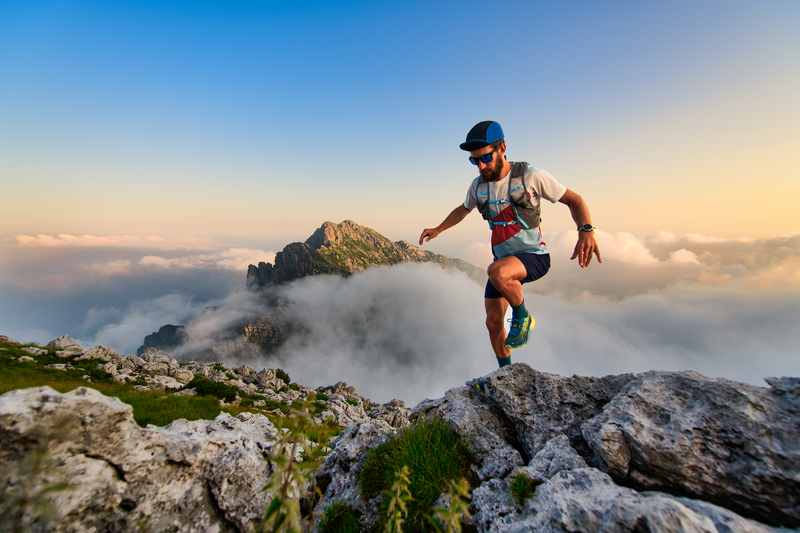
95% of researchers rate our articles as excellent or good
Learn more about the work of our research integrity team to safeguard the quality of each article we publish.
Find out more
ORIGINAL RESEARCH article
Front. Conserv. Sci. , 08 September 2022
Sec. Animal Conservation
Volume 3 - 2022 | https://doi.org/10.3389/fcosc.2022.985545
Exploration behavior can have profound effects on individual fitness. Consequently, knowledge of the proximate mechanisms underpinning exploration behavior may inform conservation breeding programs (CBPs) for threatened species. However, the environmental factors that influence exploration behavior in captivity and during the reintroduction process remain poorly understood. Dietary micronutrients, such as carotenoids, are known to affect the expression of energetically costly behavioral traits, and theoretically may also influence the degree of exploration behavior in various contexts. Here, we investigate whether dietary β-carotene supplementation in captivity influences exploration behavior upon reintroduction to the wild in the critically endangered southern corroboree frog, Pseudophryne corroboree. We conducted a manipulative dietary experiment where captive bred P. corroboree were supplemented with different doses of β-carotene for 40 weeks prior to release. Frogs (n = 115) were reintroduced to the wild using a soft-release approach, where they were released into field enclosures specifically designed for this species. Upon reintroduction, the frogs’ initial exploration behavior was measured using a standardized behavioral assay. There was no effect of diet treatment on any measure of exploration behavior (mean latency to leave the initial refuge, time spent mobile within the release apparatus and latency to disperse into the field enclosure). However, there was a significant relationship between individual body size and latency to leave the refuge, whereby smaller individuals left the refuge more rapidly. While these findings provide no evidence that β-carotene at the dosages tested influences P. corroboree exploration behavior in a reintroduction context, the effect of body size draws attention to the potential for bodily state to influence exploration behavior. We discuss the need for ongoing research investigating the influence of captive diet on post release behavior, and highlight how knowledge concerning state-dependent behavior might help to inform and direct reintroduction programs.
Conservation breeding programs (hereafter CBPs) have been established for many threatened species globally in an attempt to curb current rates of biodiversity loss (Conde et al., 2011). The objectives of many modern CBPs are multifaceted and combine ex-situ and in-situ conservation approaches. Objectives may include: i) the establishment and maintenance of genetically representative captive assurance populations, ii) providing individuals for research, iii) acting as ambassadors for conservation education, and/or iv) breeding or head-starting individuals for reintroduction into the wild (McFadden et al., 2018; Silla & Kouba, 2022). In recent years there has been a shift away from more traditional ‘ark’ approaches and greater emphasis and investment placed on the reintroduction of captive-bred individuals (Gilbert et al., 2017). Despite a growing investment in reintroduction by CBPs globally, their general success continues to be hindered by low post-release survival rates of captive individuals (Fischer and Lindenmayer, 2000; Berger-Tal et al., 2020). Low post-release survival is frequently attributed to captive-bred individuals displaying sub-optimal behavior, such as the inability to effectively evade predators, select suitable habitat, or locate resources (Matthews et al., 2005; Stamps & Swaisgood, 2007). One aspect of an individual’s behavioral phenotype that is likely to have profound effects on post-release survival and reintroduction success is exploration behavior (McDougall et al., 2006; Merrick & Koprowski, 2017; Kelleher et al., 2018). Exploration behavior is commonly defined as an individual’s reaction to a new situation, such as a novel environment or object (Reale et al., 2007). Exploration behavior has been shown to influence fitness across various taxonomic groups through impacts on the discovery and use of refugia (Germano et al., 2017), disease acquisition (Koprivnikar et al., 2012), seasonal dispersal (Dingemanse et al., 2003; van Overveld et al., 2014) and reproductive success (Dingemanse et al., 2004; Both et al., 2005).
When captive-bred individuals are reintroduced into the wild (a novel, unfamiliar environment), how quickly they leave the release point to explore their new surroundings and gather information about their environment may predict reintroduction outcomes (McDougall et al., 2006; Berger-Tal & Saltz, 2014; Kelleher et al., 2018). For instance, increased exploratory behavior post-release has been shown to influence an individual’s likelihood of encountering suitable food resources (Schmitz et al., 2015), habitat and refugia (Germano et al., 2017), and avoiding potential predators (Banks et al., 2002). Critically, exploration behavior has also been shown to positively affect post-release survival in mammals (Banks et al., 2002; Haage et al., 2017) and reptiles (Germano et al., 2017; Allard et al., 2019), suggesting that increased exploration may be a key behavioral determinant of reintroduction success in various taxa (Berger-Tal & Saltz, 2014; Merrick & Koprowski, 2017; Kelleher et al., 2018). However, increased exploratory behavior can impose significant costs (Berger-Tal & Saltz, 2014; May et al., 2016). Exploration behavior can be energetically demanding (Berger-Tal & Saltz, 2014) and in certain contexts might negatively impact reintroduction success by increasing an individual’s exposure to threats such as predators or disease (Haage et al., 2017; Kelleher et al., 2018). Regardless of the direction of the effect, there is growing recognition that knowledge concerning the causes of variation in exploration behavior within and between individuals can help inform CBPs and threatened species management.
Diet represents a ubiquitous environmental factor that can contribute to variation in a wide variety of behavioral traits, including exploration behavior (Carere et al., 2005; Han & Dingemanse, 2015, Krause et al., 2017; Han & Dingemanse, 2017). Recently, research attention has focused on understanding how certain micronutrients might influence exploration behavior, with an emphasis on dietary carotenoids (Rowe et al., 2015; Kelleher et al., 2019). Carotenoids are a group of organic micronutrients that animals can only obtain through their diet, and can be broadly grouped into two main types; carotenes (such as β-carotene and lycopene) and xanthophylls (such as lutein and zeaxanthin) (Svennson & Wong, 2011). Over the past two decades numerous empirical studies have suggested that carotenoids play an important role in animal health and physiological performance, primarily due to their capacity to function as antioxidants (Svennson & Wong, 2011; Koch & Hill, 2018). Most carotenoids can effectively receive electrons, theoretically allowing them to quench and reduce the concentration of reactive oxygen species (ROS) in muscle tissue during periods of strenuous activity, improving physiological performance and endurance (Svennson & Wong, 2011; Koch & Hill, 2018). Indeed, dietary supplementation with carotenoids has been shown to positively influence various energetically costly physiological and behavioral traits, including growth and development (Keogh et al., 2018; McInerney et al., 2019) mating displays and ornamentation (Hill et al., 2002; Arnold et al., 2010) and predator escape response (Silla et al., 2016). Thus, there is reason to think that carotenoids might also positively affect other energetically demanding behavioral traits, such as exploration. Theoretically, carotenoid supplementation might positively influence exploration behavior by providing antioxidant protection to muscle tissue or other organs during periods of exploratory movement (Rowe et al., 2015; Kelleher et al., 2019). At present, however, the effect of dietary carotenoids on exploration behavior has only been investigated by two studies, and the results remain equivocal (Rowe et al., 2015; Kelleher et al., 2019). Rowe et al. (2015) found that supplementation with high doses of xanthophyll carotenoids increased average exploration behavior in captive ducks in a novel environment (Rowe et al., 2015). In contrast a recent study in captive southern corroboree frogs found that supplementation with a mixed carotenoid at different life stages did not influence mean adult exploration behavior, but rather the amount of individual variation in exploration behavior (Kelleher et al., 2019). Considering the scarcity of studies, as well as variation in types of carotenoids used across studies, further work is required to determine how carotenoids influence exploratory behavior. Furthermore, a growing body of evidence suggests that the effects of carotenoids may be highly dose dependent, and also vary depending on the physiological and behavioral traits examined (Keogh et al., 2018; McInerney et al., 2019; McInerney et al., 2020). Thus, in order to significantly advance our understanding of the effect of dietary carotenoids on exploration behavior, additional studies investigating dose responses are required. More broadly, there is also a need to investigate the effect of carotenoids on exploration behavior under various contexts, with a clear gap in knowledge concerning the influence of captive diet on individual behavior at the time of reintroduction (Richardson et al., 2019).
One taxonomic group that would benefit from research investigating the effect of dietary carotenoids on post-release behavior are anuran amphibians. Anuran amphibians are currently the most threatened vertebrate class globally, with an upper estimate of 50% of amphibian species at risk of extinction (IUCN, 2020). Many species are now the subject of long term CBPs, though reintroduction success is often low to moderate, depending on the specific species and its main threats (Tapley et al., 2015; Soorae, 2016). Understanding how captive environmental conditions impact post-release behavior may allow conservation managers to direct the development of beneficial behavioral phenotypes. For instance, diet and micronutrient availability is easily controlled in a captive setting, allowing conservation managers to adjust the micronutrient composition of captive diets to increase the expression of particular behavioral traits (such as exploration behavior) that enhance post release performance and/or survival. The southern corroboree frog (Pseudophryne corroboree) is one of Australia’s most critically endangered anurans, and is endemic to the Snowy Mountain region of Kosciuszko National Park in NSW, Australia. The species has suffered extensive population declines since the 1980s, primarily due to the devastating effects of the virulent pathogen, the amphibian chytrid fungus (Batrachochytrium dendrobatidis, Bd). At present there are less than 30 mature adults remaining in the wild, and the species is the subject of an intensive, multi-institutional CBP (Hunter et al., 2018). Current reintroduction protocols include the release of mature captive-bred individuals into disease-free enclosures situated within their former range. At the beginning of the winter period, P. corroboree individuals enter into a period of hibernation in underground refugia to avoid freezing (Osborne, 1991). Although reintroduction success is yet to be formally quantified, it is logical to presume that individuals that do not find suitable overwintering refugia are unlikely to survive the harsh winter conditions (D. Hunter, personal observation). In regard to predation, although P. corroboree possess bright coloration that is expected to serve an aposematic function, a recent study using model frogs indicated that naïve predators that haven’t been exposed to P. corroboree following local extirpation may attack frogs (Umbers et al., 2020). Thus, in this context where warning coloration is expected to be less effective, individuals that disperse quickly after release can be expected to have a greater chance of finding hibernacula and surviving their first winter post release.
The aim of the present study was to investigate whether dietary β-carotene supplementation influences the initial exploration behavior of captive P. corroboree upon reintroduction to the wild. Specifically, we aimed to determine whether supplementation with β-carotene at different doses influenced exploration behavior, measured as latency to leave the initial refuge, proportion of time spent mobile and latency to disperse into a new environment. To address this aim we conducted a large manipulative laboratory experiment where we reared captive P. corroboree on different doses of dietary β-carotene prior to release. We then quantified initial exploration behavior upon release by conducting a standardized behavioral assay. Based on the assumptions that; (1) carotenoids have the potential to act as antioxidants and can reduce reactive oxygen species and (2) that exploration is an energetically costly behavior (following Kelleher et al., 2019), we predicted that P. corroboree receiving a higher dose of β-carotene would be more exploratory upon release (i.e., disperse more quickly and have greater mobility).
The southern corroboree frog (Pseudophryne corroboree) is a small (23- 30 mm snout-vent length) terrestrial anuran from the family Myobatrachidae (Osborne, 1991). Pseudophryne corroboree is an alpine species that is endemic to the Snowy Mountain region of Kosciuszko National Park in NSW, Australia, and its distribution is highly restricted to areas above 1300 m in elevation (Hunter et al., 2018). The species is distinguished by striking black and bright yellow longitudinal stripes located on its dorsal surface (Figure 1A). This coloration appears to function as an aposematic warning signal to predators (Umbers et al., 2020), as the skin of both males and females contains toxic lipophilic alkaloids (pumiliotoxins and pseudophrynamines; Daly et al., 2005). P. corroboree breeds annually during the Austral summer months (December – February) and undergoes a period of hibernation during the winter months (June-August) (Osborne, 1991). The diet of P. corroboree consists of silt and algae consumed during the larval life stage, and invertebrates (such as ants and crickets) consumed post-metamorphosis (Osborne, 1991), all of which naturally contain carotenoids (Lichtenthaler, 1987; Eeva et al., 2010). The species is currently listed as critically endangered by the Australian government at both the state and federal level, and by the International Union for the Conservation of Nature (IUCN) (Hunter et al., 2018) and has been the subject of an intensive, multi-institutional CBP since 2003 (McFadden et al., 2013).
Figure 1 (A) Adult Southern Corroboree frog, Pseudophryne corroboree, (B) Soft-release field enclosures located in Kosciuszko National Park, NSW, and (C) video recording set-up and release apparatus within a field enclosure. Photograph A is courtesy of A.J. Silla, photographs B and C are courtesy of P.G. Byrne.
Pseudophryne corroboree used in this study were bred and reared through to metamorphosis at Taronga Zoo, Sydney Australia, as part of the CBP for this species. Frogs were from eight distinct clutches produced by different parents. On the 15th of January 2019, the frogs were transported from Taronga Zoo to the Ecological Research Center (ERC) at the University of Wollongong (UOW). Immediately upon arrival at the ERC, frogs were placed into individual housing containers (21 cm L x 12 cm W x 12 cm H), in which they remained for the duration of the study. Each individual was randomly assigned to a diet treatment (see Section 2.3 below for details), with clutch (clutch A, B, C, D, E, F, G, H) evenly represented among treatments to control for any potential genetic effects. At the commencement of the study period, frogs were 3 to 4 months old post-metamorphosis, and weighed between 0.394 and 1.284 grams (mean ± SEM = 0.895 ± 0.014). As P. corroboree do not reach sexual maturity until 3-4 years post-metamorphosis, the sex of individuals was not determined during the study.
To determine whether β-carotene influences the exploration behavior of P. corroboree upon reintroduction to the wild, individuals (n = 115) were first assigned to one of four experimental diet treatments which differed in β-carotene concentration. These diet treatments were: T0 (a control diet; 0mg/g of β-carotene, n = 30), T1 (1 mg/g of β-carotene, n = 31), T2 (2 mg/g of β-carotene, n = 28) and T3 (3 mg/g of β-carotene, n = 26) (see section 2.4 below for further details). β-carotene is a carotene carotenoid (Svennson & Wong, 2011), and was selected as it is one of the main carotenoids found in captive P. corroboree skin (Byrne and Silla, unpublished data). β-carotene is also present in the natural diet of P. corroboree at both life stages, found in algae consumed during the larval life stage, and invertebrates consumed post-metamorphosis (Osborne, 1991). The three specific experimental treatment concentrations (1mg/g, 2mg/g and 3mg/g) were chosen based on the findings of previous studies investigating the effect of β-carotene supplementation on fitness-determining traits in P. corroboree and the Australian Booroolong frog (Litoria booroolongensis) (Keogh et al., 2018; McInerney et al., 2019). These studies investigated the effect of β-carotene supplementation at doses of 0.1mg/g, 1mg/g (Keogh et al., 2018; McInerney et al., 2019) and 10 mg/g (Keogh et al., 2018), with 1mg/g identified as the optimal dose in both studies. Individuals were maintained in captivity on experimental diet treatments for 40 weeks. After this time, P. corroboree were reintroduced into one of four disease-free enclosures (Figures 1B, C) located within Kosciuszko National Park, NSW, Australia. Each diet treatment was equally represented within each field enclosure (see section 2.6) and exploration behavior was quantified at the point of release using a standardized behavioral assay (see sections 2.4, 2.5 and 2.6).
Throughout the experimental period, frogs were kept inside a temperature-controlled room, maintained at 20°C according to husbandry conditions described previously (Walton et al., 2021). Frogs were individually housed in rectangular plastic aquaria (21cm L x 12 cm W x 12cm H) which contained a 2 cm high base layer of aquarium gravel and a layer of sphagnum moss (Sphagnum cristatum; Brunnings, Australia). Each enclosure contained a small piece of PVC plastic tube (5.7 cm L, 4.4 cm D) in one corner to provide individuals with a covered, dry refuge area. Containers were flushed twice weekly with reverse-osmosis (R.O.) water, and sphagnum moss was changed once a fortnight to remove nitrogenous waste and detritus. UV-B lighting was provided from two UV-B light bulbs (Reptisun 10.0 T5 High Output 36” bulb; Pet Pacific, Australia) that were suspended approximately 20 cm above the enclosures. UV-B lights were connected to an automatic timer which was set to a 9 h:15 h day/night light cycle. Natural, ambient light was also provided by a nearby window, providing the frogs with a seasonal photoperiod. Following arrival at the ERC, all individuals were initially fed a standard basal diet (Acheta domestica crickets) for a period of five weeks (irrespective of their assigned diet treatment) that contained a negligible amount of carotenoids (Silla et al., 2016), while they acclimatized to the new housing conditions and husbandry protocols. Experimental diets (T0, T1, T2 and T3) commenced on the 15th of March, 2020. Diets were prepared by dusting a standardized weight of Acheta domestica crickets (15 g) with one of four diet treatment powders. Each treatment powder contained a known concentration of β-carotene powder (T0: 0 mg/g, T1: 1 mg/g, T2: 2 mg/g, T3: 3 mg/g), cellulose microcrystalline powder and 0.25 g of calcium powder (Repti-Cal, Aristopet, Melbourne, Australia) (see Table 1 in Walton et al., 2021 for a breakdown of experimental diets). Cellulose powder was used as a bulking agent to standardize diet quantity across treatments and is commonly used in diet experiments as it has no known nutritional value (Dias et al., 1998; Deng et al., 2006). Basal and experimental diets were supplemented with calcium powder to prevent frogs from developing disorders associated with calcium deficiencies that are common in captive amphibians (Ferrie et al., 2014). Frogs were fed 10-15 dusted Acheta domestica crickets (7-10 days old) twice weekly (Mondays and Fridays) to eat ad libitum for the duration of the study.
To determine whether β-carotene influences exploration behavior upon reintroduction, P. corroboree were released into four circular field enclosures that were purpose built for releasing this species into a disease-free environment. The enclosures were located within the former range of P. corroboree, at 1500 meters above sea level in the sub-alpine region of Kosciuszko National Park, NSW Australia. The ‘disease free’ status of the enclosures was achieved by excluding other frogs (particularly the common eastern froglet Crinia signifera) that are a reservoir host for the amphibian chytrid fungus (Batrachochytrium dendrobatidis). The exact location details of the field site are withheld due to conservation security reasons. Each circular enclosure was a 90 cm high galvanized corrugated iron fence that was six meters in diameter and dug 30 cm into the ground. Each enclosure contained established vegetation, predominately the tussock grass Poa sieberiana. Ten wooden planks of different sizes where randomly placed in each enclosure to provide a refuge area for the frogs, and a suitable structure to encourage ant colonies, which are the primary diet of P. corroboree. As all enclosures contained large quantities of ants and other small invertebrates, no supplementary feeding was required. Two areas within each enclosure (approximately 80 cm × 200 cm) were established as moist refuges. This was achieved by excavating to a depth of 15 cm an area approximately 80cm by 200cm that was then lined with rubber matting and filled with scoria gravel and covered with wooden planks. We conducted the release in Austral summer (December), in line with the current corroboree frog reintroduction protocol. Adult P. corroboree are released during the spring and summer months as there is an abundance of invertebrate prey species during this time, and P. corroboree hibernate during winter.
Prior to arrival at the field site, individuals from each diet treatment (T0, T1, T2 and T3) were assigned to a field enclosure (1, 2, 3 or 4). Individuals from each treatment were assigned to a field enclosure based on their pre-release body weight (weight as of the 5th December 2019). This ensured that each diet treatment was equally represented within each field enclosure, and that there was no significant difference between enclosures in either the mean body weight of frogs (ANOVA: F3,111 = 0.1365, p = 0.9381) or the variance in body weight (Levene’s test: F3,111 = 0.1724, p = 0.9149). Total sample sizes for each of the field enclosures were as follows: enclosure 1: n = 29, enclosure 2: n = 29, enclosure 3: n = 28, enclosure 4: n = 29. At the time of reintroduction, frogs were approximately 12 months post metamorphosis and weighed between 1.625 and 3.309 grams (mean ± SEM = 2.415 ± 0.03 g).
On the 17th of December 2019, P. corroboree (n = 115) were transported from the ERC at UOW to the reintroduction site in Kosciuszko National Park. Frogs were transported from UOW in large, rectangular plastic pal pens (31.9cm x 17.8 cm) in groups of 5 to 12 frogs per container. Frogs were kept in their treatment groups during transport. Transport containers were filled with a deep layer of sphagnum moss (Brunnings, Australia), which provided frogs with a safe, secure refuge, to bury down in whilst being transported. Upon arrival in Kosciuszko National Park, frogs were transported into the reintroduction site by helicopter. The transport containers were covered with black opaque plastic during transit to further limit any potential disturbance (and stress) to the frogs. Upon arrival at the field site, the transport containers were placed in a shady location, and the frogs were left undisturbed for a period of one hour. Following the one-hour initial acclimation after arrival at the site, we released captive P. corroboree into one of the four field enclosures. To quantify exploration behavior immediately upon release, we conducted a standardized behavioral assay. Within each field enclosure (enclosure 1-4), assays were conducted in three blocks, with 12 frogs released simultaneously in block 1, 12 frogs released simultaneously in block 2, and the remaining 4-5 frogs released simultaneously in block 3. Diet treatments were equally represented among each testing block to control for any potential order effects. Behavioral assays were conducted in daylight between 15:00h and 17:00h as P. corroboree is diurnally active.
To conduct a standardized behavioral assay, we designed a release apparatus that consisted of a piece of transparent aquarium tube (46 cm L), which was closed with an opaque cap at one end and open at the other. We created a standardized initial starting refuge, by placing a piece of sphagnum moss from the frog’s transport container into the enclosed end of the release apparatus. This design ensured that each individual started the assay in a familiar refuge from which they could freely leave and disperse into their new environment (similar to a captive novel environment test used to measure exploration behavior, see Kelleher et al., 2017; Kelleher et al., 2019). As with captive novel environment tests, this approach ensured that the behavior observed was ‘free’ exploration behavior, as opposed to ‘forced’ exploration behavior (Carter et al., 2013; Kelleher et al., 2017; Kelleher et al., 2019). The release apparatus was positioned so that the open end of the tube was facing north, into the center of the field enclosure. This allowed us to standardize the direction of each individual release, and control for any potential directional or orientation biases, which may impact the immediate dispersal behavior of each individual.
To begin a reintroduction behavioral assay, we carefully placed a focal frog on top of the sphagnum moss at the enclosed end of the release apparatus, and, to ensure that the frog remained in the moss, placed the tube in an upright, vertical position for an acclimation period of five minutes. After the five-minute acclimation period had ended, an observer carefully lowered each tube until it lay in a horizontal position along the ground, allowing the frog to freely exit the moss refuge and move along the tube towards the opening. Frogs that made it to the end of a tube were then free to disperse into the field enclosure. After all tubes within the testing block were lowered (which took less than 10 seconds) the observer moved several meters behind the tubes and cameras so as not to disturb the frogs. Neighboring tubes within a testing block were placed 15-20 cm apart from each other in a parallel position, and long tussock grass within the field enclosures prevented neighboring frogs from seeing or interacting with each other until they exited the apparatus. Reintroduction behavioral assays were conducted for a period of 10 minutes. Assays were video recorded using a high-definition video camera (Sony FDR-AX53 4K Camera) that was attached to a tripod and positioned approximately two meters behind the release apparatus. At the end of the 10 minute trial period, any frogs that remained in the release apparatus were removed and gently released into the enclosure. The release tubes were wiped with ethanol and rinsed with reverse-osmosis (R.O.) water between every trial (including the first trial) to remove the potential for chemical signals released by frogs to influence the behavior of frogs in subsequent trials. All videos were rewatched at a later date and analyzed using the behavioral analysis software JWatcher Version 1.0 (Blumstein et al., 2000). For each frog we measured three variables as indicators of exploration behavior: (1) latency to leave the moss refuge (seconds), (2) the proportion of time spent mobile while in the release apparatus and (3) latency to disperse into the field enclosure (i.e., completely leave the release apparatus) (seconds). Individuals that did not leave the moss refuge, or disperse into the ring enclosure received the maximum latency time of 600 seconds. Trial times commenced as soon as the release apparatus was laid horizontally along the ground. Mobility was defined as any intentional locomotory movement, including head movement, and a frog was considered immobile if it stopped moving for longer than 3 seconds (following Kelleher et al., 2017). Individuals that had shorter latency times, and spent a greater proportion of time mobile when inside the release apparatus were considered to be more exploratory.
To determine the effect of diet treatment on exploration behavior upon reintroduction, we used linear mixed effects models (LMM) with Gaussian error distribution and restricted maximum likelihood (REML) parameter estimation, using the function lme in the R package lme4 version 1.1-21 (Bates et al., 2015). We constructed three separate LMM’s for each response variable: (1) latency to leave refuge, (2) proportion of time spent mobile and (3) latency to disperse. For each model, diet treatment (T0, T1, T2 and T3) and clutch (A, B, C, D, E, F, G, H) were entered as fixed categorical variables, while body size (grams) was entered as a continuous variable. Field enclosure ID (1-4) was entered as a random effect. Prior to analysis, all variables were transformed to improve normality; latency to leave the refuge and latency to disperse were log transformed and proportion of time spent mobile was arcsine (asin(sqrt[x])) transformed. For each model, we assessed normality by visual inspection of normal quantile plots, and assessed model fit by visually examining diagnostic plots of residual vs. fitted values using base R plotting. The significance (p-values) of fixed effects was obtained using Wald tests, using the function ‘Anova’ in the package car. Parameter estimates and 95% confidence intervals for all fixed effects are reported in the Supplementary Material. All statistical analyses were conducted in R Version 1.1.463 (R Core Team, 2018).
All procedures outlined in the present study were assessed and approved by the University of Wollongong Animal Ethics Committee (Protocol Number AE18/15) and authorized by the New South Wales National Parks and Wildlife Service (License Number SL102197). Reintroductions were carried out in accordance with the national corroboree frog recovery plan.
Across the four diet treatment groups, latency to leave the moss refuge ranged from 0.195 to 600 seconds (mean ± SEM = 121.836 ± 16.286). We found no significant effect of diet treatment on latency to leave the refuge (LMM: = 4.010, p = 0.260, Figure 2; Supplementary Table 1). There was also no effect of clutch on latency to leave the refuge (LMM: = 13.885, p = 0.053, Supplementary Table 1). However, there was a significant effect of body weight on latency to leave the refuge (LMM: = 5.225, p = 0.022, Supplementary Table 1), whereby smaller individuals had a shorter latency time and left the moss refuge sooner (Figure 3).
Figure 2 The effect of diet treatment (T0, T1, T2 and T3) on (A) latency to leave the refuge (seconds), (B) proportion of time spent mobile in release apparatus and (C) latency to disperse into field enclosure (seconds) in adult P. corroboree. The box plots show the median, 25th and 75th percentiles, the whiskers indicate values within 1.5 times the interquartile range. The cross represents the mean value and the circles are outliers. Data shown are untransformed. Diet treatments were: T0 (0mg/g β-carotene, n = 30), T1 (1mg/g β-carotene n = 31), T2 (2mg/g β-carotene n = 28) and T3 (3mg/g β-carotene, n = 26).
Figure 3 The relationship between body size (grams) and latency to leave the moss refuge (seconds) in adult P. corroboree (n = 115). Data shown are untransformed.
Across all four diet treatment groups, the proportion of time spent mobile by individual frogs within the release apparatus ranged from 0 to 0.987 (mean ± SEM = 0.545 ± 0.025). There was no significant effect of dietary treatment on the proportion of time spent mobile in the release apparatus (LMM: = 1.830, p = 0.609, Figure 2; Supplementary Table 2). Similarly, there was also no effect of clutch (LMM: = 6.627, p = 0.469, Supplementary Table 2) or body weight (LMM: = 0.077, p = 0.782, Supplementary Table 2) on the proportion of time spent mobile within the release apparatus.
Across all diet treatment groups, latency to disperse into the field enclosure ranged from 18.9 to 600 seconds (mean ± SEM = 382.452 ± 20.736). There was no significant effect of dietary treatment on latency to disperse (LMM: = 2.935, p = 0.402, Figure 2; Supplementary Table 3). Likewise, there was no significant effect of clutch (LMM: = 9.739, p = 0.204, Supplementary Table 3), or body weight (LMM: = 0.808, p = 0.369, Supplementary Table 3) on latency to disperse.
A growing number of studies suggest that exploration behavior can be an important determinant of post-release fitness, yet knowledge regarding the proximate mechanisms underpinning variation in this trait remains limited. In particular, we know very little about how environmental conditions in captivity, such as diet, influence exploratory behavior in a reintroduction context. Here, we aimed to determine whether supplementation with dietary β-carotene in captivity influenced exploration behavior at the time of release in the critically endangered southern corroboree frog, P. corroboree. This was achieved by conducting a manipulative dietary experiment where individual diets were supplemented with different concentrations of β-carotene for an extended period (40 weeks) prior to release. Assuming that exploration behavior is energetically demanding (Kelleher et al., 2019), and that β-carotene reduces oxidative stress and improves physiological functioning and exercise performance (Ogilvy & Preziosi, 2012), we predicted that individuals receiving a higher dose of β-carotene would be more exploratory upon release. Contrary to this prediction, our results showed there was no effect of diet treatment on any of the response variables measured (mean latency to leave the initial starting refuge, the proportion of time spent mobile in the release apparatus, or the latency to disperse into the release environment). Interestingly however, there was a significant effect of body size on latency to leave the refuge, whereby smaller individuals left the refuge more rapidly.
Our findings provide no evidence that dietary β-carotene improves exercise performance and promotes exploration behavior in P. corroboree. This was unexpected because our past dietary studies with P. corroboree have demonstrated that a mixed carotenoid dietary supplement (administered at a comparable dose to the present study, 1-1.5 mg/g) improved P. corroboree escape response performance and exercise endurance during both aquatic and terrestrial escape response trials (Silla et al., 2016; McInerney et al., 2017). The discrepancy in findings between our lab studies and present field study may have arisen because complex environmental conditions at the release site elicited more variable behavioral responses, regardless of an individual’s diet and physiological state. This may have occurred, for example, if individuals were subjected to various combinations of unfamiliar visual, acoustic and olfactory stimuli that differentially affected decision making and inflated variation in movement behavior (for a review of extrinsic factors contributing to individual differences in movement behavior see Shaw, 2020). Alternatively, the methods we employed in the present study may have limited our capacity to detect treatment effects.
Positive effects of carotenoids may depend on the type of carotenoid administered (McInerney et al., 2019). It is certainly possible that β-carotene has no or little positive effect on exercise performance in P. corroboree, with our earlier finding that frogs benefit from consuming a mixed carotenoid supplement possibly linked to the consumption of other types of carotenoids (e.g lutein or zeaxanthin). In support of this notion, McInerney et al. (2020) tested the effect of β-carotene at low and moderate doses (0.1 mg g-1 and 1 mg g-1) on larval P. corroboree burst speed and distance travelled in escape response trials, and found no evidence for enhanced performance. It is possible that P. corroboree may be unable to process and utilize β-carotene as effectively as other carotenoids. However, this seems unlikely, because we have previously shown that β-carotene administered at 1 mg g-1 expedited developmental rate in P. corroboree, whereby larvae consuming β-carotene metamorphosed sooner (McInerney et al., 2019). Given this result, an alternative reason why we did not detect a positive effect of β-carotene on exploration behavior might be that there is a sensitive phase in development during which access to dietary carotenoids has a disproportionate effect on the expression of behavior (Noguera et al., 2015; Kelleher et al., 2019; McDermott & Safran, 2021). Individuals were only exposed to β-carotene following metamorphosis, so the possibility exists that β-carotene could have an effect on behavior if supplied during the larval life stage. Such life stage-dependent effects of dietary carotenoids (including β-carotene) on trait expression have been reported previously in frogs (see Keogh et al., 2018; Kelleher et al., 2019). For instance, our previous laboratory study in P. corroboree found that the availability of a mixed carotenoid supplement during the larval life stage affected the amount of among-individual variance in adult exploration behavior, suggesting that nutritional conditions experienced early in life can have long-term effects on the development of adult behavioral phenotypes (Kelleher et al., 2019). With this in mind, testing for life stage dependent effects (as well as testing different doses and carotenoid types) would be a valuable avenue for future research.
Another alternative explanation to consider is that β-carotene was preferentially invested in other traits (sensu the “carotenoid trade off hypothesis”) (Lin et al., 2010; Koch et al., 2019). If access to carotenoids is limited (e.g. because the doses used were too low), individuals may have strategically allocated β-carotene to traits that have a greater impact on fitness, such as growth or immunity (Baeta et al., 2008). Moving forward, it would be valuable to test the effect of β-carotene on exploration behavior after supplementing diets with doses exceeding those tested in the present study. Saying this, we caution against administering β-carotene at extremely high doses because this could potentially cause harm. In a study of growth and development in the Booroolong frog (L. booroolongensis), Keogh et al. (2018) reported that β-carotene administered at 1mg/g had a positive effect on growth and development, but when administered at 10mg/g had negative effects. In the absence of any beneficial effects of β-carotene on exploration behavior at higher doses, research attention should turn towards testing the effects of mixed carotenoid supplements on exploration behavior at the time of release. We make this recommendation given that dietary supplements containing carotenoid mixes have been found to improve P. corroboree physiological performance under controlled lab conditions (Silla et al., 2016; McInerney et al., 2017).
An alternative reason why we failed to detect any effect of dietary β-carotene on post-release exploration behavior may relate to the approach we used to assay exploration behavior. In principle, dietary carotenoids are predicted to improve performance by acting as antioxidants and mitigating oxidative damage when there is a significant increase in reactive oxygen species production during intense or prolonged physical activity (Arnold et al., 2010). If assays fail to sufficiently challenge test subjects, benefits of supplementation might go undetected. For example, in budgerigars (Melopsittacus undulatus) benefits of antioxidants on flight performance were only detected for the most strenuous exercise tests over an eight-week period (Arnold et al., 2010). In the present study, frogs were only monitored for a very brief period (10 minutes) at the time of release. During this brief novelty-based test, variation between individuals in exploration behavior may have been largely underpinned by cognitive differences that influence behavioral responses to novelty (e.g. fear and spatial neophobia), which resulted in a lack of difference in mean exploration behavior between diet treatment groups (Carter et al., 2013; Greggor et al., 2015). Arguably, it might have been more informative to quantify the effect of β-carotene on exploration behavior over a longer time frame, such as distance travelled throughout the field enclosure over several hours or days. Moreover, it may also be beneficial to take repeated measures of exploration behavior post release; as our previous study in a different captive population of P. corroboree found that the availability of a mixed carotenoid supplement (at different life stages) influenced individual variation in adult exploration behavior in a novel environment test (both at the among- and within-individual level), without affecting the mean behavioral phenotype of diet treatment groups (Kelleher et al., 2019). Therefore, the possibility remains that variation in dietary β-carotene may also influence exploration behavior in a reintroduction context but at the among- or within- individual level; with any treatment effects not detectable at the population level, as measured in the present study (Han & Dingemanse, 2017; Kelleher et al., 2019). In future studies, one approach to quantify individual variation in movement behavior post release might be to fit field enclosures with a system of motion-sensor video cameras and use the unique dorsal patterns displayed by P. corroboree to quantify individual variation in exploration behavior. This approach would also allow the survival of individuals to be determined, allowing investigation into relationships between antioxidant intake and viability, and between exploration behavior and survival. Until further assays have been performed, we cannot rule out the possibility that dietary β-carotene influences P. corroboree exploration behavior in a reintroduction context.
Despite finding no evidence that β-carotene influenced exploration behavior, we found a relationship between body size and latency to leave the initial refuge, whereby smaller frogs left the moss refuge more rapidly. This finding is in line with ‘state-dependent behavioral models’ which propose that body size is an intrinsic state variable that can influence the costs and benefits of different behavioral decisions (Dingemanse & Wolf, 2010; Nyqvist et al., 2012; Sih et al., 2015; Kelleher et al., 2017). There may be several explanations for the relationship we observed. One possibility is that smaller individuals are less capable of tolerating reductions in activity due to smaller energy reserves and a greater risk of starvation (Preisser & Orrock, 2012). If so, smaller individuals may have been more motivated to leave the refuge to forage. Smaller frogs may also have been less risk averse. Body size can have strong effects on an individual’s vulnerability to predation, and there is evidence within species that smaller individuals are less susceptible to predation and less sensitive to perceived predation risk (Stankowich and Blumstein, 2005). In various species, smaller individuals can be harder for predators to detect, and predators may also preferentially target larger prey because they are more profitable (Stankowich and Blumstein, 2005; Preisser & Orrock, 2012). Smaller individuals may also be able to more effectively evade predators because they can more rapidly accelerate and manoeuvre, reflecting scaling related changes in power: mass ratios (Stankowich and Blumstein, 2005; Preisser & Orrock, 2012). Therefore, in our release context, smaller less vulnerable individuals may have been more willing to take risks to leave the familiar refuge and explore the surrounding environment. It should be noted, however, that arguments can also be made for body size having the opposite effect on exploration behavior. Larger individuals might be more motivated to explore because they have a higher metabolic rate and higher energy requirements (Riemer et al., 2018). Moreover, larger individuals may be less susceptible to predation (and less risk averse) either because they possess better developed anti-predatory defenses (Stankowich and Blumstein, 2005), or because predators are more likely to target smaller individuals that are easier to consume (Preisser & Orrock, 2012). In the absence of in-depth knowledge regarding relationships between body size and fear responses in P. corroboree, an alternative explanation for small frogs leaving the refuge sooner is that the frogs were sensitive to abiotic conditions within the refuge, with the speed of response being body-size dependent. In anuran amphibians, smaller individuals can be more sensitive to heat stress and desiccation (Tracy et al., 2010). If this is the case for P. corroboree, smaller individuals may have left the refuge sooner to avoid physiological costs arising from suboptimal conditions (Martin & Lopez, 1999).
Relationships between body size and exploration behavior have been reported previously for P. corroboree. Working with a different captive population to that studied here, Kelleher et al. (2017) reported that larger individuals were more exploratory. Body size explained 40% of the between-individual variance in exploration behavior, with larger frogs exhibiting greater mobility and travelling further in a novel environment (Kelleher et al., 2017). Given we found that body size had no effect on either time spent moving or latency to disperse into the release enclosure, there is reason to suspect that the effect of body size on certain measures of exploration behavior in P. corroboree is context dependent. It is unclear why larger frogs were more exploratory than smaller frogs in the captive environment in the previous study, but not during release in the present study. Presumably the costs and benefits of movement and exploration behavior change in different contexts (e.g. social versus nonsocial) and environments (e.g. captivity versus the wild), as reported for other species (van Oers et al., 2005). Regardless of the reason, these differences emphasize the complexity of animal behavior, and the benefit of studying individual differences in behavior across various contexts. From a conservation perspective, our findings are insightful because they suggest that managers might be able to manipulate individual behavioral responses at reintroduction by releasing frogs at certain body sizes or life stages. For instance, if smaller frogs are more exploratory and disperse more rapidly it may be beneficial to release frogs soon after metamorphosis. This approach might increase the probability that frogs quickly find suitable habitat and thrive. Such associations have been reported for other taxa (Banks et al., 2002; Haage et al., 2017; Germano et al., 2017; Allard et al., 2019). Saying this, there may be tradeoffs to consider because body size and/or life stage might influence prospects of survival in several different ways. For instance, larger individuals with greater fat reserves might have a higher probability of over winter survival (Valenzuela-Sánchez et al., 2015), and older frogs might have stronger warning coloration and be less susceptible to predation (Walton et al., 2021). Clearly, it is too early to make clear recommendations to managers and more work is needed to understand the costs and benefits of releasing frogs at different body sizes and/or life stages. With the goal of optimizing release strategies for southern corroboree frogs, this research should be prioritized.
More broadly, to the best of our knowledge the present study is the first to explore whether supplying dietary carotenoids in captivity can influence behavior upon reintroduction. While we didn’t find any evidence for a beneficial effect of carotenoid consumption, this may have been related to the type of carotenoid or life stage tested, the dose used or aspects of the behavioral assay employed. As such, we strongly encourage more work in this area, particularly given the widespread evidence for positive effects of carotenoid consumption on vertebrate performance and fitness (Richardson et al., 2019). Work of this nature will directly align with the recent push to better integrate animal behavior into conservation strategies (Berger-Tal et al., 2011; Greggor et al., 2019). Beyond studying how manipulating behavioral phenotypes in captivity can improve post release performance and survival (e.g. Shier and Owings, 2006; Vargas and Anderson, 1999; Beck et al., 2002; Richardson et al., 2019), there are numerous ways that behavioral research stands to aid conservation efforts. Most notably, behavioral research can help conservation managers to; (1) better understand how well animals acclimate to captive environments (Clubb & Mason, 2007; Yamanashi et al., 2016) (2) select optimal behavioral types and sex ratios for captive breeding and reintroduction (Robertson et al., 2006; Kelleher et al., 2018), (3) more effectively control invasive species (Hurley et al., 2015) and (4) predict individual and population level responses to agents of environmental change, including habitat fragmentation, habitat pollution, and the introduction of invasive predators and pathogens (Greggor et al., 2016; Adams et al., 2019; Berger-Tal et al., 2019). Behavioral studies can also help identify the optimal composition of social groups for translocation and help evaluate the success of reintroduction efforts (Berger-Tal et al., 2011). Harnessing evolutionary theory to build a robust conceptual framework, continued integration of animal behavior research into conservation stands to inform and vastly improve the success of in-situ and ex-situ conservation management programs globally.
The raw data supporting the conclusions of this article will be made available by the authors, without undue reservation.
The animal study was reviewed and approved by The University of Wollongong Animal Ethics Committee (Protocol Number AE18/15).
PB and AS conceived, designed and prepared the study (including submission and approval of the scientific license and animal ethics application), with input from SK, MM, and DH. MM bred the frogs and reared the embryos, and PB, SK, and AS reared the experimental frogs. PB, SK, MM, and DH released the frogs with help from volunteers. SK analyzed the video recordings and conducted the statistical analyses, with input from PB. PB wrote the abstract and discussion and SK wrote the introduction, methods and results, with input from co-authors. All authors contributed to the article and approved the submitted version.
The study was funded by the Australian Research Council (Linkage Grant LP170100351) awarded to PB, AS, and DH, NSW Environmental Trust (grant no. 2017/RD/0138), and the University of Wollongong SMAH Small Project Grant (262 27 0976) awarded to PB and AS.
The authors would like to acknowledge the Wolgalu People of the Gurmal Nation as the traditional custodians and cultural knowledge holders of the Southern Snowy Mountains, the land on which this research was conducted. We thank Emma McInerney, James Clarke, and Sara Walton for assistance with captive husbandry and data collection in the field. We also thank the herpetofauna staff at Taronga Zoo for providing the southern corroboree frog juveniles that were reared at the University of Wollongong during the present study. Research was funded by grants from the University of Wollongong Research Council, NSW Environmental Trust (grant no. 2017/RD/0138), and the Australian Research Council (grant no. LP170100351).
The authors declare that the research was conducted in the absence of any commercial or financial relationships that could be construed as a potential conflict of interest.
All claims expressed in this article are solely those of the authors and do not necessarily represent those of their affiliated organizations, or those of the publisher, the editors and the reviewers. Any product that may be evaluated in this article, or claim that may be made by its manufacturer, is not guaranteed or endorsed by the publisher.
The Supplementary Material for this article can be found online at: https://www.frontiersin.org/articles/10.3389/fcosc.2022.985545/full#supplementary-material
Adams C. A., Blumenthal A., Fernández-Juricic E., Bayne E., St. Clair C. C. (2019). Effect of anthropogenic light on bird movement, habitat selection and distribution: A systematic map protocol. Environ. Evid. 8, 1–16. doi: 10.1186/s13750-019-0155-5
Allard S., Fuller G., Torgerson-White L., Starking M. D., Yoder-Nowak T. (2019). Personality in zoo-hatched blanding’s turtles affects behavior and survival after reintroduction into the wild. Front. Psychol. 10, 2324. doi: 10.3389/fpsyg.2019.02324
Arnold K. E., Larcombe S. D., Ducaroir L., Alexander L.. (2010). Antioxidant status, flight performance and sexual signalling in wild-type parrots. Behav. Ecol. Sociobiol. 64, 1857–1866. doi: 10.1007/s00265-010-0997-x
Baeta R., Faivre B., Motreuil S., Gaillard M., Moreau J. (2008). Carotenoid trade-off between parasitic resistance and sexual display: An experimental study in the blackbird (Turdus merula). Proc. R. Soc B. 275, 427–434. doi: 10.1098/rspb.2007.1383
Banks P. B., NorrDHl K., Korpimäki E. (2002). Mobility decisions and the predation risks of reintroduction. Biol. Conserv. 103, 133–138. doi: 10.1016/S0006-3207(01)00110-0
Bates D., Maechler M., Bolker B., Walker S. (2015). Fitting linear mixed-effects models using lme4. J. Stat. Software 67, 1–48. doi: 10.18637/jss.v067.i01
Beck B., Casgro M., Stoinski T., Ballou J. (2002). “The effects of pre release environments and post release management on survivorship in reintroduced golden lion tamarins,” in Lion tamarins biol conserv, vol. 283–300 . Eds. Kleiman D., Rylands A. (Washington, DC: Smithsonian Institution Press).
Berger-Tal O., Blumstein D. T., Swaisgood R. R. (2020). Conservation translocations: A review of common difficulties and promising directions. Anim. Conserv. 23, 121–131. doi: 10.1111/acv.12534
Berger-Tal O., Polak T., Oron A., Lubin Y., Kotler B. P., Saltz D. (2011). Integrating animal behavior and conservation biology: a conceptual framework. Behav. Ecol. 22, 236–239. doi: 10.1093/beheco/arq224
Berger-Tal O., Saltz D. (2014). Using the movement patterns of reintroduced animals to improve reintroduction success. Curr. Zool. 60, 515–526. doi: 10.1093/czoolo/60.4.515
Berger-Tal O., Wong B. B. M., Candolin U., Barber J. (2019). What evidence exists on the effects of anthropogenic noise on acoustic communication in animals? a systematic map protocol. Environ. Evid. 18, 1–7 doi: 10.1186/s13750-019-0165-3
Blumstein D. T., Evans C. S., Daniel J. C. (2000) JWatcher (Version 1.0). Available at: http://www.jwatcher.ucla.edu/.
Both C., Dingemanse N. J., Drent P. J., Tinbergen J. M. (2005). Pairs of extreme avian personalities have highest reproductive success. J. Anim. Ecol. 74, 667–674. doi: 10.1111/j.1365-2656.2005.00962.x
Carere C., Drent P. J., Koolhaas J. M., Groothuis T. G. G. (2005). Epigenetic effects on personality traits: Early food provisioning and sibling competition. Behavior 142, 1329–1355. doi: 10.1163/156853905774539328
Carter A. J., Feeney W. E., Marshall H. H., Cowlishaw G., Heinsohn R. (2013). Animal personality: What are behavioral ecologists measuring? Biol. Rev. 88, 465–475. doi: 10.1111/brv.12007
Clubb R., Mason G. J. (2007). Natural behavioral biology as a risk factor in carnivore welfare: How analysing species differences could help zoos improve enclosures. Appl. Anim. Behav. Sci. 102, 303–328. doi: 10.1016/j.applanim.2006.05.033
Conde D. A., Flesness N., Colchero F., Jones O. R., Scheuerlein A. (2011). An emerging role of zoos to conserve biodiversity. Science 331, 1390–1391. doi: 10.1126/science.1200674
Daly J. W., Spande T. F., Garraffo H. M. (2005). Alkaloids from amphibian skin: A tabulation of over eight-hundred compounds. J. Nat. Prod. 68, 1556–1575. doi: 10.1021/np0580560
Deng J., Mai K., Ai Q., Zhang W., Wang X., Xu W., et al. (2006). Effects of replacing fish meal with soy protein concentrate on feed intake and growth of juvenile Japanese flounder. Paralichthys olivaceus. Aquaculture 258, 503–513. doi: 10.1016/j.aquaculture.2006.04.004
Dias J., Huelvan C., Dinis M. T., Métailler R. (1998). Influence of dietary bulk agents (silica, cellulose and a natural zeolite) on protein digestibility, growth, feed intake and feed transit time in European seabass (Dicentrarchus labrax) juveniles. Aquat. Living Resour. 11, 219–226. doi: 10.1016/S0990-7440(98)89004-9
Dingemanse N. J., Both C., Drent P. J., Tinbergen J. M. (2004). Fitness consequences of avian personalities in a fluctuating environment. Proc. R. Soc B. 271, 847–852. doi: 10.1098/rspb.2004.2680
Dingemanse N. J., Both C., van Noordwijk A. J., Rutten A. L., Drent P. J. (2003). Natal dispersal and personalities in great tits (Parus major). Proc. R. Soc B. 270, 741–747. doi: 10.1098/rspb.2002.2300
Dingemanse N. J., Wolf M. (2010). Recent models for adaptive personality differences: A review. Philos. Trans. R. Soc. Lond. B Biol. Sci. 365, 3947–3958. doi: 10.1098/rstb.2010.0221
Eeva T., Helle S., Salminen J. P., Hakkarainen H. (2010). Carotenoid composition of invertebrates consumed by two insectivorous bird species. J. Chem. Ecol. 36, 608–613. doi: 10.1007/s10886-010-9796-0
Ferrie G.M., Alford V.C., Atkins J., Baitchman E., Barber D., Blaner W.S., et al. (2014). Nutrition and health in amphibian husbandry. Zoo Biol. 33, 485–501. doi: 10.1002/zoo.21180
Fischer J., Lindenmayer D. B. (2000). An assessment of the published results of animal relocations. Biol. Conserv. 96, 1–11. doi: 10.1016/S0006-3207(00)00048-3
Germano J. M., Nafus M. G., Perry J. A., Hall D. B., Swaisgood R. R. (2017). Predicting translocation outcomes with personality for desert tortoises. Behav. Ecol. 28, 1075–1084. doi: 10.1093/beheco/arx064
Gilbert T., Gardner R., Kraaijeveld A. R., Riordan P. (2017). Contributions of zoos and aquariums to reintroductions: historical reintroduction efforts in the context of changing conservation perspectives. Int. Zoo Yb. 51, 15–31. doi: 10.1111/izy.12159
Greggor A. L., Berger-Tal O., Blumstein D. T., Angeloni L., Bessa-Gomes C., Blackwell B. F., et al. (2016). Research priorities from animal behavior for maximising conservation progress. Trends Ecol. Evol. 31, 953–964. doi: 10.1016/j.tree.2016.09.001
Greggor A. L., Blumstein D. T., Wong B. B. M., Berger-Tal O. (2019). Using animal behavior in conservation management: A series of systematic reviews and maps. Environ. Evid. 8, 1–3. doi: 10.1186/s13750-019-0164-4
Greggor A. L., Thornton A., Clayton N. S. (2015). Neophobia is not only avoidance: Improving neophobia tests by combining cognition and ecology. Curr. Opin. Behav. Sci. 6, 82–89. doi: 10.1016/j.cobeha.2015.10.007
Haage M., Maran T., Bergvall U. A., Elmhagen B., Angerbjörn A. (2017). The influence of spatiotemporal conditions and personality on survival in reintroductions–evolutionary implications. Oecologia 183, 45–56. doi: 10.1007/s00442-016-3740-0
Han C. S., Dingemanse N. J. (2015). Effect of diet on the structure of animal personality. Front. Zool. 12. doi: 10.1186/1742-9994-12-S1-S5
Han C. S., Dingemanse N. J. (2017). You are what you eat: Diet shapes body composition, personality and behavioral stability. BMC Evol. Biol. 17, 8. doi: 10.1186/s12862-016-0852-4
Hill G. E., Inouye C. Y., Montgomerie R. (2002). Dietary carotenoids predict plumage coloration in wild house finches. Proc. R. Soc. B. 269, 1119–1124. doi: 10.1098/rspb.2002.1980
Hunter D., Clemann N., Coote D., Gillespie G.R., Hollis G., Scheele B., et al. (2018). “Frog declines and associated management response in south-eastern mainland Australia and Tasmania,” in Status of conservation and decline of amphibians: Australia, new Zealand and pacific islands. Eds. Heatwole H., Rowley J. (Collingwood Australia:CSIRO Publishing, Collingwood).
Hurley B. P., Garnas J., Cooperband M. F. (2015). Assessing trap and lure effectiveness for the monitoring of Sirex noctilio. Agric. For Entomol. 17, 64–70. doi: 10.1111/afe.12081
IUCN (2020). Table 1b: Numbers of threatened species by major groups of organisms (1996–2020). Available at - https://www.iucnredlist.org/resources/summary-statistics#Summary%20Tables
Kelleher S. R., Silla A. J., Byrne P. G. (2018). Animal personality and behavioral syndromes in amphibians: a review of the evidence, experimental approaches and implications for conservation. Behav. Ecol. Sociobiol. 72, 79. doi: 10.1007/s00265-018-2493-7
Kelleher S. R., Silla A. J., Dingemanse N. J., Byrne P. G. (2017). Body size predicts between individual differences in exploration behavior in the southern corroboree frog. Anim. Behav. 129, 161–170. doi: 10.1016/j.anbehav.2017.05.013
Kelleher S. R., Silla A. J., Niemela P. T., Dingemanse N. J., Byrne P. G. (2019). Dietary carotenoids affect the development of individual differences and behavioral plasticity. Behav. Ecol. 30, 1273–1282. doi: 10.1093/beheco/arz074
Keogh L. M., Silla A. J., McFadden M. S., Byrne P. G. (2018). Dose and life stage-dependent effects of dietary beta-carotene supplementation on the growth and development of the booroolong frog. conserv. Physiol. 6, coy052. doi: 10.1093/conphys/coy052
Koch R. E., Hill G. E. (2018). Do carotenoid-based ornaments entail resource trade-offs? an evaluation of theory and data. Funct. Ecol. 32, 1908–1920. doi: 10.1111/1365-2435.13122
Koch R. E., Staley M., Kavazis A. N., Hasselquist D., Toomey M. B., Hill G. E. (2019). Testing the resource trade-off hypothesis for carotenoid-based signal honesty using genetic variants of the domestic canary. J. Exp. Biol. 222, p.jeb188102. doi: 10.1242/jeb.188102
Koprivnikar J., Gibson C. H., Redfern J. C. (2012). Infectious personalities: behavioral syndromes and disease risk in larval amphibians. Proc. R. Soc B. 279, 1544–1550. doi: 10.1098/rspb.2011.2156
Krause E. T., Kruger O., Schielzeth H. (2017). Long-term effects of early nutrition and environmental matching on developmental and personality traits in zebra finches. Anim. Behav. 128, 103–115. doi: 10.1016/j.anbehav.2017.04.003
Lichtenthaler H. K. (1987). Chlorophylls and carotenoids: pigments of photosynthetic biomembranes. Methods Enzymol. 148, 350–382. doi: 10.1016/0076-6879(87)48036-1
Lin S. M., Nieves-Puigdoller K., Brown A. C., McGraw K. J., Clotfelter E. D. (2010). Testing the carotenoid trade-off hypothesis in the polychromatic Midas cichlid, Amphilophus citrinellus. physiol. Biochem. Zool. 83, 333–342. doi: 10.1086/649965
Martin J., Lopez P. (1999). When to come out from a refuge: risk-sensitive and state-dependent decisions in an alpine lizard. Behav. Ecol. 10, 487–492. doi: 10.1093/beheco/10.5.487
Matthews F., Orros M., McLaren M., Foster G. R. (2005). Keeping fit on the ark: assessing the suitability of captive-bred animals for release. Biol. Conserv. 121, 569–577. doi: 10.1016/j.biocon.2004.06.007
May T. M., Page M. J., Fleming P. A. (2016). Predicting survivors: animal temperament and translocation. Behav. Ecol. 27, 969–977. doi: 10.1093/beheco/arv242
McDermott M. T., Safran R. J. (2021). Sensitive periods during the development and expression of vertebrate sexual signals: A systematic review. Ecol. Evol. 11, 14416–14432. doi: 10.1002/ece3.8203
McDougall P. T., Reale D., Sol D., Reader S. M. (2006). Wildlife conservation and animal temperament: Causes and consequences of evolutionary changes for captive, reintroduced and wild populations. Anim. Conserv. 9, 39–48. doi: 10.1111/j.1469-1795.2005.00004.x
McFadden M. S., Gilbert D., Bradfield K., Evans M., Marantelli G., Byrne P. G. (2018). “The role of ex situ amphibian conservation in Australia,” in Status of conservation and decline of amphibians: Australia, new Zealand and pacific islands. Eds. Heatwole H., Rowley J. (Collingwood Australia:CSIRO Publishing, Collingwood).
McFadden M., Hobbs R., Marantelli G., Harlow P., Banks C., Hunter D. (2013). Captive management and breeding of the critically endangered southern corroboree frog (Pseudophryne corroboree) (Moore 1953) at taronga and Melbourne zoos. Amphib. Reptile Conserv. 5, 70–87.
McInerney E. P., Byrne P. G., Silla A. J. (2017). The effect of dietary antioxidants and exercise training on the escape performance of southern corroboree frogs. Behav. ProC. 144, 46–50. doi: 10.1016/j.beproc.2017.08.012
McInerney E. P., Silla A. J., Byrne P. G. (2019). Effect of carotenoid class and dose on the growth and development of the critically endangered southern corroboree frog. Conserv. Physiol. 7, coz009. doi: 10.1093/conphys/coz009
McInerney E. P., Silla A. J., Byrne P. G. (2020). Carotenoid supplementation affects the post-hibernation performance of southern corroboree frogs. Behavior 157, 121–142. doi: 10.1163/1568539X-00003584
Merrick M. J., Koprowski J. L. (2017). Should we consider individual behavior differences in applied wildlife conservation studies? Biol. Conserv. 209, 34–44. doi: 10.1016/j.biocon.2017.01.021
Noguera J. C., Metcalfe N. B., Surai P. F., Monaghan P. (2015). Are you what you eat? micronutritional deficiencies during development influence adult personality-related traits. Anim. Behav. 10, 129–140. doi: 10.1016/j.anbehav.2014.12.029
Nyqvist M. J., Gozlan R. E., Cucherousset J., Britton J. R. (2012). Behavioral syndrome in a solitary predator is independent of body size and growth rate. PloS One 7 (2), e31619. doi: 10.1371/journal.pone.0031619
Ogilvy V., Preziosi R. F. (2012). Can carotenoids mediate the potentially harmful effects of ultraviolet light in Silurana (Xenopus) tropicalis larvae? J. Anim. Physiol. Anim. Nutr. (Berl) 96, 693–699. doi: 10.1111/j.1439-0396.2011.01197.x
Osborne W. S. (1991). “The biology and management of the corroboree frog (Pseudophryne corroboree),” in NSW (Hurstville, NSW: NSW National Parks and Wildlife Service).
Preisser E. L., Orrock J. L. (2012). The allometry of fear: interspecific relationships between body size and response to predation risk. Ecosphere 3, 1–27. doi: 10.1890/ES12-00084.1
R Core Team (2018). R: A language and environment for statistical computing (Vienna, Austria: R Foundation for Statistical Computing). Available at: https://www.R-project.org/.
Reale D., Reader S. M., Sol D., McDougall P. T., Dingemanse N. J. (2007). Integrating animal temperament within ecology and evolution. Biol. Rev. 82, 291–318.R. doi: 10.1111/j.1469-185X.2007.00010.x
Richardson K. M., Parlato E. H., Walker L. K., Parker K. A., Ewen J. G., Armstrong D. P. (2019). Links between personality, early natal nutrition and survival of a threatened bird. Philos. Trans. R. Soc B. 374, 20190373. doi: 10.1098/rstb.2019.0373
Riemer K., Anderson-Teixeira K. J., Smith F. A., Harris D. J., Ernest S. M. (2018). Body size shifts influence effects of increasing temperatures on ectotherm metabolism. Glob. Ecol. Biogeogr. 27, 958–967. doi: 10.1111/geb.12757
Robertson B. C., Elliott G. P., Eason D. K., Clout M. N., Gemmell N. J. (2006). Sex allocation theory aids species conservation. Biol. Lett. 2, 229–231. doi: 10.1098/rsbl.2005.0430
Rowe M., Pierson K. L., McGraw K. J. (2015). Exploratory behavior is associated with plasma carotenoid accumulation in two congeneric species of waterfowl. Behav. ProC. 115, 181–190. doi: 10.1016/j.beproc.2015.04.008
Schmitz P., Caspers S., Warren P., Witte K. (2015). First steps into the wild – exploration behavior of European bison after the first reintroduction in western Europe. PloS One 10, e0143046. doi: 10.1371/journal.pone.0143046
Shaw A. K. (2020). Causes and consequences of individual variation in animal movement. Mov. Ecol. 8, 1–12. doi: 10.1186/s40462-020-0197-x
Shier D. M., Owings D. (2006). Effects of predator training on behavior and post release survival of captive prairie dogs. Biol. Conserv. 132, 126–135. doi: 10.1016/j.biocon.2006.03.020
Sih A., Mathot K. J., Moirón M., Montiglio P. O., Wolf M., Dingemanse N. J. (2015). Animal personality and state-behavior feedbacks: A review and guide for empiricists. Trends Ecol. Evol. 30, 50–60. doi: 10.1016/j.tree.2014.11.004
Silla A. J., Kouba A. J. (2022). “Integrating reproductive technologies into the conservation toolbox for the recovery of amphibian species,” in Reproductive technologies and biobanking for the conservation of amphibians. Eds. Silla A. J., Kouba A. J., Heatwole H. (Melbourne, Australia: CSIRO).
Silla A. J., McInerney E. P., Byrne P. G. (2016). Dietary carotenoid supplementation improves the escape performance of the southern corroboree frog. Anim. Behav. 112, 213–220. doi: 10.1016/j.anbehav.2015.12.012
Soorae P. S. (2016). Global re-introduction perspectives 2016: Case-studies from around the globe(Dubai: IUCN/SSC Re-introduction Specialist Group & Environment Agency-Abu Dhabi).
Stamps J. A., Swaisgood R. R. (2007). Someplace like home: Experience, habitat selection and conservation biology. Appl. Anim. Behav. Sci. 102, 392–409. doi: 10.1016/j.applanim.2006.05.038
Stankowich T., Blumstein D. T. (2005). Fear in animals: a meta-analysis and review of risk assessment. Proc. R. Soc B. 272, 2627–2634. doi: 10.1098/rspb.2005.3251
Svennson P. A., Wong B. B. M. (2011). Carotenoid-based signals in behavioral ecology: a review. Behavior 148, 131–189. doi: 10.1163/000579510X548673
Tapley B., Bradfield K. S., Michaels C., Bungard M. (2015). Amphibians and conservation breeding programmes: do all threatened amphibians belong on the ark? Biodivers. Conserv. 24, 2625–2646. doi: 10.1007/s10531-015-0966-9
Tracy C. R., Christian K. A., Tracy C. R. (2010). Not just small, wet, and cold: effects of body size and skin resistance on thermoregulation and arboreality of frogs. Ecology 91, 1477–1484. doi: 10.1890/09-0839.1
Umbers K. D. L., Riley J. L., Kelly M. B. J., Taylor-Dalton G., Lawrence J. P., Byrne P. G. (2020). Educating the enemy: Harnessing learned avoidance behavior in wild predators to increase survival of reintroduced southern corroboree frogs. Conserv. Sci. Pract. 2, e139. doi: 10.1111/csp2.139
Valenzuela-Sánchez A., Cunningham A. A., Soto-Azat C. (2015). Geographic body size variation in ectotherms: effects of seasonality on an anuran from the southern temperate forest. Front. Zool. 12, 1–10. doi: 10.1186/s12983-015-0132-y
van Oers K., Klunder M., Drent P. J. (2005). Context dependence of personalities: risk-taking behavior in a social and a nonsocial situation. Behav. Ecol. 16, 716–723. doi: 10.1093/beheco/ari045
van Overveld T., Careau V., Adriaensen F., Matthysen E. (2014). Seasonal- and sex-specific correlations between dispersal and exploratory behavior in the great tit. Oecologia 174, 109–120. doi: 10.1007/s00442-013-2762-0
Vargas A., Anderson S. H. (1999). Effects of experience and cage enrichment on predatory skills of black-footed ferrets (Mustela nigripes). J. Mammal. 80, 263–269. doi: 10.2307/1383226
Walton S. J., Silla A. J., Endler J. A., Byrne P. G. (2021). Does dietary β-carotene influence ontogenetic color change in the southern corroboree frog? J. Exp. Biol. 1224, jeb243182. doi: 10.1242/jeb.243182
Yamanashi Y., Teramoto M., Morimura N., Hirata S., Inoue-Murayama M., Idani G. (2016). Effects of relocation and individual and environmental factors on the long-term stress levels in captive chimpanzees (Pan troglodytes): Monitoring hair cortisol and behaviors. PloS One 11, e0160029.10.1371/journal.pone.0160029.
Keywords: amphibian, behavior, conservation breeding program, exploration, carotenoids, reintroduction biology
Citation: Kelleher SR, Silla AJ, Hunter DA, McFadden MS and Byrne PG (2022) Captive diet does not influence exploration behavior upon reintroduction to the wild in a critically endangered amphibian. Front. Conserv. Sci. 3:985545. doi: 10.3389/fcosc.2022.985545
Received: 04 July 2022; Accepted: 22 August 2022;
Published: 08 September 2022.
Edited by:
Govindhaswamy Umapathy, Center for Cellular & Molecular Biology (CCMB), IndiaReviewed by:
Sinlan Poo, Memphis Zoological Society, United StatesCopyright © 2022 Kelleher, Silla, Hunter, McFadden and Byrne. This is an open-access article distributed under the terms of the Creative Commons Attribution License (CC BY). The use, distribution or reproduction in other forums is permitted, provided the original author(s) and the copyright owner(s) are credited and that the original publication in this journal is cited, in accordance with accepted academic practice. No use, distribution or reproduction is permitted which does not comply with these terms.
*Correspondence: Phillip G. Byrne, cGJ5cm5lQHVvdy5lZHUuYXU=
Disclaimer: All claims expressed in this article are solely those of the authors and do not necessarily represent those of their affiliated organizations, or those of the publisher, the editors and the reviewers. Any product that may be evaluated in this article or claim that may be made by its manufacturer is not guaranteed or endorsed by the publisher.
Research integrity at Frontiers
Learn more about the work of our research integrity team to safeguard the quality of each article we publish.