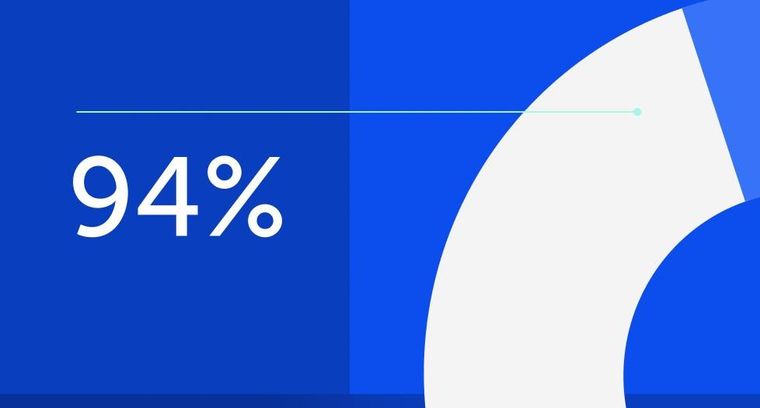
94% of researchers rate our articles as excellent or good
Learn more about the work of our research integrity team to safeguard the quality of each article we publish.
Find out more
ORIGINAL RESEARCH article
Front. Conserv. Sci., 19 November 2021
Sec. Plant Conservation
Volume 2 - 2021 | https://doi.org/10.3389/fcosc.2021.647798
The Antarctic Specially Protected Areas (ASPAs) are zones with restricted access to protect outstanding environmental, scientific, historic, aesthetic, or wilderness values adopted inside the Antarctic Treaty System. Meanwhile, in southern Patagonia, conservation initiatives are implemented by the state of Chile and private entities. However, both are considered unrepresentative. Our work evaluates the representativeness of the in situ conservation through a genetic approach of the moss Sanionia uncinata (Hedw.) Loeske among protected and neighboring free access areas in Maritime Antarctica and southern Patagonia. We discuss observed presence with both current and reconstructed past potential niche distributions (11 and 6 ka BP) in the Fildes Peninsula on King George Island. Results showed occurrence of several spatially genetic subpopulations distributed inside and among ASPA and free access sites. Some free access sites showed a higher amount of polymorphism compared with ASPA, having ancestry in populations developed in those places since 6 ka BP. The different spatial and temporal hierarchies in the analysis suggest that places for conservation of this species in Maritime Antarctica are not well-represented, and that some free access areas should be considered. This work represents a powerful multidisciplinary approach and a great challenge for decision-makers to improve the management plans and the sustainable development in Antarctica.
Early in the 1960s, the subcommittee on conservation of the Scientific Committee on Antarctic Research (SCAR) working group on Biology developed a regime known nowadays as the system of protected areas in Antarctica. Their efforts initially resulted in several recommendations for the establishment of a considerable number of sites, designated mainly for the protection of their scientific value and providing benchmarks and baselines for the use of future scientific activities. These sites evolved from sites of special scientific interest (SSSI) to Antarctic specially protected areas (ASPAs), and Antarctic specially managed areas (ASMAs) (Walton and Dingwall, 1992). The adoption of a dependent ecosystem concept was early formalized in the VIII Antarctic treaty consultative meeting (Antarctic Treaty Consultative Meeting (ATCM), 1976) during the process of refining the environmental protection policies in Antarctica, following concerns regarding the possible environmental impact in areas of the Antarctic treaty and other ecosystems dependent on the Antarctic environment if both mineral exploration and exploitation were to occur there. Recommendation XI-1 notes the unity between the continent of Antarctica and its adjacent offshore areas and stresses again the basic consideration concerning the protection of the unique Antarctic environment and the dependent ecosystems. Thus, the ATCM recommendation VIII-14 considered the importance of the environmental protection of Subantarctic ecosystems, prompted by the southern claimants (Argentina, Australia, Chile, and New Zealand). Accordingly, the special ATCM on Antarctic mineral resources (SATCM IV Wellington, 1988) was later the first to proclaim the basic considerations for the protection of the “Antarctic environment and dependent and associated ecosystem” (Berguño, 2000).
The failed Antarctic mineral resources agreement was followed by the protocol on environmental protection to the Antarctic treaty (ATS). This special focused agreement enabled the implementation of procedures for the management of protected areas in Antarctica, adopting where needed ASPA areas to be designated as areas of restricted access to protect outstanding environmental, scientific, historic, aesthetic, or wilderness values. For instance, these policies were enforced based on the account of the diverse assemblage of bird species that breed on such areas, the necessity to study their ecological relations, and the factors that affect their populations, or because these areas possess outstanding flora, with several species of lichens, mosses, and vascular plants, making these sites valuable for conservation (Antarctic Treaty Consultative Meeting (ATCM), 1991).
In the meantime, the Chilean government created the national public system of protected areas (SNASPE) in 1984 to adopt the frame of the International Union for Conservation Nature (IUCN). That effort was the climax after a series of scattered state initiatives for conservation (Pauchard and Villarroel, 2002). Four categories were established: Virgin Region Reserve, National Park, National Reserve, and National Monument. The aim of the categories goes from no intervention on nature, maintaining its essential ecosystem processes, protected areas that only allow scientific activities and even allow controlled removal of specimens of some species to allow education, research, and recreation only when not compromising conservation. High representativeness in protected areas was found in the coast of southern Patagonia and very low in the steppe vegetation (Pliscoff and Fuentes-Castillo, 2011).
Currently, the number of terrestrial ASPA sites in Antarctica designated for the protection of moss species is the second largest group, after that of sites for the protection of marine bird colonies (Pertierra and Hughes, 2013). This might suggest that, due to the vulnerability or special scientific value, numerous locations of bryophyte carpets might have been considered iconic elements of the Antarctic terrestrial ecosystems under protection, similar to colonies of breeding penguins and petrels. Several recommendations have been placed to unambiguously state the main reason for the designation of an area as ASPA (Hughes et al., 2013). However, the conservation of terrestrial biodiversity has been pointed out to be “inadequate, unrepresentative, and at risk” from a continent-wide perspective (Shaw et al., 2014), similar to what happens in Chile regarding the geographical distribution and ecosystem representation in its protected areas (Pauchard and Villarroel, 2002; Pliscoff and Fuentes-Castillo, 2011). Thus, it requires a holistic reconsideration.
Different studies have recently examined the immediate impact caused from trampling on moss communities, including Saniona uncinata (Hedw.) Loeske species, raising concerns about cumulative effects (Pertierra et al., 2013; Tejedo et al., 2016). ASPAs are often intended to limit the accessibility within ice-free areas to prevent such cumulative impacts. Thus, access restrictions allow only a low pressure from permitted activities and associated disturbances, ultimately leading to better preservation of the contained values, with new sites being steadily designated. Nevertheless, the effectiveness of the implementation of such physical barriers in minimizing the threats posed to the ecosystems remains to be assessed.
Therefore, for conservation purposes, protected areas should be managed according to the existing hierarchical structures within the different taxa. In this context, aside from universities and research institutions, there is growing consideration for the use of genetic studies as methods of conservation work that could provide useful information about the relationship and population dynamics among species inhabiting Antarctica and its neighbor zones (Laikre, 2010). To date, the use of genetic analyses in conservation referred to the report of genetic consequences of small-sized populations and to the inference of aspects of the demographic history of populations (Balding et al., 2008). These studies involved the size, structure, distribution of these populations, and their spatial or temporal changes. It is important for the formulation of conservation measures to establish key factors in extinction risk, such as the level of genetic diversity, historical population bottlenecks, habitat fragmentation, population size, and local population substructure that could be used to make informed decisions on the management of populations (DeSalle and Amato, 2004; Balding et al., 2008). Besides, conservation recommendations based on extinction risks have a great potential for application in Antarctica toward determining strategic ASPA locations and providing clues about whether the species in those remote sites represent new colonization, an inadvertent introduction, or an undetected native remnant. In this sense, although analysis of the potential distribution of species has been mostly applied to establish the projected resilience of species under different scenarios of climate changes, its application could also explain the observed genetic differences in relevant, endemic, or cosmopolitan plant species located among ASPAs and free-access sites in the present. In case differences exist among ASPAs and free access sites, it would be important to explore the means by which free-access areas might influence the survival of species in the region when their genetic diversity resources are not considered as factors in their resilience and are not under conservation. For these reasons, the aims of this study are to (1) analyze the representativeness of the in situ conservation of the species through the genetic diversity and genetic structure of several prominent populations of the cosmopolitan moss Sanionia uncinata (Hedw.) Loeske within South Shetland Islands and southern Patagonia, inside and outside of the current boundaries at different spatial hierarchies (regions, islands, and sites), (2) determine the origin of colonization after fragmentation due to vehicle transit and human trampling between the free access and ASPA sites, (3) assess the importance of the runoffs in forming subpopulations in Maritime Antarctica, and (4) reconstruct the potential distribution of this species in the Fildes peninsula, King George Island, for the past between 11 and 6 ka BP, to provide evidence of the potential spatial distribution of current populations. This multidisciplinary study provides an important tool for making informed decisions about conservation priorities and the management of protected areas in Antarctica and their connected areas based on the genetic study and potential past distribution of species.
In general, in the study sites, the ecosystems are dominated by different species. There are only two species of flowering plants native to Antarctica, Colobanthusquitensis (Kunth) Bartl. and Deschampsiaantarctica Desv., both occurring only in the Peninsula Antarctica sector (Lamb, 1970; Longton, 1985). They are also present in southern Patagonia. Both are frequent at low altitudes and occasional components of cryptogamic communities. The crustaceous lichen subformation is dominated by crustose, dwarf foliose, and other small lichens, which grow adpressed to the substratum. The principal genera are Caloplaca and Xhantoria. Fruticose and foliose lichen are widely and extensively developed from the sea level up to 500 m. The main species is Usneaantarctica. There are also algae subformations, where the dominant species is Prasiolacrispa. Fungi are few in Antarctica from the Myxomycete, Ascomycetes, and Basidiomycetes division. Mosses are most frequently encountered in Antarctica in wet areas of drainage channels, scree, and crevices of rock faces. There are short turf- and small-cushion-forming acrocarpous mosses and tall moss subformations. The dominant genera in the first one are Andreaea, Bryum, Ceratodon, and Pohlia. For the tall moss subformation, the dominant species are Polytrichumalpinum, Chorisodontiumaciphyllum, and Polytrichumalpestre. There is a subformation in wet habitats on gently sloping ground at low altitudes, around lakes or on slopes subjected to melt water seepage, where the dominant genera are Brachythecium and Sanionia (Longton, 1985). It is also possible that some individual species such as Sanioniauncinata developed more compact mats in dryer habitats and let them be dispersed by the wind (Supplementary Figure 1).
Samples of Sanionia uncinata were collected from 13 sites along the South Shetland Islands, Maritime Antarctica, inside both ASPA and surrounding free-access areas. Limits of ASPAs were taken from the Committee on Environmental Protection, Secretariat of the Antarctic Treaty. Other three sites were sampled from protected and non-protected areas in southern Patagonia (Figure 1).
Figure 1. Maps of spatial distribution and location of sampling sites in Maritime Antarctica and Southern Patagonia. Sampling sites depicted by yellow circles; Antarctic Specially Protected Areas (ASPAs) and protected areas in southern Patagonia in red. Map (a) Southern Patagonia and Antarctic Peninsula: A general map of sampled populations from sites at Riesco Island, Parrillar Lagoon, and Karukinka Natural Park in Southern Patagonia and Tierra del Fuego Island and the group of sampling sites located along South Shetland Islands. Map (b) South Shetlands Islands: A detailed location map of sampling sites at Byers Peninsula, Hanna Point, and Hurd Peninsula on Livingston Island; Coppermine Peninsula at Robert Island; and the group of sites located on King George and Nelson Islands. Map (c) Nelson and King George Islands: An inset detail of sampling sites at Elephant Beach, Ardley Peninsula, Suffield Point, Collins, and Potter Peninsula on King George Island; and Pereira Point at Nelson Island.
An average of 18 samples per site of gametophytes in a volume of 20 cm3 (2 × 2 × 5 cm)—each one was collected. The samples were divided into two subsamples and kept in plastic bags. One of them was stored at −20°C to be used in genetic analysis, and the other one was dried to make the herbarium. The samples were processed in the Plant Biotechnology Laboratory at the Magallanes University, Punta Arenas. The collected material of the project T 17-09 funded by the Chilean Antarctic Institute is stored at the Instituto de la Patagonia Herbarium.
The areas free of snow or ice, with vegetation and attractive to birds or seals, are precisely the places where it is most convenient for expeditions to establish their bases as well as for tourists to visit, where even a modest human activity can have a considerable impact. Research stations, therefore, have a disproportionate impact, and, therefore, the reserve of protection areas is essential (Fogg, 1992) (Supplementary Figures 2–6). To determine the origin of colonization after fragmentation due to vehicle transit and human trampling between the free access and ASPA sites, and to assess the importance of the runoffs in forming subpopulations, we analyzed samples aside and over the path from three sites with different levels of fragmentation. The first site analyzed was located on a 50-year-old path in Ardley Peninsula where vegetation removed by vehicle tracks was shown to recolonize the ground, which was not accessible anymore onwards from that time point (Figure 2a). We also analyzed a path on Suffield Point at Fildes Peninsula, which is, nowadays, in use by vehicles moving among the Chilean, Russian, and Uruguayan Stations (Figure 2b1 and Supplementary Figure 7). Finally, to analyze the runoffs of gametophytes to new habitats and attribute the cause of subpopulation formation, we considered a place near the 125-E Fildes Peninsula Suffield Point ASPA-protected area (Figure 2b2 and Supplementary Figure 8).
Figure 2. Maps of spatial distribution and location of sampling sites groups in Fildes Peninsula, Maritime Antarctica. Sampling is depicted by red circles. To determine the origin of colonization after fragmentation due to vehicle transit and human trampling (a) Ardley Peninsula and (b.1) Suffield Point. To assess the importance of the runoffs in forming subpopulations (b.2) Suffield Point.
One sporophyte was washed repeatedly with distilled water before proceeding with DNA extraction, performed using the E.Z.N.A® High Performance (HP) DNA Kit (OMEGA bio-tek®). Amplified fragment length polymorphism (AFLP) fingerprinting was performed according to the original protocol (Vos et al., 1995). The detailed protocol is described in Hebel et al. (2018).
Fifteen selective primer combinations were checked for band polymorphism and reproducibility, resulting in the selection of three primer combinations for selective amplification: EcoRI + AAGG/MseI + ACGG, EcoRI + ACA/MseI + AGC, and EcoRI + ACA/MseI + CAG. The generated multiloci AFLP profiles were coded as present (1), absent (0), or ambiguous (?) to create binary matrices using the MyImageAnalysis Software (Thermo Scientific). Two samples previously run were included in all polyacrylamide gels for comparability. Furthermore, to calculate the error rate, 36 samples were doubly amplified and processed in polyacrylamide gels according to Bonin et al. (2004), considering the ratio between observed and total number of phenotypic differences between replicates.
Genetic analysis considered different hierarchies; for example, within and among regions (southern Patagonia and Antarctica), islands and populations, grouping samples according to the boundaries of ASPAs reported in the plans of the management of the Antarctic Treaty Secretariat website (https://www.ats.aq/devph/es/apa-database, 1 September 2020). For the genetic diversity analysis, different descriptive statistics were calculated, including the number of alleles, allele frequencies, percentage of polymorphic loci at the 5% level, and private and fixed alleles using the AFLP SURV version 1.0 (Vekemans et al., 2002) and FAMD (Schlueter and Harris, 2006). Estimates of genetic diversity (Hj, analogous to He) and statistic differentiation among ASPA sites and open access areas in Antarctica and southern Patagonia were analyzed on fragment frequency, comparing the level of polymorphism. Following the recommendation of Vekemans et al. (2002), we computed the fragment frequency, reserving one option for haploid organisms and fixed homozygosity at each locus due to complete self-fertilization. The significance of the genetic differentiation (genetic structure) among ASPA and open-access populations (fixation index, FST) in the different hierarchies was tested under the hypothesis of no genetic structure, obtaining 10,000 random permutations of individuals among populations using the AFLP-SURV 1.0 software (Vekemans et al., 2002).
Clustering analysis was performed in a population distance matrix, containing the allele frequency data of all defined groups using the Bayesian method with a non-uniform prior among-population form (Cavalli-Sforza and Edwards, 1967), implemented in the FAMD software (Schlueter and Harris, 2006). The statistical support of the generated branches was assessed using a 10,000 bootstrap. The phylogenetic tree was drawn to scale, with branch lengths (next to branches) in the same units as those of the evolutionary distances used to infer the phylogenetic tree. The evolutionary distances were determined using the Jaccard algorithm in the FAMD software, while the evolutionary analyses were conducted using the MEGA X software (Kumar et al., 2018). The analysis of molecular variance test for genetic differentiation of population pairs was estimated with ΦPT (a statistic analogous to FST) for AFLPs. The ΦPT values with a significance level (obtained by 9,999 permutations) were calculated using the GenAlEx 6.5 software (Peakall and Smouse, 2012).
A model-based clustering method implemented in the STRUCTURE version 2.3.4 program (Falush et al., 2003) was used to infer the population structure without consideration of sampling locations or the correlation of allele frequencies within populations. The burn-in set was set to 10,000, while the MCMC was set to 50,000 to accurately estimate the Pr (X/K) in both non-admixture and admixture models. We calculated the ΔK, suggested by Evanno et al. (2005) for the best K, using the Harvester software (Earl and Vonholdt, 2012).
We developed an ancestry analysis, implemented in the STRUCTURE program, to determine the origin of colonization after fragmentation, and to assess the importance of the runoffs in forming subpopulations.
For this analysis, we used the samples coordinates data obtained in years 2010 and 2011 to locate the presence of S. uncinata in the Fildes Peninsula. This area has been reported to exhibit morphological evidence, indicating the occurrence of paraglacial and periglacial processes that began 12 ka before present, with the removal of the ice layer and subsequent occurrence of eustatic and isostatic processes, configuring the current ice-free area of the Fildes Peninsula. The relative sea level was identified to have reached 15 to 18 m above the current level (Wisz et al., 2008; Watcham et al., 2011).
The delimitation of the current ice-free area was based on a QB02 satellite image (06FEB21T133734). A digital elevation model was produced based on digital cartography (INACH-IGM, 2006) as input data to generate the present potential distribution model. QGIS and ArcGIS 10.1® were used to digitize these data in a UTM zone 21 coordinate system. The topographical relief was represented by a digital elevation model (MET Fildes, 40-m grid size), obtained through processing the contour lines (every 10 m) of the digital cartography. For past projection of the potential distribution of S. uncinata, two-time slices at 11 and 6 ka BP were chosen, considering the availability of marine and terrestrial radiocarbon and terrestrial cosmogenic ages data for the area, permitting to infer prevailing conditions of the ice cap extension and height of the mean sea level. Consequently, delineation of past ice extent boundaries and ice thickness was derived from these reconstructions based on geological and geomorphological, terrestrial, and marine evidence (Cofaigh et al., 2014), as well as from the reconstruction of the relative sea level (Watcham et al., 2011).
To predict the current potential distribution of S. uncinata, we used the Maximum Entropy model of species geographic distributions (MaxEnt), running version 3.3.3e of the MaxEnt software (Phillips et al., 2006). As this software uses training and validation data, it was assumed that 75% of the presence data were used as training points (109 samples uniformly distributed in the Peninsula Fildes), whereas 25% as validation points. The logistic output format was used for presence probability analysis. The data for input variables associated with the topography and climate prevailing on the dates of model runs were derived from reconstructed geometry of the land surface and estimated altitudinal variations in temperature.
The spatial distribution of the present temperature was constructed using a value of −2.4°C for the mean annual temperature in Fildes and a temperature lapse altitude gradient of −0.00725°C m−1 (Braun et al., 2004) for each altitude grid cell (hiMET). Consequently, a local temperature layer was generated for each point of the grid by calculating the following equation:
For the parameterization of the execution of the MaxEnt model, an upper limit of 500 iterations was defined at present. To estimate the predictive capacity of the model, the area under the curve (AUC) was analyzed. Analysis for the determination of the relative contribution of each variable was carried out using the Jacknife test (Phillips et al., 2006).
For past dates, abiotic variables were constructed from topography and available temperature data. It was also necessary to reconstruct the ice-free areas for the different time points analyzed, considering the position of the ice sheet, marine transgressions, and isostatic rebound.
In addition, the local values of elevation, slope, and exposure were calculated for the 11 and 6 ka time points. The 10.1® 3d Analyst tool was applied on the MET Fildes 11 ka and MET Fildes 6 ka surfaces, generating raster files (249 rows, 199 columns, 40-m cell size) with the respective values, obtaining a file for each of the abiotic variables for the potential distribution model. The average annual surface temperature value was corrected for the temperature anomaly with respect to the present obtained from the isotopic analysis of the ice core of James Ross Island (Mulvaney et al., 2012), being equivalent to 0.780°C for the 11 ka period and to 0.159°C for the 6 ka period.
The resulting logistic output from the potential distribution model of MaxEnt was reclassified and processed in ArcGIS 10.1 ®. The relationship between the ranges of values of each of the constructed variables and the probability of the presence of S. uncinata was established. These were reclassified into four probability classes: Class 1 = 0–50, Class 2 = 51–60, Class 3 = 61–70, and Class 4 = 71–87. The decision threshold was set to 50% probability, defining that, above this value, the model predicted over chance.
The projected distribution model for the past periods (11 and 6 ka) was based on two assumptions: (i) when using the temperature data obtained from deuterium isotope analysis of the James Ross ice core (Mulvaney et al., 2012), it was assumed that the past climatic behavior in James Ross Island (east of the Antarctic Peninsula) was similar to that in the Fildes Peninsula (western Antarctic Peninsula) (ii) that the topography of the past (MET Fildes 11 ka and MET Fildes 6 ka) in the Fildes Peninsula was similar to the current one, as only its geometry (coast line) and height (elevations) were modified.
Our genetic analysis led to the scoring of 142 alleles. The average proportion of polymorphic alleles that we identified was 18.3%. We calculated the mean scoring error from 36 duplicated samples and found it to be 1.96%. In general, our comparison of ASPA sites and free-access areas in Antarctica revealed that ASPA sites had 17 polymorphic loci, whereas free-access sites had 45, with the total genetic diversity in free access sites being higher (Hj: 0.075) than that in ASPA sites (Hj: 0.049). We further found that protected areas in southern Patagonia had a higher number of polymorphic alleles but similar total genetic diversity compared with free-access areas (Table 1).
Table 1. Results of regional genetic diversity among Antarctic Specially Protected Area (ASPA) and free access populations.
Our resampling statistics based on 10,000 random permutations of individuals among populations revealed a moderate genetic differentiation among free-access and protected areas (FST = 0.0717, ***p < 0.99). P (high) was 0.0025.
We found that ASPA sites in King George Island (KGI) had higher levels of polymorphic loci but a lower level of genetic diversity. More specifically, we observed that the difference in the level of polymorphic loci and genetic diversity was much higher in the free-access area than in the ASPA site in Robert Island. In contrast, Livingston ASPA areas were demonstrated to exhibit higher levels of polymorphism and genetic diversity compared with free-access areas (Table 2).
Table 2. Results of genetic diversity among Antarctic Specially Protected Area (ASPA) and free access areas in island sites.
The genetic diversity within islands was found to be Hw = 0.0623, with the total genetic diversity being Ht = 0.0735. We found that the population genetic structure had a moderate FST: 0.1475; however, this was not significant. The lower 95% limit obtained from the permutation test for the genetic differentiation among populations was 0.0122, whereas the upper 95% limit was 0.1982. Also, the lower 99% level was shown to be 0.0075, whereas the upper 99% limit was 0.2724. P (low) was 0.9234, whereas P (high) was 0.0767.
Our analysis revealed an average proportion of polymorphic alleles of 12.66%. We observed the largest number of polymorphic loci in free-access populations in Coppermine, Robert Island (59.2%). The largest number of polymorphic loci in ASPA sites was found in the Byers Peninsula (Livingston Island) (47.2%).
Furthermore, we noted that some free-access areas in Antarctica, such as Suffield Point (KGI), also showed a higher number of polymorphic loci than ASPA sites (38%) (Table 3). The mean Nei's gene diversity within populations of S. uncinata based on fragment frequency was shown to be Hj: 0.0424. Moreover, we identified the lowest score in ASPA sites, such as the Ardley Peninsula, whereas the highest level was found in the free-access site of the Coppermine Peninsula (0.20). Furthermore, some of the lowest scores were detected in free-access areas in Antarctica, such as Hannah Point and Elephant Beach. Our analysis of the genetic differentiation among populations revealed a moderate-to-high-genetic differentiation with a total gene diversity (Ht) of 0.0559; however, this was not significant (FST = 0.23) (Hartl and Clark, 1997; Nordborg, 2007). Additionally, our AMOVA analysis indicated that 41% of the variability of genetic diversity was detected within populations and 59% among populations (ΦPT = 0.59). The identification of clearly spatially subpopulation is fundamental to establish the boundaries of new ASPA and free-access areas to guarantee gene flow among populations. Analysis of the best K showed higher ΔK for two clusters, including samples from Maritime Antarctica and southern Patagonia regions indistinctly from Cluster 1 or 2. Our analysis showed that, in the case of Coppermine Peninsula, individuals belonging to the two identified clusters were recognized outside of the ASPA limits, which could not ensure the conservation of the complete range of variants existing within these places (Figure 3).
Table 3. Results of genetic diversity within populations in Antarctic Specially Protected Area (ASPA) and free access sites.
Figure 3. Distribution of two S. uncinata clusters found inside and outside of ASPA 112—Coppermine Peninsula, Robert Island. Labels C1 corresponds to Cluster 1 and C2 to Cluster 2. In this figure, the limits of ASPA 112 are shown to reinforce the conservation deficiency, leaving the other areas as free access.
Our generated dendrogram did not show a clear distinction between ASPA and free-access areas. For instance, we identified a long branch in the population of the free-access area in Coppermine. Accordingly, we inferred the evolutionary history using the Neighbor-Joining method. The generated optimal tree with a sum of branch length of 0.380 is shown in Figure 4.
Figure 4. A neighbor-joining tree of relatedness of Sanionia uncinata populations from Antarctica and southern Patagonia.
To assess the importance of the runoffs in forming subpopulations, the ancestry of samples aside and over the path from three sites with different levels of fragmentation was analyzed. Results for the first site analyzed located on a 50-year-old path in Ardley Peninsula historically affected by heavy machinery and vehicles showed a low genetic diversity in the cases of the Ardley Peninsula, indicating that the recolonization in this place was mostly clonal.
In contrast, the evidence on the vehicle disturbance areas near the ASPA 125b-Suffield Point site showed the existence of a mixture of individuals with ancestry in different populations associated with clusters C1 and C2, separated by a few meters and settled in small patches (Figure 5 and Supplementary Table 1). In detail, samples on the east side of ASPA 125 b, the probability to have ancestry in Coppermine populations as a parent, grandparent, great-grandparent is very high (0.89–0.99). In samples at the west side, the probability to have ancestry in Byers population is also high (0.98–0.99). One sample is a mixture with ancestry in Byers and Parrillar and other two samples which have ancestry in more than two populations. If we compare this with Karukinka Natural Park, we identified also the two clusters in few meters, but both areas and clusters are under conservation (Figure 6). Moreover, this Natural Park area is wider than ASPA sites in Antarctica, and there is the place where the only private allele was found.
Figure 5. Current boundaries of ASPA 125b—Holz Stream and 125e—Suffield Point and locations of vulnerable moss sites. C1 corresponds to Cluster 1 and C2 to Cluster 2, respectively.
Figure 6. Distribution of two Sanionia uncinata clusters found inside of Karukinka Natural Park, Southern Patagonia. The lines in red are the limits of the Park. (a,b) show zoom to sampling sites with C1 corresponding to Cluster 1 and C2 to Cluster 2.
The gametophytes that transport to new habitats as the cause of subpopulation formation by runoff show not to be relevant, according to the genetic diversity analysis, geographic subpopulation evidence, and the potential distribution analysis, where the slope has a low contribution into the model.
Our present potential distribution model was able to predict the presence in an acceptable way (AUC 0.738). The projection of the potential past distribution was given for the periods of 11 and 6 ka BP, both of which presented temperature anomalies higher than the present. Panel A (Figure 7) shows the ice-free area at 11 ka BP. This surface totalizes 8.2 km2, and, compared with the current ice-free area, it represents 29.8% of the current ice-free surface. The relative level of the sea was 18 m above the current average sea level, and the higher elevations were lower than the current ones. The current elevation range is 0–147 m. Panel B shows the ice-free area at 6 ka BP. It has an area of 21.5 km2, equivalent to 78% of the current surface. All beaches were located above those observed today on the Fildes Peninsula. The relative level of the sea was 15 m above the current level, and the higher elevations were between 1 and 149 m, all of them were lower than the current ones. Panel C is, currently, an ice-free area; it corresponds to the spatial configuration of the ice-free area that, in 2010, included the Fildes Peninsula. The elevation range of the ice-free area is between 0 and 159 m, and its surface is 28 km2. The probability of presence was shown to range from 1 to 87%. We discovered that only 8.1 km2, corresponding to 29.5% of the current ice-free surface, showed 50% or less probability for the presence of S. uncinata. Additionally, 20% of the surface of the Fildes Peninsula exhibited probability values between 51 and 60%, 6.9% of the surface had between 61 and 70% probability of presence, whereas 22.5% of the surface exhibited the higher probability values, between 71 and 87%.
Figure 7. Probability of presence of S. uncinata in the Fildes Peninsula. (A) 11 ka, (B) 6 ka, and (C) today, as modified from González et al. (2019).
Among the variables used, temperature and elevation were defined to be the most important variables for the distribution prediction. In particular, we found that both variables contributed 74.5% in the prediction of the potential distribution of S. uncinata (temperature, 37.6% and elevation, 36.9%). In contrast, the contribution of exposure (15.2%) and slope (10.3%) was demonstrated to be much lower. The probability of presence was shown to be increased with warmer temperatures. Zones with temperatures below −3°C were <50% likely to have S. uncinata. In particular, we noted that the over 70% probability was obtained with a minimum temperature of −2.47°C. In contrast, the probability of presence was found to be increased with low values of elevation and slope. Above 130 m of elevation and with slopes above 52%, the probability of presence was demonstrated to be below 50%. In contrast, with altitudes below 30 m and slopes of up to 11%, the probability of presence was over 60%. We also observed that a probability of presence could potentially be detected under all exposures; however, the probability was demonstrated to be over 70% between 157 and 360° of exposure.
Although many factors have been considered in conservation efforts, the genetic diversity of populations was not a standard tool when conservation in Antarctica and Patagonia started. Moreover, it is well to know that, as in many cases, a relationship between population size and genetic diversity might not always occur. Often, some highly clonal species with a continuous distribution, such as mosses, might have low-genetic diversity, where genetic drift played a major role in the evolutionary history in comparison with gene flow (Barrett and Kohn, 1991). The slow-growing rate in Antarctic mosses (Selkirk and Skotnicki, 2007), generating highly clonal populations, could have lost genetic variability faster (Barrett and Kohn, 1991) than mosses in other parts of the world, and significant reduction in heterozygosity should occur in population that remain small for several generations. The suggested moderate isolation of populations of Sanionia uncinata and the low diversity of haplotypes found in southern South America to Antarctica compared with the Arctic and subarctic areas could be explained because of the founders, which carried a limited proportion of the available plastid diversity (Hedenas, 2012). Although a comparison is only permitted if the same genetic markers have been used and allelic diversity has been similarly standardized (Holderegger and Segelbacher, 2016), we agree with the idea that the populations in southern Patagonia in the sub-Antarctic area represent a subset of genetic variation (Hedenas, 2012). This is demonstrated by the found two clusters that, because of the intensity of sampling, we found it in Maritime Antarctica. Of course, the amount of samples belonging to Cluster 1 was much higher than Cluster 2. Similarly to what was obtained by Pliscoff and Fuentes-Castillo (2011), where the ecosystem representativeness has identified a gap in the Chilean protection system, we assumed, based on (1) the lower average level of polymorphism and heterozygosis in ASPA sites, (2) the moderate but significant spatial genetic differentiation among ASPA and free-access areas, (3) the presence of subpopulation, and (4) the not-equivalent representativeness of both clusters in ASPA sites, that the boundaries to protect representative genetic diversity of this species are insufficient in Maritime Antarctica. Thus, the consideration of free-access areas appears to be important in conservation issues. As demonstrated by the distribution model, the current probability of presence in the Fildes Peninsula was lower (29.5%) than that for 6 ka (58.9%) and 11 ka (69.9%) from the present, in which both moments of the temperatures were higher in comparison to the current temperatures. So, with higher temperatures predicted for these past periods, a higher probability of sexual reproduction and, consequently, higher genetic diversity was assumed, according to the genetic results obtained for sites developed at 6 ka, such as the Suffield point. We observed that individuals were clustered around a few meters, independent of the slope. Moreover, their adaptation to a constantly changing niche, settling in places where the conditions might be more suitable during different periods of glacial oscillations could not be linked to a particular refuge (Hebel et al., 2018), as in the case of many Mediterranean mosses and temperate tree species (Heuertz et al., 2004; Hebel et al., 2006; Desamore et al., 2012), which resulted in a rapid dispersion and diversification from the southern part of Europe to the north after the Last Glacial Maximum (LGM) 20 ka BP (Watcham et al., 2011; Cofaigh et al., 2014).
The higher polymorphism in the Suffield point (7%), compared with other protected populations, could be explained by different migration events and different ancestries. There are individuals associated with Clusters 1 and 2, separated by a few meters, settled in small patches of subpopulations, spread in areas no larger than 15 m on average, over small hills or valleys with small slopes, where vehicles could not cross. Additionally, Elephant bay showed low-genetic variability in the wide cushion where the species is growing with limited areas with scarce vegetation. If we compared two areas in Fildes Peninsula, such as Suffield population with higher-genetic diversity and Elephant population with lower-genetic polymorphism, we can consider that the soil could have incidence in the way of colonization (sexual or asexual) and, hence, in the genetic diversity. The geology of Elephant bay is mainly basaltic lavas (Smellie et al., 1984) or pavements consisted by fine sediments, gravel or angular stones (López-Martínez et al., 2016), while Suffield point is tuffs and fine lapillistones and agglomerates (Smellie et al., 1984) with glacial till deposits and exposed rock surface (López-Martínez et al., 2016). The substrate differences follow changes for water availability, ground water content, pH, organic layer, and carry to changes in the vegetation coverage, species, which were previously reported for other moss species (Frahm, 2001; Guglielmin et al., 2014). Despite our evidence suggested that this species established in both areas at 6 ka after the LGM, the soil characteristics presumably have incidence in the genetic diversity represented by the way of colonization.
Based on the imbalance of the gene flow caused by the fragmentation or changes in the land use, reduction of the number of alleles, inbreeding depression, loss of genetic diversity, and variability, more genetic drift and less ability to adapt are expected (Briggs and Walters, 2016; Holderegger and Segelbacher, 2016), which depend mainly on the speed of population growth (Nei, 1972), which is normally very slow under the extremely harsh environmental conditions. Apparently, the high-selection pressure in free-access areas around Antarctic scientific stations and other traditional visitor sites is related to the intensive human activity and could work against the natural equilibrium. Although these effects might be imperceptible to humans, and as also mentioned by other authors, many areas have a raising anthropogenic impact (Brooks et al., 2019; Hogg et al., 2020; Leihy et al., 2020). Regarding populations that were historically affected by heavy machinery and vehicles, we found a low-genetic diversity in the cases of the Ardley Peninsula, similar to what was found by Skotnicki et al. (1999a,b), indicating that the recolonization in this place was mostly clonal, which is represented by the 0 proportion of polymorphic loci. In contrast, vehicle disturbance in areas near the ASPA 125b-Suffield Point site, where the path of moving vehicles could easily reach 40 m in width, recolonization had a low probability to occur because of the constant vehicle transit. In the near past, we have been observers of another case such as the refurbishment of the Chinese Station in KGI, which resulted in the damage of a great area covered with S. uncinata patches (https://www.umweltbundesamt.de/sites/default/files/medien/461/publikationen/4424.pdf, 28 September 2021). Another case was the installation of a research facility close to the Chilean Frei Station. This resulted in the damage of ca. 1,000 m2 of moss patches (pers. obs.). Obviously, the change in the land use has an impact on biodiversity, and the threats to genetic diversity were not measured in either of these cases. Similar to reports from Rothera Point about the botanical diversity and representativeness of ASPA sites in comparison with free-access areas (Cannone et al., 2018), our results support the idea that, if we do not protect the biodiversity, and allow human logistic and scientific activities inside free-access areas without management, S. uncinata populations at present and in the future might be facing a genetic constraint in Maritime Antarctica aggravated by human impact. Furthermore, this would lead all efforts in conservation to be restricted to few variants and not to the complete range of alleles existing within these free-access places. Consequently, this would result in local or regional genetic erosion that might reduce resilience at larger spatiotemporal scales, further revealing the fact that all current conservation measures in Antarctica are limited.
At this moment, the idea of analysis of small-scale subpopulations to establish new protected areas might be useful. Such spatial distribution is known to reflect the species distribution according to the evolutionary history of each organism, whereas, for conservation purposes, areas should be managed according to the hierarchy of biogeographic differences (Terauds et al., 2012). Our results suggested that the assessment of scientific values for the definition of ASPA sites is not exhaustive because it does not consider the molecular changes that have already happened or are still happening in these species for the last thousands of years and the loss of alleles because of human activities. Besides, prioritization of sensitive species led to the reduction of the representativeness of other species in spatial conservation (Summers et al., 2012). Based on this, it should be emphasized that there are other relevant aspects to be taken into account to determine the size of conservation areas and buffer zones, including the genetic diversity, population subdivision, population bottleneck, gene flow, and the incidence of anthropogenic effects on conservation in Antarctica. Therefore, a critical evaluation becomes necessary for the management decisions not only of ASPA sites but also of all their spatial dimension effects, including surrounding wide or buffer zones extension. Despite the establishment of ASPA buffer zones to prevent the vulnerability of the Antarctic terrestrial ecosystem, it remains particularly worrisome the fact that many ASPA sites are often designated close to human settlements (with the majority at <25 km from a station) (Hughes et al., 2013).
Therefore, the integration of the current genetic diversity pattern and potential past distribution of these species might serve as a practical guide to suggest the prioritization and expansion (Leihy et al., 2020) of sites for conservation:
Zones close to ASPA 125 sites, such as 125b-Holz Stream and 125e-Suffield Point, evidenced a high level of polymorphism, individuals associated with Clusters 1 and 2 separated by few meters, and individuals with long-distance ancestry present in this area. It is very likely that these populations colonized the area at 6 ka. as inferred from the three high-probability classes solved by the potential distribution model (Figure 8). If we analyzed the superimposed genetic information and the potential distribution in the past at 6 ka, we can see that this area could mean an important niche for the succession of the species. Nowadays, these areas are directly threatened by human trampling and vehicle trafficking. In the case of the ASPA 125e-Suffield Point, the site is protected based on other scientific values, mostly of paleontological interest. However, it is necessary to consider the protection of the existing vegetation in free-access areas alongside the road to Artigas Station, thus protecting not only the biodiversity but also the probably 11 ka developed genetic diversity, currently non-existent, due to environmental changes (see Figure 7).
Figure 8. Locations of vulnerable moss sites and current ASPAs 125b—Holz Stream and 125e—Suffield Point boundaries. Red color dots with labels C1 and C2 correspond to Cluster 1 and Cluster 2 samples, respectively. These sites are superimposed over potential distribution probability areas (red color, 71–87%; green color, 61–70%; cyan color, 51–60%, see Figure 7B) of S. uncinata at 6 ka BP, estimated by model MaxEnt (González et al., 2019). The blue color line is the coastline at 6 ka BP time. Light yellow color lines trace current vehicle paths.
The higher level of polymorphism observed in the Coppermine Peninsula might theoretically indicate a refuge or various migration events from different origins. Most of the variants were demonstrated to be shared between some populations in Maritime Antarctica and southern Patagonia such as in the case of the Riesco Island site near Seno Otway, which has recently suffered major environmental impact from coal mining and livestock farming. In peninsulas containing protected and free-access areas, such as Coppermine Peninsula, the identification of subpopulations is fundamental to the management, the land use decisions, the settings of ASPA limits or the delimitation of the protected area to manage. We recognized Sanionia uncinata individuals belonging to the two identified clusters outside of the ASPA limits. In such cases, efforts in conservation have been restricted to a single subpopulation and not the complete range of variants existing within these places. Because of the different levels of polymorphism inside and outside of ASPA sites and considering the provided identification of subpopulations, an ASPA extension would be necessary to manage conservation efforts without bias.
In very old and large populations, it is often to find alleles that are unique, i.e., those that only occur in this single population, so-called private alleles, such as in the case of Karukinka in southern Patagonia. The recognition of private alleles suggests driving forces for the evolution of the species. It could be considered something imperceptible, but that has been preserved.
“Genetic teleconnections” evidence was reported covering distances >1,000 km if the natural dispersion within Antarctica and among Antarctica and South America is considered, supporting the fact that these two regions are dependent and associated (Biersma et al., 2017; Hebel et al., 2018). Moreover, the effectiveness of the protected area zoning inside the Antarctic Treaty area might be hampered by disturbances on nearby unprotected sites in Patagonia and Antarctica. Thus, taking into account that a population in the genetic sense is not just a group of individuals but a breeding group; and that the local interbreeding units of possibly large, geographically structured populations-local population or demes are evolving units of a species (Hartl and Clark, 1997), the idea of long-term successful conservation based only on the consideration of isolated elements within Antarctica and also that the sole conservation of Antarctica does not make sense. As such, it seems logical to also include localities in southern Patagonia in a broader network of areas to conserve Antarctic biodiversity, but conservation efforts will likely remain dependent on specific localities. One problem associated with this is that every country has its own environmental impact studies when measuring the impact of productive activities, which might not require, for example, the consideration of the importance of gene flow among southern Patagonia and Antarctica or the adaptation of the species to their environment. Even though the southern Chilean Patagonia region has the greatest conserved areas (almost the 50% of the total surface) with private and governmental efforts, it is necessary to think in common management strategies inside and outside Antarctica for the areas of the neighbor. It is until now not implemented and needs its promotion between scientists, governments, and the community. According to the previous idea, the conservation of populations outside Antarctica would grant rates of gene flow and favors the resilience of species in Antarctica that might depend on the importance attached to the protection of dependent and associated environments, even when many of them are being used in productive activities.
Certainly, the vulnerability of ecosystem in Antarctica and southern Patagonia suggests that, for the sustainability of the human activity, these ideas should be considered and reinforced in legislation by decision-makers to support conservation priorities based not only on aspects of species richness for the future but also on multidisciplinary research tools like our work, considering the genetic representativeness of the species.
The original contributions presented in the study are included in the article/Supplementary Materials, further inquiries can be directed to the corresponding author/s.
IH conceived the ideas, designed study, performed research, analyzed data, and led the writing. IG performed the potential distribution model and analysis of data. RJ performed research and analyzed data. All authors discussed the results and jointly worked on the manuscript.
The authors declare that the research was conducted in the absence of any commercial or financial relationships that could be construed as a potential conflict of interest.
All claims expressed in this article are solely those of the authors and do not necessarily represent those of their affiliated organizations, or those of the publisher, the editors and the reviewers. Any product that may be evaluated in this article, or claim that may be made by its manufacturer, is not guaranteed or endorsed by the publisher.
The authors are indebted to the Chilean Antarctic Institute (project T_17-09) and CONICYT-Programa de Investigación Asociativa, PIA (Anillos Antárticos project ACT-105) for funding. We thank the Wildlife Conservation Society, which permitted our sampling and field research at the Karukinka Natural Park. We are grateful to the logistic staff who supported us during the Antarctic summer field scientific expeditions of 2010-12. Finally, we would like to thank the referees for their comments.
The Supplementary Material for this article can be found online at: https://www.frontiersin.org/articles/10.3389/fcosc.2021.647798/full#supplementary-material
Antarctic Treaty Consultative Meeting (ATCM) (1991). Final Report of the Sixteenth Antarctic Treaty Consultative Meeting. Bonn: Antarctic Treaty Secretariat. Available online at: https://documents.ats.aq/ATCM16/fr/ATCM16_fr001_e.pdf
Antarctic Treaty Consultative Meeting (ATCM) (1976). Report of the VIII Consultative Meeting. Ministry of Foreign Affairs. Oslo: Antarctic Treaty Secretariat. Available online at: https://documents.ats.aq/ATCM8/fr/ATCM8_fr001_e.pdf
Balding, D. J., Bishop, M., and Cannings, C. (2008). Handbook of Statistical Genetics. Wiltshire: John Wiley and Sons. doi: 10.1002/9780470061619
Barrett, S. C. H., and Kohn, J. (1991). “The genetic and evolutionary consequences of small population size in plant: implications for conservation,” in Genetics and Conservation of Rare Plants, ed D. Holsinger (Oxford: Oxford University Press).
Berguño, J. (2000). Development and Applications of the Concept of Dependent and Associated Ecosystem Within the Antarctic Treaty System. Concepción: Instituto Antártico Chileno.
Biersma, E. M., Jackson, J. A., Hyvonen, J., Koskinen, S., Linse, K., Griffiths, H., et al. (2017). Global biogeographic patterns in bipolar moss species. R. Soc. Open Sci. 4:13. doi: 10.1098/rsos.170147
Bonin, A., Bellemain, E., Eidesen, P. B., Pompanon, F., Brochmann, C., and Taberlet, P. (2004). How to track and assess genotyping errors in population genetics studies. Mol. Ecol. 13, 3261–3273. doi: 10.1111/j.1365-294X.2004.02346.x
Braun, M., Saurer, H., and Gossmann, H. (2004). Climate, Energy Fluxes and Ablation Rates on the Ice Cap of King George Island: Pesquisa Antártica Brasileira, 4, 87—103.
Briggs, D., and Walters, S. (2016). “Populations: origins and extinctions,” in Plant Variation and Evolution, eds D. Briggs and S. M. Walters (Cambridge: Cambridge University Press). doi: 10.1017/CBO9781139060196.012
Brooks, S. T., Jabour, J., van den Hoff, J., and Bergstrom, D. M. (2019). Our footprint on Antarctica competes with nature for rare ice-free land. Nat. Sustainabil. 2, 185–190. doi: 10.1038/s41893-019-0237-y
Cannone, N., Convey, P., and Malfasi, F. (2018). Antarctic Specially Protected Areas (ASPA): a case study at Rothera Point providing tools and perspectives for the implementation of the ASPA network. Biodivers. Conserv. 27, 2641–2660. doi: 10.1007/s10531-018-1559-1
Cavalli-Sforza, L. L., and Edwards, A. W. (1967). Phylogenetic analysis. Models and estimation procedures. Am. J. Hum. Genet. 19, 233–257.
Cofaigh, C. Ó., Davies, B. J., Livingstone, S. J., Smith, J. A., Johnson, J. S., Hocking, E. P., et al. (2014). Reconstruction of ice-sheet changes in the Antarctic Peninsula since the Last Glacial Maximum. Quat. Sci. Rev. 100, 87–110. doi: 10.1016/j.quascirev.2014.06.023
DeSalle, R., and Amato, G. (2004). The expansion of conservation genetics. Nat. Rev. Genet. 5, 702–712. doi: 10.1038/nrg1425
Desamore, A., Laenen, B., Stech, M., Papp, B., Hedenas, L., Mateo, R. G., et al. (2012). How do temperate bryophytes face the challenge of a changing environment? Lessons from the past and predictions for the future. Global Change Biol. 18, 2915–2924. doi: 10.1111/j.1365-2486.2012.02752.x
Earl, D. A., and Vonholdt, B. M. (2012). Structure Harvester: a website and program for visualizing Structure output and implementing the Evanno method. Conserv. Genet. Resour. 4, 359–361. doi: 10.1007/s12686-011-9548-7
Evanno, G., Regnaut, S., and Goudet, J. (2005). Detecting the number of clusters of individuals using the software Structure: a simulation study. Mol. Ecol. 14, 2611–2620. doi: 10.1111/j.1365-294X.2005.02553.x
Falush, D., Stephens, M., and Pritchard, J. K. (2003). Inference of population structure using multilocus genotype data: Linked loci and correlated allele frequencies. Genetics 164, 1567–1587. doi: 10.1093/genetics/164.4.1567
González, I., Hebel, I., and Jaña, R. (2019). Distribución potencial de Sanionia spp. en dos momentos del Holoceno en península Fildes isla Rey Jorge Antártica. Anales Instituto Patagonia 47, 7–17. doi: 10.4067/S0718-686X2019000200007
Guglielmin, M., Fratte, M. D., and Cannone, N. (2014). Permafrost warming and vegetation changes in continental Antarctica. Environ. Res. Lett. 9:5001. doi: 10.1088/1748-9326/9/4/045001
Hartl, D., and Clark, A. (1997). Principles of Population Genetics Sunderland. Massachusetts: Sinauer Associates, Inc. Publishers.
Hebel, I., Haas, R., and Dounavi, A. (2006). Genetic variation of common ash (Fraxinus excelsior L.) populations from provenance regions in southern Germany by using nuclear and chloroplast microsatellites. Silvae Genet. 55, 38–44. doi: 10.1515/sg-2006-0006
Hebel, I., Rudinger, M. C. D., Jana, R. A., and Bastias, J. (2018). Genetic structure and gene flow of moss sanionia uncinata (Hedw.) Loeske in Maritime Antarctica and Southern-Patagonia. Front. Ecol. Evolut. 6:152. doi: 10.3389/fevo.2018.00152
Hedenas, L. (2012). Global phylogeography in Sanionia uncinata (Amblystegiaceae: Bryophyta). Botan. J. Linnean Soc. 168, 19–42. doi: 10.1111/j.1095-8339.2011.01189.x
Heuertz, M., Fineschi, S., Anzidei, M., Pastorelli, R., Salvini, D., Paule, L., et al. (2004). Chloroplast DNA variation and postglacial recolonization of common ash (Fraxinus excelsior L.) in Europe. Mol. Ecol. 13, 3437–3452. doi: 10.1111/j.1365-294X.2004.02333.x
Hogg, C. J., Lea, M. A., Soler, M. G., Vasquez, V. N., Payo-Payo, A., Parrott, M. L., et al. (2020). Protect the Antarctic Peninsula - before it's too late. Nature 586, 496–499. doi: 10.1038/d41586-020-02939-5
Holderegger, R., and Segelbacher, G. (2016). Naturschutzgenetik. Ein Handbuch für die Praxis: Bern, Haupt.
Hughes, K. A., Pertierra, L. R., and Walton, D. W. H. (2013). Area protection in Antarctica: How can conservation and scientific research goals be managed compatibly? Environ. Sci. Policy 31, 120–132. doi: 10.1016/j.envsci.2013.03.012
Kumar, S., Stecher, G., Li, M., Knyaz, C., and Tamura, K. (2018). MEGA X: molecular evolutionary genetics analysis across computing platforms. Mol. Biol. Evol. 35, 1547–1549. doi: 10.1093/molbev/msy096
Laikre, L. (2010). Genetic diversity is overlooked in international conservation policy implementation. Conser. Genet. 11, 349–354. doi: 10.1007/s10592-009-0037-4
Lamb, I. M. (1970). Antarctic Terrestrial Plants and Their Ecology. Holdgate, MW: Antarctic ecology.
Leihy, R. I., Coetzee, B. W. T., Morgan, F., Raymond, B., Shaw, J. D., Terauds, A., et al. (2020). Antarctica's wilderness fails to capture continent's biodiversity. Nature 583, 567–571. doi: 10.1038/s41586-020-2506-3
Longton, R. E. (1985). CHAPTER 3a – Terrestrial Habitats – Vegetation. Amsterdam: Pergamon. doi: 10.1016/B978-0-08-028881-9.50012-1
López-Martínez, J., Schmid, T., Serrano, E., Mink, S., Nieto, A., and Guillaso, S. (2016). Geomorphology and landforms distribution in selected ice-free areas in the South Shetland Islands, Antarctic Northern Peninsula region. Cuadernos de Investigación Geográfica 42:2965. doi: 10.18172/cig.2965
Mulvaney, R., Abram, N. J., Hindmarsh, R. C. A., Arrowsmith, C., Fleet, L., Triest, J., et al. (2012). Recent Antarctic Peninsula warming relative to Holocene climate and ice-shelf history. Nature 489, 141–204. doi: 10.1038/nature11391
Nordborg, M. (2007). Coalescent Theory. Handbook of Statistical Genetics. Wiltshire: John Wiley and Sons, Ltd. doi: 10.1002/9780470061619.ch25
Pauchard, A., and Villarroel, P. (2002). Protected areas in Chile: History, current status, and challenges. Nat. Areas J. 22, 318–330.
Peakall, R., and Smouse, P. E. (2012). GenAlEx 6.5: genetic analysis in Excel. Population genetic software for teaching and research-an update. Bioinformatics 28, 2537–2539. doi: 10.1093/bioinformatics/bts460
Pertierra, L. R., and Hughes, K. A. (2013). Management of antarctic specially protected areas: permitting, visitation and information exchange practices. Antarctic Sci. 25, 553–564. doi: 10.1017/S0954102012001204
Pertierra, L. R., Lara, F., Tejedo, P., Quesada, A., and Benayas, J. (2013). Rapid denudation processes in cryptogamic communities from Maritime Antarctica subjected to human trampling. Antarctic Sci. 25, 318–328. doi: 10.1017/S095410201200082X
Phillips, S. J., Anderson, R. P., and Schapire, R. E. (2006). Maximum entropy modeling of species geographic distributions. Ecol. Modell. 190, 231–259. doi: 10.1016/j.ecolmodel.2005.03.026
Pliscoff, P., and Fuentes-Castillo, T. (2011). Representativeness of terrestrial ecosystems in Chile's protected area system. Environ. Conserv. 38, 303–311. doi: 10.1017/S0376892911000208
Schlueter, P. M., and Harris, S. A. (2006). Analysis of multilocus fingerprinting data sets containing missing data. Mol. Ecol. Notes 6, 569–572. doi: 10.1111/j.1471-8286.2006.01225.x
Selkirk, P. M., and Skotnicki, M. L. (2007). Measurement of moss growth in continental Antarctica. Polar Biol. 30, 407–413. doi: 10.1007/s00300-006-0197-3
Shaw, J. D., Terauds, A., Riddle, M. J., Possingham, H. P., and Chown, S. L. (2014). Antarctica's protected areas are inadequate, unrepresentative, at risk. PLoS Biol. 12:5. doi: 10.1371/journal.pbio.1001888
Smellie, J. L., Pankhurst, R. J., Thomson, M. R., and Davies, R. E. (1984). The Geology of the South Shetland Island: IV. Stratigraphy, Geochemistry and Evolution. British Antarctic Survey Scientific Reports No.87.
Summers, D. M., Bryan, B. A., Crossman, N. D., and Meyer, W. S. (2012). Species vulnerability to climate change: impacts on spatial conservation priorities and species representation. Glob. Chang. Biol. 18, 2335–2348. doi: 10.1111/j.1365-2486.2012.02700.x
Tejedo, P., Benayas, J., Cajiao, D., Albertos, B., Lara, F., Pertierra, L. R., et al. (2016). Assessing environmental conditions of Antarctic footpaths to support management decisions. J. Environ. Manage. 177, 320–330. doi: 10.1016/j.jenvman.2016.04.032
Terauds, A., Chown, S. L., Morgan, F., Peat, H. J., Watts, D. J., Keys, H., et al. (2012). Conservation biogeography of the Antarctic. Diver. Distrib. 18, 726–741. doi: 10.1111/j.1472-4642.2012.00925.x
Vekemans, X., Beauwens, T., Lemaire, M., and Roldan-Ruiz, I. (2002). Data from amplified fragment length polymorphism (AFLP) markers show indication of size homoplasy and of a relationship between degree of homoplasy and fragment size. Mol. Ecol. 11, 139–151. doi: 10.1046/j.0962-1083.2001.01415.x
Vos, P., Hogers, R., Bleeker, M., Reijans, M., Vandelee, T., Hornes, M., et al. (1995). AFLP - a new technique for DNA-fingerprinting. Nucleic Acids Res. 23, 4407–4414. doi: 10.1093/nar/23.21.4407
Walton, D. W. H., and Dingwall, P. R. (1992). Initiatives and Opportunities for Protected Areas in Antarctic Conservation. Paper presented to the SCAR/IUCN Workshop on Antarctic Protected Areas. Cambridge.
Watcham, E. P., Bentley, M. J., Hodgson, D. A., Roberts, S. J., Fretwell, P. T., Lloyd, J. M., et al. (2011). A new Holocene relative sea level curve for the South Shetland Islands, Antarctica. Quat. Sci. Rev. 30, 3152–3170. doi: 10.1016/j.quascirev.2011.07.021
Keywords: dependent ecosystem, human impact, conservation, Antarctic specially protected area, subpopulation, potential distribution model, population genetics, MaxEnt
Citation: Hebel I, Gonzalez I and Jaña R (2021) Genetic Approach on Sanionia uncinata (Hedw.) Loeske to Evaluate Representativeness of in situ Conservation Areas Among Protected and Neighboring Free Access Areas in Maritime Antarctica and Southern Patagonia. Front. Conserv. Sci. 2:647798. doi: 10.3389/fcosc.2021.647798
Received: 30 December 2020; Accepted: 15 October 2021;
Published: 19 November 2021.
Edited by:
Daniel Sánchez-Mata, Complutense University of Madrid, SpainReviewed by:
Maria Cristina Duarte, University of Lisbon, PortugalCopyright © 2021 Hebel, Gonzalez and Jaña. This is an open-access article distributed under the terms of the Creative Commons Attribution License (CC BY). The use, distribution or reproduction in other forums is permitted, provided the original author(s) and the copyright owner(s) are credited and that the original publication in this journal is cited, in accordance with accepted academic practice. No use, distribution or reproduction is permitted which does not comply with these terms.
*Correspondence: Ingrid Hebel, aW5ncmlkLmhlYmVsQHVtYWcuY2w=
Disclaimer: All claims expressed in this article are solely those of the authors and do not necessarily represent those of their affiliated organizations, or those of the publisher, the editors and the reviewers. Any product that may be evaluated in this article or claim that may be made by its manufacturer is not guaranteed or endorsed by the publisher.
Research integrity at Frontiers
Learn more about the work of our research integrity team to safeguard the quality of each article we publish.