- 1Department of Psychology, Univ Paul Valéry Montpellier 3, EPSYLON EA 4556, F34000, Montpellier, France
- 2Mind, Brain and Behavior Research Center (CIMCYC) University of Granada, Granada, Spain
- 3Department of Experimental Psychology, University of Granada, Granada, Spain
Introduction: Recent evidence suggests that the temporal expectations afforded by a regular rhythmic structure operate independently from endogenous spatial attention in simple reaction-time tasks. The most common manipulation followed in previous studies consisted of presenting a target stimulus either “in-time” or “out-of-time” (earlier or later) with a preceding rhythm. However, contrary to the proposal of entrainment models predicting a behavioral advantage for in-time compared to both early and late targets, responses were still faster for late targets, according to the so-called “foreperiod effect”. This finding makes it difficult to fully disentangle the impact of rhythm and the benefits afforded by the passage of time on the relationship between rhythm-based temporal and endogenous spatial attention.
Methods: To shed more light on this issue, we combined a spatial orienting task with a rhythmic manipulation, in which two placeholders flanking the fixation cross flickered at either a regular or irregular pace. Spatial orienting to the target location was deployed by symbolic color cues that were displayed independently of the rhythm (independent cues) or were integrated with the temporal rhythmic cues (integrated cues). Crucially, for both independent and integrated cues, and for regular and irregular rhythms, the interval between the rhythm and the target (i.e., the foreperiod) was kept fixed in Experiments 1–3 to control for the foreperiod effect, while the effect of foreperiod was explored in Experiment 4.
Results: Results showed a more beneficial effect of rhythms with independent cues as compared to integrated cues. Additionally, the benefit of rhythms was slightly but significantly larger at valid, compared to invalid, spatial locations, regardless of the foreperiod.
Discussion: Our results extend previous studies by showing that interactive effects of rhythms and endogenous spatial attention may emerge in low-demanding detection tasks.
1. Introduction
Selective attention is a crucial cognitive process to guarantee appropriate interactions with the surroundings. Adaptive behavior in rich and dynamic environments relies on efficient prioritization of some events amongst many others, which might be considered distractors. Selective attention operates by means of orienting mechanisms that unfold both in space and time. Directing attention to a specific region of space can enhance sensory processing at that location, producing faster response times (RTs) and more accurate responses (see Chica et al., 2014, for a review). In rapidly changing situations or dynamic contexts such as driving, temporal orienting enables us to focus on specific moments in time so as to enhance sensory processing during a brief period (see Capizzi and Correa, 2018; Nobre and van Ede, 2018, for reviews). Orienting in time can be driven endogenously, by symbolic cues (e.g., Kingstone, 1992; Coull and Nobre, 1998; Capizzi et al., 2013; Weinbach et al., 2015; Coull et al., 2016), or exogenously, by rhythmic sequences (e.g., Jones et al., 2002; Sanabria et al., 2011; De la Rosa et al., 2012; Rohenkohl et al., 2012; Breska and Ivry, 2018).
According to the Dynamic Attending Theory (DAT; Large and Jones, 1999), rhythmic information may automatically entrain periodic oscillations in the sensory systems leading to optimal moments in time, in contrast with other less appropriate moments. There is consistent evidence showing that sensory processing of an event appearing at the “right” moment is enhanced: responses are faster and more accurate (Sanabria et al., 2011; Rohenkohl et al., 2012; Morillon et al., 2016; Breska and Ivry, 2021). In most of the experimental settings employed to study rhythmic attention, the target location is fixed, eliminating any form of spatial uncertainty. However, in everyday life, or in more ecological situations, adaptive behavior not only requires the selection of the optimal time window for target appearance but also the optimal location in space. The question of whether the underlying mechanisms of spatial and temporal attention work independently or in a combined fashion to improve behavior is still debated (Seibold et al., 2020; Boettcher et al., 2022; Tal-Perry and Yuval-Greenberg, 2022).
The relation between spatial and temporal attention has been mainly investigated in protocols where symbolic cues predict both the target location and the most likely moment of target onset. The literature points to independent processes serving spatial and temporal attention in the context of detection tasks, as revealed by independent and additive effects (Olk, 2014; Weinbach et al., 2015). The pattern changes when the perceptual demand associated to the task increases. For instance, Rohenkohl et al. (2014) asked participants to discriminate the orientation of a Gabor-patch stimulus that was preceded by an arrow-like cue. The arrow direction predicted the Gabor location, whereas the arrow color informed about the likely onset of the Gabor (800 ms/2,000 ms). Temporal expectations were only beneficial at the attended location, in accordance with a previous study in which visual rhythmic cues were used to deploy spatial and temporal attention (Doherty et al., 2005). To account for the observed results, the authors proposed a neurophysiological model of spatiotemporal attention according to which temporal orienting leads to time-specific synchronization of neural populations in specific retinotopic receptive fields. The effects of temporal orienting would thus be spatially constrained, at least when spatial attention is endogenously driven by symbolic cues.
In the study by Rohenkohl et al. (2014), both temporal and spatial orienting operated through endogenous symbolic cues. A few studies have instead combined endogenous spatial cues and rhythmic expectations. In the paradigm by Kizuk and Mathewson (2017), a series of visual entrainers were flashed at the alpha frequency band (every 83.33 ms, 12 Hz) to enhance sensory processing at specific moments in time (periodic fluctuations). Targets were briefly flashed to the left or right location, either “in-time” (83.33 ms or 166.66 ms) or “out-of-time” (41.66 ms or 125 ms) with respect to the entrainers. Target location was predicted by an arrow-like cue that was presented at the beginning of each trial (before the entrainers). Interestingly, the authors observed interactive effects between spatial orienting and entrainment, which were indeed opposite to what could be predicted following the neurophysiological model of spatiotemporal attention (Rohenkohl et al., 2014): performance improved for targets appearing “in-time,” compared to “out-of-time,” only at invalidly attended locations.
Two further studies supply the current debate over the combination of endogenous spatial attention and rhythm-based temporal expectations. Jones (2015; 2019 Experiment 1) showed that orienting by means of endogenous spatial attention and rhythmic sequences were independent and manifested themselves in additive effects. In his work, participants detected (or discriminated) a lateralized target that was preceded by a synchronous rhythm. Spatial attention was directed to the left or right location with symbolic cues (either color, sound, or touch manipulations, depending on the experiment). The moment of target appearance was also manipulated, as targets were presented “in-time” or “out-of-time” (early or late) with the preceding rhythm. In several experiments, with different sensory modalities, Jones (2015, see also Jones, 2019) consistently demonstrated independent effects between spatial and temporal orienting elicited by rhythmic cues.
However, in the studies cited above, it is difficult to disentangle the role of rhythm and foreperiod in temporal orienting. Targets could indeed appear after variable delays (commonly called foreperiods), giving the opportunity to build expectations on the basis of elapsing time, which typically translates into better performance at longer foreperiods, i.e, the foreperiod effect (Niemi and Näätänen, 1981; Capizzi and Correa, 2018). The foreperiod effect is formally described by the hazard function (i.e., the conditional probability that an event will occur given that it has not yet occurred; Janssen and Shadlen, 2005; Herbst et al., 2018; Visalli et al., 2019, 2021). Both Kizuk and Mathewson (2017) and Jones (2015) investigated the relationship between endogenous spatial orienting and temporal expectations based on a mixture of rhythmic information and the foreperiod effect. For instance, as Kizuk and Mathewson collapsed intervals “in-time” with the entrainers (83.33 ms and 166.66 ms), and intervals “out-of-time” (41.66 ms and 125 ms), the effect of temporal orienting could have resulted from both entrainment and elapsing time. With respect to the study of Jones (2015), a pure effect of entrainment should have led to a facilitation effect for “in-time” targets as compared to both early and late targets (U-shaped performance). By contrast, there was no evidence for an advantage of “in-time” targets as compared to “late” targets, thus suggesting that temporal orienting could have been masked by the foreperiod effect.
Considering that the relation between endogenous spatial attention and rhythmic orienting remains poorly understood, the objective of the present study was to further investigate the combined effects of spatial and temporal attention. Temporal expectations were generated by rhythms, rather than guided by the hazard function. Targets were indeed preceded by a regular or irregular rhythm to enhance time-specific synchronization in the former situation. Unlike previous studies, we used a fixed foreperiod duration between the last rhythm and the target in order to obtain a “pure” effect of rhythm on target processing. The fixed duration was twice as long as the ISI used in the regular sequence in Experiments 1, 2, and 3 (see Lange, 2010; Cutanda et al., 2015, for a similar procedure) to obtain a “pure” rhythm effect and to balance the weight of the foreperiod in regular and irregular rhythm trials. In Experiment 4, the role of the foreperiod in the combination of spatial attention and temporal expectations based on rhythms was further explored (using a variable foreperiod of 400 ms and 800 ms). Cue color was used to manipulate endogenous spatial attention in all the experiments, but the type of cue changed between experiments. Besides controlling for the foreperiod effect, we also manipulated how spatial and temporal information was delivered. Previous studies on the relationship between rhythm-based temporal expectations and endogenous spatial attention have manipulated spatiotemporal information either with independent or integrated cues. However, the precise role of this manipulation has been somehow neglected in previous studies. In Experiment 1, two independent cues were used to provide spatial and temporal information, as in the study by Kizuk and Mathewson (2017). In Experiment 2, spatial and temporal information was still conveyed by independent cues but we used a central rhythmic cue to orient attention in space (as in the studies by Jones, 2015, 2019), which might facilitate the integration of spatial and temporal information. Finally, in Experiments 3 and 4, we used a peripheral rhythmic cue as the contour of the placeholder flickered in different colors to provide both spatial and temporal information (hereafter referred to as integrated cues).
2. Experiment 1: independent cues
The goal of Experiment 1 was to investigate whether target detection could be enhanced by means of endogenous spatial symbolic cues and visually-presented rhythmic sequences. Spatial information was provided by manipulating the color of the fixation cross, which predicted the target location on approximately 70% of the trials (see Methods). Additionally, targets were always preceded by a series of placeholders flickering either in a synchronous or asynchronous pace (hereafter referred to as regular and irregular rhythm, respectively). We aimed to test whether participants could combine spatial endogenous orienting with rhythmic information to enhance target detection while controlling for the foreperiod effect. In this first experiment, two independent cues provided both types of information independently.
2.1. Method
2.1.1. Participants
Thirty-eight undergraduate psychology students from the University of Paul Valéry Montpellier (France) participated in the study as part of a course requirement. All participants (in this and the following experiments) had a normal or corrected-to-normal vision, none of them was color-blind, and all gave written informed consent before their inclusion. The studies had ethical approval from the local committee (CER UPVM-n°2020-02) and were conducted in accordance with the Declaration of Helsinki.
Three participants were excluded from data analysis due to excessive responses to catch trials (> 40%). One additional participant was excluded for low performance (>20% missing responses), whereas another participant was excluded for low compliance with task instructions (only one response on invalid trials), leaving a final sample size of 33 participants (mean age = 19.80 years, age range = 18–26 years, 3 males, 5 left-handed). A posteriori sensitivity power analysis (G*Power 3 software; Faul et al., 2007) showed that the sample size was adequate to detect significant (α = 0.05) mean differences between two dependent means (i.e., the main effect or the interaction effect of a 2-by-2 repeated measures ANOVA) with a medium effect size d = 0.5 (Cohen, 1977) and a statistical power of 0.80.
2.1.2. Apparatus and stimuli
The experiment was run on 22″ Intel® Core™ i5-64002 Duo personal computers in a group testing room at the University of Paul Valéry Montpellier. Stimulus presentation and data recording were controlled by E-prime v2 software (Schneider et al., 2002). The viewing distance was approximately 60 cm. All stimuli were presented against a gray background. Stimuli consisted of a fixation point, two placeholders, and a target. The fixation point was a black cross (0.38° × 0.38° visual angle) that was filled in with red or green color to signal the likely spatial location of target appearance, or with black color during the inter-trial-interval (ITI) (see below). The placeholders were two gray circles (diameter: 0.21° of visual angle; located 0.57° to the left and right of the fixation cross) whose contours were lighter than the background gray color. The target was a Gabor patch (0.17°) that could appear inside one of the two placeholders. For comparison with future studies involving discrimination requirements, the Gabor was tilted either 45° to the left or 45° to the right, with each orientation equally likely to be presented. Gabor patches were created in Matlab (version 2018a; Mathworks Inc., Natick, MA) with a maximum contrast of 1.
2.1.3. Procedure and task
Figure 1 illustrates the timing and the sequence of events in a given trial. Each trial started with the presentation of a central fixation cross for a random duration ranging from 1,000 to 1,500 ms. The color of the fixation cross (green or red) indicated the side (left or right) at which the target was more likely to occur (cue colors were counterbalanced between participants). The (red or green) fixation cross remained on the screen during the entire trial. Next, a series of placeholders (six in total) were displayed sequentially to create a rhythmic sequence. Specifically, each placeholder's presentation lasted for 50 ms and was spaced from the other one by a blank inter-stimulus interval (ISI). The duration of the ISI varied as a function of the rhythm condition used in the trial, assuming either a fixed duration of 400 ms (regular rhythm) or a random duration among the following values: 100, 250, 400, 550, and 700 ms (irregular rhythm). For both regular and irregular sequences, the ISI between the last placeholder and the target (i.e., the foreperiod) was always 800 ms (i.e., twice as long as the ISI used in the regular sequence; see Lange, 2010; Cutanda et al., 2015, for a similar procedure). Therefore, both regular and irregular rhythmic sequences comprised the same number of visual stimuli (6 placeholder repetitions) and had an identical duration before target onset, such that the only difference between the two conditions concerned the regularity of the rhythm. After the foreperiod elapsed, the Gabor stimulus appeared inside one of the two placeholders for 100 ms. Participants had to respond to the onset of the Gabor by pressing the spacebar on the computer keyboard. A maximum interval of 1,500 ms was allowed to respond. The ITI lasted for 1,000 ms. During the ITI, the color of the fixation cross changed to black.
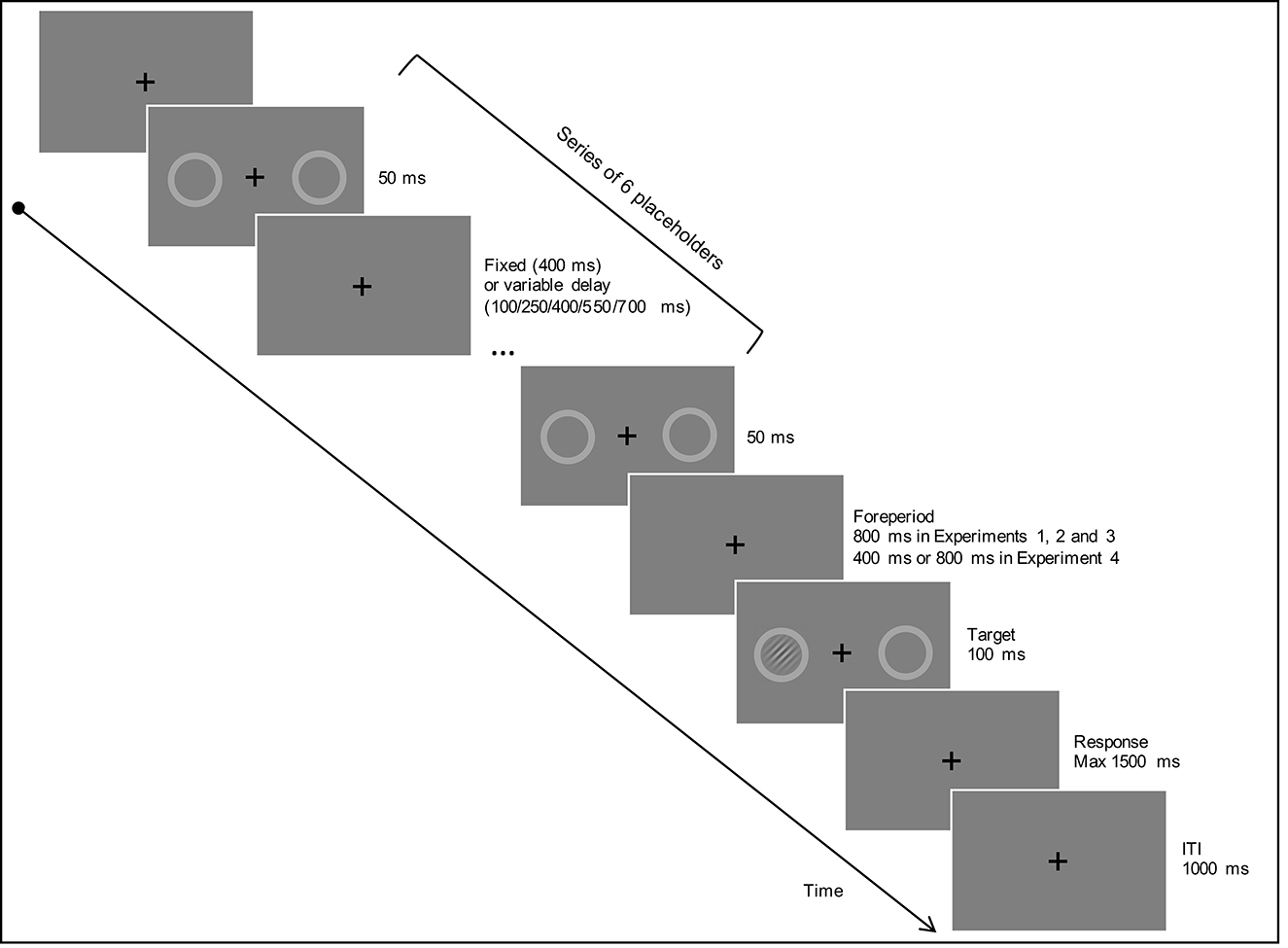
Figure 1. Illustrates the timing and the sequence of events in a given trial in Experiments 1–4. For Experiments 1 and 2, the color of the fixation cross oriented spatial attention to the left or right side. The fixation cross was steady in Experiment 1 and flickered at the same pace as the rhythm in Experiment 2. In Experiments 3 and 4, the fixation cross was presented in black (as in the figure) and the color of the placeholders changed to orient spatial attention. Please refer to the main text for further details on each experiment.
Participants were explicitly instructed to keep their gaze on the fixation cross at all times. They were encouraged to use the color of the fixation cross to predict the likely location of target onset, whereas they were told that the preceding rhythm was task-irrelevant and could therefore ignore it. The task consisted of four blocks, for a total of 192 trials, equally divided into regular and irregular rhythm conditions (96 trials each). For each rhythm condition, 68 were spatially valid trials (in which the Gabor stimulus appeared at the location indicated by the color cue, 70.83%), 20 were spatially invalid trials (in which the Gabor stimulus appeared at the opposite location to the one indicated by the color cue, 20.83%), and 8 were catch trials (in which no Gabor stimulus was presented, 8.33%).
The experimental blocks were preceded by a short practice session comprising two sequential parts. In the first part, participants completed 10 trials to familiarize themselves with the general task structure. No instructions about the meaning of the fixation cross were provided. In the second part, comprising 10 extra practice trials, they were informed about the meaning of the fixation cross color and instructed to use it to predict the target location. After each practice trial, participants received feedback on their reaction time (RT) performance; the French translations for the expressions: “Correct!,” “Correct! But try to be faster” (for responses slower than 700 ms), “Pay attention! Target absent” (for responses to catch trials), and “Too late! Be faster” (for no responses), were displayed for 1,000 ms. No feedback was given during experimental trials. The experiment lasted about 35 min.
2.1.4. Data analysis
Catch trials and data from the practice session were discarded before any further analysis. Trials without responses, trials with responses given during the foreperiod, and trials with premature responses (i.e., RTs < 100 ms) were excluded (0.6% of all the trials). For each trial type, RT values more extreme than one and a half times the interquartile range (i.e., the difference between the upper and lower quartile) above the upper quartile or below the lower quartile were identified as outliers (Borcard et al., 2011; see also Vallesi et al., 2022, for a similar approach) and removed (5.75% of the remaining trials). Mean RTs were then computed for each trial type and submitted to a repeated-measures analysis of variance (ANOVA) with Validity (valid, invalid) and Rhythm (regular, irregular) as within-participant factors (JASP Team, 2022).
2.2. Results and discussion
The ANOVA yielded significant main effects of Validity [F(1,32) = 43.06, p < 0.001, η2p = 0.57], and Rhythm [F(1,32) = 29.67, p < 0.001, η2p = 0.48], showing that participants were faster for valid compared to invalid trials (M = 318 ms and M = 332 ms, respectively), and after the regular compared to the irregular rhythm (M = 318 ms and M = 331 ms, respectively), (see Figure 2). The Validity by Rhythm interaction was not significant [F(32,1) = 0.12, p = 0.74, ns, η2p = 0.004].
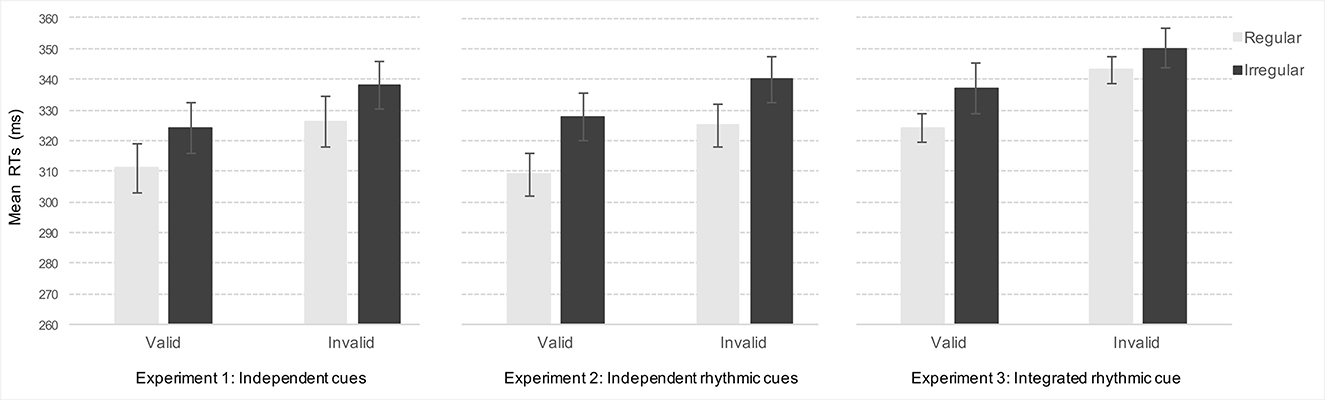
Figure 2. Depicts mean reaction times (RTs) in milliseconds (ms) for valid and invalid targets as a function of Rhythm (regular or irregular) in Experiments 1–3.
Despite the lack of significant Validity by Rhythm interaction in our data, we were interested in testing whether the effect of rhythm was significant in both spatial conditions. Additional post-hoc analyses, Bonferroni corrected, confirmed that the effect of Rhythm significantly enhanced RTs both at valid and invalid target locations (p < 0.001 and p = 0.04, respectively).
In sum, Experiment 1 suggests that endogenous spatial cueing and rhythmic orienting can act independently to enhance target detection and yield additive effects. However, the combination of spatial and temporal attention might depend on how information is conveyed by the cues and whether the integration of spatial and temporal information is favored. When separate and dissociable cues convey spatial and temporal information, one could argue that spatial expectations might be generated first, allowing for a later occurrence of rhythmic expectations that would develop independently from spatial ones, thus limiting their interaction (Mattler, 2003). Experiment 2 tests the possibility that rhythmic cues could favor the interaction between spatial and temporal attention by triggering the updating of spatial information at a regular or irregular pace.
3. Experiment 2: independent rhythmic cues
Experiment 2 was very similar to Experiment 1 except that the endogenous spatial cue provided by the color of the fixation cross was not steady but rather flickered at the same pace as the placeholders (see Figure 1).
3.1. Method
3.1.1. Participants
Thirty-five new undergraduate psychology students took part in the study, but only 31 were included in the analysis (mean age = 20.26 years, age range = 18–30 years, 6 males, 6 left-handed). Data from two participants were excluded for excessive responses to catch trials (> 40%), whereas two additional participants were discarded for the presence of a high proportion of missing responses (> 20%). A posteriori sensitivity power analysis (G*Power 3 software; Faul et al., 2007) showed that the sample size was adequate to detect significant (α = 0.05) mean differences between two dependent means (i.e., the main effect or the interaction effect of a 2-by-2 repeated measures ANOVA) with a medium effect size d = 0.52 (Cohen, 1977) and a statistical power of 0.80.
3.1.2. Apparatus, stimuli, and procedure
The apparatus, stimuli, and procedure were the same as in Experiment 1 with the following exception: the fixation cross flickered at the same pace (regular or irregular) of the rhythm used in a given trial. Participants were instructed that only the color of the fixation cross, but not its flickering, was predictive of the target location.
3.1.3. Data analysis
The same cleaning procedure (0.1% of rejected trials) and RT outlier removal (4.32%) were applied to the data as in Experiment 1. A 2 × 2 repeated measures ANOVA was used on mean RTs, with Validity (valid, invalid) and Rhythm (regular, irregular) as within-participant factors.
3.2. Results and discussion
Replicating Experiment 1, the ANOVA yielded significant main effects of Validity, F(1,30) = 17.39, p < 0.001, η2p = 0.37, and Rhythm, F(1,30) = 52.59, p < 0.001, η2p = 0.63. As expected, mean RTs were faster for valid targets (M = 318 ms) compared to invalid targets (M = 333 ms), and when targets were preceded by the regular rhythm (M = 317 ms) compared to the irregular rhythm (M = 334 ms). The Validity by Rhythm interaction was not significant, F(1,30) = 1.69, p = 0.20, η2p = 0.05 (see Figure 2). Post-hoc analyses, Bonferroni corrected, confirmed that the effect of Rhythm significantly enhanced RTs at valid and invalid target locations (p < 0.001 and p = 0.002, respectively).
To summarize, Experiment 2 showed again no evidence for an interaction between spatial and temporal orienting. As expected, target detection improved at validly attended (as compared to invalidly attended) locations, and when preceded by a regular (as compared to an irregular) rhythm. Despite the use of rhythmic spatial cues, the two effects did not interact.
4. Experiment 3: integrated rhythmic cues
In Experiment 3, spatial and temporal information was conveyed by a single cue to emphasize their integration. According to the adjusted expectancy model, the type of cues influences how expectations combine (Mattler, 2003), favoring additive effects with independent and separate cues but leading to interactive effects with an integrated cue. Rhythmic information was thus provided by the flickering of the placeholders (triggering regular or irregular rhythms) as in Experiments 1 and 2, but the placeholder color was manipulated to endogenously orient spatial attention. Therefore, a single signal, a red or green placeholder flickering, could serve to anticipate both the moment in time in which the target was presented and its location. We hypothesized that interactive effects between endogenous spatial cueing and rhythmic orienting might be observed when spatial and temporal information is integrated using an integrated cue (Mattler, 2003).
4.1. Method
4.1.1. Participants
Fifty new undergraduate psychology students took part in the study. Of these, six participants were excluded for excessive responses to catch trials (> 40%), leaving a final sample size of 44 (mean age= 20.55 years, age range= 18–34 years, 7 males, 5 left-handed). Note that more participants were included in Experiment 3 because of course requirements and enrollment rates. As in the previous experiments, the sample size was adequate to detect significant (α = 0.05) mean differences between two dependent means with a small/medium effect size d = 0.43 (Cohen, 1977) and a statistical power of 0.80.
4.1.2. Apparatus, stimuli, and procedure
The apparatus, stimuli, and procedure were the same as in Experiment 1 except for the following aspects. The fixation cross was always presented in black, whereas the color (red or green) of the placeholders predicted the target location. The placeholders turned gray at the target onset. Participants were instructed that only the color of the placeholders, but not their flickering, was useful to anticipate the target location.
4.1.3. Data analysis
After the cleaning procedure (2.13 % of rejected trials) and RT outlier removal (3.67 %), mean RTs were submitted to a 2 × 2 repeated measures ANOVA with Validity (valid, invalid) and Rhythm (regular, irregular) as within-participant factors.
4.2. Results and discussion
Again, the ANOVA showed significant main effects of Validity, F(1,43) = 12.71, p < 0.001, η2p = 0.23, and Rhythm, F(1,43) = 13.15, p < 0.001, η2p = 0.23. Participants were faster for valid (M = 331 ms) compared to invalid targets (M = 346 ms), and when targets were preceded by the regular rhythm (M = 334 ms) compared to the irregular rhythm (M = 343 ms). The Validity by Rhythm interaction was not significant, F(1,43) = 1.35, p = 0.25, η2p = 0.03 (see Figure 2). However, post-hoc analyses, Bonferroni corrected, showed that the effect of Rhythm was significant only at valid target locations (p < 0.001), but not at invalid locations (p = 1).
In Experiment 3, we expected to emphasize the integration of spatial and temporal attention by using an integrated cue that provided information about both the target location and the moment of target appearance. Although we did not observe a significant Validity by Rhythm interaction, additional explorative analyses revealed that rhythmic information only improved performance at valid locations. Overall, the present results hint at the possibility that temporal orienting can be spatially constrained when an integrated cue is used, in line with the neurophysiological model of spatiotemporal attention (Doherty et al., 2005; Nobre and Rohenkohl, 2014; Rohenkohl et al., 2014). In Experiment 4, we aimed to further test this hypothesis in a design similar to the one of Experiment 3 with a single rhythmic cue conveying both spatial and temporal information. Moreover, we were interested in controlling for another aspect common to the last three previous experiments. That is, even if we employed one foreperiod for both regular and irregular rhythms, one might wonder whether the use of a long duration doubling the ISI of the regular rhythm (i.e., 800 ms) might have somehow introduced some sort of temporal preparation for the target in both regular and irregular rhythm conditions, thus, mitigating general rhythmic effects. To strengthen the reliability of the rhythm in Experiment 4, we intermixed two foreperiod durations, one matching the regular rhythm (400 ms, “in time”) and one doubling it (800 ms, “out of time”) on a trial-by-trial basis. Our objective was to test whether the combination of spatial and temporal attention could be enhanced when targets appeared at the in-time critical foreperiod (400 ms) as compared to when they appeared at the longer foreperiod (800 ms).
5. Experiment 4: integrated rhythmic cue with variable foreperiod
In Experiment 4, we added an in-time critical foreperiod (400 ms) in opposition to Experiments 1–3 in which targets were only presented at a critical foreperiod that was a multiplication of the in-time rhythm (two steps of the regular rhythms-800 ms).
5.1. Method
5.1.1. Participants
Forty-nine new undergraduate psychology students took part in the study. Of these, six participants were excluded for excessive responses to catch trials (> 40%), while one was excluded for excessive missing responses (> 20%), leaving a final sample size of 42 (mean age= 20.21 years, age range= 18–35 years, 4 males, 4 left-handed). As in Experiment 3, the sample size was adequate to detect significant (α = 0.05) mean differences between two dependent means with a small/medium effect size d = 0.44 (Cohen, 1977) and a statistical power of 0.80.
5.1.2. Apparatus, stimuli, and procedure
The apparatus, stimuli, and procedure were the same as in Experiment 3 except that two foreperiods of either 400 or 800 ms were used. For each rhythm condition, there were 36 valid-short foreperiod trials, 36 valid-long foreperiod trials, 12 invalid-short foreperiod trials, 12 invalid-long foreperiod trials, and 8 catch trials.
5.1.3. Data analysis
The same cleaning procedure (1.69% of rejected trials) and RT outlier removal (6.21%) were applied to the data as in Experiment 1. A 2 × 2 repeated measures ANOVA was used on mean RTs, with Validity (valid, invalid), Rhythm (regular, irregular), and Foreperiod (short, long) as within-participant factors.
5.2. Results and discussion
As predicted, the ANOVA revealed significant main effects of Validity, Rhythm, and Foreperiod [F(1,41) = 17.52, p < 0.001, η2p = 0.29, F(1,41) = 3.98, p = 0.053, η2p = 0.09, F(1,41) = 143.14, p < 0.001, η2p = 0.78, respectively]. Targets appearing at the attended location or after a regular rhythm were detected faster than targets at the unattended location or after an irregular rhythm, thus confirming efficient spatial and temporal orienting. Regarding the foreperiod, participants were faster to targets appearing after an 800 ms delay, compared to the 400 ms delay (M = 340 ms and M = 370 ms, respectively). None of the other interactions reached significance [F(1,41) = 2.06, p = 0.16, η2p = 0.05 for Rhythm x Foreperiod; Fs<1 for Validity x Foreperiod and Validity x Rhythm x Foreperiod], except for the Validity x Rhythm interaction that was marginally significant [F(1,41) = 3.97, p = 0.053, η2p = 0.09, see Figure 3]. Post-hoc analyses, Bonferroni corrected, revealed that participants benefited from regular rhythm only on valid trials (M = 349 ms and M = 359 ms, respectively, for regular and irregular rhythm, p < 0.001). At invalid locations, rhythm did not enhance target detection (M = 363 ms and M = 363 ms, respectively for regular and irregular rhythm, p = 0.995).
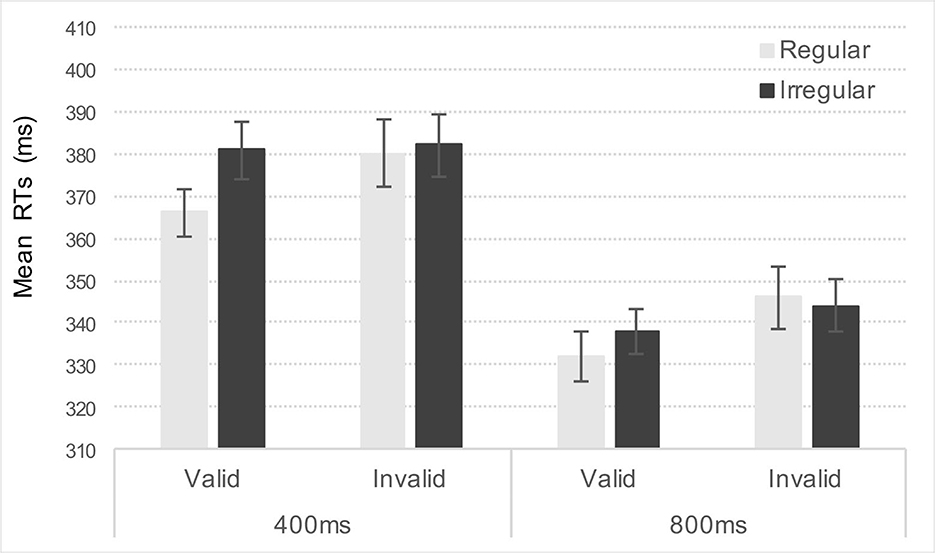
Figure 3. Depicts mean reaction times (RTs) in milliseconds (ms) for valid and invalid targets as a function of Rhythm (regular or irregular) for the 400 ms and 800 ms foreperiods.
In sum, Experiment 4 showed that endogenous spatial attention and rhythm-based temporal expectations can interact under certain situations in simple detection tasks. Importantly, this interaction is independent of the foreperiod effect (no significant Rhythm x Foreperiod and Validity x Rhythm x Foreperiod interactions). Performance benefits from regular rhythms were only observed at valid locations, regardless of whether targets appeared at 400 or 800 ms after the last entrainer.
To clarify the impact of independent and integrated cues on the combination of temporal and spatial attention, we conducted a global analysis with the factor Cues manipulated between participants (Independent cues by grouping Experiments 1 and 2 vs. Integrated cues by grouping Experiments 3 and 4) and the factors Validity and Rhythm manipulated within participants.1 The analysis showed a main effect of Cue [F(1,148) = 12.5, p < 0.001, η2p = 0.08], suggesting that mean RTs were slower for integrated compared to independent cues (M = 348 ms and M = 325 ms, respectively). As expected, the analysis revealed main effects of Validity and Rhythm [F(1,148) = 63.17, p < 0.001, η2p = 0.29 and F(1,148) = 74.04, p < 0.001, η2p = 0.33, respectively]. Interestingly, the Validity x Rhythm interaction was significant [F(1,148) = 5.24, p = 0.02, η2p = 0.03] and was not qualified by the Cue factor [F(1,148) = 0.98, p = 0.32, η2p = 0.007). These results revealed that the effect of Rhythm was significant at both valid and invalid locations (ps < 0.001, Bonferroni corrected post-hoc), but was larger at valid locations compared to invalid locations (see Figure 4). Additionally, the analysis highlighted a significant Rhythm x Cue interaction [F(1,148) = 8.37, p < 0.004, η2p = 0.05]. Bonferroni corrected post-hoc comparisons confirmed that although the effect of Rhythm was significant for both independent and integrated cues (ps < 0.001), the effect was larger when using independent cues. The interaction Validity x Cue was not significant [F(1,148) = 0.36, p = 0.55, η2p = 0.002]. In short, the present series of experiments reveals two main findings. First, temporal expectations based on rhythms improve target detection to a larger extent at validly attended locations (an effect that is evident when a large number of participants are analyzed in a simple detection task). Second, the use of integrated cues providing both spatial and temporal attention reduces the beneficial effect of temporal expectations based on rhythms.
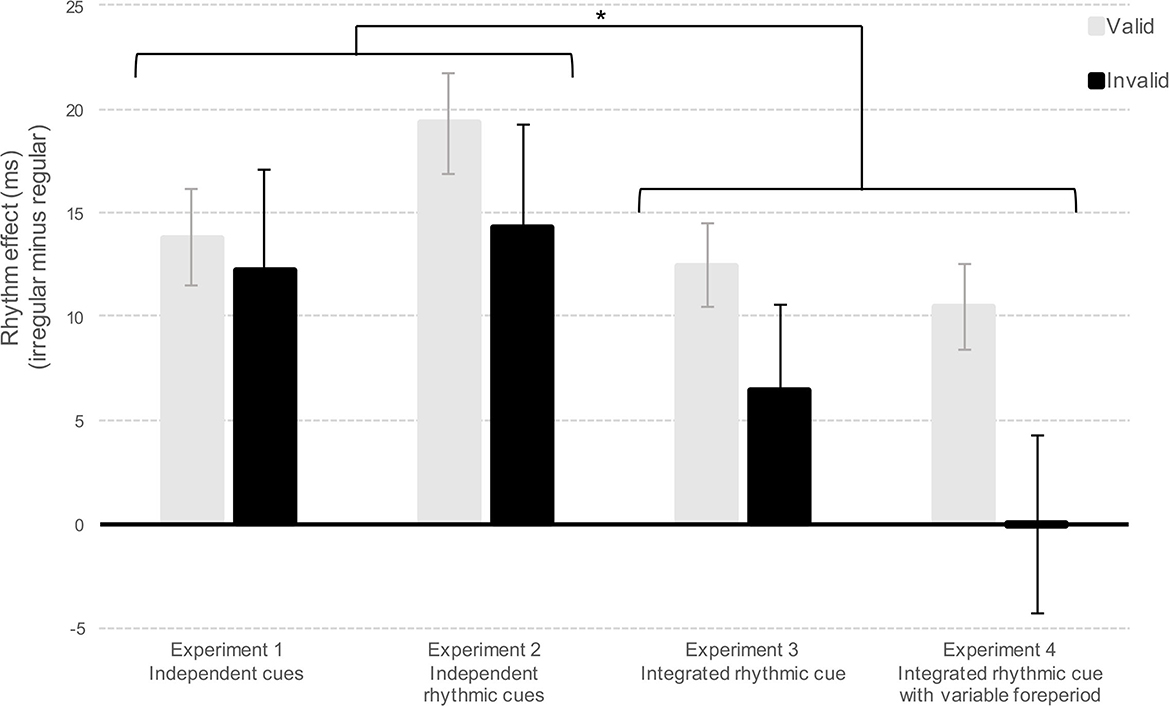
Figure 4. Depicts the Rhythm effect (RTs from regular minus RTs from irregular) in milliseconds (ms) for Experiments 1, 2, 3, and 4 as a function of spatially valid or invalid locations.
6. General discussion
The present study investigated the relationship between endogenous spatial attention and rhythmic temporal expectations. Across four experiments, participants detected a suprathreshold Gabor target appearing after a regular or irregular rhythm, either at the left or right side of space. In Experiments 1 and 2, attentional orienting in space and time was delivered by independent cues. The color of the fixation cross remained steady throughout the whole trial in Experiment 1, or flickered at the same pace as the rhythm in Experiment 2 (central rhythmic cue). In Experiments 3 and 4, an integrated cue (the flickering of the placeholders with colored contours, see Figure 1) was employed to orient spatial and temporal attention. Overall, target detection was enhanced when targets were preceded by the regular compared to the irregular rhythm and when presented at valid compared to invalid locations. When considering Experiments 1 and 2 separately, our data suggest independent and additive effects of endogenous spatial orienting and rhythmic temporal expectations on target detection. However, a global analysis in which the four experiments were collapsed as a function of cue type (Independent in Experiments 1 and 2; Integrated in Experiments 3 and 4) revealed two major findings. First, the data pointed to minor benefits of temporal expectations when temporal information was delivered by integrated cues (Experiments 3 and 4), as compared to independent cues (Experiments 1 and 2). Second, temporal expectations based on rhythms appeared to be stronger at valid compared to invalid locations.
6.1. Independent vs. integrated cues
Extending previous studies, our results showed that the advantage provided by regular rhythms depended on how the two expectancies were generated. When cues consisted of two clearly separable events, the effect of rhythm was maximized. However, when the same cue was used to generate both expectancies, temporal expectations were less beneficial. Our data are in line with the idea of a general phenomenon of expectancy interaction detailed in the Adjusted Expectancy model (Mattler, 2003, 2004), according to which, cues are processed in parallel when consisting of two separate pieces of information. Hence, failure to comply with one expectation does not interfere with the remaining cueing information. However, when the two pieces of information are integrated, partial noncompliance disrupts the global cueing effects. Previous studies on the impact of combined perceptual expectancies in a broader perspective demonstrated reduced or even absent spatial cueing effects on trials with an unexpected target stimulus as compared to an expected target stimulus (Klein, 1980; Lambert and Hockey, 1986; Klein and Hansen, 1987, 1990; Lambert, 1987; Kingstone, 1992). A similar result has been observed with perceptual and motor expectancies (Mattler, 2003, 2004). The Adjusted Expectancy model (Mattler, 2003, 2004) could account for the attenuated benefits afforded by rhythms with integrated, as compared to independent, cues. However, Kingstone (1992) proposed that crosstalk between expectations led to favoring the more automatic information compared to a more resource-demanding attribute. This view is hard to reconcile with our findings given that rhythm-based temporal expectations are supposed to be more automatically driven than endogenous spatial attention. One could hypothesize that the cue associated with task-relevant information, here the spatial information, is prioritized when combining expectancies. In our study, integrated cues did not impact the endogenous orienting of spatial attention which was predictive of target location, in opposition to the task-irrelevant rhythm. The integration process might depend on the task relevance of spatial and temporal information. Overall, these observations suggest a more global structural mechanism for integrating expectations, beyond spatial and temporal orienting.
6.2. Independent vs. interactive contributions of spatial and temporal attention
In the literature, there is compelling evidence that endogenous spatial orienting operates independently of temporal attention in low-demanding tasks, at least when using symbolic cues or contextual associations (i.e. distribution of foreperiods). For example, Weinbach et al. (2015) conducted a study in which participants detected targets as fast as possible. In the first two blocks, targets were preceded by a symbolic central cue that either predicted the target location or the moment of target appearance. In a third block, the cue predicted, as a function of its color and shape, both the location and the moment of target appearance. This study provided evidence for independent and additive effects of spatial and temporal endogenous forms of attention. Olk (2014) drew similar conclusions with pure symbolic cues that predicted the target location, the moment of target appearance, or both. More recently, the combination of endogenous spatial cues and temporal expectations elicited by contextual associations was also investigated (Tal-Perry and Yuval-Greenberg, 2022). In that experiment, combining endogenous spatial cues with a blocked manipulation of temporal expectations (i.e., different probability distributions of the foreperiod were used between participants) led to independent effects of spatial attention and temporal expectations (foreperiod and sequential effects). These studies demonstrate that spatial orienting is independent of temporal expectations emerging from associative or contextual information (symbolic cues, foreperiod, and sequential effects).
With respect to rhythm-based expectations, the picture is less clear-cut with studies reporting either independent or interactive effects of endogenous spatial attention and rhythmic expectations (Jones, 2015, 2019; Kizuk and Mathewson, 2017). Our results complement these observations by showing that rhythmic expectations and endogenous spatial orienting can combine interactively to foster target processing even in a simple reaction time task with low perceptual demands. The benefit afforded by regular rhythms was indeed larger in the attended region of space. Yet, this effect only emerged when temporal uncertainty was introduced by means of a variable foreperiod (Experiment 4) or when collapsing the data of the four experiments, but it still suggests that the interaction of spatial and temporal attention might occur in a low-demanding context. Temporal expectations based on rhythms facilitated target detection at both attended and unattended locations in our study, but exerted a larger effect in the attended region of space. The finding that rhythmic temporal expectations can be spatially constrained is consistent with the spatiotemporal neurophysiological model of spatiotemporal attention (Doherty et al., 2005; Nobre and Rohenkohl, 2014; Rohenkohl et al., 2014). This model assumes that temporal orienting leads to time-specific synchronization of neural populations in specific retinotopic receptive fields. The present study extends this model by showing an interactive contribution of spatiotemporal orienting to behavior, not only when temporal expectations are endogenously driven by symbolic cues but also when triggered by irrelevant synchronous rhythmic cues. Interestingly, this neurophysiological model emphasizes the role of task demands in this interaction, as temporal expectations are proposed to interact synergistically with spatial predictions to improve perceptual discrimination of visual events (Doherty et al., 2005; Rohenkohl and Nobre, 2011; Nobre and Rohenkohl, 2014; Rohenkohl et al., 2014; van Ede et al., 2020). Overall, our findings suggest that the proposal of the spatiotemporal view could likely apply to simple reaction-time tasks in well-powered experiments (see our global analysis). This observation raises questions about the role of the motor system in the combination of spatial and temporal attention. The contribution of the motor system in predictive behavior, and more specifically in temporal expectations, is not new to date and has been mainly investigated in the auditory domain (Schubotz, 2007; Morillon et al., 2014; Morillon and Baillet, 2017). Recent evidence also suggests that temporal expectations can enhance both motor preparation and perceptual discrimination as a function of task demands in the visual domain (van Ede et al., 2020). Here, we further show from a simple RT-task stressing motor preparation that the motor system could play a role in the combination of spatial and temporal attention.
Finally, with respect to our study, the interaction between spatial and temporal orienting approached significance when temporal uncertainty was introduced by using a variable foreperiod (Experiment 4). Of importance, these interactive effects were not modulated by the foreperiod, revealing that the orienting mechanisms could persist over time. Of importance, these interactive effects were not modulated by the foreperiod, thus extending previous work (Jones, 2015; Kizuk and Mathewson, 2017).
In addition to the debate of independent vs. interactive processes, further research should tackle the issue of the underlying processes responsible for interactive effects. Kizuk and Mathewson (2017) indeed reported interactive effects that are opposite to our findings and to the predictions of the neurophysiological model of spatiotemporal attention (Doherty et al., 2005; Rohenkohl and Nobre, 2011; Nobre and Rohenkohl, 2014; Rohenkohl et al., 2014). Participants localized toughly visible targets embedded in a stream of entrainers displayed at a pace of 12 Hz. By manipulating the foreperiod, targets appeared either “in-time” or “out-of-time” with the entrainers. Unexpectedly, they provided both behavioral and electrophysiological evidence for an enhancement of target processing when targets were presented at the unattended region of space (invalid with respect to a 70% validly colored cue). The study confirmed previous EEG evidence that spatial attention triggers more lateralized alpha power during the cue and target periods, and it additionally suggests that rhythmic entrainment would more easily modulate larger alpha power at the contralateral side of target expectation. As a consequence, larger benefits of temporal expectations are observed at unexpected regions of space where spatial attention is expected to inhibit alpha oscillations (Klimesch et al., 2007; Jensen and Mazaheri, 2010; Mathewson et al., 2011; Jensen et al., 2014; Kizuk and Mathewson, 2017).
Taking into consideration previous models that account for expectation combinations with various dimensions (such as space, feature, or response; Kingstone, 1992; Mattler, 2003, 2004) could help revisit these discrepancies by elaborating a more general view. Further investigation is definitely needed to draw more straightforward conclusions on the combination of spatial and temporal attention. Current theoretical frameworks stipulate that the relation between temporal orienting and spatial attention depends on perceptual task demands (van Ede et al., 2020). The present study adds further insights into this issue by revealing small but significant interactive effects in low-demanding detection tasks, thus paving the way for future investigation on the role of task demands in the relationship between spatial and rhythmic temporal attention.
Data availability statement
The raw data supporting the conclusions of this article will be made available by the authors, without undue reservation.
Ethics statement
The studies involving human participants were reviewed and approved by CER UPVM-n°2020-02-Université Paul Valéry Montpellier-Local Committee. The patients/participants provided their written informed consent to participate in this study.
Author contributions
PC, MC, and AC participated in the study conception and design. PC and MC programmed the experimental tasks, and PC was in charge of data collection. MC analyzed the data. PC wrote the draft manuscript. MC and AC provide substantial feedback to the draft and revised the draft critically for important intellectual content. All authors contributed to the article and approved the submitted version.
Funding
This work was supported by the Agence National de Recherche grant (ANR-18-CE28-0009-01) to PC and MC is supported by a grant (PID2021-128696NA-I00) funded by MCIN/AEI/10.13039/501100011033 and ERDF A way of making Europe, and by a María Zambrano Fellowship at the University of Granada from the Spanish Ministry of Universities and the European Union NextGeneration. AC is supported by MCIN/AEI/(research projects PSI2017-88136 and PID2020-119033GB-I00) and by ERDF A way of making Europe. AC was also supported by the Junta de Andalucía (I+D+I Programa Operativo FEDER Andalucía 2014-2020, B.SEJ.570.UGR20).
Conflict of interest
MC and AC declared that they were editorial board members of Frontiers, at the time of submission. This had no impact on the peer review process and the final decision.
The remaining author declares that the research was conducted in the absence of any commercial or financial relationships that could be construed as a potential conflict of interest.
Publisher's note
All claims expressed in this article are solely those of the authors and do not necessarily represent those of their affiliated organizations, or those of the publisher, the editors and the reviewers. Any product that may be evaluated in this article, or claim that may be made by its manufacturer, is not guaranteed or endorsed by the publisher.
Footnotes
1. ^As neither the interaction Validity x Foreperiod, nor the Rhythm x Foreperiod interaction reached significance (ps > 0.15), in the former analysis, we collapsed behavioral data for 400 and 800 ms in Experiment 4.
References
Boettcher, S. E. P., Shalev, N., Wolfe, J. M., and Nobre, A. C. (2022). Right place, right time: Spatiotemporal predictions guide attention in dynamic visual search. J. Exp. Psychol. Gen. 151, 348–362. doi: 10.1037/xge0000901
Borcard, D., Gillet, F., and Legendre, P. (2011). Numerical Ecology with R. New York: Springer. doi: 10.1007/978-1-4419-7976-6
Breska, A., and Ivry, R. B. (2018). Double dissociation of single-interval and rhythmic temporal prediction in cerebellar degeneration and Parkinson's disease. Proceedings of the National Academy of Science of the United States of America. 115, 12283–12288. doi: 10.1073/pnas.1810596115
Breska, A., and Ivry, R. B. (2021). The human cerebellum is essential for modulating perceptual sensitivity based on temporal expectations. eLife. 10, e66743. doi: 10.7554/eLife.66743.sa2
Capizzi, M., and Correa, Á. (2018). “Measuring temporal preparation.” in Timing and time perception: Procedures, measures, and applications. Ed. Brill, Leiden Boston, Eds A. Vatakis, F. Balci, M. Di Luca, and Á. Correa, 216–232. doi: 10.1163/9789004280205_011
Capizzi, M., Correa, A., and Sanabria, D. (2013). Temporal orienting of attention is interfered by concurrent working memory updating. Neuropsychologia. 51, 326–339. doi: 10.1016/j.neuropsychologia.2012.10.005
Chica, A. B., Martín-Arévalo, E., Botta, F., and Lupiáñez, J. (2014). The Spatial Orienting paradigm: how to design and interpret spatial attention experiments. Neurosci. Biobehav. Rev. 40, 35–51. doi: 10.1016/j.neubiorev.2014.01.002
Cohen, J. (1977). Statistical Power Analysis for the Behavioral Sciences (rev. ed.). Hillsdale, NJ: Lawrence Erlbaum Associates, Inc.
Coull, J. T., Cotti, J., and Vidal, F. (2016). Differential roles for parietal and frontal cortices in fixed versus evolving temporal expectations: dissociating prior from posterior temporal probabilities with fMRI. NeuroImage. 141, 40–51. doi: 10.1016/j.neuroimage.2016.07.036
Coull, J. T., and Nobre, A. C. (1998). Where and when to pay attention: the neural systems for directing attention to spatial locations and to time intervals as revealed by both PET and fMRI. J. Neurosci. 18, 7426–7435. doi: 10.1523/JNEUROSCI.18-18-07426.1998
Cutanda, D., Correa, Á., and Sanabria, D. (2015). Auditory temporal preparation induced by rhythmic cues during concurrent auditory working memory tasks. J. Exp. Psychol.: Hum. Perce. 41, 790–797. doi: 10.1037/a0039167
De la Rosa, M. D., Sanabria, D., Capizzi, M., and Correa, Á. (2012). Temporal preparation driven by rhythms is resistant to working memory interference. Front. Psychol. 3, 308. doi: 10.3389/fpsyg.2012.00308
Doherty, J. R., Rao, A., Mesulam, M. M., and Nobre, A. C. (2005). Synergistic effect of combined temporal and spatial expectations on visual attention. J. Neurosci. 25, 8259–8266. doi: 10.1523/JNEUROSCI.1821-05.2005
Faul, F., Erdfelder, E., Lang, A. G., and Buchner, A. (2007). G*Power 3: a flexible statistical power analysis program for the social, behavioral, and biomedical sciences. Behav. Res. Methods. 39, 175–191. doi: 10.3758/BF03193146
Herbst, S. K., Fiedler, L., and Obleser, J. (2018). Tracking temporal hazard in the human electroencephalogram using a forward encoding model. eNeuro. 5, ENEURO-0017. doi: 10.1523/ENEURO.0017-18.2018
Janssen, P., and Shadlen, M. N. (2005). A representation of the hazard rate of elapsed time in macaque area LIP. Nat. Neurosci. 8, 234–241. doi: 10.1038/nn1386
Jensen, O., Gips, B., Bergmann, T. O., and Bonnefond, M. (2014). Temporal coding organized by coupled alpha and gamma oscillations prioritize visual processing. Trends Neurosci. 37, 357–369. doi: 10.1016/j.tins.2014.04.001
Jensen, O., and Mazaheri, A. (2010). Shaping functional architecture by oscillatory alpha activity: gating by inhibition. Front. Hum. Neurosci. 4, 186. doi: 10.3389/fnhum.2010.00186
Jones, A. (2015). Independent effects of bottom-up temporal expectancy and top-down spatial attention: an audiovisual study using rhythmic cueing. Front. Integr. Neurosci. 8, 96. doi: 10.3389/fnint.2014.00096
Jones, A. (2019). Temporal expectancies and rhythmic cueing in touch: the influence of spatial attention. Cognition. 182, 140–150. doi: 10.1016/j.cognition.2018.09.011
Jones, M. R., Moynihan, H., MacKenzie, N., and Puente, J. (2002). Temporal aspects of stimulus-driven attending in dynamic arrays. Psychol. Sci. 13, 313–319. doi: 10.1111/1467-9280.00458
Kingstone, A. (1992). Combining expectancies. Q. J. Exp. Physiol. 44A, 69–104. doi: 10.1080/14640749208401284
Kizuk, S. A. D., and Mathewson, K. E. (2017). Power and phase of alpha oscillations reveal an interaction between spatial and temporal visual attention. J. Cogn. Neurosci. 29, 480–494. doi: 10.1162/jocn_a_01058
Klein, R. (1980). Does oculomotor readiness mediate cognitive control of visual attention? Attention and performance. 8, 259–276.
Klein, R., and Hansen, E. (1987). Spotlight failure in covert orienting. Bulletin Psychonomic Society. 25, 447–450. doi: 10.3758/BF03334737
Klein, R., and Hansen, E. (1990). Chronometric analysis of apparent spotlight failure in endogenous visual orienting. J. Exp. Psychol.: Hum. Percept. 16, 790–801. doi: 10.1037/0096-1523.16.4.790
Klimesch, W., Sauseng, P., and Hanslmayr, S. (2007). EEG alpha oscillations: the inhibition-timing hypothesis. Brain Res. Rev. 53, 63–88. doi: 10.1016/j.brainresrev.2006.06.003
Lambert, A. (1987). Expecting different categories at different locations and spatial selective attention. Q. J. Exp. Psychol. 39, 61–76. doi: 10.1080/02724988743000033
Lambert, A. J., and Hockey, R. (1986). Selective attention and performance with a multidimensional visual display. J. Exp. Psychol.: Hum. Perce. 12, 484. doi: 10.1037/0096-1523.12.4.484
Lange, K. (2010). Can a regular context induce temporal orienting to a target sound? Int. J. Psychophysiol. 78, 231–238. doi: 10.1016/j.ijpsycho.2010.08.003
Large, E. W., and Jones, M. R. (1999). The dynamics of attending: how we track time varying events. Psychol. Rev. 106, 119–159. doi: 10.1037/0033-295X.106.1.119
Mathewson, K. E., Lleras, A., Beck, D. M., Fabiani, M., Ro, T., and Gratton, G. (2011). Pulsed out of awareness: EEG alpha oscillations represent a pulsed-inhibition of ongoing cortical processing. Front. Psychol. 2, 99. doi: 10.3389/fpsyg.2011.00099
Mattler, U. (2003). Combined perceptual or motor-related expectancies modulated by type of cue. Percept. Psychophys. 65, 649–666. doi: 10.3758/BF03194589
Mattler, U. (2004). Combined expectancy effects are modulated by the relation between expectancy cues. The Quarterly journal of experimental psychology. A, Human experimental psychology. 57, 193–221. doi: 10.1080/02724980343000161
Morillon, B., and Baillet, S. (2017). Motor origin of temporal predictions in auditory attention. Proceedings of the National Academy of Sciences of the United States of America. 114, E8913–E8921. doi: 10.1073/pnas.1705373114
Morillon, B., Schroeder, C., and Wyart, V. (2014). Motor contributions to the temporal precision of auditory attention. Nat. Commun. 5, 5255. doi: 10.1038/ncomms6255
Morillon, B., Schroeder, C. E., Wyart, V., and Arnal, L. H. (2016). Temporal prediction in lieu of periodic stimulation. J. Neurosci. 36, 2342–2347. doi: 10.1523/JNEUROSCI.0836-15.2016
Niemi, P., and Näätänen, R. (1981). Foreperiod and simple reaction time. Psychol. Bull. 89, 133–162. doi: 10.1037/0033-2909.89.1.133
Nobre, A. C., and Rohenkohl, G. (2014). “Time for the fourth dimension in attention.” The Oxford handbook of attention, Eds A. C. Nobre and S. Kastner (Oxford University Press), 676–721. doi: 10.1093/oxfordhb/9780199675111.001.0001
Nobre, A. C., and van Ede, F. (2018). Anticipated moments: temporal structure in attention. Nat. Rev. Neurosci. 19, 34–48. doi: 10.1038/nrn.2017.141
Olk, B. (2014). Effects of spatial, temporal and spatiotemporal cueing are alike when attention is directed voluntarily. Exp. Brain Res. 232, 3623–3633. doi: 10.1007/s00221-014-4033-7
Rohenkohl, G., Cravo, A. M., Wyart, V., and Nobre, A. C. (2012). Temporal expectation improves the quality of sensory information. J. Neurosci. 32, 8424–8428. doi: 10.1523/JNEUROSCI.0804-12.2012
Rohenkohl, G., Gould, I. C., Pessoa, J., and Nobre, A. C. (2014). Combining spatial and temporal expectations to improve visual perception. J. Vis. 14:8, 1–13. doi: 10.1167/14.4.8
Rohenkohl, G., and Nobre, A. C. (2011). Alpha oscillations related to anticipatory attention follow temporal expectations. J. Neurosci, 31, 14076–14084. doi: 10.1523/JNEUROSCI.3387-11.2011
Sanabria, D., Capizzi, M., and Correa, A. (2011). Rhythms that speed you up. J. Exp. Psychol. Hum. Percept. Perform. 37, 236–244. doi: 10.1037/a0019956
Schneider, W., Eschman, A., and Zuccolotto, A. (2002). E-Prime Reference Guide. Pittsburge, PA: Psychology Software Tools.
Schubotz, R. I. (2007). Prediction of external events with our motor system: towards a new framework. Trends Cogn. Sci. 11, 211–218. doi: 10.1016/j.tics.2007.02.006
Seibold, V. C., Stepper, M. Y., and Rolke, B. (2020). Temporal attention boosts perceptual effects of spatial attention and feature-based attention. Brain and Cognition. 142, 105570. doi: 10.1016/j.bandc.2020.105570
Tal-Perry, N., and Yuval-Greenberg, S. (2022). The spatiotemporal link of temporal expectations: contextual temporal expectation is independent of spatial attention. J. Neurosci. 42, 2516–2523. doi: 10.1523/JNEUROSCI.1555-21.2022
Vallesi, A., Visalli, A., Gracia-Tabuenca, Z., Tarantino, V., Capizzi, M., Alcauter, S., et al. (2022). Fronto-parietal homotopy in resting-state functional connectivity predicts task-switching performance. Brain Struct. Funct. 227, 655–672. doi: 10.1007/s00429-021-02312-w
van Ede, F., Rohenkohl, G., Gould, I., and Nobre, A. C. (2020). Purpose-dependent consequences of temporal expectations serving perception and action. J. Neurosci. 40, 7877–7886. doi: 10.1523/JNEUROSCI.1134-20.2020
Visalli, A., Capizzi, M., Ambrosini, E., Kopp, B., and Vallesi, A. (2021). Electroencephalographic correlates of temporal Bayesian belief updating and surprise. NeuroImage. 231, 117867. doi: 10.1016/j.neuroimage.2021.117867
Visalli, A., Capizzi, M., Ambrosini, E., Mazzonetto, I., and Vallesi, A. (2019). Bayesian modeling of temporal expectations in the human brain. NeuroImage. 202, 116097. doi: 10.1016/j.neuroimage.2019.116097
Keywords: endogenous spatial orienting, attention, entrainment, foreperiod, rhythm, temporal expectations
Citation: Charras P, Chica AB and Capizzi M (2023) On the relationship between rhythm-based temporal expectations and endogenous spatial attention in simple reaction-time tasks. Front. Cognit. 2:1191595. doi: 10.3389/fcogn.2023.1191595
Received: 22 March 2023; Accepted: 24 May 2023;
Published: 13 June 2023.
Edited by:
Mei-Ching Lien, Oregon State University, United StatesReviewed by:
Irene Echeverria-Altuna, University of Oxford, United KingdomVerena Seibold, University of Tübingen, Germany
Timothy Patrick, Randolph College, United States
Copyright © 2023 Charras, Chica and Capizzi. This is an open-access article distributed under the terms of the Creative Commons Attribution License (CC BY). The use, distribution or reproduction in other forums is permitted, provided the original author(s) and the copyright owner(s) are credited and that the original publication in this journal is cited, in accordance with accepted academic practice. No use, distribution or reproduction is permitted which does not comply with these terms.
*Correspondence: Pom Charras, cG9tLmNoYXJyYXMmI3gwMDA0MDt1bml2LW1vbnRwMy5mcg==