mentioned the inadequacy and ineffectiveness of current fire prevention precautions and suggested that additional systemic structures are needed to support the fire protection services, raise awareness, and lobby for more financial resources for fire control (Dirk, 2022). With the inclusion of the official climate predictions from the IPCC in future scenario analysis, the potential impacts of a hot dry climate (scenario 2) and drought (scenario 1) on fire could be further explored.
This research provides a comprehensive structure for stakeholder engagement in the proposed conceptual meta-framework and its replication in different contexts. Traditional formats of paleoproxy diagrams included in scientific articles of peer-reviewed journals such as Figure 5 could be perceived as too technical for anyone outside the field (and particularly for key local stakeholders) and may therefore be ineffective for on-the-ground impact. The present study provides an example of how the past–present–future conceptual meta-framework can be used and demonstrated in a user-friendly modeling tool for stakeholders (Figure 10). Although this fell outside of the scope of the study, it is essential to consider the application of this model to other regions and cultures, as well as the effectiveness of stakeholder engagement in a variety of contexts. Finally, it is important to examine if stakeholder engagement leads to more holistic approaches to ecosystem service management.
5 Conclusion
This article uses a past–present–future conceptual meta-framework to explore the variability of ecosystem services and land-use drivers in the Cape Floristic Region (CFR) of South Africa, a globally recognized biodiversity hotspot. Blending paleoecology and participatory system dynamics (PSD) provides context for understanding dynamic social–ecological systems to explore scenarios, and yields safe operating parameters and management targets for social–ecological resilience and sustainability. The following are the key takeaways from this study. 1) The paleoecological data indicate the main changes including a loss of plant biodiversity post-1950, specifically an increase in Renosterbos and a loss of palatable Asteraceous shrubs. 2) The loss of diversity was driven by increased grazing and burning, which continued throughout the transition from a farm to a nature reserve. 3) System dynamics modeling can be used to identify feedback in the dynamic social–ecological system structure. The dynamic hypothesis articulated a state shift in causal loop dominance from the abundance of multiple Asteraceous shrubs to unpalatable E. rhinocerotis (Renosterbos), and an interdisciplinary approach was used to understand the interacting processes that drive the dynamics of ecosystem services provision. 4) The changes in biodiversity, fire, and herbivory in the future could have a major impact on the ecosystem function and resilience of Elandsberg PNR. 5) Paleoecological data can be used to define the historical range of variability in plant biodiversity, herbivory, and fire. Simulation models suggest a potentially new ecological regime or system state dominated by unpalatable Renosterbos, with a higher fire frequency and difficulty in reversing it. Reduction in grazing and burning would be beneficial but might not return the plant diversity to its former state. Recommended actions to increase ecosystem resilience include adaptive grazing–fire management, reducing land-use disturbance by reducing fire and grazing, and increasing stakeholders’ awareness of paleoecological data and system dynamics. Active restoration of previous vegetation composition, such as clearing of Renosterbos, is suggested, but it can be costly and challenging. However, changes in these factors may maintain the sustainability of the ecosystem but could also affect the cultural services provided by the ecosystem.
By combining paleoecological data and participatory SD modeling, the study is a proof of concept showing that it is possible for the changes in ecosystem services, drivers, and interactions between system processes identified in the paleoecological record to be simulated. The process translates paleoecological data into a form useful for policymakers and land-use managers. A Land Management Decision Support Tool is available to facilitate discussion and explore scenarios and can be used as an education and outreach tool. By applying a contextual evidence- and process-based understanding of the temporal variability and dynamic feedback between ecosystem services and drivers, this proof of concept showed the benefits of blending paleoecology and PSD to explore future scenarios. The results of the combined approach can be used to articulate safe operating parameters and management targets that can enhance social–ecological resilience and sustainability of systems like the Elandsberg PNR, supporting the broad objectives of sustainable land management and biodiversity conservation.
Data availability statement
The datasets presented in this study can be found in online repositories. The names of the repository/repositories and accession number(s) can be found below: https://vula.uct.ac.za/portal/directtool/2a1a2592-86f6-4f27-ac3c-82f0cc0634cd/. The datasets generated and analyzed for this study can be found in the Palaeoecology Laboratory (Plant Conservation Unit, University of Cape Town, South Africa); PI: Professor Lindsey Gillson.
Ethics statement
The studies involving humans were approved by Dr Shari Daya (Chair: Faculty of Science Research Ethics Committee); Faculty of Science, University of Cape Town, Rondebosch, South Africa, 7701. The studies were conducted in accordance with the local legislation and institutional requirements. The participants provided their written informed consent to participate in this study. Written informed consent was obtained from the individual(s) for the publication of any potentially identifiable images or data included in this article.
Author contributions
CF was the lead modeller and author on this paper and carried out the palaeoecological, stakeholder engagement and system dynamics modelling analysis. JC-H assisted in model development and writing. LG conceptualised the study, supervised the palaeoecological work, contributed to stakeholder workshops, and contributed to editing and providing financial support. All authors have read and agreed to the current version of the manuscript. This research was funded by the National Research Foundation Global Change Grand Challenge (SASSCAL) (Grant number 118589).
Funding
The National Research Foundation’s (NRF) Global Change Grand Challenge, Southern African Science Service Centre for Climate Change and Adaptive Land Management (SASSCAL) (Grant number 118589) provided core funding for field work, laboratory work and equipment. I would also like to thank the following for providing co-funding: Department of Biological Sciences (Dorothy Cameron Scholarship – 2016), David and Elaine Potter Foundation (Potter Fellowship – 2017-2019) and PAST (2020).
Conflict of interest
The authors declare that the research was conducted in the absence of any commercial or financial relationships that could be construed as a potential conflict of interest.
Publisher’s note
All claims expressed in this article are solely those of the authors and do not necessarily represent those of their affiliated organizations, or those of the publisher, the editors and the reviewers. Any product that may be evaluated in this article, or claim that may be made by its manufacturer, is not guaranteed or endorsed by the publisher.
Supplementary material
The Supplementary Material for this article can be found online at: https://www.frontiersin.org/articles/10.3389/fcosc.2023.1160043/full#supplementary-material
References
Allington G. R. H., Fernandez-Gimenez M. E., Chen J., Brown D. G. (2018). Combining participatory scenario planning and systems modeling to identify drivers of future sustainability on the Mongolian Plateau. Ecol. Soc. 23. doi: 10.5751/ES-10034-230209
Appleby P. G. (2001). “Chronostratigraphic techniques in recent sediments,” in Tracking Environmental Change Using Lake Sediments vol. 1 Basin Analysis, Coring, and Chronological Techniques. Eds. Last W. M., Smol J. P. (Dordrecht: Kluwer).
Appleby P. G., Oldfield F., Thompson R., Huttunen P., Tolonen K. (1979). 210Pb dating of annually laminated lake sediments from Finland. Nature 280, 53–55. doi: 10.1038/280053a0
Armstrong McKay D. I., Dearing J. A., Dyke J. G., Poppy G. M., Firbank L. G. (2019). To what extent has sustainable intensification in England been achieved? Sci. Total Environ. 648, 1560–1569. doi: 10.1016/j.scitotenv.2018.08.207
Aronson J., Shackleton S., Sikutshwa L. (2019). Joining the puzzle pieces: reconceptualising ecosystem-based adaptation in South Africa within the current natural resource management and adaptation context.
Balmford A. (2003). Conservation planning in the real world: South Africa shows the way. Trends Ecol. Evol. 18, 435–438. doi: 10.1016/S0169-5347(03)00217-9
Beall A., Fiedler F., Boll J., Cosens B. (2011). Sustainable water resource management and participatory system dynamics. Case study: Developing the palouse basin participatory model. Sustainability 3, 720–742. doi: 10.3390/su3050720
Bennett E. M., Cumming G. S., Peterson G. D. (2005). A systems model approach to determining resilience surrogates for case studies. Ecosystems 8, 945–957. doi: 10.1007/s10021-005-0141-3
Bennett K. D., Willis K. J. (2002). “Pollen,” in Tracking Environmental Change Using Lake Sediments (Dordrecht: Kluwer Academic Publishers).
Bennich T., Belyazid S., Kopainsky B., Diemer A. (2018). Understanding the transition to a bio-based economy: Exploring dynamics linked to the agricultural sector in Sweden. Sustain 10, 1504. doi: 10.3390/su10051504
Bergh N. G., Verboom G. A., Rouget M., Cowling R. M. (2014). “Vegetation types of the greater Cape Floristic Region,” in Fynbos: Ecology, evolution, and conservation of a megadiverse region. Eds. Allsopp N., Verboom G. A. (London: Oxford University Press).
Berkes F. (2017). Environmental governance for the anthropocene? Social-ecological systems, resilience, and collaborative learning. Sustain 9, 1232. doi: 10.3390/su9071232
Bieber N., Ker J. H., Wang X., Triantafyllidis C., van Dam K. H., Koppelaar R. H. E. M., et al. (2018). Sustainable planning of the energy-water-food nexus using decision making tools. Energy Policy 113, 584–607. doi: 10.1016/j.enpol.2017.11.037
Biggs H., Rogers K. (2003). An Adaptive System to Link Science, Monitoring, and Management in Practice. Eds. du Toit J. T., Rogers K. H., Biggs H. C. (Washington DC: Island Press).
Biggs R., Carpenter S. R., Brock W. A. (2009). Turning back from the brink: Detecting an impending regime shift in time to avert it. Proc. Natl. Acad. Sci. 106, 826–831. doi: 10.1073/pnas.0811729106
Birks H. J. B. (2012). Ecological palaeoecology and conservation biology: Controversies, challenges, and compromises. Int. J. Biodivers. Sci. Ecosyst. Serv. Manag. 8, 292–304. doi: 10.1080/21513732.2012.701667
Black L. J. (2013). When visuals are boundary objects in system dynamics work. Syst. Dyn. Rev. 29, 70–86. doi: 10.1002/sdr.1496
Bond W. J. (2019). Open Ecosystems: Ecology and evolution beyond the forest edge (Oxford: Oxford University Press).
Bots P. W. G., Van Daalen C. E. (2008). Participatory model construction and model use in natural resource management: A framework for reflection. Syst. Pract. Action Res. 21, 389–407. doi: 10.1007/s11213-008-9108-6
Bou Nassar J., Malard J., Adamowski J., Ramírez Ramírez M., Medema W., Tuy H. (2020). Multi-level storylines for participatory sociohydrological modelling – involving marginalized communities in Tz’olöj Ya’, Mayan Guatemala. Hydrol. Earth Syst. Sci. Discuss, 1–27. doi: 10.5194/hess-2020-437
Brent A., Musango J. K., Smit S., Pillay N. S., Botha A., Louw R., et al. (2017a). Utilization of system dynamics in Southern Africa: A systematic review. Syst. Res. Behav. Sci. 34, 657–670. doi: 10.1002/sres.2401
Brent A., Simelane T., Clifford-Holmes J. K. (2017b). “Introduction to system dynamics as a decision-support tool for developmental planning,” in System Dynamics Models for Africa’s Developmental Planning. Eds. Brent A., Simelane T. (Pretoria: Africa Institute of South Africa).
Brzezina N., Kopainsky B., Mathijs E. (2016). Can organic farming reduce vulnerabilities and enhance the resilience of the European food system? A critical assessment using system dynamics structural thinking tools. Sustain 8, 971. doi: 10.3390/su8100971
Carnohan S. A., Clifford-Holmes J. K., Retief H., McKnight U. S., Pollard S. (2021). Climate change adaptation in rural South Africa: Using stakeholder narratives to build system dynamics models in data-scarce environments. J. Simul. 15, 5–22. doi: 10.1080/17477778.2020.1762516
Carpenter S. R., Mooney H. A., Agard J., Capistrano D., Defries R. S., Diaz S., et al. (2009). Science for managing ecosystem services: Beyond the Millennium Ecosystem Assessment. Proc. Natl. Acad. Sci. U.S.A. 106, 1305–1312. doi: 10.1073/pnas.0808772106
Carson L. (2011). Designing a public conversation using the world cafe method. Soc. Altern. 30, 10–14.
Chapin F. S., Carpenter S. R., Kofinas G. P., Folke C., Abel N., Clark W. C., et al. (2010). Ecosystem stewardship: sustainability strategies for a rapidly changing planet. Trends Ecol. Evol. 25, 241–249. doi: 10.1016/j.tree.2009.10.008
Chase B., Meadows M. (2007). Late Quaternary dynamics of southern Africa’s winter rainfall zone. Earth-Sci. Rev. 84, 103–138. doi: 10.1016/j.earscirev.2007.06.002
Chevalier M., Chase B. M. (2015). Southeast African records reveal a coherent shift from high- to low-latitude forcing mechanisms along the east African margin across last glacial-interglacial transition. Quat. Sci. Rev. 125, 117–130. doi: 10.1016/j.quascirev.2015.07.009
Ciobanu N., Saysel A. K. (2020). Using social–ecological inventory and group model building for resilience assessment to climate change in a network governance setting: a case study from Ikel watershed in Moldova. Environ. Dev. Sustain. 23, 1065–1085. doi: 10.1007/s10668-020-00590-8
Clifford-Holmes J. K., Slinger J. H., Palmer C. G. (2017a). “Using system dynamics modeling in South African water management and planning,” in System Dynamics models for Africa’s Developmental planning. UK: John Wiley & Sons Ltd.
Clifford-Holmes J. K., Slinger J. H., de Wet C., Palmer C. G. (2017b). Modelling in the ‘muddled middle’: A case study of water service delivery in post-apartheid South Africa. Soc. Syst. Eng. Des. Complex, 215–234. doi: 10.1002/9781118974414.ch11
Collins R. D., de Neufville R., Claro J., Oliveira T., Pacheco A. P. (2013). Forest fire management to avoid unintended consequences: A case study of Portugal using system dynamics. J. Environ. Manage 130, 1–9. doi: 10.1016/j.jenvman.2013.08.033
Cowling R., Pressey R. (2003). Introduction to systematic conservation planning in the Cape Floristic Region. Biol. Conserv. 112, 1–13. doi: 10.1016/S0006-3207(02)00418-4
Crookes D. J., Blignaut J. N., de Wit M. P., Esler K. J., Le Maitre D. C., Milton S. J., et al. (2013). System dynamic modelling to assess economic viability and risk trade-offs for ecological restoration in South Africa. J. Environ. Manage 120, 138–147. doi: 10.1016/j.jenvman.2013.02.001
Cumming T., Driver A., Botha M., Manuel J., Dini J., Stephens A. (2014). A Framework for Investing in Ecological Infrastructure in South Africa. South Africa: SANBI.
Currie B. (2018). Social learning for public participation in environmental governance: The case of Knysna Municipality (South Africa: Nelson Mandela University).
Davidson N. C. (2016). Editorial: Understanding change in the ecological character of internationally important wetlands. Mar. Freshw. Res. 67, 685–686. doi: 10.1071/MF16081
Dawson T. P. (2011). Beyond predictions: Biodiversity conservation in a changing climate. Science 332, 53–58. doi: 10.1126/science.1200303
Dearing J. (2008). Landscape change and resilience theory: a palaeoenvironmental assessment from Yunnan, SW China. Holocene 18, 117–127. doi: 10.1177/0959683607085601
Dearing J. A., Acma B., Bub S., Chambers F. M., Chen X., Cooper J., et al. (2015). Social-ecological systems in the Anthropocene: The need for integrating social and biophysical records at regional scales. Anthr. Rev. 2, 220–246. doi: 10.1177/2053019615579128
Dearing J. A., Wang R., Zhang K., Dyke J. G., Haberl H., Hossain M. S., et al. (2014). Safe and just operating spaces for regional social-ecological systems. Glob. Environ. Chang. 28, 227–238. doi: 10.1016/j.gloenvcha.2014.06.012
Dearing J. A., Yang X., Dong X., Zhang E., Chen X., Langdon P. G., et al. (2012). Extending the timescale and range of ecosystem services through paleoenvironmental analyses, exemplifiedin the lower Yangtze basin. Proc. Natl. Acad. Sci. U.S.A. 109, 1111–1120. doi: 10.1073/pnas.1118263109
Dirk C. J. (2022). Using applied palaeoecology and participatory system dynamics modelling to investigate changes in ecosystem services in response to climate and social-ecological drivers within the middle berg river catchment, South Africa (South Africa: University of Cape Town).
Ekblom A., Gillson L. (2017). The importance of paleoecology in the conservation and restoration of cultural landscapes. Past. Glob. Chang. Mag. 25, 88–89. doi: 10.22498/pages.25.2.88
Finlayson C. M., Bellio M. G., Lowry J. B. (2005). A conceptual basis for the wise use of wetlands in northern Australia - Linking information needs, integrated analyses, drivers of change and human well-being. Mar. Freshw. Res. 56, 269–277. doi: 10.1071/MF04077
Fischer J., Riechers M. (2019). A leverage points perspective on sustainability. People Nat. 1, 115–120. doi: 10.1002/pan3.13
Folke C. (2006). Resilience: The emergence of a perspective for social-ecological systems analyses. Glob. Environ. Chang. 16, 253–267. doi: 10.1016/j.gloenvcha.2006.04.002
Folke C., Carpenter S., Walker B., Scheffer M., Elmqvist T., Gunderson L., et al. (2004). Regime shifts, resilience, and biodiversity in ecosystem management. Annu. Rev. Ecol. Evol. Syst. 35, 557–581. doi: 10.1146/annurev.ecolsys.35.021103.105711
Forbes C. J. (2014). Benchmarks for the future: long-term vegetation change derived from palaeoecological techniques in West-Coast Renosterveld, South Africa (South Africa: University of Cape Town).
Forbes C. J., Gillson L., Hoffman M. T. (2018). Shifting baselines in a changing world: Identifying management targets in endangered heathlands of the Cape Floristic Region, South Africa. Anthropocene 22, 81–93. doi: 10.1016/j.ancene.2018.05.001
Ford A. (2000). Modeling the environment: an introduction to system dynamics modeling of environmental systems. Int. J. Sustain. High Educ. 1. doi: 10.1108/ijshe.2000.24901aae.002
Forsyth G. G., van Wilgen B. W. (2008). The recent fire history of the Table Mountain National Park and implications for fire management. Koedoe 50, 3–9. doi: 10.4102/koedoe.v50i1.134
Gelderblom C. M., Van Wilgen B. W., Nel J. L., Sandwith T., Botha M., Hauck M. (2003). Turning strategy into action: Implementing a conservation action plan in the Cape Floristic Region. Biol. Conserv. 112, 291–297. doi: 10.1016/S0006-3207(02)00399-3
Gell P. (2012). “With the benefit of hindsight: the utility of palaeoecology in wetland condition assessment and identification of restoration targets,” in Ecology of Industrial Pollution. UK: Cambridge University Press.
Gell P., Mills K., Grundell R. (2013). A legacy of climate and catchment change: The real challenge for wetland management. Hydrobiologia 708, 133–144. doi: 10.1007/s10750-012-1163-4
Gell P. A., Perga M.-E., Finlayson C. M. (2018). “Changes over time,” in Freshwater Ecology and Conservation. UK: Oxford University Press.
Gell P., Tibby J., Fluin J., Leahy P., Reid M., Adamson K., et al. (2005). Accessing limnological change and variability using fossil diatom assemblages, south-east Australia. River Res. Appl. 21, 257–269. doi: 10.1002/rra.845
Gillson L. (2015). Biodiversity conservation and environmental change: Using palaeoecology to manage dynamic landscapes in the Anthropocene. UK: Oxford University Press.
Gillson L., Biggs H., Smit I. P. J., Virah-Sawmy M., Rogers K. (2019). Finding common ground between adaptive management and evidence-based approaches to biodiversity conservation. Trends Ecol. Evol. 34, 31–44. doi: 10.1016/j.tree.2018.10.003
Gillson L., Duffin K. I. (2007). Thresholds of potential concern as benchmarks in the management of African savannahs. Philos. Trans. R Soc. B Biol. Sci. 362, 309–319. doi: 10.1098/rstb.2006.1988
Gillson L., MacPherson A. J., Hoffman M. T. (2020). Contrasting mechanisms of resilience at mesic and semi-arid boundaries of fynbos, a mega-diverse heathland of South Africa. Ecol. Complex 42, 100827. doi: 10.1016/j.ecocom.2020.100827
Gillson L., Marchant R. (2014). From myopia to clarity: Sharpening the focus of ecosystem management through the lens of palaeoecology. Trends Ecol. Evol. 29, 317–325. doi: 10.1016/j.tree.2014.03.010
Gillson L., Willis K. (2004). “As Earth’s testimonies tell”: wilderness conservation in a changing world. Ecol. Lett. 7, 990–998. doi: 10.1111/j.1461-0248.2004.00658.x
Gil-Romera G., Lopez-Merino L., Carrion J. S., Gonzalez-Samperiz P., Martin-Puertas C., Lopez Saez J. A., et al. (2010). Interpreting resilience through long-term ecology: potential insights in Western Mediterranean landscapes. Open Ecol. J. 3, 43–53. doi: 10.2174/1874213001003020043
Goldblatt P., Manning J. (2000). Plant diversity of the Cape region of southern Africa. Ann. Missouri Bot. Gard. 89, 281–302. doi: 10.2307/3298566
González Sagrario M.d. l. Á., Musazzi S., Córdoba F. E., Mendiolar M., Lami A. (2020). Inferring the occurrence of regime shifts in a shallow lake during the last 250 years based on multiple indicators. Ecol. Indic. 117, 106536. doi: 10.1016/j.ecolind.2020.106536
Grace W., Pope J. (2015). “A systems approach to sustainability assessment,” in Handbook of sustainability assessment (Camberley, UK: Edward Elgar Publishing).
Griggs D., Stafford-Smith M., Gaffney O., Rockström J., Öhman M. C., Shyamsundar P., et al (2013). Sustainable development goals for people and planet. Nature 495(7441):305–7.
Groesser S. N., Schwaninger M. (2012). Contributions to model validation: Hierarchy, process, and cessation. Syst. Dyn. Rev. 28, 157–181. doi: 10.1002/sdr.1466
Haensler A., Hagemann S., Jacob D. (2011). The role of the simulation setup in a long-term high-resolution climate change projection for the southern African region. Theor. Appl. Climatol. 106, 153–169. doi: 10.1007/s00704-011-0420-1
Helfgott A. (2018). Operationalising systemic resilience. Eur. J. Oper. Res. 268, 852–864. doi: 10.1016/j.ejor.2017.11.056
Herrera de Leon H. J., Kopainsky B. (2019). Do you bend or break? System dynamics in resilience planning for food security. Syst. Dyn. Rev. 35, 287–309. doi: 10.1002/sdr.1643
Hoffman M. T., Cramer M. D., Gillson L., Wallace M. (2011). Pan evaporation and wind run decline in the Cape Floristic Region of South Africa, (1974-2005): Implications for vegetation responses to climate change. Clim. Change 109, 437–452. doi: 10.1007/s10584-011-0030-z
Holden P. B., Ziervogel G., Hoffman M. T., New M. G. (2021). Transition from subsistence grazing to nature-based recreation: A nuanced view of land abandonment in a mountain social-ecological system, southwestern Cape, South Africa. Land Use Policy 105, 105429. doi: 10.1016/j.landusepol.2021.105429
Holling C. S. (1973). Resilience and stability of ecological systems. Annu. Rev. Ecol. Syst. 4, 1–23. doi: 10.1146/annurev.es.04.110173.000245
Hossain M. S., Dearing J. A., Eigenbrod F., Johnson F. A. (2017). Operationalizing safe operating space for regional social-ecological systems. Sci. Total Environ. 584–585, 673–682. doi: 10.1016/j.scitotenv.2017.01.095
Hossain M. S., Dearing J. A., Rahman M. M., Salehin M. (2016). Recent changes in ecosystem services and human well-being in the Bangladesh coastal zone. Reg. Environ. Chang. 16, 429–443. doi: 10.1007/s10113-014-0748-z
Inam A., Adamowski J., Halbe J., Prasher S. (2015). Using causal loop diagrams for the initialization of stakeholder engagement in soil salinity management in agricultural watersheds in developing countries: A case study in the Rechna Doab watershed, Pakistan. J. Environ. Manage 152, 251–267. doi: 10.1016/j.jenvman.2015.01.052
Jackson L. C., Wood R. A. (2018). Hysteresis and resilience of the AMOC in an eddy-permitting GCM. Geophys. Res. Lett. 45, 8547–8556. doi: 10.1029/2018GL078104
Jean S., Medema W., Adamowski J., Delaney P., Wals A. (2018). Serious games as a catalyst for boundary crossing, collaboration and knowledge co-creation in a watershed governance context. J. Environ. Manage 223, 1010–1022. doi: 10.1016/j.jenvman.2018.05.021
Jeffers E. S., Nogué S., Willis K. J. (2015). The role of palaeoecological records in assessing ecosystem services. Quat. Sci. Rev. 112, 17–32. doi: 10.1016/j.quascirev.2014.12.018
Kim D. H., Anderson V. (1998). Systems Archetype Basics: From Story to Structure. Massachusetts, USA: Pegasus Communications, Inc.
Kirsten K. L., Forbes C. J., Finch J., Gillson L. (2024). The application of paleoenvironmental research in supporting land management approaches and conservation in south africa. In: von Maltitz G. P., et al Sustainability of Southern African Ecosystems under Global Change. Ecological Studies, vol 248. Springer, Cham. doi: 10.1007/978-3-031-10948-5_12
Knight A. T., Cowling R. M., Rouget M., Balmford A., Lombard A. T., Campbell B. M. (2008). Knowing but not doing: Selecting priority conservation areas and the research-implementation gap. Conserv. Biol. 22, 610–617. doi: 10.1111/j.1523-1739.2008.00914.x
Kopainsky B., Hager G., Herrera H., Nyanga P. H. (2017). Transforming food systems at local levels: Using participatory system dynamics in an interactive manner to refine small-scale farmers’ mental models. Ecol. Modell. 362, 101–110. doi: 10.1016/j.ecolmodel.2017.08.010
Kraaij T., Wilgen B. W. (2014). “Drivers, ecology, and management of fire in fynbos,” in Fynbos: Ecology, evolution, and conservation of a megadiverse region. UK: Oxford University Press.
Lake P. S. (2013). Resistance, resilience and restoration. Ecol. Manag. Restor. 14, 20–24. doi: 10.1111/emr.12016
Langsdale S. (2007). Participatory Model Building for Exploring Water Management and Climate Change in the Okanagan Basin, British Columbia (Canada: University of British Columbia).
Lew A. A., Ng P. T., Ni C., Wu T.-C. (2016). Community sustainability and resilience: similarities, differences and indicators. Tour Geogr. 8 (1), 18–27. doi: 10.1080/14616688.2015.1122664
Low A., Rebelo A. (1996). Vegetation of South Africa, Lesotho and Swaziland: A companion to the vegetation map of South Africa, Lesotho and Swaziland (Pretoria: Department of Environmental Affairs and Tourism).
Ludwig D., Walker B., Holling C. S. (1997). Sustainability, stability, and resilience. Conserv. Ecol. 1 (1), 1–24. doi: 10.5751/ES-00012-010107
Maani K. (2013). Decision-making for climate change adaptation: a systems thinking approach. Australia: National Climate Change Adaptation Research Facility.
Maani K. E., Cavana R. Y. (2007). Systems thinking, system dynamics: Managing change and complexity (North Shore, N.Z.: Pearson Prentice Hall).
MacPherson A. J., Gillson L., Hoffman M. T. (2019). Between- and within-biome resistance and resilience at the fynbos-forest ecotone, South Africa. Holocene 29, 1801–1816. doi: 10.1177/0959683619862046
Mahajan S. L., Glew L., Rieder E., Ahmadia G., Darling E., Fox H. E., et al. (2019). Systems thinking for planning and evaluating conservation interventions. Conserv. Sci. Pract. 1, 1–8. doi: 10.1111/csp2.44
Manning J., Goldblatt P. (2012). Plants of the greater Cape Floristic Region 1: The Core Cape Flora (Pretoria: South African National Biodiversity Institute).
Marchant R., Lane P. (2014). Past perspectives for the future: Foundations for sustainable development in East Africa. J. Archaeol. Sci. 51, 12–21. doi: 10.1016/j.jas.2013.07.005
Marchese D., Reynolds E., Bates M. E., Morgan H., Clark S. S., Linkov I. (2018). Resilience and sustainability: Similarities and differences in environmental management applications. Sci. Total Environ. 613–614, 1275–1283. doi: 10.1016/j.scitotenv.2017.09.086
Martinez-Moyano I. J. (2012). Documentation for model transparency. Syst. Dyn. Rev. 28, 199–208. doi: 10.1002/sdr.1471
Meadows M. E. (2006). Global change and Southern Africa. Geogr. Res. 44, 135–145. doi: 10.1111/j.1745-5871.2006.00375.x
Menendez H. M., Wuellner M. R., Turner B. L., Gates R. N., Dunn B. H., Tedeschi L. O. (2020). A spatial landscape scale approach for estimating erosion, water quantity, and quality in response to South Dakota grassland conversion. Nat. Resour. Model. 33, 1–31. doi: 10.1111/nrm.12243
Midgley S., New M., Spelman S., Parkere K. (2014). The Food-Energy-Water-Land-Biodiversity (FEWLB) Nexus and Local Economic Development in the Berg River Catchment: Framework and Description. South Africa: African Climate and Development Initiative, University of Cape Town.
Millar C. I., Stephenson N. L., Stephens S. L. (2007). Climate change and forests of the future: managing in the face of uncertainty. Ecol. Appl. 17, 2145–2151. doi: 10.1890/06-1715.1
Millennium Ecosystem Assessment (2005). Ecosystems and Human Well-being: Synthesis. Washington, DC, USA: Island Press.
Miller B. W., Symstad A. J., Frid L., Fisichelli N. A., Schuurman G. W. (2017). Co-producing simulation models to inform resource Management: A case study from southwest South Dakota. Ecosphere 8 (12), e02020. doi: 10.1002/ecs2.2020
Mittermeier R., Myers N., Thomsen J., Da Fonseca G., Olivieri S. (1998). Biodiversity hotspots and major tropical wilderness areas: approaches to setting conservation priorities. Conserv. Biol. 12, 516–520. doi: 10.1046/j.1523-1739.1998.012003516.x
Monat J. P., Gannon T. F. (2015). What is systems thinking? A review of selected literature plus recommendations. Am. J. Syst. Sci. 4, 11–26. doi: 10.5923/j.ajss.20150401.02
Mucina L., Rutherford M. C. (2006). Vegetation Atlas of South Africa, Lesotho and Swaziland, Strelitzia. South Africa: South African National Biodiversity Institute, Pretoria.
Myers N., Mittermeier R. A., Mittermeier C. G., Fonseca G. A. B., Kent J. (2000). Biodiversity hotspots for conservation priorities. Nature 403, 853–858. doi: 10.1038/35002501
Olen B., Audouin M. (2007). Strategic Environmental Assessment for the management of Ecosystem Services within the Cape Winelands District Municipal Area. Pretoria, South Africa: Department of Environmental Affairs and Tourism (DEAT).
Oliver T. H., Heard M. S., Isaac N. J. B., Roy D. B., Procter D., Eigenbrod F., et al. (2015). Biodiversity and resilience of ecosystem functions. Trends Ecol. Evol. 30, 673–684. doi: 10.1016/j.tree.2015.08.009
Palmer A. R. (2010). Report on the clearing trial of Pteronia incana (bluebush) and Elytroppappus rhinocerotis (renosterbos) in Mgwalana catchment, Ngqushwa District, Eastern Cape Province: rehabilitating degraded rangeland. Grassroots 10, 22–27.
Pasquini L., Cowling R. M. (2015). Opportunities and challenges for mainstreaming ecosystem-based adaptation in local government: evidence from the Western Cape, South Africa. Environ. Dev. Sustain. 17, 1121–1140. doi: 10.1007/s10668-014-9594-x
Pederson G. T., Gray S. T., Fagre D. B., Graumlich L. J. (2006). Long-duration drought variability and impacts on ecosystem services: A case study from Glacier National Park, Montana. Earth Interact. 10, 1–28. doi: 10.1175/EI153.1
Radloff F. G. T., Mucina L., Snyman D. (2014). The impact of native large herbivores and fire on the vegetation dynamics in the Cape renosterveld shrublands of South Africa: Insights from a six-year field experiment. Appl. Veg. Sci. 17, 456–469. doi: 10.1111/avsc.12086
Rebelo A. G., Boucher C., Helme N., Mucina L., Rutherford M. C. (2006). “Fynbos biome 4,” in The Vegetation of South Africa (Lesotho and Swaziland: South African National Biodiversity Institute Pretoria).
Reed M. S., Evely A. C., Cundill G., Fazey I., Glass J., Laing A., et al. (2010). What is social learning? Ecol. Soc. 15. doi: 10.5751/ES-03564-1504r01
Rehan R., Knight M. A., Haas C. T., Unger A. J. A. (2011). Application of system dynamics for developing financially self-sustaining management policies for water and wastewater systems. Water Res. 45, 4737–4750. doi: 10.1016/j.watres.2011.06.001
Reyers B., Folke C., Moore M. L., Biggs R., Galaz V. (2018). Social-ecological systems insights for navigating the dynamics of the anthropocene. Annu. Rev. Environ. Resour. 43, 267–289. doi: 10.1146/annurev-environ-110615-085349
Rockström J., Steffen W., Noone K., Persson Å., Chapin F. S., Lambin E., et al. (2009). Planetary boundaries: Exploring the safe operating space for humanity. Ecol. Soc. 14, 1–33. doi: 10.5751/ES-03180-140232
Rouget M., Barnett M., Cowling R. M., Cumming T., Daniels F., Hoffman M. T., et al. (2014). “Conserving the Cape Floristic Region,” in Fynbos: ecology, evolution and conservation of a megadiverse region. Eds. Allsopp N., Verboom G. A., Colville J. F. (London: Oxford University Press).
Roux D. J., Nel J. L., Cundill G., O’Farrell P., Fabricius C. (2017). Transdisciplinary research for systemic change: who to learn with, what to learn about and how to learn. Sustain. Sci. 12, 711–726. doi: 10.1007/s11625-017-0446-0
Rupnik R., Kukar M., Vračar P., Košir D., Pevec D., Bosnić Z. (2019). AgroDSS: A decision support system for agriculture and farming. Comput. Electron. Agric. 161, 260–271. doi: 10.1016/j.compag.2018.04.001
Schwaninger M., Groesser S. (2009). “System dynamics modeling: validation for quality assurance,” in Encyclopedia of Complexity and Systems Science. NY, USA: Springer New York.
Simonovic S. P. (2009). A new method for spatial and temporal analysis of risk in water resources management. J. Hydroinformatics 11, 320–329. doi: 10.2166/hydro.2009.018
Slingsby J. A., Ackerly D. D., Latimer A. M., Linder H. P., Pauw A. (2014). The assembly and function of Cape plant communities in a changing world. Fynbos, 200–223. doi: 10.1093/acprof:oso/9780199679584.003.0009
Star S. L., Griesemer J. R. (1989). Institutional ecology, ‘Translations’ and boundary objects: amateurs and professionals in Berkeley’s museum of vertebrate zoology 1907–39. Soc. Stud. Sci. 19 (3), 387–420. doi: 10.1177/030631289019003001
Stave K. A. (2003). A system dynamics model to facilitate public understanding of water management options in Las Vegas, Nevada. J. Environ. Manage 67, 303–313. doi: 10.1016/S0301-4797(02)00205-0
Stave K. A., Kopainsky B. (2015). A system dynamics approach for examining mechanisms and pathways of food supply vulnerability. J. Environ. Stud. Sci. 5, 321–336. doi: 10.1007/s13412-015-0289-x
Sterman J. (2000). Business dynamics: systems thinking and modeling for a complex world (New York, USA: Irwin McGrawHill).
Sterman J. D. (2002). “System dynamics: systems thinking and modeling for a complex world,” in ESD International Symposium.
Tidwell V. C., Passell H. D., Conrad S. H., Thomas R. P. (2004). System dynamics modeling for community-based water planning: Application to the Middle Rio Grande. Aquat. Sci. 66, 357–372. doi: 10.1007/s00027-004-0722-9
Turner K. G., Anderson S., Gonzales-Chang M., Costanza R., Courville S., Dalgaard T., et al. (2016). A review of methods, data, and models to assess changes in the value of ecosystem services from land degradation and restoration. Ecol. Modell. 319, 190–207. doi: 10.1016/j.ecolmodel.2015.07.017
Turner B. L., Kodali S. (2020). Soil system dynamics for learning about complex, feedback-driven agricultural resource problems: model development, evaluation, and sensitivity analysis of biophysical feedbacks. Ecol. Modell. 428, 109050. doi: 10.1016/j.ecolmodel.2020.109050
Turner B. L., Menendez H. M., Gates R., Tedeschi L. O., Atzori A. S. (2016). System dynamics modeling for agricultural and natural resource management issues: Review of some past cases and forecasting future roles. Resources 5, 1–24. doi: 10.3390/resources5040040
Turpie J., Winkler H., Spalding-Fecher R., Midgley G. (2002). Economic impacts of climate change in South Africa: a preliminary analysis of unmitigated damage costs. South Africa: Energy Research Centre, University of Cape Town (UCT) (ERC).
van den Belt M. (2004). Mediated Modeling: A System Dynamics Approach to Environmental Consensus Building (USA: Island press).
van den Belt M., Blake D. (2015). Mediated modeling in water resource dialogues connecting multiple scales. J. Am. Water Resour. Assoc. 51, 1581–1599. doi: 10.1111/1752-1688.12330
Van Wilgen B., Richardson D. M. (1985). The effects of alien shrub invasions on vegetation structure and fire behaviour in South African fynbos shrublands: A simulation study. J. Appl. Ecol. 22, 955–966. doi: 10.2307/2403243
Vermeulen E. A., Clifford-Holmes J. K., Scharler U. M., Lombard A. T. (2022). A system dynamics model to support marine spatial planning in Algoa Bay, South Africa. Environ. Model. Softw. 160 (4), 105601. doi: 10.1016/j.envsoft.2022.105601
Videira N., Antunes P., Santos R., Lopes R. (2010). A participatory modelling approach to support integrated sustainability assessment processes. Syst. Res. Behav. Sci. 27, 446–460. doi: 10.1002/sres.1041
Voinov A., Bousquet F. (2010). Modelling with stakeholders. Environ. Model. Softw. 25, 1268–1281. doi: 10.1016/j.envsoft.2010.03.007
Voinov A., Kolagani N., McCall M. K., Glynn P. D., Kragt M. E., Ostermann F. O., et al. (2016). Modelling with stakeholders - Next generation. Environ. Model. Softw. 77, 196–220. doi: 10.1016/j.envsoft.2015.11.016
Von Loeper W., Musango J., Breny A., Drimie S. (2016). Analysing challenges facing smallholder farmers and conservation agriculture in South Africa: A system dynamics approach. South Afr. J. Econ. Manag. Sci. 19, 747–773. doi: 10.4102/sajems.v19i5.1588
Walker B., Meyers J. A. (2004). Thresholds in ecological and social-ecological systems: A developing database. Ecol. Soc. 9. doi: 10.5751/es-00664-090203
Wang X., Liu Y. Y. (2020). Overcome competitive exclusion in ecosystems. iScience 23, 101009. doi: 10.1016/j.isci.2020.101009
Wang X.-j., Zhang J.-y., Liu J.-f., et al. (2011). Water resources planning and management based on system dynamics: A case study of Yulin city. Environ. Dev. Sustain. 13, 331–351. doi: 10.1007/s10668-010-9264-6
Weiner B. G., Posfai A., Wingreen N. S. (2019). Spatial ecology of territorial populations. Proc. Natl. Acad. Sci. U.S.A. 116, 17874–17879. doi: 10.1073/pnas.1911570116
Weller F., Sherley R. B., Waller L. J., Ludynia K., Geldenhuys D., Shannon L. J., et al. (2016). System dynamics modelling of the Endangered African penguin populations on Dyer and Robben islands, South Africa. Ecol. Modell. 327, 44–56. doi: 10.1016/j.ecolmodel.2016.01.011
Wheeler A., Knight A. T., Difford M., Vetter S. (2019). Ostrich farmer characteristics predict conservation opportunity. S Afr. J. Sci. 115, 1–8. doi: 10.17159/sajs.2019/5540
Wilkerson B., Aguiar A., Gkini C., Czermainski de Oliveira I., Lunde Trellevik L. K., Kopainsky B. (2020). Reflections on adapting group model building scripts into online workshops. Syst. Dyn. Rev. 36, 358–372. doi: 10.1002/sdr.1662
Willis K. J., Birks H. J. B. (2006). What is natural? The need for a long-term perspective in biodiversity conservation. Science (80-) 314, 1261–1265. doi: 10.1126/science.1122667
Keywords: sustainable land management, ecosystem services, multi-stakeholder engagement, threshold behaviour, scenario-thinking, hysteresis
Citation: Forbes CJ, Clifford-Holmes J and Gillson L (2024) A past–present–future lens of environmental change: blending applied paleoecology and participatory system dynamics modeling at a conservation site in the Cape Floristic Region, South Africa. Front. Conserv. Sci. 4:1160043. doi: 10.3389/fcosc.2023.1160043
Received: 06 February 2023; Accepted: 06 September 2023;
Published: 16 January 2024.
Edited by:
Maria Cristina Duarte, University of Lisbon, PortugalReviewed by:
Trevor Hill, University of KwaZulu-Natal, South AfricaSílvia Catarino, University of Lisbon, Portugal
Copyright © 2024 Forbes, Clifford-Holmes and Gillson. This is an open-access article distributed under the terms of the Creative Commons Attribution License (CC BY). The use, distribution or reproduction in other forums is permitted, provided the original author(s) and the copyright owner(s) are credited and that the original publication in this journal is cited, in accordance with accepted academic practice. No use, distribution or reproduction is permitted which does not comply with these terms.
*Correspondence: Cherié Janine Forbes, Y2hlcmllLmouZm9yYmVzQGdtYWlsLmNvbQ==