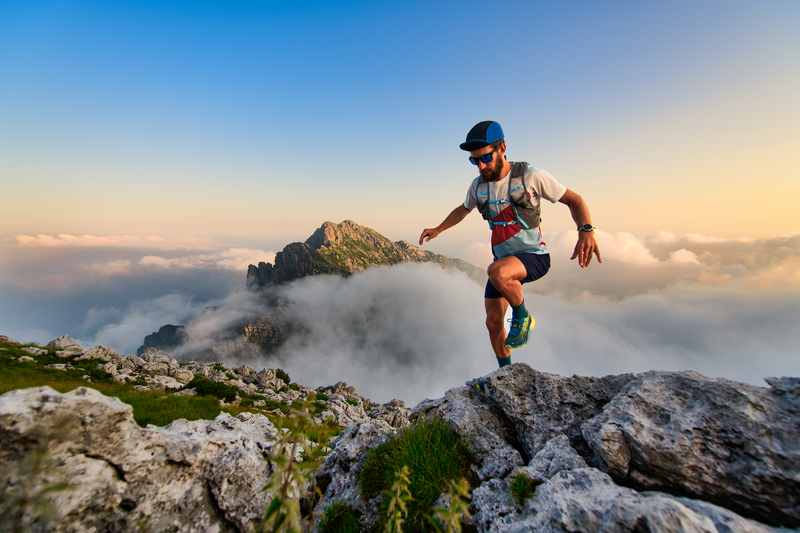
95% of researchers rate our articles as excellent or good
Learn more about the work of our research integrity team to safeguard the quality of each article we publish.
Find out more
ORIGINAL RESEARCH article
Front. Conserv. Sci. , 02 June 2022
Sec. Plant Conservation
Volume 3 - 2022 | https://doi.org/10.3389/fcosc.2022.870041
This article is part of the Research Topic Overlooked and Understudied – Rare Plant Taxa and their Importance for Biodiversity Conservation View all 4 articles
Sustainable conservation of crop wild relatives is one of the pathways to securing global food security amid climate change threats to biodiversity. However, their conservation is partly limited by spatio-temporal distribution knowledge gaps mostly because they are not morphologically charismatic species to attract conservation attention. Therefore, to contribute to the conservation planning of crop wild relatives, this study assessed the present-day distribution and predicted the potential effect of climate change on the distribution of 15 Vigna crop wild relative taxa in Benin under two future climate change scenarios (RCP 4.5 and RCP 8.5) at the 2055-time horizon. MaxEnt model, species occurrence records, and a combination of climate- and soil-related variables were used. The model performed well (AUC, mean = 0.957; TSS, mean = 0.774). The model showed that (i) precipitation of the driest quarter and isothermality were the dominant environmental variables influencing the distribution of the 15 wild Vigna species in Benin; (ii) about half of the total land area of Benin was potentially a suitable habitat of the studied species under the present climate; (iii) nearly one-third of the species may shift their potentially suitable habitat ranges northwards and about half of the species may lose their suitable habitats by 5 to 40% by 2055 due to climate change; and (iv) the existing protected area network in Benin was ineffective in conserving wild Vigna under the current or future climatic conditions, as it covered only about 10% of the total potentially suitable habitat of the studied species. The study concludes that climate change will have both negative and positive effects on the habitat suitability distribution of Vigna crop wild relatives in Benin such that the use of the existing protected areas alone may not be the only best option to conserve the wild Vigna diversity. Integrating multiple in situ and ex situ conservation approaches taking into account “other effective area-based conservation measures” is recommended. This study provides a crucial step towards the development of sustainable conservation strategies for Vigna crop wild relatives in Benin and West Africa.
Conserving biodiversity under the changing climate is one of the greatest challenges of our time, with recent studies predicting expansions or contractions of suitable habitats for many taxa (Aguirre-Gutiérrez et al., 2017; Phillips et al., 2017; Ratnayake et al., 2021; Zuza et al., 2021; Hoveka et al., 2022), shifts in phenology (Lima et al., 2021) and unprecedented biodiversity loss (Bellard et al., 2012; Habibullah et al., 2022). It is predicted that most plants may lose over half of their suitable habitats if the global surface temperatures are to rise by 3°C by 2100 (Warren et al., 2018). Yet, global surface temperatures are predicted to increase by up to 5.7°C by the end of this Century under the business as usual scenario (IPCC, 2021). Therefore, to optimise conservation and sustain ecosystem services that biodiversity brings to people (Jaradat, 2015; Zimmerer et al., 2019), it is important to understand the effects of climate change on the habitat suitability distribution of species and identify those that are vulnerable and require urgent conservation attention (Pacifici et al., 2015; Phillips et al., 2017). Species distribution models (SDMs), also known as ecological niche models (ENMs) or habitat suitability models (HDMs) (Elith and Graham, 2009) have been used in this regard across a range of taxa including crop wild relatives (CWRs) (Guisan et al., 2013; Phillips et al., 2017; Vincent et al., 2019; Ratnayake et al., 2021). These are numerical tools that correlate known occurrences with environmental variables to explain and predict a species' potential range (Barlow et al., 2021).
Crop wild relatives (CWRs) are wild plant species that are genetically closely related to domesticated plants including their progenitors (Maxted et al., 2006). The genus Vigna Savi (Family Fabaceae) is a tropical and subtropical taxon, comprising nearly 105 species from which only nine have been domesticated (Somta et al., 2019; Catarino et al., 2021). The potential contribution of Vigna CWR species to the global food security improvement as well as human and ecological health is well-documented (Maxted et al., 2004; Tomooka et al., 2014; Harouna et al., 2018; Takahashi and Tomooka, 2020; van Zonneveld et al., 2020; Catarino et al., 2021). Like other CWRs, Vigna CWR taxa have high genetic diversity since they have not gone through domestication bottlenecks and artificial selection (Dempewolf et al., 2017; Zhang et al., 2017; Bohra et al., 2021), and harbour various genes responsible for environmental stress adaptation (Takahashi et al., 2016; van Zonneveld et al., 2020). These traits may be used in the development of more productive, nutritious, and resilient Vigna crop varieties (Castañeda-Álvarez et al., 2016) against the background of the cascading impacts of climate change on the productivity of domesticated species (Dempewolf et al., 2017; Ortiz-Bobea et al., 2021), the projected risks on the future food systems (Müller and Robertson, 2014), and the increasing concerns over food and nutrition insecurity (Godfray, 2014; Willett et al., 2019). Moreover, the actual and potential uses of CWRs in crop improvement are widely reported (Hajjar and Hodgkin, 2007; Dempewolf et al., 2017), the annual contribution of their use to the global economy is estimated between USD 120–186 (PricewaterhouseCooper, 2013; Tyack et al., 2020), and their importance is well recognised in the global business and political agenda (e.g., CBD, 2005, 2010; FAO, 2009). Besides, Vigna CWRs are potential candidates for neo-domestication (Tomooka et al., 2014), and as with other CWRs, they could also be commercialised (Abdelghany et al., 2021). As such, the conservation and sustainable use of Vigna CWR diversity is not only pertinent but urgent for securing global food, economy, and other ecosystem services in a warming world (Dempewolf et al., 2014; Fitzgerald et al., 2019; Zimmerer et al., 2019), thus potentially contributing to the attainment of the United Nations Development Goals (e.g., SDG 2- End hunger; SDG 13-Resilience and adaptation to climate change) (United Nations, 2015).
However, as with other CWRs, conservation planning of Vigna CWR taxa is partly limited by fundamental knowledge gaps in their distributions under the changing climate (Khoury et al., 2020). This is mostly because CWRs get relatively less conservation attention since they are not morphologically charismatic species (Maxted et al., 2016; Adamo et al., 2021, but see Veríssimo et al., 2017). Meanwhile, they are increasingly threatened by climate change in their natural environments (Jarvis et al., 2008; Vincent et al., 2019; Goettsch et al., 2021). For instance, Jarvis et al. (2008) predicted that 16–22% of Vigna CWR species risk extinction globally by the year 2055 and the majority of the species may lose over 50% of their climatically suitable habitats. The authors further observed differential responses to different environmental factors in Vigna CWR species. On the other hand, Vincent et al. (2019) identified 150 potential sites for the conservation of 66% of the studied 1,261 CWRs that included selected Vigna species in light of future climate change (2060–2089), and a few areas around West, Central and East Africa were among the sites earmarked. However, findings obtained at the global scale are less likely to inform conservation decision-making at a local scale (Phillips et al., 2017; Stephan et al., 2020). Moreover, the use of only the optimistic Representative Concentration Pathway (RCP 4.5) by Vincent et al. (2019) might have overestimated the potentially severe impacts of climate change (Scridel et al., 2021), especially for areas where climate change impacts are predicted to become more severe such as West Africa (IPCC, 2021). Therefore, understanding how Vigna CWR taxa would respond to future climate change effects in Benin would help in planning for their adaptive management approaches (Iriondo et al., 2021).
To preserve CWR genetic diversity and ensure they meet future food security needs under the changing climate, in situ conservation of CWR diversity has long been considered the best conservation option (Maxted and Kell, 2009; Maxted et al., 2011; Bellon et al., 2017; FAO, 2017). In this regard, the establishment of genetic reserves within or on the fringes of the existing protected areas (PAs) has been strongly recommended (Maxted and Kell, 2009; Maxted et al., 2016; FAO, 2017). But, the effectiveness of PAs in conserving CWRs has been put into question, considering that PAs were established for charismatic species (Maxted et al., 2016), and are static establishments while species are shifting due to climate change (Thomas and Gillingham, 2015; Heywood, 2019). With regard to the use of PAs, Maxted et al. (2004) and Moray et al. (2014) showed that most African Vigna CWR species could be effectively conserved in the existing PAs in Africa. But, the extent to which such PAs would become potential refugia for Vigna CWR diversity under the changing climate (Jarvis et al., 2008) was not addressed. Further, as part of the initial steps towards the development of sustainable conservation measures for CWRs in the region, prioritisation of CWRs has been done in Benin (Idohou et al., 2013) and West Africa (Nduche et al., 2021), but studies on the effects of climate change on CWRs are nebulous in the literature for the region. To date, many studies that have been conducted in this respect have mostly been on non-CWR tree species (e.g., Favi et al., 2021; Lompo et al., 2021; Salako et al., 2021; Assogba et al., 2022), palms (Idohou et al., 2017a; Salako et al., 2019), and woody lianas (Vihotogbé et al., 2021).
This study intended to address these gaps and contribute to the existing efforts in the conservation of CWRs in West Africa and the global network of genetic reserves (Maxted and Kell, 2009). It was aimed at assessing the present-day distribution and forecasting the potential effect of climate change on the distribution of 15 Vigna CWR taxa in Benin under two future climate change scenarios (RCP 4.5 and RCP 8.5) at the 2055-time horizon. Three objectives were formulated: (1) to identify environmental factors influencing the distribution of habitats suitable for 15 Vigna CWR taxa in Benin; (2) to map the present-day habitat suitability distribution of 15 wild Vigna taxa and project their future distribution under two climate scenarios (RCP 4.5 and RCP 8.5) in the 2055-time horizon; and (3) to prioritise in situ conservation sites for Vigna CWR taxa in Benin and evaluate the effectiveness of PAs in conserving the studied taxa. The study hypothesised that (1) different Vigna CWR taxa would respond differentially to a different suite of environmental variables (Jarvis et al., 2008); (2) there would be an increase in the northern habitat (Lenoir et al., 2020); and (3) the existing PA network in Benin will be less effective in conserving Vigna CWR diversity than non-PAs.
Benin is located in West Africa between 6°25′ N to 12°30′N and 0°45′E to 4°00′E. Generally, Benin is a low lying country, with altitudes varying from sea level to 400 m a.s.l., although it can go up to 650 m in the northwest of the country, where temperatures can also be exceptionally high (35–40°C) (Adomou et al., 2006). Agriculture is the main source of livelihood in rural areas. Like most developing countries, Benin is experiencing rapid population growth, with the recent human population estimated at 12.5 million people (United Nations, 2021) and associated with increasing urbanisation and land-use change (Guidigan et al., 2019).
Benin is divided into three contrasting climatic zones (Adomou et al., 2006): Guinean (in the south), Sudanian (in the north), and Sudano-Guinean (a transition zone in the centre). Basically, the Guinean zone is characterised by a sub-humid climate, bimodal rainfall (April–June & September–November) averaging 1,200 mm/year, a temperature range of 18–33°C, relative humidity ranging between 30 and 98%, ferrallitic and hydromorphic soils without concretions, and woodlands and fallows as the dominant habitats and/or vegetation types. While, sub-humid climate, unimodal rainfall (May–October) ranging between 900 and 1,100 mm/year, an annual temperature range of 20–36°C, relative humidity of 31–98%, ferruginous to somewhat tropical ferruginous and ferrallitic soils on crystalline basement characterise the Sudano-Guinean zone. This zone is dominated by a mosaic of savanna woodlands, dense shrub and tree forests, and gallery forests. On the other hand, the Sudanian zone is characterised by Sudano-Sahelian climate, unimodal rainfall (700–900 mm/year), a relatively wide temperature range (17–42°C), and a wide relative humidity (18–99%). Furthermore, this zone is dominated by ferruginous soils on crystalline basement and mostly dry shrubby forests and savannas (Adomou et al., 2006; Assogbadjo et al., 2011; Hounkpatin et al., 2022). Like in most parts of the sub-Saharan Africa, the soils in Benin are mostly sandy and infertile (Hounkpatin et al., 2022).
Vigna CWRs are herbaceous, annual or perennial, climbing, scrambling or prostrate plants. Perennial species generally have large, woody rootstocks which often die back in cooler months only to grow again in warm weather or following burning e.g., Vigna frutescens A. Rich (Maxted et al., 2004). Their sizes generally range from <1 m for species like Vigna laurentii De Wild. to over 7 m e.g., Vigna racemosa (G. Don) Hutch. & Dalziel. Their stems may be glabrous or with various levels of pubescence. They are found in a wide range of habitats such as savannas, grasslands, open woodlands, and shrublands usually at low altitudes, with some species such as Vigna luteola (Jacq.) Benth. and V. laurentii often associated with wet areas. Most Vigna CWR taxa in Benin flower and reproduce between August/September and December. Like other leguminous plants, wild Vigna species have a ballochory (explosive dehiscence) seed dispersal as their primary seed dispersal mechanism (Lush et al., 1980; Trzeciak-Limeira et al., 2013); thus, they are likely to be mostly short-distance dispersed plants, probably dispersing their seeds over a 1–5 m radius (Vittoz and Engler, 2007; Parker et al., 2021). A few species like V. luteola are known to be dispersed over long distances by sea-drifting (Miryeganeh et al., 2014). While those that are used as forage such as V. racemosa and Vigna reticulata Hook. f. (Catarino et al., 2021), are likely to be dispersed over long distances by secondary agents such as herbivores (Ramírez-Rodríguez et al., 2021; Wang and Hou, 2021). Vigna CWR taxa favour warm temperatures, but higher temperatures (>36°C) are detrimental to their metabolic processes such as photosynthesis (Farooq et al., 2017).
Currently, Benin has about 31 Vigna CWR taxa that have been described (Supplementary Table 1), but this study used only 15 of these; the rest were left out as they had ≤ 10 occurrence records (Yesuf et al., 2021). The full list of the studied taxa were Vigna comosa Baker, Vigna filicaulis Hepper, V. frutescens, Vigna gracilis (Guill. & Perr.) Hook. f., Vigna heterophylla A. Rich., V. laurentii, Vigna longifolia (Benth.) Verdc., V. luteola, Vigna multinervis Hutch. & Dalziel, Vigna nigritia Hook. f., Vigna oblongifolia A. Rich., V. racemosa, V. reticulata, Vigna unguiculata subsp. baoulensis (A. Chev.) Pasquet, and Vigna unguiculata var. spontanea (Schweinf.) Pasquet. Of these 15 taxa, only three (V. heterophylla, V. unguiculata subsp. baoulensis, and V. unguiculata var. spontanea) were at the time of this study not yet assessed using the IUCN Red List Categories and Criteria (Supplementary Table 1). The spatial distributions of the 15 studied taxa are presented in Figure 1.
This study is a follow-up on the national inventory and prioritisation of CWRs for Benin (Idohou et al., 2013). The Global Biodiversity Information Facility (GBIF, www.gbif.org) was the main source of species occurrence records (1,952) [accessed on July 14, 2020]. The RAINBIO (https://gdauby.github.io/rainbio) [accessed on July 16, 2020], and the Genesys Global Portal on Plant Genetic Resources (https://www.genesys-pgr.org) [accessed on July 15, 2020], provided additional records, 128 and 24, respectively.
In addition, random field visits were made to verify location data related to the distribution of some species and partly minimise the sampling bias in the database (Meng et al., 2021). To ensure data quality, visual inspection was used to identify outliers and these were clipped out. Records collected earlier than 1990 were removed from the data set to reduce the effects of temporary bias (Idohou et al., 2017b). To reduce clumping bias, duplicate records were removed from the data set, and occurrence records were spatially thinned to a geographic distance of 1 × 1 km2 (Idohou et al., 2017b) using Environmental Niche Modelling (ENM) tools (www.ENMTools.com) (Warren et al., 2010) performed in QGIS version 3.8.1 (QGIS Project https://qgis.org). Spatial thinning not only reduces model overfitting but also improves the performance of models better than background manipulation (Ratnayake et al., 2021). The Flora of Benin (Akoegninou et al., 2006) and experts were consulted to verify the adequacy of the observed range distribution of the target species.
The study used a combination of bioclimatic and soil variables. Bioclimatic data represent annual trends in climate conditions, seasonality and climate extremes, which may impact reproduction and survival of species over broad extents (Aguirre-Gutiérrez et al., 2017; Idohou et al., 2017a). On the other hand, soil variables may directly constrain the establishment and development of species (Aguirre-Gutiérrez et al., 2017), and their incorporation in SDM, especially at the local scale, which was the case in the current study, appears to improve the predictive capacity of models (Hageer et al., 2017; Zuquim et al., 2020).
Twenty-one bioclimatic variables for the present and future scenarios were downloaded from the AfriClim database version 3.0 at a spatial resolution of 30 arc seconds (~1 km2) (https://www.york.ac.uk/environment/research/kite/resources/) (Platts et al., 2015) [accessed on March 17, 2020]. The database spans 10 general circulation models (GCMs), downscaled using five bias-corrected regional climate models (RCMs) and four contemporary baselines, under two representative concentration pathways of the IPCC-AR5 (RCP4.5 and RCP8.5) (Platts et al., 2015). For the current conditions, the WorldClim v1.4 option with the baseline year of 1975 (1950–2000) was used from the database. For the future climatic conditions—horizon 2055 (2041–2070), the AfriClim Ensembles 3.0 with the WorldClim as the baseline was used, and two scenarios, RCP 4.5 (optimistic scenario) and RCP 8.5 (pessimistic scenario) (Platts et al., 2015) were considered. The two scenarios seem to be plausible for Africa (Platts et al., 2015), and have been widely used in SDM studies across Africa (Lompo et al., 2021; Zuza et al., 2021; Assogba et al., 2022). The mid-Century horizon (the 2050s) was chosen to align with the United Nations framework of global challenges in agriculture and food security (Zuza et al., 2021), which also dovetails well with the Agenda 2063 for Africa.
Soil data were obtained at 250 m resolution from the Africa Soil Profiles Database (http://www.isric.org) (Hengl et al., 2017). Eleven sets of bio-physiochemical soil characteristics were downloaded. These were bulk density (t/m3), soil organic carbon (g/kg), pH in water, clay content (%), sand content (%), silt content (%), cation exchange capacity (cmolc/kg), exchangeable acidity (cmolc/kg), exchangeable Ca (cmolc/kg), exchangeable K (cmolc /kg), and exchangeable Mg (cmolc/kg). These soil variables have been used in previous studies in Benin (e.g., Idohou et al., 2017b; Vihotogbé et al., 2021). Since soil-plant interactions seem to be critical within the 0–16 cm soil depth (Goebes et al., 2019) and given that Vigna species are herbaceous small-statured plants, only a maximum of three soil depth horizons (0–5, 5–15, and 15–30 cm) were considered for this study. A total of 30 soil layers were thus downloaded. The soil data were resampled at 1 km resolution to match the resolution of the bioclimatic variables.
Maximum Entropy (Maxent ver. 3.4.1) algorithm (Phillips et al., 2006) was used. As with other correlative models, the MaxEnt procedure establishes the relationship between species occurrence records at sites and the environmental variables and/or spatial characteristics of such sites (Phillips et al., 2006; Elith et al., 2011). Despite its limitations (Lissovsky and Dudov, 2021), MaxEnt is among the many SDM tools (see Elith and Graham, 2009) with increasing use in conservation-oriented studies owing to its high predictive accuracy, stability and reliability even with presence-only data and small data sets (Elith et al., 2011; Phillips et al., 2017; Vincent et al., 2019; Çoban et al., 2020; Mponya et al., 2021; Ratnayake et al., 2021). Moreover, it produces spatially open habitat suitability maps and evaluates the significance level of individual environmental variables using the built-in Jackknife test (Çoban et al., 2020), which were among the core objectives of the current study.
Dealing with variable collinearity in SDM is evolving just like the algorithms themselves, with the use of the Pearson's correlation test in ENMTools (Warren et al., 2010) and priori selection of variables based on the ecological system (Scridel et al., 2021) as some of the common approaches to reducing potential multicollinearity. However, Feng et al. (2019) showed that the exclusion of highly correlated variables does not significantly influence model performance, especially those built by MaxEnt, as the algorithm accounts for redundancy in variables. According to Mod et al. (2016), neglecting eco-physiological meaningful predictors could result in incomplete niche quantifications, thereby limiting the predictive power of SDMs. The authors thus suggested that the selection of climatic-related variables should be determined by the environmental conditions of the study site and the requirements of the target species. Therefore, this study followed the approach used by Singh et al. (2021) of eliminating variables that consistently contributed less or nothing to the model for three successive runs. Firstly, two variables, minimum temperature of the coldest month (BIO 6), and mean temperature of the coldest quarter (BIO 11), were deemed unsuitable and were thus removed based on expert knowledge (Vihotogbé et al., 2021). Thereafter, all the remaining 49 variables were used in the model pre-assessment (but see Dormann et al., 2013) from which variables that contributed <5 % to each of the model explanatory power after every run for three successive runs were excluded following a Jackknife test (Singh et al., 2021). Finally, five variables that contributed the most to each of the 15 models were retained for modelling (Table 1).
Table 1. Occurrence records and environmental variables used for modelling the 15 Vigna CWR taxa in Benin.
Since the area included in the background can influence MaxEnt model fit (Merow et al., 2013; Pang et al., 2021), and given that models are more reliable when built at a larger scale (Barve et al., 2011), as this reduces the risk of niche truncations (Pang et al., 2021), species occurrence records were thus first filtered for West Africa, and models trained by projecting the present-day variables over West Africa. To further improve the model performance, the maximum number of iterations in MaxEnt was adjusted to 5,000, the cross-validation method was used, and the number of replications was increased to 15 (Abrha et al., 2018). Increasing the number of iterations and replications provides, respectively, ample time for the model to converge and run multiple times to develop superlative averaged results (Merow et al., 2013; Abrha et al., 2018). The “clogclog” was used as an output format (Favi et al., 2021; Scridel et al., 2021), since it appears to provide the model output with a stronger theoretical justification than the logistic transform (Qu et al., 2018) and seems to give realistic binary predictions of species distributions (Scridel et al., 2021). The jackknife test was used to determine the variable contribution to the models.
The model performance was assessed through two widely used metrics, the threshold-independent Area Under the Curve (AUC) of the Receiver Operating Characteristic (ROC) curve and the threshold-dependent True Skill Statistic (TSS) (Fielding and Bell, 1997; Allouche et al., 2006; Favi et al., 2021; Ratnayake et al., 2021). The AUC measures the model's ability to distinguish between random and background points (AUC = 0.5) with values ranging from 0 to 1 (Fielding and Bell, 1997; Favi et al., 2021). AUC values closer to 1 indicate good-performance models, and a stronger correlation between predictor variables and the distribution of the target species (Fielding and Bell, 1997). As such, models were considered acceptable if 0.7 ≤ AUC < 0.8, good if 0.8 ≤ AUC < 0.9 and excellent if AUC ≥ 0.9 (Ratnayake et al., 2021). The TSS is a measure of accuracy i.e., the capacity of the model to detect the true presence (sensitivity) and true absences (specificity), expressed as the sensitivity plus specificity-1 (Allouche et al., 2006). Its values range from −1 to +1, and like AUC, TSS values closer to 1 indicate good-performance models (Allouche et al., 2006). Therefore, models were described as poor (TSS <0.4), acceptable (0.4 ≤ TSS < 0.8), and very good (TSS > 0.8) (Ratnayake et al., 2021). Response curves were used to further evaluate and quantify the biological plausibility of the models since they show the predicted relative occurrence rate (ROR) of species against the value of a predictor variable (Merow et al., 2013). The variables were finally clipped to Benin and converted to Ascii format using SDM Tools for use in modelling.
MaxEnt was used to predict the habitat suitability distribution for each of the 15 species under the current and future climate change scenarios using 1,432 occurrence records (range: 14 to 333) and a combination of bioclimatic and edaphic variables as inputs (Table 1). The same settings as in the model calibration were used. Habitat suitability maps were developed following Ramírez-Rodríguez et al. (2021) with slight modifications. Briefly, output rasters from MaxEnt were converted into binary layers and exported to QGIS to develop habitat maps. Binarization of continuous habitats, in spite of its shortcomings (Santini et al., 2021), is still one of the popular approaches for delimiting habitats in SDM, quantifying range changes and building species richness overtime in conservation studies (Politi et al., 2021; Ramírez-Rodríguez et al., 2021; Singh et al., 2021; Vihotogbé et al., 2021; Lima et al., 2022). It is believed that binairization avoids the effects of model over-fitting (Vihotogbé et al., 2021), and that it makes interpretation of distribution maps much easier compared with the more liberal interpretation of a continuous habitat output (Singh et al., 2021). Using the 10th percentile presence threshold to separate suitable from unsuitable habitats Ramírez-Rodríguez et al. (2021), all values above and below this threshold were considered suitable and unsuitable habitats, respectively. According to Politi et al. (2021), compared with other percentile thresholds, the 10th percentile maximises the correct prediction of the percentage of presences and absences, thereby providing conservative species distributional ranges.
Subsequently, to calculate range changes between the current and future habitat distributions, binary-thresholded future rasters were subtracted from the current rasters (Ramírez-Rodríguez et al., 2021) using the range shift tool in the SDM Toolbox. This tool classifies output layers into four classes: expansion in range (absence in current, presence in future), no occupancy (absence in both current and future), occupancy or stable (presence in current and future) and contraction in range (presence in current, absence in future). Accordingly, three range changes in habitat suitability (stability, expansion and contraction) were mapped and areal extents were calculated.
To prioritise areas for in situ conservation, binary raster layers for the range change of all the 15 species and each of the three distributions (current, 2055 RCP 4.5 and 2055 RCP 8.5) were summed up to show overlaps of potential habitat distributions (Ramírez-Rodríguez et al., 2021). Each suitable distribution was thereafter reclassified into three classes (low suitability, moderate suitability, and high suitability) using the SDM Toolbox. Finally, these were overlaid onto the PA network of Benin to further estimate the extent to which the PAs would conserve the 15 Vigna CWR taxa. The area of each distribution was calculated using the extract by mask tool in the SDM Toolbox.
The AUC and TSS values ranged from 0.914 to 0.997 (median = 0.956; mean = 0.957) and 0.617 to 0.876 (median = 0.771; mean = 0.774), respectively (Figure 2). Likewise, the ROC curves were away from the random distribution (not shown here), and there were lower differences between AUC values for training and test samples for the model corresponding to all the 15 taxa (range = 0.001–0.025; mean = 0.008), indicating good performance and high accuracy of the model for generalisation. The models were thus considered excellent and highly informative to describe the distribution patterns in the 15 modelled Vigna CWR species in Benin.
The modelled species demonstrated differential responses to different environmental variables. Based on the frequency of the power of predictive contribution of each variable across all the 15 species, three variables, precipitation of the driest quarter (BIO 17), isothermality (BIO 3), and mean diurnal range in temperature (BIO 2), in that order, were found to contribute the most in explaining the majority of the models (Table 2). For instance, precipitation of the driest quarter was consistently found among the dominant three variables for 10 of the 15 models (V. frutescens, V. gracilis, V. heterophylla, V. longifolia, V. luteola, V. nigritia, V. oblongifolia, V. racemosa, V. reticulata, and V. unguiculata subsp. baoulensis). This was closely followed by isothermality (seven models: V. heterophylla, V. longifolia, V. multinervis, V. racemosa, V. reticulata, V. unguiculata subsp. baoulensis, and V. unguiculata var. spontanea); whilst mean diurnal range in temperature predicted five models (V. filicaulis, V. frutescens, V. heterophylla, V. laurentii and V. racemosa). Also worth noting was the influence of soil variables on some models. For instance, exchangeable acidity (KCl) for 10 cm depth (EACKCL_T_M _sd2) was important for V. filicaulis and V. unguiculata subsp. baoulensis,; while soil texture fraction sand for 2.5 cm depth (SNDPPT_T_M_sd1), was among the key variables for V. laurentii, V. nigritia and V. oblongifolia. Another important result was the influence of the length of the driest month (LLDS) on V. comosa, where it was the top-most predictor variable (53.7 %).
Table 2. The top three dominant environmental factors affecting the distribution of 15 Vigna CWR taxa in Benin.
However, the Jackknife test of variable importance (not included here) flagged the mean diurnal range in temperature as containing the most important information by itself and, therefore, explaining the gain in six models (V. filicaulis, V. frutescens, V. heterophylla, V. laurentii, V. oblongifolia, and V. racemosa). This was followed by isothermality for four models (V. longifolia, V. multinervis, V. unguiculata subsp. Baoulensis, and V. unguiculata var. spontanea) and precipitation of the driest quarter for three models (V. gracilis, V. luteola, and V. nigritia). On the other hand, isothermality appeared to have contained the most unique information that could not be found in all other variables for nine models (V. frutescens, V. heterophylla, V. longifolia, V. multinervis, V. oblongifolia, V. racemosa, V. reticulata, V. unguiculata subsp. Baoulensis, and V. unguiculata var. spontanea).
Suitable ranges of the three most important environmental variables for each model are represented by their respective response curves (Supplementary Figure 3). Generally, suitable habitats for the majority of the models were suggested highly likely to be in areas characterised by precipitation of the driest quarter (BIO 17) ranging between 5.5 and 133.5 mm; isothermality (BIO 3), with an average range of 64.3 to 80.4; and mean diurnal range in temperature (BIO 2), with an average range of 7.4–12.2°C. For the edaphic-related factors, suitable habitats were likely to be found in sites having a wide range of sandy soils, with an average soil texture fraction sand for 2.5 cm depth (SNDPPT_T_M_sd1) ranging between 17 and 90%, and somewhat acid soils, with an average exchangeable acidity (KCl) for 10 cm depth (EACKCL_T_M_sd2) below 0.6 cmolc/kg.
Nearly half of the land surface area in Benin was predicted to be presently a suitable habitat for the 15 Vigna CWR taxa, although uneven patterns were observed, with some species showing striking gaps (patches), while others were narrowly or widely distributed (Figure 3). For instance, V. racemosa was predicted to have the largest potentially suitable area (103, 817.6 km2), representing nearly 88.20% of the total surface area of Benin. This was closely followed by V. heterophylla (91, 664 km2), representing about 77.88% of the land area. The habitats of these two models were predicted to be mostly in the Sudanian and Sudano-Guinean Zones. On the contrary, V. laurentii had the least potentially suitable habitat distribution range (1,911 km2) and was closely followed by V. oblongifolia (3,735 km2), representing ~1.62 and 3.17% of the total land, respectively. Both of these species were predicted to be localised at the southern tip of the Guinean Zone (Figure 3).
Figure 3. (A–C) Predicted distribution maps of the 15 Vigna CWR taxa in Benin under the current and future climates.
Climate change was predicted to positively or negatively affect the future ranges of habitat suitability distribution of the 15 Vigna CWR taxa (Figure 3) with their per cent changes shown in Figure 4. Nearly half of the species were predicted to potentially lose their suitable habitats by 5–36% under moderate conditions (RCP 4.5) and 8–40% under severe conditions (RCP 8.5). A substantial contraction (35.57–39.53%) was registered for V. multinervis, with zero expansion under both climate change scenarios. Interestingly, a zero contraction was forecast for V. frutescens under both scenarios. On the other hand, five models including V. heterophylla, V. laurentii, and V. oblongifolia had insignificant retraction (<1.5%) in their habitat distribution ranges under RCP 4.5. The same trend was observed for these models under RCP 8.5, thus making V. frutescens, V. heterophylla, V. laurentii, V. nigritia, V. racemosa, and V. reticulata among the models whose potentially suitable habitats were predicted to remain the most stable under future climatic conditions. It was further observed that about one-third of the models including V. comosa and V. longifolia tended to expand their suitable areas towards the north of Benin i.e., higher latitude and altitude, while another one third including V. frutescens and V. unguiculata var. spontanea showed a tendency to expand to the other directions.
Figure 4. Graph of per cent changes in the suitable habitat distribution ranges of the 15 Vigna CWR taxa in Benin under current and future climates.
As was expected, when the two future climate change scenarios were compared, slightly higher contractions were predicted under severe climatic conditions (RCP 8.5: mean = 10.87%; median = 2.4%) than under moderate conditions (RCP 4.5: mean = 6.94%; median = 4.73%), although, this was also accompanied by a slightly higher expansion under RCP 8.5 (mean = 14.96%; median = 6.57 %). The models of V. unguiculata subsp. baoulensis and V. unguiculata var. spontanea showed the most marked changes in contractions between the two scenarios. On the contrary, the model of V. filicaulis registered a small retraction under RCP 8.5 compared with RCP 4.5.
Figure 5 represents the results from the predicted potential distribution of the suitable habitat richness of the 15 modelled taxa under both the current and future climatic conditions, taking into account the PA network in Benin. Under the current conditions, the largest portion of the highly suitable area was predicted to be in the Sudano-Guinean Zone, with a few patches in the Sudanian and Guinean Zones. The current potential suitable habitat accounted for 39,647.88 km2, representing ~33.69% of the land surface area of Benin. Out of this area, an estimated 3,931.72 km2 (9.92%) fell in the existing PA network.
Figure 5. Predicted hotspot distribution maps of potentially suitable habitats of the 15 Vigna CWR taxa in Benin under the current and future climates.
As for the future climate change scenarios, only about 27,107.87 km2, representing about 23.03% of the land surface area was predicted potentially suitable under the moderate emission scenario (RCP 4.5). This represented a substantial loss (~31.63%) relative to the present conditions, mostly in the northerly located patches. Out of this suitable area, 2,718.18 km2 (10.03% of the land surface area), was predicted to fall in the existing PA network. A somewhat different situation was projected under the severe climate change scenario (RCP 8.5). Here, the suitable habitat appeared to expand northwards, while the southern portion became less favourable. An estimated 48,933.01 km2 (~41.57%) of the total land surface area was predicted to become potentially highly suitable, representing an increase of about 23.42% relative to the present conditions. From this area, about 5,322.57 km2 (~10.88%) of the land surface area of Benin, was predicted to fall under the existing PA network.
The results from this study showed variations in taxa response to different environmental factors, thus supporting the first hypothesis that different Vigna CWR species would respond differentially to a different suite of environmental variables. Jarvis et al. (2008) reported a similar tendency among Vigna species. Several other studies have also reported similar generic tendencies such as in Cucurbita in Mexico (Lira et al., 2009), Piper and Oryza in Sri Lanka (Ratnayake et al., 2021), Vaccinium in the Netherlands (van Treuren et al., 2020) and Adansonia in Madagascar (Tagliari et al., 2021). Differential response of species to environmental factors underscores the need for species-specific studies on climate change effects (Jarvis et al., 2008).
While climate-related factors are generally the key environmental factors influencing species distribution (Jarvis et al., 2008; Amissah et al., 2014; Lompo et al., 2021), several studies have also shown that habitat distribution of species, especially at a local scale, is influenced by a combination of climatic and edaphic factors (Hageer et al., 2017; Idohou et al., 2017b; Zuquim et al., 2020; Assogba et al., 2022; Liu et al., 2022). The results from this study were consistent with these observations. The influence of edaphic factors appears to be particularly critical for narrowly distributed or understorey species (e.g., Hageer et al., 2017; Idohou et al., 2017b; Wang et al., 2019; Roe, 2020), and may be related to specialised habitat requirements by species that constrain their distribution (Corlett and Tomlinson, 2020). For instance, Idohou et al. (2017b) observed that the potential cultivable areas for the relatively localised and understorey palms such as Raphia hookeri and R. vinifera in Benin were more characterised by soil factors compared with overstorey palm species. Similarly, soil factors were the most discriminating factors in the distribution of an endemic orchid Spiranthes parksii (Navasota ladies' tresses) in the USA (Wang et al., 2019). Indeed, disregarding edaphic factors in SDM may overestimate future habitat adaptability of many plant species (Bertrand et al., 2012; Zuquim et al., 2020, but see Feng et al., 2020). Soil texture, for instance, is important for plant root development, especially for the relatively high-biomass rooted plants like most Vigna CWR species (Iseki et al., 2018), while exchangeable acidity (KCL) is crucial for soil nutrition and texture balance (Liebenberg et al., 2020).
The importance of precipitation of the driest quarter (BIO 17) to Vigna species as found in this study may relate to their reproductive fitness and adaptation to arid- and semi-arid areas. Given that reproduction and maturity in the modelled species largely coincide with the driest quarter, precipitation during this period might be important for gamete formation and viability, pod-set, and pod-filling (Nadeem et al., 2019). It may also play a role in seed dormancy (Smýkal et al., 2014). Precipitation of the driest quarter was also found to be one of the most important predictor variables for four legume species (Adenocarpus mannii, Afzelia bella, Afzelia bipindensis, and Baphia nitida) from the Nigeria—Cameroon border in West Africa (Salako et al., 2021) and for A. digitata in Benin (Assogba et al., 2022). Except for species that largely thrive in wet areas including V. laurentii, V. luteola, and V. multinervis, most Vigna CWR species are renowned for their persistence in arid areas, partly owing to their absorptive root systems or tuberous rootstocks (Maxted et al., 2004; Iseki et al., 2018). This might explain why the majority of species in this study demonstrated less demand for heavy precipitation. In Tibet in China, Xin et al. (2021) attributed the absence of excessive demand for precipitation in Sophora moorcroftiana and the distribution of this species in drought-prone areas to its strong absorptive root system.
On the other hand, large isothermality against the affinity for low temperatures as found in this study may, according to Zhang et al. (2018), suggest that the species use the relatively high temperatures during the day for photosynthesis while reserving energy at night through decreased respiration when temperatures are relatively low. Isothermality was suggested to be the second most important predictor factor for barbed goatgrass (Aegilops triuncialis) in Iran (Mousavi Kouhi and Erfanian, 2020), and its importance in shaping the distribution of plant taxa in the tropics has been widely reported (Amissah et al., 2014; Xin et al., 2021; Zuza et al., 2021).
These results provide an understanding of the key environmental factors that are shaping the distribution of wild Vigna species in Benin, and how changes in these factors as a result of future climate might affect the distribution of the studied taxa. This knowledge is critical in effective planning for adaptive management approaches of wild Vigna taxa in the face of climate change (Iriondo et al., 2021).
Several studies have demonstrated that climate change is causing species to shift their climatically suitable habitats towards higher latitudes and elevations (Aguirre-Gutiérrez et al., 2017; Lenoir et al., 2020). The observed northerly expansion in suitable habitats found in this study was thus consistent with these global findings. This partly confirmed the second hypothesis that there would be an increase in the northern habitat. However, it is also becoming apparent that some species may shift their distributions towards other directions (Tagliari et al., 2021; Balima et al., 2022). This was also the case in this study with some models like those of V. frutescens, V. heterophylla, V. racemosa, V. reticulata, and V. unguiculata var. spontanea that appeared to shift their suitable habitats eastwards (Figure 3).
The predicted patterns in the expansions and contractions in the current study might relate to an increase in the number of suitable habitat patches and a reduction in the sizes of those patches, respectively, as was reported by Jarvis et al. (2008). The discrepancies between this study and Jarvis et al. (2008), including the higher contraction rate of over 50% as observed by Jarvis et al. (2008), might be due to the differences in the sources of environmental data, types of species studied, the scale of the study, and the methods used. For example, Jarvis et al. (2008) did not incorporate edaphic variables in their study. Bertrand et al. (2012) suggested that edaphic factors may increase the tolerance of a species in confronting climate constraints, which could have been the case in the current study.
Climatic models have predicted that global climate change will lead to increased night temperatures and prolonged droughts, with West Africa being one of the most affected regions (IPCC, 2021). As a result, lower isothermality values and reduced precipitation of the driest quarter around the 2055s are anticipated than currently observed (Platts et al., 2015). The predicted contractions and expansions in the suitable habitats in the mid-Century observed in the studied species might partly reflect these changes.
The suggested minimum negative effects of climate change on the habitat suitability distribution of the seven species (V. frutescens, V. heterophylla, V. laurentii, V. nigritia, V. oblongifolia, V. racemosa, and V. reticulata) (Figures 3, 4) may be explained by several factors. First, the stability in the key predictor variables, for species like V. frutescens which appeared to be spatially confined to the north of Benin (Figure 1). Second, the relatively higher habitat heterogeneity that may provide a wider range of microhabitat options (Jarvis et al., 2008; Foden et al., 2019) for species such as V. heterophylla, V. racemosa, and V. reticulata. Indeed, these three species were among the most spatially distributed, spanning the different eco-geographical zones of Benin (Figure 1) and were predicted to have comparably wider habitat distributions under the present and future conditions (Figure 3). According to Hirst et al. (2017), common taxa as these within a clade are expected to perform relatively well across a wider range of novel environmental conditions than their rarer relatives. Lastly, it might be due the constraining influence of edaphic factors (Bertrand et al., 2012), especially on the narrowly-distributed species like V. laurentii, V. nigritia, and V. oblongifolia. These three species were among the least spatially distributed (Figure 1) and appear to have specialised habitats, with a predilection for marshy or seasonally inundated areas that have poor soils (Supplementary Table 1).
The habitat distribution maps generated in this study provide insights into potentially suitable habitats for the 15 Vigna taxa which may help in planning for taxa-targeted conservation measures including in situ and ex situ approaches. Further, they may aid in the promotion of cultivation of populations of some taxa to enhance their direct use by local communities so as to diversify the quantity and quality of the food basket, while acting as an incentive for the conservation of wild populations.
The area that was predicted to remain stable under future climatic conditions may be considered for conservation (Iriondo et al., 2021), since stability suggests the existence of favourable climatic conditions that may provide refugia for genetic diversity of species (Cobben et al., 2011). Given that the creation of new PAs solely for the conservation of CWRs may have huge cost implications (Maxted et al., 2016), and may also escalate the existing human-conservation conflicts over land (Tranquilli et al., 2014), the existing PA network together with its bordering landscape in the predicted stable sites is thus recommended for the conservation of Vigna species.
The suggested PAs in this regard include (not in the order of importance) Oueme Superior, Wari-Maro, Mont Kouffe, Agoua, Savalou, Oueme Boukou, and Dogo Forests [in the Sudano-Guinean Zone]; Pendjari National Park [Sudanian Zone]; and Lama, Ahozou (Pahou) and Drabo Gbo Forests [Guinean Zone]. However, it has been suggested that aligning these sites with hotspots of other plant species would help optimise conservation resources (Maxted et al., 2016; Vincent et al., 2022). In this respect, the relative high species richness and endemism in Pendjari National Park (Akoegninou et al., 2006; Neuenschwander et al., 2011) and its high legal protection status (Neuenschwander et al., 2011) would probably make conservation efforts within and around Pendjari National Park more capturing, less expensive, and practically manageable.
For effective conservation efforts in the suggested conservation sites, the following are recommended: species population monitoring, floristic inventories, habitat characterisation, genetic diversity, and ethnobotanical studies (Iriondo et al., 2008; Jarvis et al., 2015; FAO, 2017). Equally important are studies on the reproductive ecology, seedling recruitment, seed longevity, dispersal mechanisms, and responses to abiotic and biotic stresses of Vigna CWR species. Given that nearly 40 of the estimated 63 Africa Vigna species (54 out of an estimated 105 Vigna spp. globally) have presently been assessed using the IUCN Red List Categories and Criteria (https://www.iucnredlist.org) (accessed 02 June 2021), a risk assessment of the remaining 23 African Vigna spp. (51 spp. globally) is also recommended.
The favourable conditions in disturbed areas outside PAs where most CWRs have often persisted for long periods (Jarvis et al., 2015), coupled with slight increases in potentially suitable habitats in non-PAs (Figure 3) appeared to have rendered the PA network cover a comparably small habitat suitability area for the modelled species. This finding supported the third hypothesis that the existing PA network in Benin would be less effective in conserving Vigna CWR diversity than the non-PAs. This poses a threat to the conservation of these species, considering the increasing anthropogenic pressure outside the PAs (Guidigan et al., 2019), which, if taken into account, would substantially reduce the predicted suitable habitat (Riordan and Rundel, 2014). These results also corroborate previous reports of increased mismatches between climatically suitable habitats for CWRs and existing PAs, such as in Ethiopia (Davis et al., 2019), Mexico (Lira et al., 2009), the Netherlands (Aguirre-Gutiérrez et al., 2017), and Sri Lanka (Ratnayake et al., 2021). These results, therefore, buttress the calls for effective conservation of CWRs both within and outside PAs, using an integration of multiple approaches (Riordan and Nabhan, 2019; Iriondo et al., 2021) that takes into account “other effective area-based conservation measures” (OECMs) (IUCN, 2019), since PAs may not be the only best option (Goettsch et al., 2021). To achieve this, and for long-term monitoring and active management that involves participation of various stakeholders including local communities, a wide range of guidelines have long been made available (e.g., Iriondo et al., 2008, 2021; Maxted and Kell, 2009; Hunter and Heywood, 2011; FAO, 2017; IUCN, 2019).
The main caveat of this study is that the models represent only potentially suitable habitats for the modelled species. Distribution, access and persistence may be controlled by many other factors including anthropogenic such as land use, biotic interactions such as pollination, parasitism and diseases, and dispersal (Feng et al., 2020; Spicer et al., 2021). This study did not consider these factors, and, therefore, caution should be exercised when interpreting these results.
Although MaxEnt has proved to be a robust SDM tool in delineating habitat suitability maps for many taxa under the changing climate (e.g., Aguirre-Gutiérrez et al., 2017; Phillips et al., 2017; Ratnayake et al., 2021), the use of a single SDM algorithm does not provide for comparisons, and therefore accuracy in predictions may be put into question (Zuza et al., 2021). Future studies may consider a combination of models.
Another weakness of this study could be the use of publicly available occurrence data from the herbarium and/or online databases. It has been posited that such data may not randomly sample the true occurrences of species since such data is often biassed towards easily accessible areas such as roads and human settlements (Barlow et al., 2021). According to Barlow et al. (2021), such a spatial bias may present an over-representation of environmental conditions associated with regions of higher sampling effort. While this shortcoming is recognised, it should also be pointed out that scientific evidence is growing indicating that CWRs are often associated with such anthropogenically disturbed areas (Jarvis et al., 2015; Iriondo et al., 2021). As such, given that sites that have been accessible to species for a long time are ideal for species distribution modelling (Barve et al., 2011), it might as well be argued that data from such disturbed sites may provide a true reflection of the potential habitat distribution ranges of these taxa.
Nevertheless, this study has identified potential areas for prioritising conservation efforts for the 15 Vigna taxa in Benin. Further, it has raised a red flag for species that may need more attention, considering the predicted vulnerability of their potentially suitable habitats. The taxa include V. comosa, V. filicaulis, V. multinervis, V. unguiculata subsp. baoulensis, and V. unguiculata var. spontanea. Likewise, close attention ought to be paid to the predicted locally narrowly-distributed species, V. laurentii and V. oblongifolia. Although the suitable habitats for these two species were suggested to be negligibly affected by climate change, the species may fail to move and thus become predisposed to intense anthropogenic pressure (Qu et al., 2018; Corlett and Tomlinson, 2020; Spicer et al., 2021). Especially worrying about the two models of V. laurentii and V. oblongifolia is that their distribution ranges are localised at the southern tip of Benin, where land-use change is the greatest due to increasing urbanisation and agriculture (Guidigan et al., 2019). Moreover, V. laurentii is classified as Endangered on the IUCN Red List of species with a declining population (McFarlane and Maxted, 2019). Therefore, population monitoring of the seven species ought to be considered to inform the development of appropriate area-and species-based measures. Equally important are complimentary ex situ collections for long-term conservation of these taxa.
Understanding the habitat suitability distribution range of a species under the changing climate is crucial for its effective conservation planning. Using MaxEnt, occurrence records of 15 Vigna CWR taxa and a combination of climatic and edaphic factors in Benin for the 2055-time horizon, this study for the first time evaluated the effects of climate change on the distribution of multiple Vigna CWR taxa at a local scale. The model showed that climatic factors that shape the distribution of species are likely to change with future climate, consequently resulting in negative or positive changes in the distribution ranges of potentially suitable habitats of the species. The study concludes that in situ conservation of CWRs using the existing PA network alone may not be the only best option. Therefore, to effectively conserve Vigna CWR diversity, an integration of multiple in situ and ex situ conservation approaches (Iriondo et al., 2021) taking into account “other effective area-based conservation measures” (OECMs) (IUCN, 2019; Iriondo et al., 2021) is recommended to inform appropriate area-based and species-based conservation actions (Heywood, 2019). This study provides a crucial step towards the development of sustainable conservation strategies for Vigna CWRs in Benin and West Africa. It also provides a stepping stone for generating hypotheses about mechanistic links between Vigna CWR taxa and their environment (Kearney, 2006).
The original contributions presented in the study are included in the article/Supplementary Material. Further inquiries can be directed to the corresponding author.
AEA and RI conceived the idea and together with CA supervised the work. LM collected the data, ran the models, and drafted the manuscript. All the authors read the manuscript and gave consent for publication.
This study was supported by the Regional Universities Forum for Capacity Building in Agriculture (RUFORUM) through the Intra-Africa—Partnership for Training Regional academic exchange for enhanced skills in fragile ecosystems management in Africa (REFORM) scholarship (2018-2022). RI acknowledges support from the Rufford Foundation (Grant 31042-D) which provided a foundation for the current project.
The authors declare that the research was conducted in the absence of any commercial or financial relationships that could be construed as a potential conflict of interest.
All claims expressed in this article are solely those of the authors and do not necessarily represent those of their affiliated organizations, or those of the publisher, the editors and the reviewers. Any product that may be evaluated in this article, or claim that may be made by its manufacturer, is not guaranteed or endorsed by the publisher.
The authors are grateful to the funders for providing a Ph.D., scholarship to LM. Messrs. Gafarou Agounde and Medard Kafoutchoni for helping with model running and cartographic work. The invaluable comments made by reviewers on the draft manuscript. Otherwise, omissions and errors are our responsibility.
The Supplementary Material for this article can be found online at: https://www.frontiersin.org/articles/10.3389/fcosc.2022.870041/full#supplementary-material
Abdelghany, G., Wurm, P., Hoang, L. T. M., and Bellairs, S. M. (2021). Commercial cultivation of Australian wild Oryza spp.: a review and conceptual framework for future research needs. Agronomy 12, 42. doi: 10.3390/agronomy12010042
Abrha, H., Birhane, E., Hagos, H., and Manaye, A. (2018). Predicting suitable habitats of endangered Juniperus procera tree under climate change in Northern Ethiopia. J. Sustain. For. 37, 842–853. doi: 10.1080/10549811.2018.1494000
Adamo, M., Chialva, M., Calevo, J., Bertoni, F., Dixon, K., and Mammola, S. (2021). Plant scientists' research attention is skewed towards colourful, conspicuous and broadly distributed flowers. Nat. Plants 7, 574–578. doi: 10.1038/s41477-021-00912-2
Adomou, A. C., Sinsin, B., and Van Der Maesen, L. J. G. (2006). Phytosociological and chorological approaches to phytogeography: a meso-scale study in Benin. Syst. Geogr. Plants 76, 155–178.
Aguirre-Gutiérrez, J., van Treuren, R., Hoekstra, R., and van Hintum, T. J. L. (2017). Crop wild relatives range shifts and conservation in Europe under climate change. Divers. Distrib. 23, 739–750. doi: 10.1111/ddi.12573
Akoegninou, A., van der Burg, W. J., and van der Maesen, L. J. G. (2006). Flore Analytique de Bénin. Leiden: Backhuys Publication.
Allouche, O., Tsoar, A., and Kadmon, R. (2006). Assessing the accuracy of species distribution models: prevalence, kappa and the true skill statistic (TSS). J. Appl. Ecol. 43, 1223–1232. doi: 10.1111/j.1365-2664.2006.01214.x
Amissah, L., Mohren, G. M. J., Bongers, F., Hawthorne, W. D., and Poorter, L. (2014). Rainfall and temperature affect tree species distribution in Ghana. J. Trop. Ecol. 30, 435–446. doi: 10.1017/S026646741400025X
Assogba, D., Idohou, R., Chirwa, P., and Assogbadjo, A. E. (2022). On opportunities and challenges to conserve the African baobab under present and future climates in Benin (West Africa). J. Arid Environ.198, 104692. doi: 10.1016/j.jaridenv.2021.104692
Assogbadjo, A. E., Kakaï, R. G., Adjallala, F. H., Azihou, A. F., Vodouhê, G. F., Kyndt, T., et al. (2011). Ethnic differences in use value and use patterns of the threatened multipurpose scrambling shrub (Caesalpinia bonduc L.) in Benin. J. Med. Plants Res. 5, 1549–1557.
Balima, L. H., Nacoulma, B. M. I., Da, S. S., Ouédraogo, A., Soro, D., and Thiombiano, A. (2022). Impacts of climate change on the geographic distribution of African oak tree (Afzelia africana Sm.) in Burkina Faso, West Africa. Heliyon 8, e08688. doi: 10.1016/j.heliyon.2021.e08688
Barlow, M. M., Johnson, C. N., McDowell, M. C., Fielding, M. W., Amin, R. J., and Brewster, R. (2021). Species distribution models for conservation: identifying translocation sites for eastern quolls under climate change. Glob. Ecol. Conserv. 29, e01735. doi: 10.1016/j.gecco.2021.e01735
Barve, N., Barve, V., Jiménez-Valverde, A., Lira-Noriega, A., Maher, S. P., Peterson, A. T., et al. (2011). The crucial role of the accessible area in ecological niche modeling and species distribution modeling. Ecol. Modell. 222, 1810–1819. doi: 10.1016/j.ecolmodel.2011.02.011
Bellard, C., Bertelsmeier, C., Leadley, P., Thuiller, W., and Courchamp, F. (2012). Impacts of climate change on the future of biodiversity. Ecol. Lett. 15, 365–377. doi: 10.1111/j.1461-0248.2011.01736.x
Bellon, M. R., Dulloo, E., Sardos, J., Thormann, I., and Burdon, J. J. (2017). In situ conservation—harnessing natural and human-derived evolutionary forces to ensure future crop adaptation. Evol. Appl. 10, 965–977. doi: 10.1111/eva.12521
Bertrand, R., Perez, V., and Gégout, J. C. (2012). Disregarding the edaphic dimension in species distribution models leads to the omission of crucial spatial information under climate change: the case of Quercus pubescens in France. Glob. Chang. Biol. 18, 2648–2660. doi: 10.1111/j.1365-2486.2012.02679.x
Bohra, A., Kilian, B., Sivasankar, S., Caccamo, M., Mba, C., McCouch, S. R., et al. (2021). Reap the crop wild relatives for breeding future crops. Trends Biotechnol. 40, 412–431. doi: 10.1016/j.tibtech.2021.08.009
Castañeda-Álvarez, N. P., Khoury, C. K., Achicanoy, H. A., Bernau, V., Dempewolf, H., Eastwood, R. J., et al. (2016). Global conservation priorities for crop wild relatives. Nat. Plants 2, 16022. doi: 10.1038/nplants.2016.22
Catarino, S., Rangel, J., Darbyshire, I., Costa, E., Duarte, M. C., and Romeiras, M. M. (2021). Conservation priorities for African Vigna species: unveiling angola's diversity hotspots. Glob. Ecol. Conserv. 25, e01415. doi: 10.1016/j.gecco.2020.e01415
CBD (2005). Handbook of the Convention on Biological Diversity Including Its Cartagena Protocol on Biosafety, 3rd ed. Montreal, QC: Secretariat of the Convention on Biological Diversity.
CBD (2010). The Strategic Plan for Biodiversity (2011–2020) and the Aichi Biodiversity Targets. Secretariat of the Convention on Biological Diversity, Montreal, Cananda. Available online at: https://www.cbd.int/sp/ (accessed March 22, 2022).
Çoban, H. O., Örücü, Ö. K., and Arslan, E.S. (2020). Maxent modeling for predicting the current and future potential geographical distribution of Quercus libani Olivier. Sustainability 12, 1–17. doi: 10.3390/su12072671
Cobben, M. M. P., Verboom, J., Opdam, P. F. M., Hoekstra, R. F., Jochem, R., Arens, P., et al. (2011). Projected climate change causes loss and redistribution of genetic diversity in a model metapopulation of a medium-good disperser. Ecography 34, 920–932. doi: 10.1111/j.1600-0587.2011.06713.x
Corlett, R. T., and Tomlinson, K. W. (2020). climate change and edaphic specialists: irresistible force meets immovable object? Trends Ecol. Evol. 35, 367–376. doi: 10.1016/j.tree.2019.12.007
Davis, A. P., Chadburn, H., Moat, J., O'Sullivan, R., Hargreaves, S., and Lughadha, E. N. (2019). High extinction risk for wild coffee species and implications for coffee sector sustainability. Sci. Adv. 5, eaav3473. doi: 10.1126/sciadv.aav3473
Dempewolf, H., Baute, G., Anderson, J., Kilian, B., Smith, C., and Guarino, L. (2017). Past and future use of wild relatives in crop breeding. Crop Sci. 57, 1070–1082. doi: 10.2135/cropsci2016.10.0885
Dempewolf, H., Eastwood, R. J., Guarino, L., Khoury, C. K., Müller, J. V., and Toll, J. (2014). Adapting agriculture to climate change: a global initiative to collect, conserve, and use crop wild relatives. Agroecol. Sustain. Food Syst. 38, 369–377. doi: 10.1080/21683565.2013.870629
Dormann, C. F., Elith, J., Bacher, S., Buchmann, C., Carl, G., Carr,é, G., et al. (2013). Collinearity: A review of methods to deal with it and a simulation study evaluating their performance. Ecography 36, 27–46. doi: 10.1111/j.1600-0587.2012.07348.x
Elith, J., and Graham, C. H. (2009). Do they? How do they? WHY do they differ? on finding reasons for differing performances of species distribution models. Ecography 32, 66–77. doi: 10.1111/j.1600-0587.2008.05505.x
Elith, J., Phillips, S. J., Hastie, T., Dudík, M., Chee, Y. E., and Yates, C. J. (2011). A statistical explanation of MaxEnt for ecologists. Divers. Distrib. 17, 43–57. doi: 10.1111/j.1472-4642.2010.00725.x
FAO (2009). International Treaty on Plant Genetic Resources for Food and Agriculture. Rome: FAO. Available online at: https://www.fao.org/3/i0510e/i0510e.pdf (accessed December 4, 2021).
FAO (2017). Voluntary Guidelines for the Conservation and Sustainable Use of Crop Wild Relatives and Wild Food Plants. Rome: Food and Agriculture Organization of the United Nations.
Farooq, M., Nadeem, F., Gogoi, N., Ullah, A., Alghamdi, S. S., Nayyar, H., et al. (2017). Heat stress in grain legumes during reproductive and grain-filling phases. Crop Pasture Sci. 68, 985–1005. doi: 10.1071/CP17012
Favi, G. A., Dassou, G. H., Agound,é, G., Ouachinou, J. M. A. S., Djidohokpin, D., Adomou, A. C., et al. (2021). Current and future distribution pattern of Cochlospermum planchonii and Cochlospermum tinctorium in Benin (West Africa), in response to climate change scenario. Model. Earth Syst. Environ. 8, 1–14. doi: 10.1007/s40808-021-01109-4
Feng, L., Sun, J., Shi, Y., Wang, G., and Wang, T. (2020). Predicting suitable habitats of Camptotheca acuminata considering both climatic and soil variables. Forests 11, 891. doi: 10.3390/f11080891
Feng, X., Park, D. S., Liang, Y., Pandey, R., and Papeş, M. (2019). Collinearity in ecological niche modeling: confusions and challenges. Ecol. Evol. 9, 10365–10376. doi: 10.1002/ece3.5555
Fielding, A. H., and Bell, J. F. (1997). A review of methods for the assessment of prediction errors in conservation presence/absence models. Environ. Conserv. 24, 38–49. doi: 10.1017/S0376892997000088
Fitzgerald, H., Palmé, A., Asdal, Å., Endresen, D., Kiviharju, E., Lund, B., et al. (2019). A regional approach to Nordic crop wild relative in situ conservation planning. Plant Genet. Resour. Character. Util. 17, 196–207. doi: 10.1017/S147926211800059X
Foden, W. B., Young, B. E., Akçakaya, H. R., Garcia, R. A., Hoffmann, A. A., Stein, B. A., et al. (2019). Climate change vulnerability assessment of species. Wiley Interdiscip. Rev. Clim. Chang. 10, 1–36. doi: 10.1002/wcc.551
Godfray, H. C. J. (2014). The challenge of feeding 9-10 billion people equitably and sustainably. J. Agric. Sci. 152, S2–S8. doi: 10.1017/S0021859613000774
Goebes, P., Schmidt, K., Seitz, S., Both, S., Bruelheide, H., Erfmeier, A., et al. (2019). The strength of soil-plant interactions under forest is related to a critical soil depth. Sci. Rep. 9, 8635. doi: 10.1038/s41598-019-45156-5
Goettsch, B., Urquiza-Haas, T., Koleff, P., Acevedo Gasman, F., Aguilar-Meléndez, A., Alavez, V., et al. (2021). Extinction risk of mesoamerican crop wild relatives. Plants People Planet 3, 775–795. doi: 10.1002/ppp3.10225
Guidigan, M. L. G., Sanou, C. L., Ragatoa, D. S., Fafa, C. O., and Mishra, V. N. (2019). Assessing land use/land cover dynamic and its impact in benin republic using land change model and CCI-LC products. Earth Syst. Environ. 3, 127–137. doi: 10.1007/s41748-018-0083-5
Guisan, A., Tingley, R., Baumgartner, J. B., Naujokaitis-Lewis, I., Sutcliffe, P. R., Tulloch, A. I. T., et al. (2013). Predicting species distributions for conservation decisions. Ecol. Lett. 16, 1424–1435. doi: 10.1111/ele.12189
Habibullah, M. S., Din, B. H., Tan, S. H., and Zahid, H. (2022). Impact of climate change on biodiversity loss: global evidence. Environ. Sci. Pollut. Res. 29, 1073–1086. doi: 10.1007/s11356-021-15702-8
Hageer, Y., Esperón-Rodríguez, M., Baumgartner, J. B., and Beaumont, L. J. (2017). Climate, soil or both? Which variables are better predictors of the distributions of Australian shrub species? PeerJ 5, e3446. doi: 10.7717/peerj.3446
Hajjar, R., and Hodgkin, T. (2007). The use of wild relatives in crop improvement: a survey of developments over the last 20 years. Euphytica 156, 1–13. doi: 10.1007/s10681-007-9363-0
Harouna, D. V., Venkataramana, P. B., Ndakidemi, P. A., and Matemu, A. O. (2018). Under-exploited wild Vigna species potentials in human and animal nutrition: a review. Glob. Food Sec. 18, 1–11. doi: 10.1016/j.gfs.2018.06.002
Hengl, T., De Jesus, J. M., Heuvelink, G. B. M., Gonzalez, M. R., Kilibarda, M., Blagotić, A., et al. (2017). SoilGrids250m: global gridded soil information based on machine learning. PLoS ONE 12, e0169748. doi: 10.1371/journal.pone.0169748
Heywood, V. H. (2019). Conserving plants within and beyond protected areas—still problematic and future uncertain. Plant Divers. 41, 36–49. doi: 10.1016/j.pld.2018.10.001
Hirst, M. J., Griffin, P. C., Sexton, J. P., and Hoffmann, A. A. (2017). Testing the niche-breadth–range-size hypothesis: habitat specialization vs. performance in Australian alpine daisies. Ecology 98, 2708–2724. doi: 10.1002/ecy.1964
Hounkpatin, K. O. L., Bossa, A. Y., Yira, Y., Igue, M. A., and Sinsin, B. A. (2022). Assessment of the soil fertility status in Benin (West Africa)—digital soil mapping using machine learning. Geoderma Reg. 28, e00444. doi: 10.1016/j.geodrs.2021.e00444
Hoveka, L. N., van der Bank, M., and Davies, T. J. (2022). Winners and losers in a changing climate: how will protected areas conserve red list species under climate change? Divers. Distrib. 28, 1–11. doi: 10.1111/ddi.13488
Hunter, D., and Heywood, V. (2011). Crop Wild Relatives: A Manual of in situ Conservation. London; Washington, DC: Earthscan.
Idohou, R., Assogbadjo, A. E., Fandohan, B., Gouwakinnou, G. N., Glele Kakai, R. L., Sinsin, B., et al. (2013). National inventory and prioritization of crop wild relatives: case study for Benin. Genet. Resour. Crop Evol. 60, 1337–1352. doi: 10.1007/s10722-012-9923-6
Idohou, R., Assogbadjo, A. E., Kakaï, R. G., and Peterson, A. T. (2017a). Spatio-temporal dynamic of suitable areas for species conservation in West Africa: eight economically important wild palms under present and future climates. Agrofor. Syst. 91, 527–540. doi: 10.1007/s10457-016-9955-6
Idohou, R., Townsend Peterson, A., Assogbadjo, A. E., Vihotogbe, R. L., Padonou, E., and Glèlè Kakaï, R. (2017b). Identification of potential areas for wild palm cultivation in the Republic of Benin through remote sensing and ecological niche modeling. Genet. Resour. Crop Evol. 64, 1383–1393. doi: 10.1007/s10722-016-0443-7
IPCC (2021). “Summary for policymakers,” in Climate Change 2021: The Physical Science Basis. Contribution of Working Group I to the Sixth Assessment Report of the Intergovernmental Panel on Climate Change, eds V. Masson-Delmotte, P. Zhai, A. Pirani, S. L. Connors, C. Péan, S. Berger, N. Caud, Y. Chen, L. Goldfarb, M. I. Gomis, M. Huang, K. Leitzell, E. Lonnoy, J. B. R. Matthews, T. K. Maycock, T. Waterfield, O. Yelekçi, R. Yu, and B. Zhou (Geneva: Intergovernmental Panel on Climate Change), 40.
Iriondo, J. M., Magos Brehm, J., Dulloo, M. E., and Maxted, N, (eds). (2021). Crop wild relative population management guidelines. Farmer's Pride: Networking, Partnerships and Tools to Enhance in situ Conservation of European Plant Genetic Resources. Available online at: http://www.farmerspride.eu/ (accessed March 26, 2022).
Iriondo, J. M., Maxted, N., and Dulloo, M. E. (2008). Conserving Plant Genetic Diversity in Protected Areas: Population Management of Crop Wild Relatives. Wallingford: CAB International. doi: 10.1079/9781845932824.0000
Iseki, K., Takahashi, Y., Muto, C., Naito, K., and Tomooka, N. (2018). Diversity of drought tolerance in the genus Vigna. Front. Plant Sci. 9, 729. doi: 10.3389/fpls.2018.00729
IUCN (2019). Recognising and Reporting Other Effective Area-Based Conservation Measures. Gland: IUCN, International Union for Conservation of Nature.
Jaradat, A. A. (2015). “Beyond biodiversity: ecosystem services of crop wild relatives, in Crop Wild Relatives and Climate Change, eds R. Redden, S. S. Yadav, N. Maxted, E. Dulloo, L. Guarino, and P. Smith (Hoboken, NJ: John Wiley & Sons, Inc), 336–349. doi: 10.1002/9781118854396.ch19
Jarvis, A., Lane, A., and Hijmans, R. J. (2008). The effect of climate change on crop wild relatives. Agric. Ecosyst. Environ. 126, 13–23. doi: 10.1016/j.agee.2008.01.013
Jarvis, S., Fielder, H., Hopkins, J., Maxted, N., and Smart, S. (2015). Distribution of crop wild relatives of conservation priority in the UK landscape. Biol. Conserv. 191, 444–451. doi: 10.1016/j.biocon.2015.07.039
Kearney, M. (2006). Habitat, environment and niche: what are we modelling? Oikos 115, 186–191. doi: 10.1111/j.2006.0030-1299.14908.x
Khoury, C. K., Carver, D., Kates, H. R., Achicanoy, H. A., van Zonneveld, M., Thomas, E., et al. (2020). Distributions, conservation status, and abiotic stress tolerance potential of wild cucurbits (Cucurbita L.). Plants People Planet 2, 269–283. doi: 10.1002/ppp3.10085
Lenoir, J., Bertrand, R., Comte, L., Bourgeaud, L., Hattab, T., Murienne, J., et al. (2020). Species better track climate warming in the oceans than on land. Nat. Ecol. Evol. 4, 1044–1059. doi: 10.1038/s41559-020-1198-2
Liebenberg, A., van der Nest, J. R., Hardie, A. G., Labuschagne, J., and Swanepoel, P. A. (2020). Extent of soil acidity in no-tillage systems in the western cape province of South Africa. Land 9, 361. doi: 10.3390/land9100361
Lima, D. F., Mello, J. H. F., Lopes, I. T., Forzza, R. C., Goldenberg, R., and Freitas, L. (2021). Phenological responses to climate change based on a hundred years of herbarium collections of tropical Melastomataceae. PLoS One 16:e0251360. doi: 10.1371/journal.pone.0251360
Lima, V. P., de Lima, R. A. F., Joner, F., Siddique, I., Raes, N., and ter Steege, H. (2022). Climate change threatens native potential agroforestry plant species in Brazil. Sci. Rep. 12, 2267. doi: 10.1038/s41598-022-06234-3
Lira, R., Téllez, O., and Dávila, P. (2009). The effects of climate change on the geographic distribution of Mexican wild relatives of domesticated Cucurbitaceae. Genet. Resour. Crop Evol. 56, 691–703. doi: 10.1007/s10722-008-9394-y
Lissovsky, A. A., and Dudov, S. V. (2021). Species-distribution modeling: advantages and limitations of its application. 2. MaxEnt. Biol. Bull. Rev. 11, 265–275. doi: 10.1134/S2079086421030087
Liu, D., Lei, X., Gao, W., Guo, H., Xie, Y., Fu, L., et al. (2022). Mapping the potential distribution suitability of 16 tree species under climate change in northeastern China using Maxent modelling. J. For. Res. doi: 10.1007/s11676-022-01459-4
Lompo, O., Dimobe, K., Mbayngone, E., Savadogo, S., Sambaré, O., Thiombiano, A., et al. (2021). Climate influence on the distribution of the yellow plum (Ximenia americana L.) in Burkina Faso. Trees For. People 4, 100072. doi: 10.1016/j.tfp.2021.100072
Lush, W. M., Evans, L. T., and Wien, H. C. (1980). Environmental adaptation of wild and domesticated cowpeas [Vigna unguiculata (L.) walp.]. F. Crop. Res. 3, 173–187. doi: 10.1016/0378-4290(80)90023-4
Maxted, N., Amri, A., Castañeda-Álvarez, N. P., Dias, S., Dulloo, M. E., Fielder, H., et al. (2016). “Joining up the dots: a systematic perspective of crop wild relative conservation and use,” in Enhancing Crop Genepool Use: Capturing Wild Relative and Landrace Diversity for Crop Improvement, eds N. Maxted, M. E. Dulloo, and B. V. Ford-Lloyd (Wallingford: CABI), 87–124. doi: 10.1079/9781780646138.0087
Maxted, N., Ford-Lloyd, B. V., Jury, S., Kell, S., and Scholten, M. (2006). Towards a definition of a crop wild relative. Biodivers. Conserv. 15, 2673–2685. doi: 10.1007/s10531-005-5409-6
Maxted, N., Kell, S., and Brehm, J. M. (2011). Options to Promote Food Security: On-Farm Management and in situ Conservation of Plant Genetic Resources for Food and Agriculture. Commission on Genetic Resources for Food and Agriculture. Available online at: http://www.fao.org/docrep/meeting/022/am489e.pdf (accessed March 8, 2022).
Maxted, N., and Kell, S. P. (2009). Establishment of a Global Network for the in situ Conservation of Crop Wild Relatives: Status and Needs. Rome: FAO Commission on Genetic Resources for Food and Agriculture. Available online at: http://www.fao.org/3/i1500e/i1500e18d.pdf (accessed May 10, 2021).
Maxted, N., Mabuza-Diamini, P., Moss, H., Padulosi, S., Jarvis, A., and Guarino, L. (2004). An Ecogeographic Study: African Vigna, An Ecogeographic Study: African Vigna. Rome: International Plant Genetic Resources Centre.
McFarlane, D., and Maxted, N. (2019). Vigna laurentii. Gland: The IUCN Red List of Threatened Species 2019.
Meng, J., Li, M., Guo, J., Zhao, D., and Tao, J. (2021). Predicting suitable environments and potential occurrences for Cinnamomum camphora (Linn.) presl. Forests 12, 18–21. doi: 10.3390/f12081126
Merow, C., Smith, M. J., and Silander, J. A. (2013). A practical guide to MaxEnt for modeling species' distributions: what it does, and why inputs and settings matter. Ecography 36, 1058–1069. doi: 10.1111/j.1600-0587.2013.07872.x
Miryeganeh, M., Takayama, K., Tateishi, Y., and Kajita, T. (2014). Long-distance dispersal by sea-drifted seeds has maintained the global distribution of Ipomoea pescaprae subsp. brasiliensis (Convolvulaceae). PLoS ONE 9, e0091836. doi: 10.1371/journal.pone.0091836
Mod, H. K., Scherrer, D., Luoto, M., and Guisan, A. (2016). What we use is not what we know: environmental predictors in plant distribution models. J. Veg. Sci. 27, 1308–1322. doi: 10.1111/jvs.12444
Moray, C., Game, E. T., and Maxted, N. (2014). Prioritising in situ conservation of crop resources: a case study of African cowpea (Vigna unguiculata). Sci. Rep. 4, 5247. doi: 10.1038/srep05247
Mousavi Kouhi, S. M., and Erfanian, M. B. (2020). Predicting the present and future distribution of medusahead and barbed goatgrass in Iran. Ecopersia 8, 41–46.
Mponya, N., Chanyenga, T., Magos Brehm, J., and Maxted, N. (2021). In situ and ex situ conservation gap analyses of crop wild relatives from Malawi. Genet. Resour. Crop Evol. 68, 759–771. doi: 10.1007/s10722-020-01021-3
Müller, C., and Robertson, R. D. (2014). Projecting future crop productivity for global economic modeling. Agric. Econ. (United Kingdom) 45, 37–50. doi: 10.1111/agec.12088
Nadeem, M., Li, J., Yahya, M., Sher, A., Ma, C., Wang, X., et al. (2019). Research progress and perspective on drought stress in legumes: a review. Int. J. Mol. Sci. 20, 2541. doi: 10.3390/ijms20102541
Nduche, M., Magos Brehm, J., Abberton, M., Omosun, G., and Maxted, N. (2021). West African crop wild relative checklist, prioritization and inventory. Genet. Resour. 2, 55–65. doi: 10.46265/genresj.EIFL1323
Neuenschwander, P., Sinsin, B., and Goergen, G. E. (2011). Protection de la nature en Afrique de l'Ouest: une liste rouge pour le Bénin, International Institute of Tropical Agriculture. Ibadan: International Institute of Tropical Agriculture (IITA).
Ortiz-Bobea, A., Ault, T. R., Carrillo, C. M., Chambers, R. G., and Lobell, D. B. (2021). Anthropogenic climate change has slowed global agricultural productivity growth. Nat. Clim. Chang. 11, 306–312. doi: 10.1038/s41558-021-01000-1
Pacifici, M., Foden, W. B., Visconti, P., Watson, J. E. M., Butchart, S. H. M., Kovacs, K. M., et al. (2015). Assessing species vulnerability to climate change. Nat. Clim. Chang. 5, 215–225. doi: 10.1038/nclimate2448
Pang, S. E. H., De Alban, J. D. T., and Webb, E. L. (2021). Effects of climate change and land cover on the distributions of a critical tree family in the Philippines. Sci. Rep. 11, 276. doi: 10.1038/s41598-020-79491-9
Parker, T. A., Lo, S., and Gepts, P. (2021). Pod shattering in grain legumes: emerging genetic and environment-related patterns. Plant Cell 33, 179–199. doi: 10.1093/plcell/koaa025
Phillips, J., Magos Brehm, J., van Oort, B., Asdal, Å., Rasmussen, M., and Maxted, N. (2017). Climate change and national crop wild relative conservation planning. Ambio 46, 630–643. doi: 10.1007/s13280-017-0905-y
Phillips, S. J., Anderson, R. P., and Schapire, R. E. (2006). Maximum entropy modeling of species geographic distributions. Ecol. Modell. 190, 231–259. doi: 10.1016/j.ecolmodel.2005.03.026
Platts, P. J., Omeny, P. A., and Marchant, R. (2015). AFRICLIM: high-resolution climate projections for ecological applications in Africa. Afr. J. Ecol. 53, 103–108. doi: 10.1111/aje.12180
Politi, N., Rivera, L., Martinuzzi, S., Radeloff, V. C., and Pidgeon, A. M. (2021). Conservation prioritization when species distribution data are scarce. Landsc. Urban Plan. 210, 104067. doi: 10.1016/j.landurbplan.2021.104067
PricewaterhouseCooper (2013). Crop Wild Relatives: A Valuable Resource for Crop Development. PricewaterhouseCooper. Available online at: https://pwc.blogs.com/files/pwc-seed-bank-analysis-for-msb-0713.pdf (accessed April 24, 2019).
Qu, H., Wang, C. J., and Zhang, Z. X. (2018). Planning priority conservation areas under climate change for six plant species with extremely small populations in China. Nat. Conserv. 25, 89–106. doi: 10.3897/natureconservation.25.20063
Ramírez-Rodríguez, R., Melendo-Luque, M., Rus-Moreno, J. D., and Amich, F. (2021). Potential changes in the distribution of Delphinium bolosii and related taxa of the series Fissa from the Iberian Peninsula under future climate change scenarios. Nat. Conserv. 43, 147–166. doi: 10.3897/natureconservation.43.63876
Ratnayake, S. S., Kariyawasam, C. S., Kumar, L., Hunter, D., and Liyanage, A. S. U. (2021). Potential distribution of crop wild relatives under climate change in Sri Lanka: implications for conservation of agricultural biodiversity. Curr. Res. Environ. Sustain. 3, 100092. doi: 10.1016/j.crsust.2021.100092
Riordan, E. C., and Nabhan, G. P. (2019). Trans situ conservation of crop wild relatives. Crop Sci. 59, 2387–2403. doi: 10.2135/cropsci2019.06.0356
Riordan, E. C., and Rundel, P. W. (2014). Land use compounds habitat losses under projected climate change in a threatened California ecosystem. PLoS ONE 9, e0086487. doi: 10.1371/journal.pone.0086487
Roe, N. (2020). Determining the Factors That Drive Understory Plant Species Distribution in the White Mountains of New Hampshire. Durham, NC: University of New Hampshire.
Salako, G., Oyebanji, O. O., Olagunju, T. E., and Howe, G. T. (2021). Potential impact of climate change on the distribution of some selected legumes in Cameroon and adjoining Nigeria border. Afr. J. Ecol. 59, 959–975. doi: 10.1111/aje.12915
Salako, V. K., Vihotogbé, R., Houéhanou, T., Sodé, I. A., and Glèlè Kakaï, R. (2019). Predicting the potential impact of climate change on the declining agroforestry species Borassus aethiopum Mart. in Benin: a mixture of geostatistical and SDM approach. Agrofor. Syst. 93, 1513–1530. doi: 10.1007/s10457-018-0262-2
Santini, L., Benítez-López, A., Maiorano, L., Cengić, M., and Huijbregts, M.A.J. (2021). Assessing the reliability of species distribution projections in climate change research. Divers. Distrib. 27, 1035–1050. doi: 10.1111/ddi.13252
Scridel, D., Brambilla, M., de Zwaan, D. R., Froese, N., Wilson, S., Pedrini, P., et al. (2021). A genus at risk: predicted current and future distribution of all three Lagopus species reveal sensitivity to climate change and efficacy of protected areas. Divers. Distrib. 27, 1759–1774. doi: 10.1111/ddi.13366
Singh, M., Badcock-Scruton, J., and Matilda Collins, C. (2021). What will remain? Predicting the representation in protected areas of suitable habitat for endangered tropical avifauna in borneo under a combined climate- and land-use change scenario. Sustainability 13, 2792. doi: 10.3390/su13052792
Smýkal, P., Vernoud, V., Blair, M. W., Soukup, A., and Thompson, R. D. (2014). The role of the testa during development and in establishment of dormancy of the legume seed. Front. Plant Sci. 5, 351. doi: 10.3389/fpls.2014.00351
Somta, P., Dachapak, S., Yimram, T., Srinives, P., Poonchaivilaisak, S. (2019). Genetic diversity of zombi pea (Vigna vexillata) assessed by microsatellite markers. Acta Hortic. 1241, 143–149. doi: 10.17660/ActaHortic.2019.1241.21
Spicer, M. E., Radhamoni, H. V. N., Duguid, M. C., Queenborough, S. A., and Comita, L. S. (2021). Herbaceous plant diversity in forest ecosystems: patterns, mechanisms, and threats. Plant Ecol. 223, 117–119. doi: 10.1007/s11258-021-01202-9
Stephan, J., Bercachy, C., Bechara, J., Charbel, E., and López-Tirado, J. (2020). Local ecological niche modelling to provide suitability maps for 27 forest tree species in edge conditions. IForest 13, 230–237. doi: 10.3832/ifor3331-013
Tagliari, M. M., Danthu, P., Leong Pock Tsy, J. M., Cornu, C., Lenoir, J., Carvalho-Rocha, V., et al. (2021). Not all species will migrate poleward as the climate warms: the case of the seven baobab species in Madagascar. Glob. Chang. Biol. 27, 6071–6085. doi: 10.1111/gcb.15859
Takahashi, Y., Somta, P., Muto, C., Iseki, K., Naito, K., Pandiyan, M., et al. (2016). Novel genetic resources in the genus vigna unveiled from gene bank accessions. PLoS One 11:e0147568. doi: 10.1371/journal.pone.0147568
Takahashi, Y., and Tomooka, N. (2020). “Taxonomy of mungbean and its relatives.” in The Mungbean Genome, Compendium of Plant Genomes, eds R. M. Nair, R. Schafleitner, S. H. Lee (Cham: Springer International Publishing), 27–41. doi: 10.1007/978-3-030-20008-4_3
Thomas, C. D., and Gillingham, P. K. (2015). The performance of protected areas for biodiversity under climate change. Biol. J. Linn. Soc. 115, 718–730. doi: 10.1111/bij.12510
Tomooka, N., Naito, K., Kaga, A., Sakai, H., Isemura, T., Ogiso-Tanaka, E., et al. (2014). Evolution, domestication and neo-domestication of the genus Vigna. Plant Genet. Resour. Character. Util. 12, 2011–2014. doi: 10.1017/S1479262114000483
Tranquilli, S., Abedi-Lartey, M., Abernethy, K., Amsini, F., Asamoah, A., Balangtaa, C., et al. (2014). Protected areas in tropical Africa: assessing threats and conservation activities. PLoS ONE 9, e0114154. doi: 10.1371/journal.pone.0114154
Trzeciak-Limeira, F., Pinto, D. D., and Mourão, K. S. M. (2013). Pericarp ontogenesis with emphasis on the dispersal apparatus of three weed species of faboideae (Fabaceae). Acta Bot. Brasilica 27, 723–729. doi: 10.1590/S0102-33062013000400011
Tyack, N., Dempewolf, H., and Khoury, C. K. (2020). The potential of payment for ecosystem services for crop wild relative conservation. Plants 9, 1–14. doi: 10.3390/plants9101305
United Nations (2015). Transforming our world: the 2030 Agenda for Sustainable Development. A/RES/70/1 Resolution adopted by the General Assembly on 25 September 2015. Available online at: https://www.un.org/ga/search/view_doc.asp?symbol=A/RES/70/1&Lang=E (accessed April 12, 2022).
United Nations (2021). Benin Population 2021 (Demographics, Maps, Graphs) [WWW Document]. Available online at: https://worldpopulationreview.com/countries/benin-population (accessed December 26, 2021).
van Treuren, R., Hoekstra, R., Wehrens, R., and van Hintum, T. (2020). Effects of climate change on the distribution of crop wild relatives in the Netherlands in relation to conservation status and ecotope variation. Glob. Ecol. Conserv. 23, e01054. doi: 10.1016/j.gecco.2020.e01054
van Zonneveld, M., Rakha, M., Tan, S., Chou, Y. Y., Chang, C. H. Yen, J. Y. Schafleitner, R., et al. (2020). Mapping patterns of abiotic and biotic stress resilience uncovers conservation gaps and breeding potential of Vigna wild relatives. Sci. Rep. 10, 2111. doi: 10.1038/s41598-020-58646-8
Veríssimo, D., Vaughan, G., Ridout, M., Waterman, C., MacMillan, D., and Smith, R. J. (2017). Increased conservation marketing effort has major fundraising benefits for even the least popular species. Biol. Conserv. 211, 95–101. doi: 10.1016/j.biocon.2017.04.018
Vihotogbé, R., Idohou, R., Vianou, A., Spies, P., Salako, V., Assogbadjo, A., et al. (2021). Abundance and effects of climate change on geographical distribution of Mondia whitei (Hook.f.) skeels (Apocynaceae) in the dahomey gap (West Africa). Afr. J. Ecol. 59, 924–933. doi: 10.1111/aje.12914
Vincent, H., Amri, A., Castañeda-Álvarez, N. P., Dempewolf, H., Dulloo, E., Guarino, L., et al. (2019). Modeling of crop wild relative species identifies areas globally for in situ conservation. Commun. Biol. 2, 136. doi: 10.1038/s42003-019-0372-z
Vincent, H., Hole, D., and Maxted, N. (2022). Congruence between global crop wild relative hotspots and biodiversity hotspots. Biol. Conserv. 265, 109432. doi: 10.1016/j.biocon.2021.109432
Vittoz, P., and Engler, R. (2007). Seed dispersal distances: a typology based on dispersal modes and plant traits. Bot. Helv. 117, 109–124. doi: 10.1007/s00035-007-0797-8
Wang, H. H., Wonkka, C. L., Treglia, M. L., Grant, W. E., Smeins, F. E., and Rogers, W. E. (2019). Incorporating local-scale variables into distribution models enhances predictability for rare plant species with biological dependencies. Biodivers. Conserv. 28, 171–182. doi: 10.1007/s10531-018-1645-4
Wang, S., and Hou, F. (2021). Short-term study on the yak dung seed bank on the Qinghai-Tibetan plateau: effects of grazing season, seed characteristics and forage preferences. Plant Soil 465, 367–383. doi: 10.1007/s11104-021-05009-5
Warren, D. L., Glor, R. E., and Turelli, M. (2010). ENMTools: a toolbox for comparative studies of environmental niche models. Ecography 33, 607–611. doi: 10.1111/j.1600-0587.2009.06142.x
Warren, R., Price, J., Graham, E., Forstenhaeusler, N., and VanDerWal, J. (2018). The projected effect on insects, vertebrates, and plants of limiting global warming to 1.5°C rather than 2°C. Science 360, 791–795. doi: 10.1126/science.aar3646
Willett, W., Rockström, J., Loken, B., Springmann, M., Lang, T., Vermeulen, S., et al. (2019). Food in the anthropocene: the EAT–lancet commission on healthy diets from sustainable food systems. Lancet 393, 447–492. doi: 10.1016/S0140-6736(18)31788-4
Xin, F., Liu, J., Chang, C., Wang, Y., and Jia, L. (2021). Evaluating the influence of climate change on Sophora moorcroftiana (Benth.) baker habitat distribution on the tibetan plateau using maximum entropy model. Forests 12, 1230. doi: 10.3390/f12091230
Yesuf, G. U., Brown, K. A., Walford, N. S., Rakotoarisoa, S. E., and Rufino, M. C. (2021). Predicting range shifts for critically endangered plants: Is habitat connectivity irrelevant or necessary? Biol. Conserv. 256, 109033. doi: 10.1016/j.biocon.2021.109033
Zhang, H., Mittal, N., Leamy, L. J., Barazani, O., and Song, B. H. (2017). Back into the wild—apply untapped genetic diversity of wild relatives for crop improvement. Evol. Appl. 10, 5–24. doi: 10.1111/eva.12434
Zhang, K., Yao, L., Meng, J., and Tao, J. (2018). Maxent modeling for predicting the potential geographical distribution of two peony species under climate change. Sci. Total Environ. 634, 1326–1334. doi: 10.1016/j.scitotenv.2018.04.112
Zimmerer, K. S., de Haan, S., Jones, A. D., Creed-Kanashiro, H., Tello, M., Carrasco, M., et al. (2019). The biodiversity of food and agriculture (agrobiodiversity) in the anthropocene: research advances and conceptual framework. Anthropocene 25, 100192. doi: 10.1016/j.ancene.2019.100192
Zuquim, G., Costa, F. R. C., Tuomisto, H., Moulatlet, G. M., and Figueiredo, F. O. G. (2020). The importance of soils in predicting the future of plant habitat suitability in a tropical forest. Plant Soil 450, 151–170. doi: 10.1007/s11104-018-03915-9
Keywords: crop wild relatives, conservation biases, climate change, in situ conservation, biodiversity conservation
Citation: Manda L, Idohou R, Assogbadjo AE and Agbangla C (2022) Climate Change Reveals Contractions and Expansions in the Distribution of Suitable Habitats for the Neglected Crop Wild Relatives of the Genus Vigna (Savi) in Benin. Front. Conserv. Sci. 3:870041. doi: 10.3389/fcosc.2022.870041
Received: 05 February 2022; Accepted: 25 April 2022;
Published: 02 June 2022.
Edited by:
Jacopo Calevo, University of Naples Federico II, ItalyReviewed by:
Gabriele Casazza, University of Genoa, ItalyCopyright © 2022 Manda, Idohou, Assogbadjo and Agbangla. This is an open-access article distributed under the terms of the Creative Commons Attribution License (CC BY). The use, distribution or reproduction in other forums is permitted, provided the original author(s) and the copyright owner(s) are credited and that the original publication in this journal is cited, in accordance with accepted academic practice. No use, distribution or reproduction is permitted which does not comply with these terms.
*Correspondence: Leonard Manda, bG1hbmRhOEBnbWFpbC5jb20=
Disclaimer: All claims expressed in this article are solely those of the authors and do not necessarily represent those of their affiliated organizations, or those of the publisher, the editors and the reviewers. Any product that may be evaluated in this article or claim that may be made by its manufacturer is not guaranteed or endorsed by the publisher.
Research integrity at Frontiers
Learn more about the work of our research integrity team to safeguard the quality of each article we publish.