- 1Department of Psychology, Arizona State University, Tempe, AZ, United States
- 2Swammerdam Institute for Life Sciences, University of Amsterdam, Amsterdam, Netherlands
Introduction
Cerebellar microanatomy has inspired many computational models that contribute to both motor and nonmotor function. Key contributors to understanding cerebellar function, including Marr, Albus, and Ito, described error signals within the cerebellum that drive synaptic plasticity and contribute to motor learning (Marr, 1969; Albus, 1971; Ito, 1972). These error signals within the cerebellum are the result of comparing motor output with sensory feedback from the environment. The resulting predictions and corrections for movement are transferred to output regions via bidirectional connections between the cerebellum and cortical regions. The establishment of these pathways in development and plasticity occurs early in life. Gross cerebellar development including the migration, growth, and maturation of neurons continues after birth, up to 3 weeks postnatally in rodents and one year postnatally in humans (Ten Donkelaar and Lammens, 2009). Total cerebellar volume continues to expand until adolescence, peaking earlier for girls (around 12 years of age) than for boys (around 16 years of age), similar to cerebral volume (Tiemeier et al., 2010). However, compared to other brain regions involved in aging such as the hippocampus, the aging cerebellum and its contribution to neurodegenerative diseases has received less attention. Recent evidence has shown that cerebellar connections become dysregulated in aging and may contribute to cognitive decline in Alzheimer's disease (AD), schizophrenia, and Parkinson's disease (Andersen et al., 2012; Douaud et al., 2014; Gellersen et al., 2021). Here, we argue that there are long-term consequences of atypical cerebellar development (Figure 1), and that patterns of cerebellar abnormality may be different between males and females. Furthermore, different patterns of cerebellar abnormality may explain both the diversity and variation of phenotypes in neurodevelopmental disorders and aging dementias. In this review we will largely focus on the prefrontal cortex, due to the significance of this region in cognitive and social behavior. Understanding how cerebellar-cerebrum networks break down in disease pathologies can lead to further understanding of neural networks and improve future therapeutics.
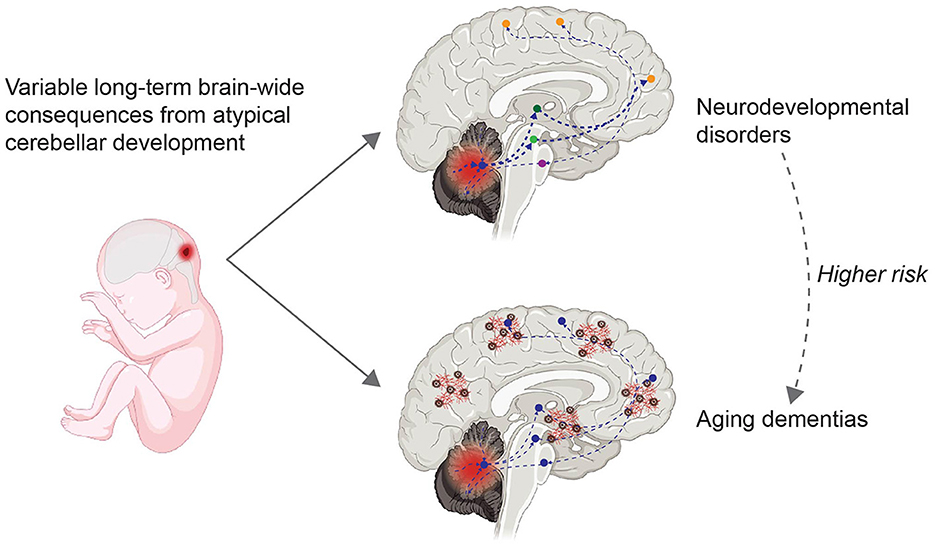
Figure 1. Variable long-term consequences of atypical cerebellar development. Atypical cerebellar development, i.e. due to perinatal injury to the cerebellum (left), can have long-term brain-wide consequences due to bidirectional connections between the cerebellum and neocortical areas (right). Information from the cerebellar cortex flows via the cerebellar nuclei (indicated by a blue circle) and subcortical regions such as the thalamus (dark green) or VTA (light green) to frontal regions (orange), and information from the frontal region flows back to the cerebellum via the pons (purple). Dashed lines indicate altered connections. People diagnosed with neurodevelopmental disorders (top right) have an increased risk of developing aging dementias (bottom right). Red and brown cortical elements in the bottom right indicate amyloid beta plaques and tau tangles, characteristics of aging dementias. Created with BioRender.com.
Brain-wide cerebellar circuits can explain diversity in cerebellum-mediated phenotypes
The cerebellum projects disynaptically to all of neocortex as well as subcortical areas, including regions important for cognition and affective behavior, such as the medial prefrontal cortex (mPFC), anterior cingulate cortex (ACC), parietal cortex, and amygdala (Sveljo et al., 2014; Onuki et al., 2015; Pisano et al., 2021; Zhu et al., 2023). The largest cells of the cerebellar cortex, Purkinje cells, integrate information from ~70 billion neurons in the cerebellar cortex (Lange, 1975). GABAergic Purkinje cells are the only neuron type whose axon leaves the cerebellar cortex to synapse onto cells in the cerebellar nuclei (CN), therefore modulating excitatory CN output to the rest of the brain (Houck and Person, 2014; Judd et al., 2021). In addition, cerebellar output is transferred from the cerebellar cortex to the cerebellar nuclei in unique functional microzones which are organized in a largely lobule-dependent manner (Schweighofer, 1998; Kostadinov et al., 2019; De Zeeuw, 2021). The CN have direct connections with the thalamus, the ventral tegmental area (VTA) and dozens of different nuclei across the brain, and thereby indirect connections with the rest of the brain (Hoshi et al., 2005; Bostan and Strick, 2010; Watabe-Uchida et al., 2012; Hunnicutt et al., 2014; Houck and Person, 2015; Carta et al., 2019; Sathyamurthy et al., 2020; Kebschull et al., 2024; Washburn et al., 2024). In this way, cerebellar output can have widespread influence on the rest of the brain, including cognitive- and socially-associated brain regions.
In humans, Purkinje and granule cells are born and migrate starting at 7 weeks of age (van der Heijden and Sillitoe, 2021), then undergo multiple phases of plasticity and pruning prior to maturation in adulthood. During early development, neurons are sensitive to environmental stimuli as they undergo growth, differentiation, and pruning (Huttenlocher and Dabholkar, 1997). Cerebellar long-range synaptic connectivity, plasticity, and functional activity may be particularly vulnerable in early-life. Hence, there is a need to define causal links between cerebellar plasticity, circuit development, and behavior. Mouse models of social and cognitive deficits exhibit altered expression of signaling molecules and inhibitory circuits resulting in disruption of the excitatory/inhibitory (E/I) balance, reduced cortical connectivity, reduced gray matter, shorter axons, and reduced spine numbers (Schofield et al., 2011; Portera-Cailliau, 2012; Stoya et al., 2014; Shapiro et al., 2017). However, the role of cerebellar-thalamic-cortical projections in later stages of the lifespan has not been characterized and the distinct contributions of cerebellar signaling input on cerebral structure is unknown. Similarly to how the role of the cerebellum in shaping development is likely to be region-specific (Stoodley et al., 2017), different patterns of cerebellar abnormality leading to impaired anatomical and/or physiological connections with the neocortex could explain both the diversity and variation of phenotypes in neurodevelopmental disorders and aging dementias (Badura et al., 2018; Gellersen et al., 2021; Oostland et al., 2022; Bernard et al., 2023). Yet, specific details of the different patterns of cerebellar abnormality within cerebellar-cerebral pathways leading to neurodevelopmental and/or neurodegenerative disorders remain an open question.
Unique cerebellar contributions to behavior between young and adult phenotypes
The cerebellum is among the most-often-reported sites of abnormality in a variety of developmental disorders, including autism spectrum disorder (ASD) and attention deficit hyperactivity disorder (ADHD) (Courchesne, 1997; Courchesne et al., 2001; Carper et al., 2002). Cerebellar injury in newborns, most commonly a stroke, leads to an overall 36-fold risk of an ASD diagnosis by age two (Limperopoulos et al., 2007; Wang et al., 2014). However, adult cerebellar injury does not cause ASD (Limperopoulos et al., 2007). Instead, it has been suggested that the cerebellum interprets and processes sensory stimuli (Baumann et al., 2015), and individuals without normal sensory stimuli in early life are at risk for developing ASD endophenotypes (Chugani et al., 2001; Le Mare and Audet, 2006). One likely target of cerebellar influence is the neocortex, which is highly plastic during development and whose circuitry is perturbed in ASD (Kalia, 2008; LeBlanc and Fagiolini, 2011). Neurons responsible for polysynaptic long-distance communication between the cerebellum and neocortex are highly plastic in early development, including Purkinje cells and cortical interneurons (Hoxha et al., 2016; Rupert and Shea, 2022), suggesting that starting in early life, the cerebellum may influence the development of cognitive and social capacities.
Disruptions in both long-distance and local brain signaling are thought to shape ASD, leading to gross anatomical deficits, including brain size and spine density. Deficits in cell migration, unbalanced excitatory-inhibitory networks, and improper synapse formation and pruning may contribute to these phenotypes (Johnston, 2004; Paus et al., 2008; Takeuchi, 2011; Thomas et al., 2011). During early postnatal development, an increase in synaptic growth, brain glucose metabolism, and gray matter density is followed by a significant pruning event (Andersen, 2003). This sensitive period of development allows for enhanced plasticity and shaping of neural circuits. Excitatory neural output from the cerebellar nuclei on nonmotor neocortical regions may shape dendritic arborization and spine formation to establish and maintain synaptic connections. We propose that the cerebellum shapes synapsing thalamic connections and disruptions during neural development alter growth and maturation of distal forebrain circuits.
The role of the cerebellum in aging is nearly completely unexplored and while the cerebellum does demonstrate aging symptoms, such as thinning of the cerebellar cortex and deposition of amyloid (Aranca et al., 2016; Jacobs et al., 2018; Miguel et al., 2021; Frangou et al., 2022; Toniolo et al., 2023; Arleo et al., 2024), the cerebellum demonstrates aging neuroprotective properties as well. Cerebellar injury to adults results in Cerebellar Cognitive Affective Syndrome and symptoms are distinct from ASD (Schmahmann, 2004, 2013). Indeed the cerebellum has been hypothesized to have neuroprotective properties (Gellersen et al., 2021) that may slow aging, including less age-related DNA methylation (Horvath et al., 2015), mitochondrial DNA deletions (Corral-Debrinski et al., 1992), oxidative damage (Mecocci et al., 1993), and resistance to soluble amyloid-beta (Kim et al., 2003; Yadollahikhales and Rojas, 2023). Unlike the cerebellar cortex, the CN displays more abundant neurodegenerative pathology including tau accumulation (Coakeley et al., 2017; Ikeda et al., 2017; Whitwell et al., 2017; Hammes et al., 2018), iron deposition in Friedrich's ataxia (Akhlaghi et al., 2012; Dogan et al., 2016; Vavla et al., 2018), cell atrophy in frontotemporal dementia (Braak and Braak, 1998; Braak et al., 1999; Rajan et al., 2015; Kneynsberg et al., 2017), Lewy body accumulation (Wu and Hallett, 2013; Bernard et al., 2023; Sivaranjini and Sujatha, 2024), and changes in cell cycle expression (Chen et al., 2010; Beeraka et al., 2022; Ali et al., 2024). It is thought that glia play an important role in aging dementias, but while the cerebellar cortex has more neurons than the rest of the brain, there are lower concentrations of glia. Bergmann glia or Golgi epithelial cells, astrocytes unique to the cerebellum, are situated predominantly in the Purkinje cell layer and are critical for neural migration in early development (Rakic and Sidman, 1973a,b; Xu et al., 2013). Evidence suggests that Bergmann glia support Purkinje cells and modulate structural plasticity, but how these may contribute or protect the cerebellum in aging is unknown (Matsui and Jahr, 2003; Coesmans et al., 2004; Matsui et al., 2005). There are multiple questions of the aging cerebellum to answer and understanding these biological mechanisms may explain contributions or resilience of the cerebellum to aging-related diseases.
Sex differences in cerebellar anatomy and influence on aging
Is the cerebellum sexually differentiated? Along with the neocortex, the cerebellum is larger in males, with additional variations including differences in gray and white matter quantity (Ruigrok et al., 2014). In addition, males show stronger connections between the left cerebellar hemisphere and contralateral cortex (Tiemeier et al., 2010; Wheelock et al., 2019). In ASD, female brains have cortico-cerebellar hyperconnectivity, while males have a hypoconnective pattern (Smith et al., 2019). Neuroendocrine hormones, such as estrogen and progesterone, also regulate cerebellar cortical formation. Within the cerebellum, Purkinje cells express the estrogen receptors (ER) ERα and ERβ, the aromatase gene (Cyp19a), and intranuclear receptors for progesterone throughout life (Ukena et al., 1998; Sakamoto et al., 2001, 2002, 2003; Ikeda and Nagai, 2006; Hoffman et al., 2016; Hedges et al., 2018). Purkinje cell development may be modulated by estradiol through prostaglandin E2 (PGE2) and aromatase activity (Hoffman et al., 2016). During neonatal life, Purkinje cells synthesize progesterone from cholesterol de novo (Furukawa et al., 1998; Ukena et al., 1998, 1999). Progesterone increases Purkinje cell dendritic growth, spinogenesis, and synaptogenesis (Sakamoto et al., 2001, 2002). Some of the progesterone Purkinje cells metabolize may be converted to allopregnanolone, which has been shown to be neuroprotective and even delay neurodegeneration for Purkinje and granule cells (Griffin et al., 2004). These neuroendocrine factors may have unique functions across the lifespan. In development the cerebellum has been implicated in ASD, which is more commonly diagnosed in males than females (3:1) (Loomes et al., 2017). In aging, AD is twice as common in females than in males (Seshadri et al., 1997; Podcasy and Epperson, 2016), and risk of AD increases post-menopause, when estrogen and progesterone levels are diminished. Could neuroendocrine factors play a role in both ASD and AD incidence? As estrogen is thought to be neuroprotective, what changes occur in Purkinje cells in aging and what is their function in the neuroendocrine system in aging?
Long-term consequences of atypical cerebellar development
Atypical cerebellar development may result from genetic mutations, many of which have been linked to ASD (Peter et al., 2016; Brady et al., 2020; Van Overwalle et al., 2020). In development, these genetic mutations result in calcium overload and mitochondrial dysfunction, which may result in abnormal brain growth, synaptic plasticity, and connectivity (Zeidán-Chuliá et al., 2014). These genetic mutations can also have long-term consequences on distally connected brain regions, thereby impacting motor, cognitive, and social development as well as the aging brain. Strikingly, 40% of AD-related genes are altered in the cerebellum of autistic patients (Zeidán-Chuliá et al., 2014). Autistic patients develop higher levels of beta-amyloid and are 2.6x more likely to be diagnosed with AD and other dementias (Vivanti et al., 2021). In ASD patients, neural connectivity deficits, excitatory/inhibitory imbalance, and reductions in cerebral gray matter increase risk for development of AD and other dementias (Bodensteiner and Johnsen, 2005; Limperopoulos et al., 2007, 2010; Messerschmidt et al., 2008; Vivanti et al., 2021), while exact mechanisms in aging are less known. There is a need for additional research following ASD patients throughout their lifespan to understand the underlying biological mechanisms that put these patients at higher risk for dementia to determine possible preventative treatments. Future research should also investigate similar cerebellar biological mechanisms between dementias and other neurodegenerative disorders, such as Parkinson's disease (Wu and Hallett, 2013).
Discussion
In conclusion, the cerebellum has bidirectional connections throughout the brain which may have a large impact on behavior and health across the lifespan. Cerebellar abnormalities can have acute and long-term consequences via modulation of activity in other brain regions, which may be sex dependent. The cerebellar neuroendocrine system is understudied in both development and aging, but there is evidence that Purkinje cells may play a role in sexually differentiating the cerebellar cortex. Understanding these functions could be critical for understanding neurodevelopmental disorders and various aging health challenges, including menopause and dementia. Cerebellar contributions to aging, dementias, and neuropsychiatric disease should receive more attention in clinical settings, for both males and females, and needs to be included in neuroimaging studies, as too often the cerebellum is used only as a reference region (Lacalle-Aurioles et al., 2013; Shigemoto et al., 2018; Young et al., 2021; Leng et al., 2023). There are many unanswered questions. In particular: what biological mechanisms are similar between neural developmental disorders, neurodegeneration, and neuropsychiatric disorders? Do we need novel experimental approaches to answer these questions and are our assays sensitive enough to distinguish sex differences? Lastly, interventions aimed at improving health and disease should include cerebellar function and these cerebellar-cerebral pathways might be a possible treatment avenue with novel targets not yet explored.
Author contributions
JV: Conceptualization, Writing – original draft, Writing – review & editing. MO: Conceptualization, Writing – original draft, Writing – review & editing.
Funding
The author(s) declare financial support was received for the research, authorship, and/or publication of this article. This work was supported by Arizona Alzheimer's Consortium, Institute for Social Science Research, Institute for Mental Health Research, and Arizona State University.
Conflict of interest
The authors declare that the research was conducted in the absence of any commercial or financial relationships that could be construed as a potential conflict of interest.
Publisher's note
All claims expressed in this article are solely those of the authors and do not necessarily represent those of their affiliated organizations, or those of the publisher, the editors and the reviewers. Any product that may be evaluated in this article, or claim that may be made by its manufacturer, is not guaranteed or endorsed by the publisher.
References
Akhlaghi, H., Corben, L., Georgiou-Karistianis, N., Bradshaw, J., Delatycki, M. B., Storey, E., et al. (2012). A functional MRI study of motor dysfunction in Friedreich's ataxia. Brain Res. 1471, 138–154. doi: 10.1016/j.brainres.2012.06.035
Albus, J. S. (1971). A theory of cerebellar function. Math. Biosci. 10, 25–61. doi: 10.1016/0025-5564(71)90051-4
Ali, M., Wani, S. U. D., Dey, T., Sridhar, S. B., and Qadrie, Z. L. (2024). A common molecular and cellular pathway in developing Alzheimer and cancer. Biochem. Biophys. Rep 37:101625. doi: 10.1016/j.bbrep.2023.101625
Andersen, K., Andersen, B. B., and Pakkenberg, B. (2012). Stereological quantification of the cerebellum in patients with Alzheimer's disease. Neurobiol. Aging 33, e11–20. doi: 10.1016/j.neurobiolaging.2010.06.013
Andersen, S. L. (2003). Trajectories of brain development: point of vulnerability or window of opportunity? Neurosci. Biobehav. Rev. 27, 3–18. doi: 10.1016/S0149-7634(03)00005-8
Aranca, T. V., Jones, T. M., Shaw, J. D., Staffetti, J. S., Ashizawa, T., Kuo, S.-H., et al. (2016). Emerging therapies in Friedreich's ataxia. Neurodegener. Dis. Manag. 6, 49–65. doi: 10.2217/nmt.15.73
Arleo, A., Bareš, M., Bernard, J. A., Bogoian, H. R., Bruchhage, M. M. K., Bryant, P., et al. (2024). Consensus paper: cerebellum and ageing. Cerebellum 23, 802–832. doi: 10.1007/s12311-023-01577-7
Badura, A., Verpeut, J. L., Metzger, J. M., Pereira, T. D., Pisano, T. J., Deverett, B., et al. (2018). Normal cognitive and social development require posterior cerebellar activity. Elife 7:e037. doi: 10.7554/eLife.36401.037
Baumann, O., Borra, R. J., Bower, J. M., Cullen, K. E., Habas, C., Ivry, R. B., et al. (2015). Consensus paper: the role of the cerebellum in perceptual processes. Cerebellum 14, 197–220. doi: 10.1007/s12311-014-0627-7
Beeraka, N. M., Nikolenko, V. N., Khaidarovich, Z. F., Valikovna, O. M., Aliagayevna, R. N., Arturovna, Z. L., et al. (2022). Recent investigations on the functional role of cerebellar neural networks in motor functions & nonmotor functions -neurodegeneration. Curr. Neuropharmacol. 20, 1865–1878. doi: 10.2174/1570159X20666220310121441
Bernard, J. A., McOwen, K. M., and Huynh, A. T. (2023). New frontiers for the understanding of aging: the power and possibilities of studying the cerebellum. Curr. Opin. Behav. Sci. 54. doi: 10.1016/j.cobeha.2023.101311
Bodensteiner, J. B., and Johnsen, S. D. (2005). Cerebellar injury in the extremely premature infant: newly recognized but relatively common outcome. J. Child Neurol. 20, 139–142. doi: 10.1177/08830738050200021101
Bostan, A. C., and Strick, P. L. (2010). The cerebellum and basal ganglia are interconnected. Neuropsychol. Rev. 20, 261–270. doi: 10.1007/s11065-010-9143-9
Braak, E., Arai, K., and Braak, H. (1999). Cerebellar involvement in Pick's disease: affliction of mossy fibers, monodendritic brush cells, and dentate projection neurons. Exp. Neurol. 159, 153–163. doi: 10.1006/exnr.1999.7131
Braak, H., and Braak, E. (1998). Involvement of precerebellar nuclei in Pick's disease. Exp. Neurol. 153, 351–365. doi: 10.1006/exnr.1998.6895
Brady, R. O. Jr, Beermann, A., Nye, M., Eack, S. M., Mesholam-Gately, R., Keshavan, M. S., et al. (2020). Cerebellar-cortical connectivity is linked to social cognition trans-diagnostically. Front. Psychiatry 11:573002. doi: 10.3389/fpsyt.2020.573002
Carper, R. A., Moses, P., Tigue, Z. D., and Courchesne, E. (2002). Cerebral lobes in autism: early hyperplasia and abnormal age effects. Neuroimage 16, 1038–1051. doi: 10.1006/nimg.2002.1099
Carta, I., Chen, C. H., Schott, A. L., Dorizan, S., and Khodakhah, K. (2019). Cerebellar modulation of the reward circuitry and social behavior. Science 363:aav0581. doi: 10.1126/science.aav0581
Chen, J., Cohen, M. L., Lerner, A. J., Yang, Y., and Herrup, K. (2010). DNA damage and cell cycle events implicate cerebellar dentate nucleus neurons as targets of Alzheimer's disease. Mol. Neurodegener. 5:60. doi: 10.1186/1750-1326-5-60
Chugani, H. T., Behen, M. E., Muzik, O., Juhász, C., Nagy, F., and Chugani, D. C. (2001). Local brain functional activity following early deprivation: a study of postinstitutionalized Romanian orphans. Neuroimage 14, 1290–1301. doi: 10.1006/nimg.2001.0917
Coakeley, S., Cho, S. S., Koshimori, Y., Rusjan, P., Harris, M., Ghadery, C., et al. (2017). Positron emission tomography imaging of tau pathology in progressive supranuclear palsy. J. Cereb. Blood Flow Metab. 37, 3150–3160. doi: 10.1177/0271678X16683695
Coesmans, M., Weber, J. T., De Zeeuw, C. I., and Hansel, C. (2004). Bidirectional parallel fiber plasticity in the cerebellum under climbing fiber control. Neuron 44, 691–700. doi: 10.1016/j.neuron.2004.10.031
Corral-Debrinski, M., Horton, T., Lott, M. T., Shoffner, J. M., Beal, M. F., and Wallace, D. C. (1992). Mitochondrial DNA deletions in human brain: regional variability and increase with advanced age. Nat. Genet. 2, 324–329. doi: 10.1038/ng1292-324
Courchesne, E. (1997). Brainstem, cerebellar and limbic neuroanatomical abnormalities in autism. Curr. Opin. Neurobiol. 7, 269–278. doi: 10.1016/S0959-4388(97)80016-5
Courchesne, E., Karns, C. M., Davis, H. R., Ziccardi, R., Carper, R. A., Tigue, Z. D., et al. (2001). Unusual brain growth patterns in early life in patients with autistic disorder: an MRI study. Neurology 57, 245–254. doi: 10.1212/WNL.57.2.245
De Zeeuw, C. I. (2021). Bidirectional learning in upbound and downbound microzones of the cerebellum. Nat. Rev. Neurosci. 22, 92–110. doi: 10.1038/s41583-020-00392-x
Dogan, I., Tinnemann, E., Romanzetti, S., Mirzazade, S., Costa, A. S., Werner, C. J., et al. (2016). Cognition in Friedreich's ataxia: a behavioral and multimodal imaging study. Ann Clin Transl Neurol 3, 572–587. doi: 10.1002/acn3.315
Douaud, G., Groves, A. R., Tamnes, C. K., Westlye, L. T., Duff, E. P., Engvig, A., et al. (2014). A common brain network links development, aging, and vulnerability to disease. Proc. Natl. Acad. Sci. U. S. A. 111, 17648–17653. doi: 10.1073/pnas.1410378111
Frangou, S., Modabbernia, A., Williams, S. C. R., Papachristou, E., Doucet, G. E., Agartz, I., et al. (2022). Cortical thickness across the lifespan: data from 17,075 healthy individuals aged 3-90 years. Hum. Brain Mapp. 43, 431–451. doi: 10.1002/hbm.25364
Furukawa, A., Miyatake, A., Ohnishi, T., and Ichikawa, Y. (1998). Steroidogenic acute regulatory protein (StAR) transcripts constitutively expressed in the adult rat central nervous system: colocalization of StAR, cytochrome P-450SCC (CYP XIA1), and 3beta-hydroxysteroid dehydrogenase in the rat brain. J. Neurochem. 71, 2231–2238. doi: 10.1046/j.1471-4159.1998.71062231.x
Gellersen, H. M., Guell, X., and Sami, S. (2021). Differential vulnerability of the cerebellum in healthy ageing and Alzheimer's disease. Neuroimage Clin 30, 102605. doi: 10.1016/j.nicl.2021.102605
Griffin, L. D., Gong, W., Verot, L., and Mellon, S. H. (2004). Niemann–Pick type C disease involves disrupted neurosteroidogenesis and responds to allopregnanolone. Nat. Med. 10, 704–711. doi: 10.1038/nm1073
Hammes, J., Drzezga, A., and van Eimeren, T. (2018). The role of Tau imaging in Parkinsonian disorders. Curr. Neurol. Neurosci. Rep. 18, 86. doi: 10.1007/s11910-018-0898-3
Hedges, V. L., Chen, G., Yu, L., Krentzel, A. A., Starrett, J. R., Zhu, J.-N., et al. (2018). Local estrogen synthesis regulates parallel fiber–Purkinje cell neurotransmission within the cerebellar cortex. Endocrinology 159, 1328–1338. doi: 10.1210/en.2018-00039
Hoffman, J. F., Wright, C. L., and McCarthy, M. M. (2016). A critical period in Purkinje cell development is mediated by local estradiol synthesis, disrupted by inflammation, and has enduring consequences only for males. J. Neurosci. 36, 10039–10049. doi: 10.1523/JNEUROSCI.1262-16.2016
Horvath, S., Mah, V., Lu, A. T., Woo, J. S., Choi, O.-W., Jasinska, A. J., et al. (2015). The cerebellum ages slowly according to the epigenetic clock. Aging 7, 294–306. doi: 10.18632/aging.100742
Hoshi, E., Tremblay, L., Féger, J., Carras, P. L., and Strick, P. L. (2005). The cerebellum communicates with the basal ganglia. Nat. Neurosci. 8, 1491–1493. doi: 10.1038/nn1544
Houck, B. D., and Person, A. L. (2014). Cerebellar loops: a review of the nucleocortical pathway. Cerebellum 13, 378–385. doi: 10.1007/s12311-013-0543-2
Houck, B. D., and Person, A. L. (2015). Cerebellar premotor output neurons collateralize to innervate the cerebellar cortex. J. Comp. Neurol. 523, 2254–2271. doi: 10.1002/cne.23787
Hoxha, E., Tempia, F., Lippiello, P., and Miniaci, M. C. (2016). Modulation, plasticity and pathophysiology of the parallel fiber-Purkinje cell synapse. Front. Synaptic Neurosci. 8:35. doi: 10.3389/fnsyn.2016.00035
Hunnicutt, B. J., Long, B. R., Kusefoglu, D., Gertz, K. J., Zhong, H., and Mao, T. (2014). A comprehensive thalamocortical projection map at the mesoscopic level. Nat. Neurosci. 17, 1276–1285. doi: 10.1038/nn.3780
Huttenlocher, P. R., and Dabholkar, A. S. (1997). Regional differences in synaptogenesis in human cerebral cortex. J. Comp. Neurol. 387, 167–178.
Ikeda, C., Yokota, O., Miki, T., Takenoshita, S., Ishizu, H., Mori, Y., et al. (2017). Pick's disease with neuronal four-repeat tau accumulation in the basal ganglia, brain stem nuclei and cerebellum. Neuropathology 37, 544–559. doi: 10.1111/neup.12394
Ikeda, Y., and Nagai, A. (2006). Differential expression of the estrogen receptors alpha and beta during postnatal development of the rat cerebellum. Brain Res. 1083, 39–49. doi: 10.1016/j.brainres.2006.02.025
Ito, M. (1972). Neural design of the cerebellar motor control system. Brain Res. 40, 81–84. doi: 10.1016/0006-8993(72)90110-2
Jacobs, H. I. L., Hopkins, D. A., Mayrhofer, H. C., Bruner, E., van Leeuwen, F. W., Raaijmakers, W., et al. (2018). The cerebellum in Alzheimer's disease: evaluating its role in cognitive decline. Brain 141, 37–47. doi: 10.1093/brain/awx194
Johnston, M. V. (2004). Clinical disorders of brain plasticity. Brain Dev. 26, 73–80. doi: 10.1016/S0387-7604(03)00102-5
Judd, E. N., Lewis, S. M., and Person, A. L. (2021). Diverse inhibitory projections from the cerebellar interposed nucleus. Elife 10:e66231. doi: 10.7554/eLife.66231.sa2
Kalia, M. (2008). Brain development: anatomy, connectivity, adaptive plasticity, and toxicity. Metab. Clin. Exp. 57, S2–5. doi: 10.1016/j.metabol.2008.07.009
Kebschull, J. M., Casoni, F., Consalez, G. G., Goldowitz, D., Hawkes, R., Ruigrok, T. J. H., et al. (2024). Cerebellum lecture: the cerebellar nuclei-core of the cerebellum. Cerebellum 23, 620–677. doi: 10.1007/s12311-022-01506-0
Kim, H.-J., Chae, S.-C., Lee, D.-K., Chromy, B., Lee, S. C., Park, Y.-C., et al. (2003). Selective neuronal degeneration induced by soluble oligomeric amyloid beta protein. FASEB J. 17, 118–120. doi: 10.1096/fj.01-0987fje
Kneynsberg, A., Combs, B., Christensen, K., Morfini, G., and Kanaan, N. M. (2017). Axonal degeneration in tauopathies: disease relevance and underlying mechanisms. Front. Neurosci. 11:572. doi: 10.3389/fnins.2017.00572
Kostadinov, D., Beau, M., Blanco-Pozo, M., and Häusser, M. (2019). Predictive and reactive reward signals conveyed by climbing fiber inputs to cerebellar Purkinje cells. Nat. Neurosci. 22, 950–962. doi: 10.1038/s41593-019-0381-8
Lacalle-Aurioles, M., Alemán-Gómez, Y., Guzmán-De-Villoria, J. A., Cruz-Orduña, I., Olazarán, J., Mateos-Pérez, J. M., et al. (2013). Is the cerebellum the optimal reference region for intensity normalization of perfusion MR studies in early Alzheimer's disease? PLoS ONE 8:e81548. doi: 10.1371/journal.pone.0081548
Lange, W. (1975). Cell number and cell density in the cerebellar cortex of man and some other mammals. Cell Tissue Res. 157, 115–124. doi: 10.1007/BF00223234
Le Mare, L., and Audet, K. (2006). A longitudinal study of the physical growth and health of postinstitutionalized Romanian adoptees. Paediatr. Child Health 11, 85−91.
LeBlanc, J. J., and Fagiolini, M. (2011). Autism: a “critical period” disorder? Neural Plast. 2011:921680. doi: 10.1155/2011/921680
Leng, F., Hinz, R., Gentleman, S., Hampshire, A., Dani, M., Brooks, D. J., et al. (2023). Neuroinflammation is independently associated with brain network dysfunction in Alzheimer's disease. Mol. Psychiatry 28, 1303–1311. doi: 10.1038/s41380-022-01878-z
Limperopoulos, C., Bassan, H., Gauvreau, K., Robertson, R. L. Jr, Sullivan, N. R., Benson, C. B., et al. (2007). Does cerebellar injury in premature infants contribute to the high prevalence of long-term cognitive, learning, and behavioral disability in survivors? Pediatrics 120, 584–593. doi: 10.1542/peds.2007-1041
Limperopoulos, C., Chilingaryan, G., Guizard, N., Robertson, R. L., and Du Plessis, A. J. (2010). Cerebellar injury in the premature infant is associated with impaired growth of specific cerebral regions. Pediatr. Res. 68, 145–150. doi: 10.1203/PDR.0b013e3181e1d032
Loomes, R., Hull, L., and Mandy, W. P. L. (2017). What is the male-to-female ratio in autism spectrum disorder? A systematic review and meta-analysis. J. Am. Acad. Child Adolesc. Psychiatry 56, 466–474. doi: 10.1016/j.jaac.2017.03.013
Marr, D. (1969). A theory of cerebellar cortex. J. Physiol. 202, 437–470. doi: 10.1113/jphysiol.1969.sp008820
Matsui, K., and Jahr, C. E. (2003). Ectopic release of synaptic vesicles. Neuron 40, 1173–1183. doi: 10.1016/S0896-6273(03)00788-8
Matsui, K., Jahr, C. E., and Rubio, M. E. (2005). High-concentration rapid transients of glutamate mediate neural-glial communication via ectopic release. J. Neurosci. 25, 7538–7547. doi: 10.1523/JNEUROSCI.1927-05.2005
Mecocci, P., MacGarvey, U., Kaufman, A. E., Koontz, D., Shoffner, J. M., Wallace, D. C., et al. (1993). Oxidative damage to mitochondrial DNA shows marked age-dependent increases in human brain. Ann. Neurol. 34, 609–616. doi: 10.1002/ana.410340416
Messerschmidt, A., Fuiko, R., Prayer, D., Brugger, P. C., Boltshauser, E., Zoder, G., et al. (2008). Disrupted cerebellar development in preterm infants is associated with impaired neurodevelopmental outcome. Eur. J. Pediatr. 167, 1141–1147. doi: 10.1007/s00431-007-0647-0
Miguel, J. C., Perez, S. E., Malek-Ahmadi, M., and Mufson, E. J. (2021). Cerebellar calcium-binding protein and neurotrophin receptor defects in down syndrome and Alzheimer's disease. Front. Aging Neurosci. 13:645334. doi: 10.3389/fnagi.2021.645334
Onuki, Y., Van Someren, E. J. W., De Zeeuw, C. I., and Van der Werf, Y. D. (2015). Hippocampal-cerebellar interaction during spatio-temporal prediction. Cereb. Cortex 25, 313–321. doi: 10.1093/cercor/bht221
Oostland, M., Kislin, M., Chen, Y., Chen, T., Venditto, S. J., Deverett, B., et al. (2022). Cerebellar acceleration of learning in an evidence-accumulation task. bioRxiv. doi: 10.1101/2021.12.23.474034
Paus, T., Keshavan, M., and Giedd, J. N. (2008). Why do many psychiatric disorders emerge during adolescence? Nat. Rev. Neurosci. 9, 947–957. doi: 10.1038/nrn2513
Peter, S., Ten Brinke, M. M., Stedehouder, J., Reinelt, C. M., Wu, B., Zhou, H., et al. (2016). Dysfunctional cerebellar Purkinje cells contribute to autism-like behaviour in Shank2-deficient mice. Nat. Commun. 7:12627. doi: 10.1038/ncomms12627
Pisano, T. J., Dhanerawala, Z. M., Kislin, M., Bakshinskaya, D., Engel, E. A., Hansen, E. J., et al. (2021). Homologous organization of cerebellar pathways to sensory, motor, and associative forebrain. Cell Rep. 36:109721. doi: 10.1016/j.celrep.2021.109721
Podcasy, J. L., and Epperson, C. N. (2016). Considering sex and gender in Alzheimer disease and other dementias. Dialogues Clin. Neurosci. 18, 437–446. doi: 10.31887/DCNS.2016.18.4/cepperson
Portera-Cailliau, C. (2012). Which comes first in fragile X syndrome, dendritic spine dysgenesis or defects in circuit plasticity? Neuroscientist 18, 28–44. doi: 10.1177/1073858410395322
Rajan, K. B., Wilson, R. S., Weuve, J., Barnes, L. L., and Evans, D. A. (2015). Cognitive impairment 18 years before clinical diagnosis of Alzheimer disease dementia. Neurology 85, 898–904. doi: 10.1212/WNL.0000000000001774
Rakic, P., and Sidman, R. L. (1973a). Organization of cerebellar cortex secondary to deficit of granule cells in weaver mutant mice. J. Comp. Neurol. 152, 133–161. doi: 10.1002/cne.901520203
Rakic, P., and Sidman, R. L. (1973b). Weaver mutant mouse cerebellum: defective neuronal migration secondary to abnormality of Bergmann glia. Proc. Natl. Acad. Sci. U. S. A. 70, 240–244. doi: 10.1073/pnas.70.1.240
Ruigrok, A. N. V., Salimi-Khorshidi, G., Lai, M.-C., Baron-Cohen, S., Lombardo, M. V., Tait, R. J., et al. (2014). A meta-analysis of sex differences in human brain structure. Neurosci. Biobehav. Rev. 39, 34–50. doi: 10.1016/j.neubiorev.2013.12.004
Rupert, D. D., and Shea, S. D. (2022). Parvalbumin-positive interneurons regulate cortical sensory plasticity in adulthood and development through shared mechanisms. Front. Neural Circuits 16:886629. doi: 10.3389/fncir.2022.886629
Sakamoto, H., Shikimi, H., Ukena, K., and Tsutsui, K. (2003). Neonatal expression of progesterone receptor isoforms in the cerebellar Purkinje cell in rats. Neurosci. Lett. 343:163–166. doi: 10.1016/S0304-3940(03)00362-8
Sakamoto, H., Ukena, K., and Tsutsui, K. (2001). Effects of progesterone synthesized de novo in the developing Purkinje cell on its dendritic growth and synaptogenesis. J. Neurosci. 21, 6221–6232. doi: 10.1523/JNEUROSCI.21-16-06221.2001
Sakamoto, H., Ukena, K., and Tsutsui, K. (2002). Dendritic spine formation in response to progesterone synthesized de novo in the developing Purkinje cell in rats. Neurosci. Lett. 322, 111–115. doi: 10.1016/S0304-3940(02)00077-0
Sathyamurthy, A., Barik, A., Dobrott, C. I., Matson, K. J. E., Stoica, S., Pursley, R., et al. (2020). Cerebellospinal neurons regulate motor performance and motor learning. Cell Rep. 31:107595. doi: 10.1016/j.celrep.2020.107595
Schmahmann, J. D. (2004). Disorders of the cerebellum: ataxia, dysmetria of thought, and the cerebellar cognitive affective syndrome. J. Neuropsychiatry Clin. Neurosci. 16, 367–378. doi: 10.1176/jnp.16.3.367
Schmahmann, J. D. (2013). “Cerebellar cognitive affective syndrome and the neuropsychiatry of the cerebellum,” in Handbook of the Cerebellum and Cerebellar Disorders (Cham: Springer International Publishing), 1717–1751.
Schofield, C. M., Hsu, R., Barker, A. J., Gertz, C. C., Blelloch, R., and Ullian, E. M. (2011). Monoallelic deletion of the microRNA biogenesis gene Dgcr8 produces deficits in the development of excitatory synaptic transmission in the prefrontal cortex. Neural Dev. 6:11. doi: 10.1186/1749-8104-6-11
Schweighofer, N. (1998). A model of activity-dependent formation of cerebellar microzones. Biol. Cybern. 79, 97–107. doi: 10.1007/s004220050462
Seshadri, S., Wolf, P. A., Beiser, A., Au, R., McNulty, K., White, R., et al. (1997). Lifetime risk of dementia and Alzheimer's disease. the impact of mortality on risk estimates in the Framingham study. Neurology 49, 1498–1504. doi: 10.1212/WNL.49.6.1498
Shapiro, L. P., Parsons, R. G., Koleske, A. J., and Gourley, S. L. (2017). Differential expression of cytoskeletal regulatory factors in the adolescent prefrontal cortex: implications for cortical development. J. Neurosci. Res. 95, 1123–1143. doi: 10.1002/jnr.23960
Shigemoto, Y., Sone, D., Imabayashi, E., Maikusa, N., Okamura, N., Furumoto, S., et al. (2018). Dissociation of tau deposits and brain atrophy in early Alzheimer's disease: a combined positron emission tomography/magnetic resonance imaging study. Front. Aging Neurosci. 10:223. doi: 10.3389/fnagi.2018.00223
Sivaranjini, S., and Sujatha, C. M. (2024). Analysis of cognitive dysfunction in Parkinson's disease using voxel based morphometry and radiomics. Cogn. Process. 25, 521–532. doi: 10.1007/s10339-024-01197-x
Smith, R. E. W., Avery, J. A., Wallace, G. L., Kenworthy, L., Gotts, S. J., and Martin, A. (2019). Sex differences in resting-state functional connectivity of the cerebellum in autism spectrum disorder. Front. Hum. Neurosci. 13:104. doi: 10.3389/fnhum.2019.00104
Stoodley, C. J., D'Mello, A. M., Ellegood, J., Jakkamsetti, V., Liu, P., Nebel, M. B., et al. (2017). Altered cerebellar connectivity in autism and cerebellar-mediated rescue of autism-related behaviors in mice. Nat. Neurosci. 20, 1744–1751. doi: 10.1038/s41593-017-0004-1
Stoya, G., Redies, C., and Schmid-Hertel, N. (2014). Inversion of layer-specific cadherin expression profiles and maintenance of cytoarchitectonic areas in the allocortex of the reeler mutant mouse. J. Comp. Neurol. 522, 3106–3119. doi: 10.1002/cne.23572
Sveljo, O., Culić, M., Koprivšek, K., and Lučić, M. (2014). The functional neuroimaging evidence of cerebellar involvement in the simple cognitive task. Brain Imaging Behav. 8, 480–486. doi: 10.1007/s11682-014-9290-3
Takeuchi, Y. (2011). “Serotonergic neurotransmission in autism spectrum disorders,” in Autism – A Neurodevelopmental Journey From Genes to Behaviour (InTech). doi: 10.5772/17509
Ten Donkelaar, H. J., and Lammens, M. (2009). Development of the human cerebellum and its disorders. Clin. Perinatol. 36, 513–530. doi: 10.1016/j.clp.2009.06.001
Thomas, M. S. C., Knowland, V. C. P., and Karmiloff-Smith, A. (2011). Mechanisms of developmental regression in autism and the broader phenotype: a neural network modeling approach. Psychol. Rev. 118, 637–654. doi: 10.1037/a0025234
Tiemeier, H., Lenroot, R. K., Greenstein, D. K., Tran, L., Pierson, R., and Giedd, J. N. (2010). Cerebellum development during childhood and adolescence: a longitudinal morphometric MRI study. Neuroimage 49, 63–70. doi: 10.1016/j.neuroimage.2009.08.016
Toniolo, S., Pisotta, I., and Manto, M. (2023). Editorial: The role of the cerebellum in dementia and neurodegenerative diseases. Front. Neurosci. 17:1229624. doi: 10.3389/fnins.2023.1229624
Ukena, K., Kohchi, C., and Tsutsui, K. (1999). Expression and activity of 3β-hydroxysteroid dehydrogenase/Δ5-Δ4-isomerase in the rat Purkinje neuron during neonatal life. Endocrinology 140, 805–813. doi: 10.1210/endo.140.2.6516
Ukena, K., Usui, M., Kohchi, C., and Tsutsui, K. (1998). Cytochrome P450 side-chain cleavage enzyme in the cerebellar Purkinje neuron and its neonatal change in rats. Endocrinology 139, 137–147. doi: 10.1210/endo.139.1.5672
van der Heijden, M. E., and Sillitoe, R. V. (2021). Interactions between Purkinje cells and granule cells coordinate the development of functional cerebellar circuits. Neuroscience 462, 4–21. doi: 10.1016/j.neuroscience.2020.06.010
Van Overwalle, F., Manto, M., Cattaneo, Z., Clausi, S., Ferrari, C., Gabrieli, J. D. E., et al. (2020). Consensus paper: cerebellum and social cognition. Cerebellum 19, 833–868. doi: 10.1007/s12311-020-01155-1
Vavla, M., Arrigoni, F., Nordio, A., De Luca, A., Pizzighello, S., Petacchi, E., et al. (2018). Functional and structural brain damage in Friedreich's ataxia. Front. Neurol. 9:747. doi: 10.3389/fneur.2018.00747
Vivanti, G., Tao, S., Lyall, K., Robins, D. L., and Shea, L. L. (2021). The prevalence and incidence of early-onset dementia among adults with autism spectrum disorder. Autism Res. 14, 2189–2199. doi: 10.1002/aur.2590
Wang, S. S.-H., Kloth, A. D., and Badura, A. (2014). The cerebellum, sensitive periods, and autism. Neuron 83, 518–532. doi: 10.1016/j.neuron.2014.07.016
Washburn, S., Oñate, M., Yoshida, J., Vera, J., Bhuvanasundaram, R., Khatami, L., et al. (2024). The cerebellum directly modulates the substantia nigra dopaminergic activity. Nat. Neurosci. 27, 497–513. doi: 10.1038/s41593-023-01560-9
Watabe-Uchida, M., Zhu, L., Ogawa, S. K., Vamanrao, A., and Uchida, N. (2012). Whole-brain mapping of direct inputs to midbrain dopamine neurons. Neuron 74, 858–873. doi: 10.1016/j.neuron.2012.03.017
Wheelock, M. D., Hect, J. L., Hernandez-Andrade, E., Hassan, S. S., Romero, R., Eggebrecht, A. T., et al. (2019). Sex differences in functional connectivity during fetal brain development. Dev. Cogn. Neurosci. 36:100632. doi: 10.1016/j.dcn.2019.100632
Whitwell, J. L., Lowe, V. J., Tosakulwong, N., Weigand, S. D., Senjem, M. L., Schwarz, C. G., et al. (2017). [18 F]AV-1451 tau positron emission tomography in progressive supranuclear palsy. Mov. Disord. 32, 124–133. doi: 10.1002/mds.26834
Wu, T., and Hallett, M. (2013). The cerebellum in Parkinson's disease. Brain 136, 696–709. doi: 10.1093/brain/aws360
Xu, H., Yang, Y., Tang, X., Zhao, M., Liang, F., Xu, P., et al. (2013). Bergmann glia function in granule cell migration during cerebellum development. Mol. Neurobiol. 47, 833–844. doi: 10.1007/s12035-013-8405-y
Yadollahikhales, G., and Rojas, J. C. (2023). Anti-amyloid immunotherapies for Alzheimer's disease: a 2023 clinical update. Neurotherapeutics 20, 914–931. doi: 10.1007/s13311-023-01405-0
Young, C. B., Landau, S. M., Harrison, T. M., Poston, K. L., Mormino, E. C., and ADNI (2021). Influence of common reference regions on regional tau patterns in cross-sectional and longitudinal [18F]-AV-1451 PET data. Neuroimage 243:118553. doi: 10.1016/j.neuroimage.2021.118553
Zeidán-Chuliá, F., de Oliveira, B.-H. N., Salmina, A. B., Casanova, M. F., Gelain, D. P., Noda, M., et al. (2014). Altered expression of Alzheimer's disease-related genes in the cerebellum of autistic patients: a model for disrupted brain connectome and therapy. Cell Death Dis. 5:e1250. doi: 10.1038/cddis.2014.227
Keywords: cerebellum, Alzheimer's disease, neurodevelopment, aging, Purkinje cell, neural circuits, sex differences
Citation: Verpeut JL and Oostland M (2024) The significance of cerebellar contributions in early-life through aging. Front. Comput. Neurosci. 18:1449364. doi: 10.3389/fncom.2024.1449364
Received: 14 June 2024; Accepted: 12 August 2024;
Published: 27 August 2024.
Edited by:
Arpan Banerjee, National Brain Research Centre (NBRC), IndiaReviewed by:
Hirofumi Fujita, University of Texas Southwestern Medical Center, United StatesCopyright © 2024 Verpeut and Oostland. This is an open-access article distributed under the terms of the Creative Commons Attribution License (CC BY). The use, distribution or reproduction in other forums is permitted, provided the original author(s) and the copyright owner(s) are credited and that the original publication in this journal is cited, in accordance with accepted academic practice. No use, distribution or reproduction is permitted which does not comply with these terms.
*Correspondence: Jessica L. Verpeut, jverpeut@asu.edu