- 1Université de Lorraine, UMR 7274 CNRS, Laboratoire Réactions et Génie des Procédés, Nancy, France
- 2CITHEFOR, Université de Lorraine, Nancy, France
The encapsulation of active ingredients is an important process in various industrial sectors including pharmaceutics, foods and cosmetics. For the first time, the capacity of non-conventional anti-Bancroft oil-in-water Pickering emulsions stabilized by partially hydrophobized silica to encapsulate an apolar active is addressed. A dispersed phase volume of paraffin oil of 50% coupled to 0.5 wt.% of silica has been employed to avoid excess of silica in the continuous phase and encapsulate higher amount of ibuprofen (the model drug). Three ibuprofen contents ranging from 100 mg (1.6 mg/mL of paraffin) to 420 mg (6 mg/mL of paraffin) have been tested. The encapsulation efficiency as well as the emulsions properties are investigated by the means of light diffusion, microscopy, rheology, and HPLC coupled to mass balance. The Pickering emulsion is very efficient for the encapsulation of ibuprofen with encapsulation rates of 99% obtained inside droplets of 30 µm for all the 3 ibuprofen concentrations. This encapsulation ability is perfectly maintained, whether during ageing (during 90 days), or when the emulsion is diluted by a factor 100 inside physiological media at basic and acidic pH.
1 Introduction
The encapsulation of active ingredients is an important process in various industrial sectors. Pharmaceutic, food, and cosmetics are some examples. To this aim, several strategies have been developed including emulsions, micelles, capsules, microparticles, lipids and liposomes (Zabot et al., 2022; Repka et al., 2023; Yan and Kim, 2024). Among them, the encapsulation of active ingredients inside droplets of emulsion appears as an interesting way in a wide variety of applications. Encapsulation of antibacterial products, essential oils, sunscreens, and drug delivery for pharmaceuticals applications are some examples. Up until now, the majority of active ingredients have been encapsulated inside classical surfactant-stabilized emulsions. The major problem with these types of emulsions is the leakage of active ingredients (Wang et al., 2020). This leakage can be produced by the coalescence of the droplets. It can be also due to micellar solubilization of the active ingredients in the presence of an excess of micelles of surfactants in the continuous phase (Rangel-Yagui et al., 2005). However, emulsions stabilized by particles, known as Pickering emulsions, are able to avoid these drawbacks.
Pickering emulsions appear then as an interesting alternative for encapsulation of active ingredients due to the absence of surfactants and, also, because of their high stability against coalescence (Harman et al., 2019; Tai et al., 2020; Sun et al., 2022). This high stability comes from the large adsorption energy of the particles at the O/W interfaces. Energies of desorption of the order of 104–105 kT are generally encountered. In comparison with classical molecular surfactant-stabilized emulsions, emulsions stabilized with particles display a lot of advantages. A large resistance to coalescence, coupled to long-term stability and dispersibility of liquid droplets, biocompatibility, tunable properties, and environmental friendliness are the most interesting characteristics of these systems. In addition, Pickering emulsions leads to encapsulation inside larger droplets which is not possible with classical surfactant-stabilized emulsions. For instance, ibuprofen has been encapsulated inside triglycerides acid in the presence of Mg(OH)2 particles (Sy et al., 2018), also inside corn oil in the presence of chitosan-alginate particles (Mao et al., 2020), and fish oil with cellulose-based nanofibrils (Li and Yu, 2023). Other active ingredients such as curcumin, caffeine, indomethacin, have been introduced inside oil droplets stabilized by various silica particles (Frelichowska et al., 2009; Simovic et al., 2010; Tikekar et al., 2013; Yaakov, et al., 2022). The previous examples highlight the high capacity of encapsulation of active ingredients with Pickering emulsions as compared with conventional systems stabilized by surfactants.
The versatility of Pickering emulsions to form O/W and W/O emulsions with the same system, i.e., oil, water and particles, has been demonstrated (Zanini et al., 2017; Ramos et al., 2023a). The versatility is mainly attributed to the roughness of the particles. In parallel, the initial wetting of the particles inside the continuous phase is also a major parameter which drives the type of emulsion (Binks and Lumsdon, 2000; Yan et al., 2001; Roques-Carmes et al., 2022). As a matter of fact, when the particles are initially pre-wetted and dispersed in water, O/W emulsions are obtained while W/O emulsions become feasible for particles initially wetted in the oily phase (Gonzalez Ortiz et al., 2020). Recently, we have demonstrated that partially hydrophobic silica, more precisely HDK 30, follows this kind of versatility (Ramos et al., 2023a). O/W and W/O emulsions were successively formulated with different oils (paraffin and dodecane) for dispersed-phase volume fractions up to 50%. In addition, these direct and reverse emulsions have a high stability and mainly against coalescence. This behavior was particularly true for the so-called non-conventional O/W emulsions stabilized by the silica. The term non-conventional indicates that the emulsion does not follow the Bancroft rule, or wetting rule, which means that hydrophobic particles with contact angle larger than 90° are theoretically unable to stabilize O/W emulsions. In that case, after pre-wetting in water, the silica particles are particularly well anchored at the O/W interfaces. We think that this kind of simple system can be useful for the encapsulation of active ingredients.
As far as the active ingredients are concerned, hydrophobic or apolar ingredients are generally encapsulated for food, cosmetic and pharmaceutical domains. Focusing more particularly to pharmaceutical applications, it can be noticed that the majority of pharmaceutical active ingredients (API) are lipophilic or hydrophobic (Ditzinger et al., 2019). However, an aqueous environment is generally encountered in the physiological environment. Consequently, the bioavailability of lipophilic API in an aqueous environment remains weak. One of the solutions to improve the bioavailability of lipophilic API is to encapsulate the API inside the oil droplets of oil-in-water (O/W) or even W/O/W double emulsions (Stauffer et al., 2019; Felix et al., 2022). Here, ibuprofen is selected as a model active ingredient. It is generally used in pharmacy but it has to be view here as a more general model for apolar constituents. No mode of application and route of administration (oral, cutaneous or intravenous administration) is really targeted in the present study. The emulsion was the same as previously discussed and is based on paraffin oil, water and HDK 30 silica. Paraffin oil and silica particles are officially approved pharmaceutical vehicles. Paraffin oil can be used in cosmetic but is less suitable for food applications. The dispersed-phase volume of paraffin oil is fixed to 50% while the silica content is maintained at 0.5 wt%. This low particle concentration should ensure good emulsion stability while avoiding excess of silica in the continuous phase. The high amount of oil aims to encapsulate a large amount of ibuprofen. Three amounts of ibuprofen are used. They range from 100 mg (1.6 mg/mL of paraffin) to 420 mg (6 mg/mL of paraffin).
2 Materials and methods
2.1 Materials
The stabilizing particles were silica HDK H30 from Wacker. They were partially hydrophobic silica particles which displayed a native particle size of the order of 20 nm (obtained from commercial data). In water, aggregates of particles of around 600 nm are detected by the means of dynamic light scattering (DLS) with a Malvern High Performance Particle Sizer (HPPS) instrument (Ramos et al., 2023b) even after the sonication step (see part 2.2). In detail, the suspensions were lightly turbid while the aggregates were not visible to the naked eyes. The hydrophobic character of this silica was demonstrated through a value of 122° of the contact angle of paraffin droplet on this silica in an aqueous environment coupled to a critical surface energy of the particles of 38 mJ/m2 (Ramos et al., 2023a). The paraffin oil used had a pharmaceutical quality (Cooper). Ibuprofen was selected as the active ingredient to be encapsulated. It was obtained from Sigma-Aldrich. The other chemicals such as methanol and hydrochloric acid were also provided from Sigma-Aldrich.
2.2 Emulsions preparation for ibuprofen encapsulation
The silica particle suspensions were prepared at 0.5 wt.% of particles content relatively to emulsion volume. A mass of 0.35 g of powder of silica particles was introduced into the aqueous phase (35 mL). The particles were then wetted and dispersed by the aqueous continuous phase with a magnetic stirrer for 48 h. This long time was compulsory because of the hydrophobic nature of the silica (Ramos et al., 2023b). The aggregates of silica were broken by using a sonic probe for 20 min (550 Fisher sonic probe). The power of the apparatus was maintained at 120 W. Alternative ultrasound pulsation was applied (2 s on/2 s off).
The ibuprofen was dissolved in paraffin oil under magnetic agitation. Three ibuprofen contents were tested and introduced in 35 mL of paraffin oil, i.e., 100 mg, 210 mg, and 420 mg which corresponded to concentrations of ibuprofen in paraffin oil of 1.6 mg/mL, 3 mg/mL, and 6 mg/mL, respectively. Note that 420 mg of ibuprofen corresponds to the solubility limit of this product inside the oil. Note that the solubility limit of ibuprofen in paraffin was experimentally evaluated. For this purpose, a given mass of ibuprofen was dissolved inside 35 mL of paraffin oil and maintained under magnetic agitation during 24 h at ambient temperature.
Oil-in-water (O/W) emulsions were formulated in batches of volume of 70 mL. The paraffin oil content was fixed to 50 vol.%. To produce the emulsion, 35 mL of paraffin oil containing ibuprofen was introduced in the aqueous silica suspension (volume of 35 mL). An equivalent length of 2 diameters of the rotor-stator stem (UltraTurrax® DI 25 Basic using the S 25 N-10G stem from IKA-Werke GmbH & CO) was immersed into the oily phase. The rotor-stator energy was applied at 13,500 rpm (corresponding to 2038 g) during 10 min.
For each ibuprofen content tested, 3 separate emulsions of a volume of 70 mL were formulated. Each analysis (size, ibuprofen content encapsulated, rheology, etc.) was performed with the 3 samples and the data reported was the average of the results of the 3 samples.
2.3 Stability of ibuprofen loaded Pickering emulsions
The emulsion stability and the encapsulation stability were followed. For the temporal stability, the emulsions were stored at ambient temperature in the absence of light. Note that none of the emulsions contained preservative or bactericide. After 30, 60, and 90 days, the emulsion was simply mixed by hands prior to the analysis (size, encapsulation ratio, rheology).
The effect of the dilution of the emulsion in different physiological media at various pH on the emulsion stability and encapsulation stability was also studied. The dilution of the emulsion and all the buffers were made at a constant ionic strength. The measurements under physiological conditions were carried at ambient temperature. For this purpose, physiological media buffers of pH of 7.4, 6.8 and 1.2 were prepared. The buffers were prepared following European Pharmacopeia recommendations. The physiological medium at pH 1.2 was based on a mixture of 0.2 M of hydrochloric acid and 0.2 M of sodium chloride. The physiological medium at pH 6.8 was prepared by mixing 0.2 M potassium dihydrogen phosphate and 0.2 M of sodium hydroxide. The physiological medium at pH 7.4 was also based on phosphate buffer. pH values of prepared solutions were controlled before and after dilution of the emulsion. For the dilution, 0.1 mL of the emulsion was mixed with 9.9 mL of the buffer solution under magnetic stirring at 400 rpm (corresponding to 9 g) during 1 h. For each ibuprofen content and physiological media, 3 separate emulsions of a volume of 70 mL were formulated.
Another test was performed mainly for rheological purpose. The emulsion was directly prepared in the buffer at pH 1.2 instead of water. The buffer physiological medium at pH 1.2 contained the silica particles. The paraffin oil content was fixed to 50%v/v and the silica to 0.5 wt%.
2.4 Methods of characterization of the prepared emulsions
Measurements of O/W interfacial tensions were made, at room temperature (20°C), with a tensiometer (Kruss) equipped with a platinum ring. The measurements were conducted for water/paraffin oil (without silica and ibuprofen), water-silica/paraffin oil (without ibuprofen), water/paraffin oil-ibuprofen (without silica), and water-silica/paraffin oil-ibuprofen. The mass of ibuprofen introduced in the paraffin oil (35 mL) was equal to 420 mg. The silica particle concentration in water was equal to 0.5 wt.% relatively to water volume.
A MasterSizer 2000® (Malvern) was utilized to measure the droplet size distribution of the emulsions. The emulsions were gently hand-mixed prior to sampling. In parallel, images of the droplets inside the emulsions were captured via a digital microscope DinoLite AM4515T8 in order to confirm and refine the droplet size distribution. In the special case of unloaded emulsion (without ibuprofen encapsulated), confocal microscopy was used to probe the arrangement of the silica particles in the emulsions. A LSM 800 confocal microscope from ZEISS International was used. In this case, the rhodamine B at 10 ppm concentration, was utilized to tag the particles. The paraffin oil was not marked with any fluorescent dye.
An ARES strain-controlled rheometer (TA Instruments) was used to probe the rheological properties of the emulsions. The tests were performed with a plate-plate geometry with a diameter of 50 mm. The gap between the plate was maintained at 1 mm. The temperature was fixed to 20°C. Prior to the analysis, the emulsion was gently hand-shaken. For the measurements, a shear rate sweep from 100 s−1 to 1 s−1 in logarithmic sweeping mode was applied to the sample. To ensure the steady state regime, each shear rate was imposed during 20 s and the shear stress measurement was averaged over the following 20 s.
2.5 Encapsulation rate of ibuprofen loaded Pickering emulsions
In order to estimate the amount of ibuprofen encapsulated and the ibuprofen content remaining in the continuous phase, a protocol was developed. To this aim, a volume of 35 mL of emulsion was used for the fresh emulsions. For the measurements after 30, 60, and 90 days of storage, 11.66 mL of the same emulsions were used instead of 35 mL. A first volume of 10 mL was measured with a 10 mL graduated pipette and the 1.66 mL were then measured with an automatic micropipette. It was necessary to separate the continuous phase from the dispersed phase without breaking the emulsion. The centrifugation process was used to produce an accelerated creaming of the droplets. An Allegra X-22 centrifuge from Beckman-Coulter was utilized. The centrifugation of the emulsions took place at 3,000 rpm (corresponding to 1,207 g) for 30 min. At the end of the centrifugation, two phases can be distinguished. On the one hand, the lightest phase contained the dispersed phase, i.e., the droplets and the ibuprofen encapsulated. On the other hand, the phase at the bottom was constituted of the continuous phase which included the remaining silica non-adsorbed onto the droplets and the ibuprofen non-encapsulated. The amount of ibuprofen in the recovered continuous phase was determined using an HPLC system (Shimadzu LC20AD) with UV/Vis detection at 220 nm on a C18 column (Hypersil BDS C18 150 × 4.6 mm; 3 µm) with a mobile phase composed of acetonitrile HPLC grade and ultrapure water (60:40, v/v, pH = 2.7, adjusted with orthophosphoric acid). Method was validated in terms of linearity and specificity from 1 μg/mL to 25 μg/mL. Samples of continuous phase were filtered through 0.45 µm PVDF filter and diluted in mobile phase before injection. The results from the measurement and calibration curve were given in terms of µg/mL. The detection limit and the quantification limit were estimated to 129 ng/mL and 148 ng/mL, respectively, using the signal-to noise approach according to ICH Q2(R2) guideline. The ibuprofen concentration was converted in mass in order to compare it with the mass of ibuprofen initially introduced in the paraffin oil. For the dispersed phase, the samples of dispersed phase were diluted in methanol prior centrifugation and dilution of supernatant in mobile phase. In details, the recovered dispersed phase was diluted inside 10 mL of methanol in order to destabilize the droplets. The mixture was stirred using a vortex during 30 s. Then, the solution was centrifuged at 10,000 rpm (corresponding to 13,416 g) for 30 min. The aim of the centrifugation was to separate the silica particles from the solution. The supernatant from the centrifugation was then diluted in mobile phase prior to enter the HPLC system for the quantification of the amount of ibuprofen. For each emulsion, a mass-balance was conducted to ensure the validity of the ibuprofen contents measured. In all the cases, the mass-balance was satisfactorily based on the experimental margin of errors. The encapsulation efficiency was calculated by the following formula detailed in Equation 1:
where m(dispersed) is the mass of ibuprofen detected in the dispersed phase and m(continuous) is the mass of ibuprofen detected in the continuous phase.
3 Results and discussion
3.1 Properties of non-loaded and ibuprofen loaded fresh emulsions
We start with the O/W emulsions in the absence of ibuprofen. Paraffin oil/water interfacial tensions in the presence and absence of particles are similar, i.e., around 33 mN/m and 34 mN/m (Table 1). This does not mean that the particles do not adsorb at the paraffin/water interface since many studies highlight the absence of variation of the O/W interfacial tensions in the presence of adsorbed particles at the surfaces of the droplets (Fernandez-Rodriguez et al., 2015; Powell and Chauhan, 2016; Zhang et al., 2017; Dekker et al., 2023; Velandia et al., 2025). Non-conventional direct O/W emulsions are successively formulated with a phase content of 50 %v/v. An average droplet diameter of 40 µm is obtained (Figure 1A). The confocal microscopic image of the emulsion emphasizes that the silica particles adsorb at the paraffin/water interfaces (Figure 1B). In addition, no visible silica particles can be detected in the continuous phase. This seems to indicate that only few silica particles are in the continuous phase and that the majority of silica is situated at the O/W interfaces. This corresponds well with our previous finding where we measured that around 80% of the silica is at the interface (Ramos et al., 2021).

Table 1. Paraffin oil/water interfacial tension in the presence of silica particles in the water (“Silica aqueous suspension”) and ibuprofen in the paraffin oil (“Paraffin oil + Ibuprofen”).
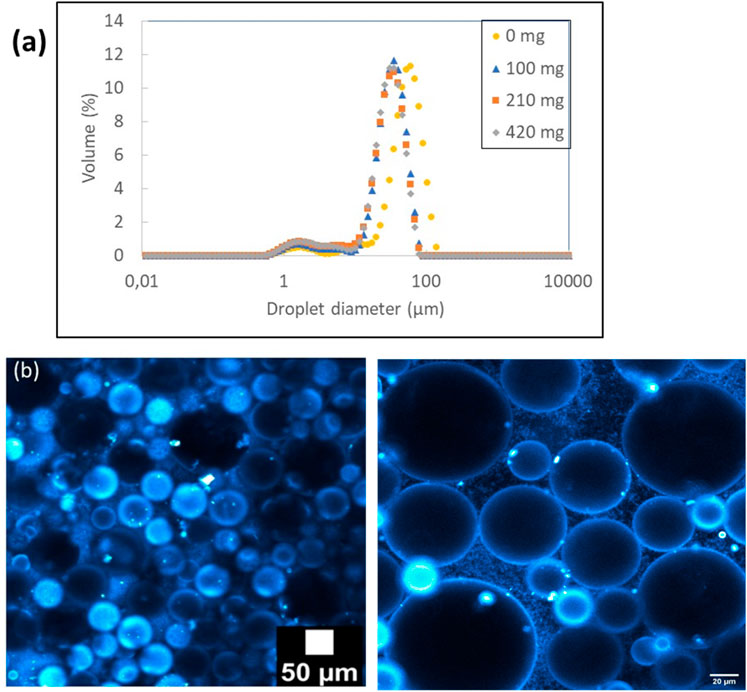
Figure 1. (A) Droplet size distribution of O/W emulsions with various amounts of ibuprofen. (B) Confocal fluorescence images of emulsion prepared in absence of ibuprofen. Silica particles appear bluish.
In the presence of ibuprofen, the O/W interfacial tension decreases from 35 mN/m (absence of ibuprofen) to 25 mN/m in the presence of ibuprofen (Table 1). Two approaches can explain this diminution. First, a possible interfacial activity of ibuprofen can be considered. In that case, the ibuprofen cannot be seen as a surfactant since lower interfacial tensions are expected with surfactants (Roques-Carmes et al., 2009; Biswal and Singh, 2016; Akhlaghi et al., 2021; Jiang et al., 2021). Second, the change of the dielectric constant of paraffin in the presence of ibuprofen could take place. This change of dielectric constant was also observed for water in the presence of K-carrageenan (KC) leading to a decrease in the indopol/water-KC interfacial tension (Jaber et al., 2022). It is then considered that the drop of interfacial tension is not due to the interfacial activity of ibuprofen but to the change of dielectric constant of the paraffin oil in the presence of ibuprofen. The droplet size is reduced in the presence of ibuprofen (Figure 1A). The average droplets diameter decreases from 40 µm (absence of ibuprofen) to 30 µm in the presence of ibuprofen. The reduction of the O/W interfacial tension in the presence of ibuprofen explains this diminution of the droplets size. Actually, the droplets size diminishes as the interfacial tension decreases if the stirring energy remains constant which is the case here. The droplets size seems not significantly impacted by the amount of ibuprofen loaded.
Figure 2 displays the rheological properties (viscosity vs shear rate) of the fresh emulsions without and with ibuprofen at different contents. Unloaded and ibuprofen-loaded emulsions exhibit non-Newtonian shear-thinning behaviors as expected for emulsions (Derkach, 2009). In other words, the viscosity decreases with the shear rate for all the emulsions. In the absence of ibuprofen, the viscosity is slightly larger than for the emulsions containing ibuprofen. Viscosity of ibuprofen-loaded emulsions seems to follow the following order η (0 mg) > η (100 mg) > η (210 mg) > η (420 mg). However, the difference between the curves is relatively low.
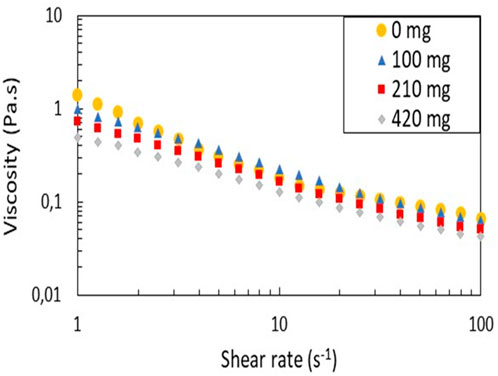
Figure 2. Flow behavior of fresh unloaded and ibuprofen-loaded emulsions with various amounts of ibuprofen.
3.2 Ibuprofen encapsulation inside fresh emulsions
It is relevant to measure the amount of ibuprofen encapsulated and the ibuprofen content remaining in the continuous phase. The results are reported in Table 2. The results are expressed in terms of masses of ibuprofen in order to compare them with the mass of ibuprofen initially introduced in the paraffin. The masses of ibuprofen measured in the dispersed phase are 52 mg, 109 mg, and 230 mg for the theoretically introduced masses of 50 mg, 105 mg, and 210 mg, respectively. The masses of ibuprofen encapsulated are close to those initially introduced. In parallel, the masses of ibuprofen remaining in the continuous phase are low since they are lower than or equal to 1 mg. It is interesting to note that for all the samples, the mass-balance is satisfactorily based on the experimental margin of errors. From these data, it can be concluded that encapsulation ratios of ibuprofen larger than or equal to 99% are obtained regardless of the introduced ibuprofen content. In addition, a very small amount of non-encapsulated ibuprofen remains in the continuous phase. The partition of the ibuprofen between the droplets and the continuous phase is in favor of the droplets. All the ibuprofen is inside the droplets. The distribution is less marked with surfactants-stabilized emulsions (Repka et al., 2023).
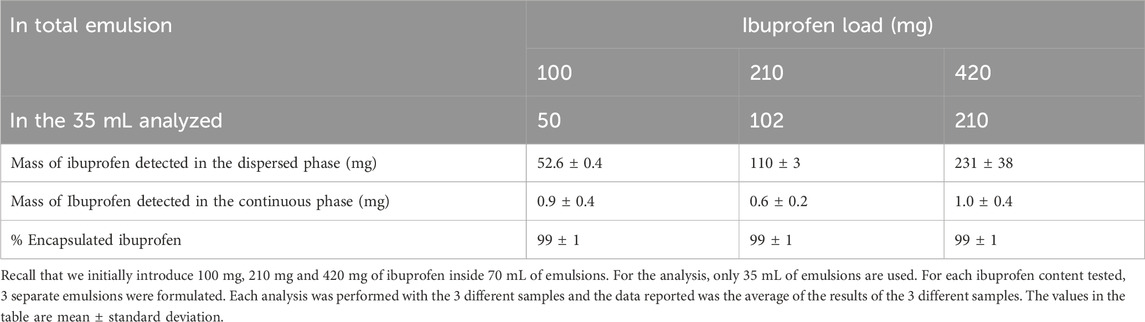
Table 2. Repartition of ibuprofen between the dispersed and continuous phases of fresh O/W emulsions loaded with 100, 210 or 420 mg of ibuprofen.
3.3 Long-term stability and encapsulation efficiency of ibuprofen loaded emulsions
The long-term stability is assessed here in terms of emulsion stability, amount of ibuprofen encapsulated and also rheology properties, during 30, 60, and 90 days. The emulsions were stored at room temperature in a cupboard away from light. The temporal variation of the average diameter of the droplets of the emulsion loaded with various contents of ibuprofen is reproduced in Figure 3. The evolution of droplet size distribution of Pickering emulsions loaded with 100 mg and 420 mg of ibuprofen through 0, 30 or 60 days are also depicted in Figure 4. For each initial ibuprofen content, the average droplets size does not evolve during 90 days. In addition, the absence of oil leakage during this period confirms the absence of coalescence and highlights the stability of the emulsions. This stability occurs for all the emulsions regardless of the ibuprofen content. It is also interesting to note the absence of visible fungus and bacteria inside the emulsions after 90 days despite the absence of preservative and bactericide.
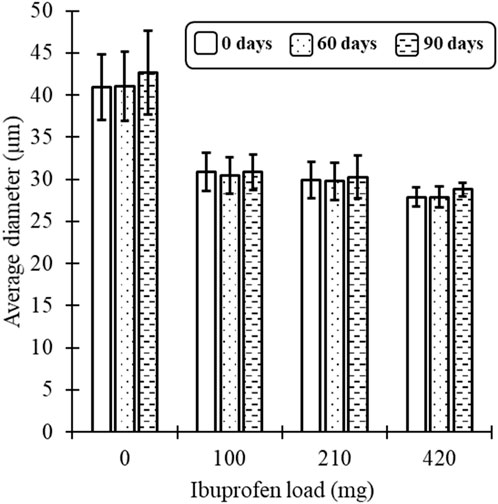
Figure 3. Evolution of average droplet size of emulsions with various amounts of ibuprofen through 0, 60 or 90 days. Results are presented as mean of 3 independent emulsions.
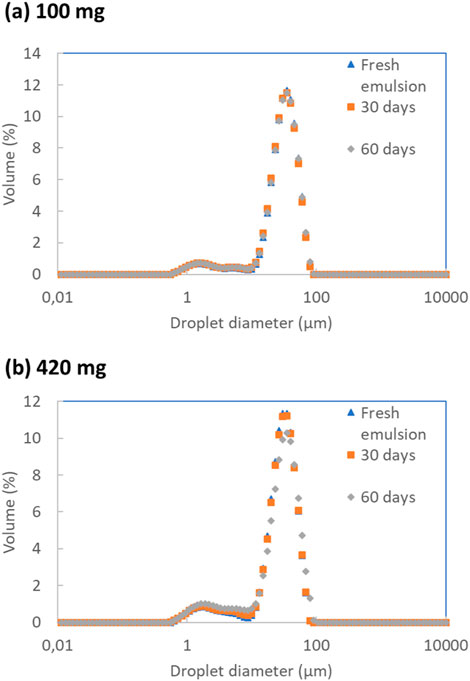
Figure 4. Evolution of droplet size distribution of Pickering emulsions loaded with (A) 100 mg and (B) 420 mg of ibuprofen through 0, 30 or 60 days.
The evolution of the viscosity is evaluated during 60 days (fresh emulsion, emulsions after 30 days and 60 days). The results are depicted in Figure 5. The flow curves (viscosity vs shear rate) depend on the amount of ibuprofen encapsulated inside the droplets. In the absence of ibuprofen, the flow curves do not significantly vary with time. In details, the flow curve slightly decreases after 30 days of storage. On the opposite, in the presence of ibuprofen, a significant decrease of the viscosity and flow curve take place after 30 days as compared to the fresh emulsion. The viscosities after 30 days and 60 days remain similar. For the largest amount of ibuprofen (420 mg), a regular diminution of the viscosity and flow curve can be observed between the fresh emulsion and after 30 and 60 days. Looking carefully at all the curves, it appears that the difference in viscosity with time is significant at low shear rates (1–10 s−1) while the viscosities are equal at high shear rates of 100 s−1. This result was expected since at high shear rates, the viscosity of dispersed systems such as suspensions, dispersions and emulsions, tend towards the viscosity of the continuous phase (Derkach, 2009). In other words, the viscosity values do not depend on the organization of the droplets inside the emulsion. Looking deeply at the viscosities at low shear rate around 1 s−1 gives more information about the structuration and organization of the droplets inside the emulsion. After 30 days, the substantial reduction of the viscosity emphasizes that the structuration of the droplets is reduced. This phenomenon occurs mainly in the presence of ibuprofen. Actually, the diminution of the viscosity cannot be attributed to change in droplet size (Figures 3, 4). It can be probably explained by the rearrangement inside the droplets and at the interfaces with time. As a hypothesis, the modification of particle organization and wettability at the interface could take place with time. The reorganization of silica due to the presence of ibuprofen occurs with time. It can be also concluded that the emulsions just after preparation are not at equilibrium and evolve with time. For these kinds of systems, the batch produced cannot be release for quality analysis via rheology measurements just after preparation.
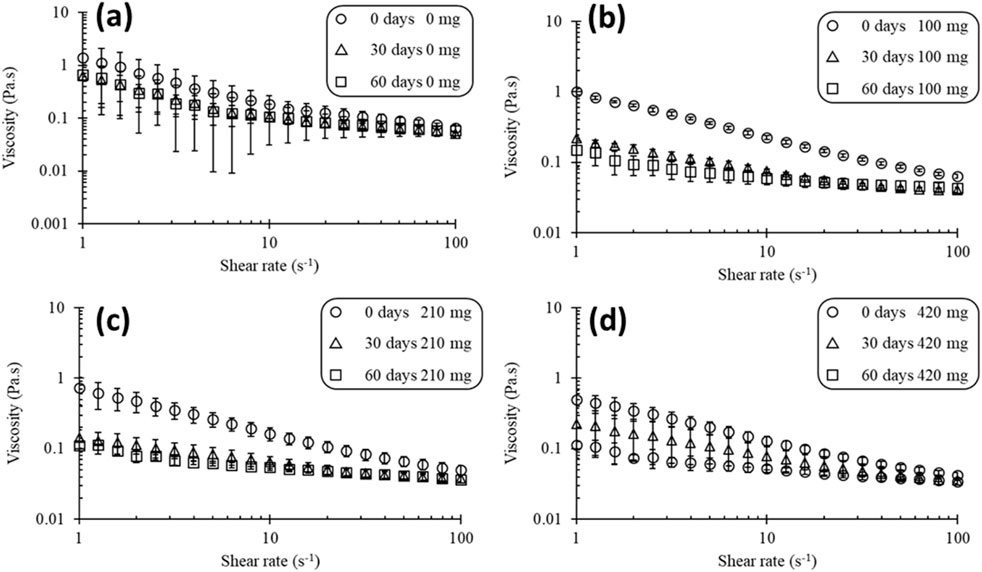
Figure 5. Flow behavior of fresh (“0 days”) and 30 or 60 days old (A) unloaded and (B) 100 mg, (C) 210 mg or (D) 420 mg ibuprofen-loaded Pickering emulsions. Results are presented as mean of 3 independent emulsions.
The amount of ibuprofen encapsulated as well as the partition between the droplets and the continuous phase are also monitored during 60 days. The data are displayed in Table 3. For each ibuprofen concentration, the mass of ibuprofen in the dispersed phase decreases from 17 to 14 mg, from 36 to 31 mg, and from 76 to 74 mg after 30 and 60 days. The difference is low and seems due to experimental uncertainties rather than a real release of the ibuprofen. This is confirmed by the slight increase of the mass of ibuprofen remaining in the continuous phase which does not correspond to a real release of the active ingredient. After 30 and 60 days, the percentage of ibuprofen encapsulated remains similar to that initially measured just after preparation (“0 Day”). In other words, after 30 and 60 days, the rate of encapsulation still remains over 99%. These results remain valid for all the ibuprofen concentrations studied here. This confirms unambiguously that the ibuprofen remains encapsulated since the ratio of ibuprofen does not change during the storage during 60 days. The lipophilic behavior of the ibuprofen coupled to the absence of droplets coalescence hinder its diffusion towards the aqueous phase. Ibuprofen is a small molecule. If a space between interfacial particles exists, i.e., a hole in the particle’s physical interfacial barrier, the active ingredient such as ibuprofen can theoretically cross the interface by the means of passive diffusion. For this to occur, it is necessary that: 1) the space between particles actually exist at the particle-laden interface and 2) the absence of supplementary obstacle or barrier created by electrostatic repulsions due to the particle’s charges. The confocal pictures displayed in Figure 1B seems to highlight a full coverage of the interface by the particles with one or more adsorbed layer. The value of logP has also to be considered. The value of logP in our system is equal to 3.51 confirming the low aqueous solubility of ibuprofen. This indicates that only a small amount of ibuprofen will be able to go through the aqueous phase. Even in the presence of a high gradient of concentration, the amount of ibuprofen in water will be relatively low. It is also interesting to note that recent studies showed and postulated that for Pickering emulsions, a stable barrier around emulsions droplets protect encapsulated materials (Haji et al., 2022). In the same spirit, it is evidenced that a dense and rigid solid particles-based network was observed to partition to the interface of the droplets in the Pickering emulsions. In addition, in the present study, we used original non-conventional particles in a sense of « anti-Bancroft ». These non-conventional particles could also create a second lipophilic barrier due to the hydrophobic character of the particles used. This adds a physico-chemical barrier in addition to the physical-barrier due to the solid nature of the particles. Finally, droplets coalescence is also a major cause of the leakage of active ingredients. The coalescence is hindered due to the presence of the particles. This limits even more the possibility of diffusion of ibuprofen inside the water.
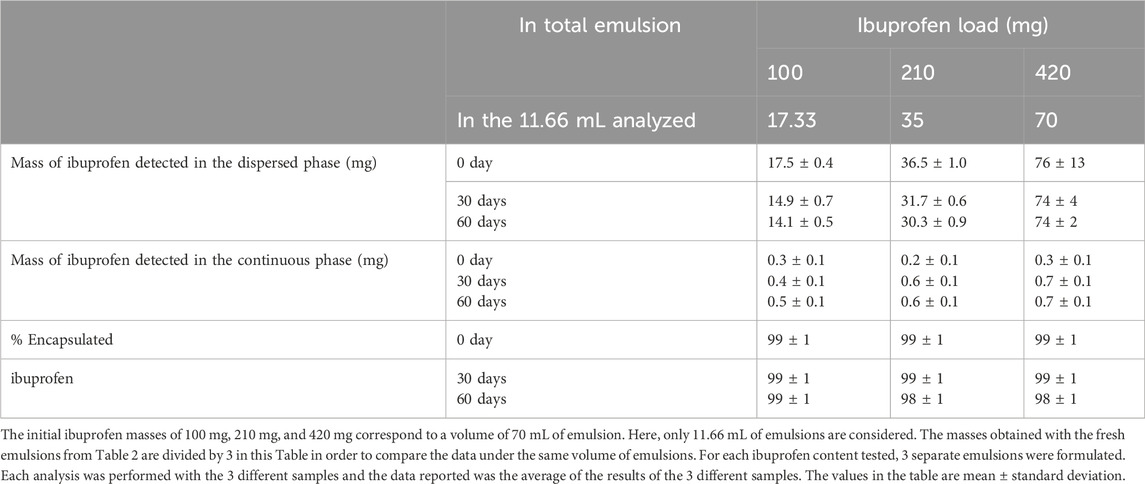
Table 3. Repartition of ibuprofen between the dispersed and continuous phases of fresh emulsions (“Day 0”) and after 30, and 60 days of storage loaded with 100, 210 or 420 mg of ibuprofen.
3.4 Behavior of ibuprofen loaded emulsions diluted in different physiological media
In this part, the effect of the dilution of the emulsion in different physiological media at various pH on the emulsion stability and encapsulation stability is discussed. We start with emulsions diluted 100 times in water, and buffers physiological media of pH of 7.4, 6.8 or 1.2. The pH of the ibuprofen loaded Pickering emulsions, containing initially 420 mg of Ibuprofen, diluted 100 times in distilled water or in a physiological media buffer were measured. The pH of water decreases from 5.56 to 4.51 when adding the ibuprofen loaded Pickering emulsion. Conversely, the pH values do not evolve after dilution in physiological media buffer. The final pH after dilution correspond, respectively, to pH = 1.44 ± 0.19, pH = 7.30 ± 0.01, and pH = 6.88 ± 0.08. The same pH were recorded for the 3 ibuprofen contents.
Figure 6 depicts the microscopic images and the droplet size distribution of the emulsions loaded with 100 mg, 210 mg or 420 mg of ibuprofen and diluted 100 times in water or in a buffer physiological media at different pH. For each ibuprofen content, the droplet size distributions are similar regardless of the dilution media. More interestingly, the droplet size distributions correspond well to that obtained with the fresh non-diluted emulsions (Figure 1A). Exposing ibuprofen loaded Pickering emulsions to physiological media with different pH do not impact their average droplet size. The average droplet size of ibuprofen loaded Pickering emulsions is not only unchangeable through time but also when exposed to the physiological media at different pH. This highlights the stability of the emulsion toward dilution in water or physiological media. This confirms that the silica particles do not desorb under dilution or change of pH.
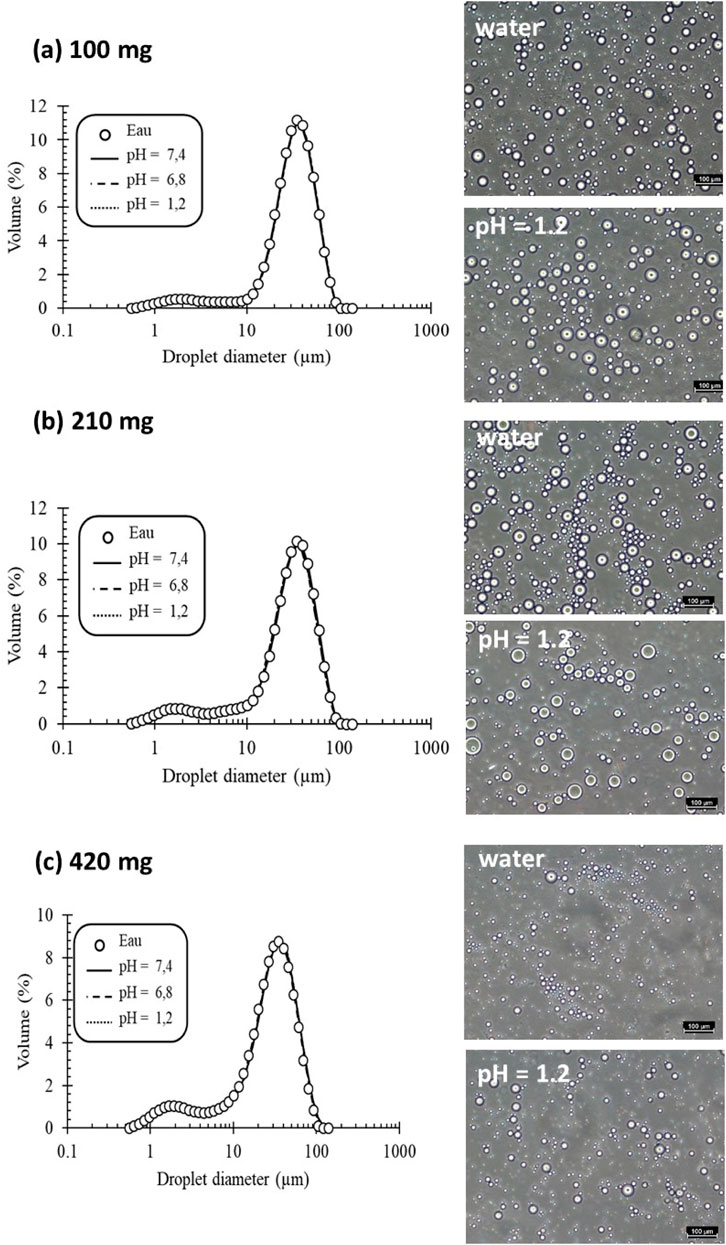
Figure 6. (Left) Droplet size distribution of Pickering emulsions diluted 100 times in water (“Eau”) or in a physiological buffer media (pH = 7.4, pH = 6.8, pH = 1.2). (Right) Microscopic images of each emulsion diluted in water or in physiological buffer media at acidic pH of 1.2. Similar pictures are obtained with the physiological buffer media at the other pH (6.8 and 7.4). The emulsions are loaded with (A) 100 mg, (B) 210 mg or (C) 420 mg of ibuprofen.
In the following, the physiological medium at pH 1.2 was selected in order to test the stability of the encapsulation properties of the emulsions under severe conditions. The water is also used as a reference. The content of ibuprofen encapsulated and the partition between the droplets and the continuous phase are followed after dilution in water and physiological media at pH 1.2 during 1 h. The data are displayed in Table 4.
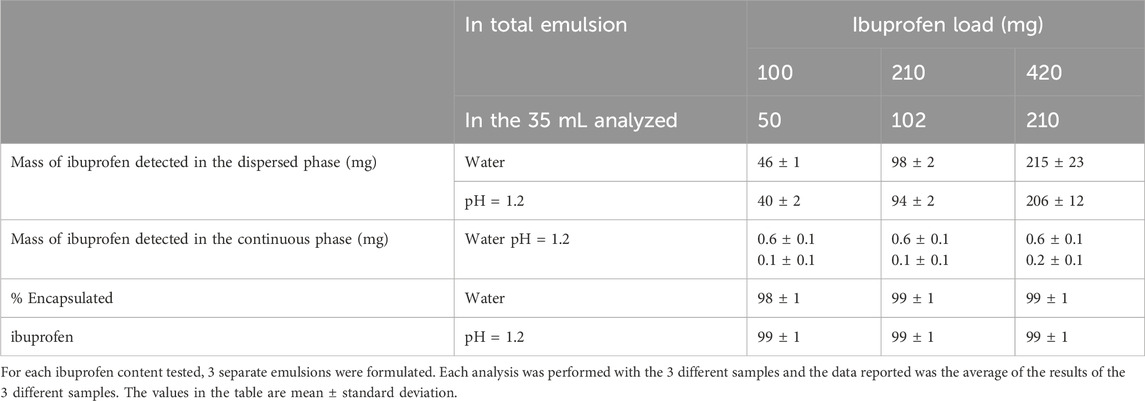
Table 4. Repartition of ibuprofen between the dispersed and continuous phases of Pickering emulsions diluted 100 times in water (“Water”) and in a pH buffer physiological media at pH 1.2 (“pH = 1.2”).
The amount of ibuprofen encapsulated, i.e., the percentage of ibuprofen encapsulated, appears rather close to that obtained with the fresh non-diluted emulsions (Table 2). This is true for the acidic physiological media and water. The rate of encapsulation still remains over 98%. In parallel, the remaining ibuprofen content detected in the continuous phase appears weak and smaller than 1 mg. These results remain valid for the physiological media and water. This confirms unambiguously that the ibuprofen remains encapsulated. This means that the pH or the dilution are not a source of instability or release of ibuprofen. The fact that the rate of encapsulation remains high and constant after 1 h of dilution under stirring in the physiological media and water indicates the absence of early “burst” release which are sometimes encountered with Pickering emulsions due to the porosity and structure of the particles. The absence of release can be explained by the remarkable stability of this non-conventional O/W Pickering emulsions stabilized by partially hydrophobic silica. According to the structure of ibuprofen (pKa = 4.9), the presence of the carboxylic group may add two problems related to the pH: the possible dimer formation through H-bonds between protonated carboxylic group, COOH, and a change in the partition behavior due to the ionization of this group. According to the results, both events seem not to take place here. It is interesting to note that for classical surfactant-stabilized emulsions the behavior is different. The dilution of the emulsion can produce a modification of the repartition of the surfactants and the active ingredient toward the continuous phase. Actually, the surfactant could desorb (Geffroy et al., 2000; He et al., 2015; Sharipova et al., 2017) and favors the emulsion instability and active ingredient release.
The same dilution experiments were performed with emulsions after 30 days and 60 days (data not shown). Similarly to that obtain with the fresh emulsions, the dilution in the physiological media at different pH does not modify the droplets average size, the droplet size distribution, and the encapsulation efficiency. This confirms the high stability of the emulsion and the encapsulation during dilution and in physiological media at different pH even for aged emulsions.
It was not relevant to perform rheology experiments with the diluted emulsions because the data cannot be directly compared to those of the fresh non-diluted emulsions since the viscosity of an emulsion depends significantly on the dispersed phase volume fraction (Derkach, 2009; Velandia et al., 2021). To tackle this issue and have a constant dispersed phase volume while varying the pH, the emulsions were directly prepared with the buffer solution which contained the silica particles. It was decided to only prepare one type of emulsion for which the continuous phase was constituted of the buffer physiological medium at pH 1.2. The rheograms (viscosity as a function of the shear rate) of the unloaded emulsion and emulsions loaded with 100 mg, 210 mg, and 420 mg are reported in Figure 7 (“Buffer pH 1.2”). The data obtained with the same emulsions prepared with water are also inserted in the figure for sake of comparison (“Classical Emulsion”).
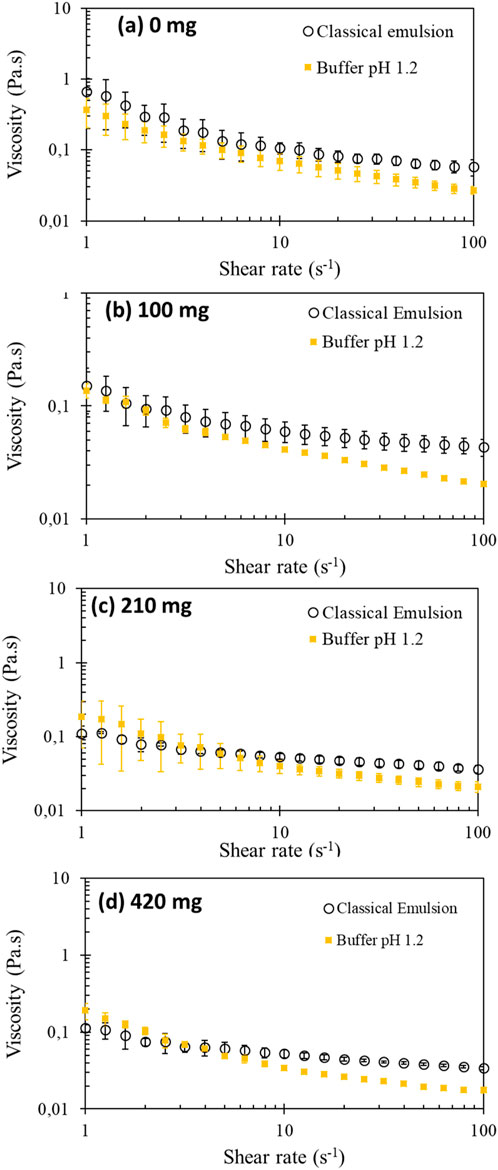
Figure 7. Comparison of flow behavior of the emulsions prepared with the particles dispersed in water (“Classical Emulsion”) and those directly prepared with the buffer solution at pH 1.2 (“Buffer pH 1.2,” particles dispersed in the buffer physiological medium at pH 1.2). The emulsions are loaded with (A) 0 mg, (B) 100 mg, (C) 210 mg or (D) 420 mg of ibuprofen. Results are presented as mean of 3 independent emulsions.
The trend of the flow curves (viscosity vs shear rate) of the classical emulsions and those prepared in a buffer medium at acidic pH are similar. The values of the viscosity are quite close at low shear rates while a larger difference occurs at high shear rates. Since the viscosity at high shear rates is related to the viscosity of the continuous phase, it can be observed that the acidic buffered continuous phase is less viscous and more fluidic than the water continuous phase. In addition, the absence of significant difference of viscosity at small shear rates indicates that the structuration and organization of the droplets inside the emulsion is not affected by the acidic buffer.
4 Conclusion
For the first time, non-conventional anti-Bancroft O/W Pickering emulsions were used as platform to encapsulate an apolar active ingredient. A high internal dispersed-phase volume of 50% of paraffin oil was employed in the presence of 0.5 wt% of partially hydrophobized silica particles. The weak silica concentration allowed to avoid excess of particles inside the continuous phase. The larger amount of dispersed phase aimed to encapsulate high amounts of ibuprofen used as model active ingredients. Three amounts of hydrophobic ibuprofen ranging from 100 mg (1.6 mg/mL of paraffin) to 420 mg (6 mg/mL of paraffin) have been studied.
The confocal images indicated that the majority of silica was adsorbed at the interface of the droplets and that only a weak amount remained in the continuous phase. In the presence of ibuprofen, the O/W interfacial tension decreased to 25 mN/m and produced concentrated emulsions of droplets of diameter of 30 µm lower than in absence of ibuprofen (40 µm). High ibuprofen loadings were obtained with encapsulation rates of 99%. The ibuprofen concentration had a slight impact on the viscosity of the emulsions.
The emulsions remained stable during 90 days. The encapsulation of increasing dose of ibuprofen had no impact on the emulsion stability. During this period, the ibuprofen remained encapsulated inside the droplets. The flow curves indicated that the emulsions became more fluid with time due to a change of the structure and organization of the droplets with time. The effect of the dilution of the emulsion in different physiological media was also evaluated. No variation of the droplet size distribution could be detected for all the pH and media. The size of the droplets was similar to that of the non-diluted emulsions. The emulsions displayed high stability toward dilution and pH. The ibuprofen remained encapsulated after 1 h of dilution under stirring indicating the absence of early burst release of the active ingredient.
Future studies will aim to demonstrate that the same system can be used with water/paraffin oil/silica reverse emulsions in order to encapsulate polar active ingredients such as insulin. This will pave the way for a platform for encapsulation based on paraffin oil, water and partially hydrophobic silica. This will be possible due to the versatility of this silica for the formulation of direct O/W and reverse O/W emulsions.
Data availability statement
The raw data supporting the conclusions of this article will be made available by the authors, without undue reservation.
Author contributions
DR: Conceptualization, Formal Analysis, Investigation, Methodology, Validation, Writing–original draft. AS-M: Data curation, Funding acquisition, Project administration, Resources, Supervision, Validation, Writing–review and editing. PM: Data curation, Formal Analysis, Methodology, Writing–review and editing. MP: Data curation, Methodology, Writing–review and editing. VS: Project administration, Supervision, Writing–review and editing. TR-C: Conceptualization, Data curation, Formal Analysis, Investigation, Methodology, Project administration, Supervision, Validation, Visualization, Writing–original draft, Writing–review and editing.
Funding
The author(s) declare that no financial support was received for the research, authorship, and/or publication of this article.
Acknowledgments
We would like to thank Frédérick Niepceron and Lazhar Benyahia [Institut des Molécules et Matériaux du Mans (IMMM), Le Mans Université] for their help during the confocal microscopy measurements. The authors thank Thomas Chaigneau (Université de Lorraine, CITHEFOR) for his help during the HPLC measurements.
Conflict of interest
The authors declare that the research was conducted in the absence of any commercial or financial relationships that could be construed as a potential conflict of interest.
The author(s) declared that they were an editorial board member of Frontiers, at the time of submission. This had no impact on the peer review process and the final decision.
Publisher’s note
All claims expressed in this article are solely those of the authors and do not necessarily represent those of their affiliated organizations, or those of the publisher, the editors and the reviewers. Any product that may be evaluated in this article, or claim that may be made by its manufacturer, is not guaranteed or endorsed by the publisher.
References
Akhlaghi, N., Riahi, S., and Parvaneh, R. (2021). Interfacial tension behavior of a nonionic surfactant in oil/water system; salinity, pH, temperature, and ionic strength effects. J. Petroleum Sci. Eng. 198, 108177. doi:10.1016/j.petrol.2020.108177
Binks, B. P., and Lumsdon, S. O. (2000). Influence of particle wettability on the type and stability of surfactant-free emulsions. Langmuir 16, 8622–8631. doi:10.1021/la000189s
Biswal, N. R., and Singh, J. K. (2016). Interfacial behavior of nonionic Tween 20 surfactant at oil–water interfaces in the presence of different types of nanoparticles. RSC Adv. 6, 113307–113314. doi:10.1039/C6RA23093H
Dekker, R. I., Velandia, S. F., Kibbelaar, H. V. M., Morcy, A., Sadtler, V., Roques-Carmes, T., et al. (2023). Is there a difference between surfactant-stabilised and Pickering emulsions? Soft Matter 19, 1941–1951. doi:10.1039/D2SM01375D
Derkach, S. R. (2009). Rheology of emulsions. Adv. Colloid Interface Sci. 151, 1–23. doi:10.1016/j.cis.2009.07.001
Ditzinger, F., Price, D. J., Ilie, A.-R., Kohl, N. J., Jankovic, S., Tsakiridou, G., et al. (2019). Lipophilicity and hydrophobicity considerations in bio-enabling oral formulations approaches – a PEARRL review. J. Pharm. Pharmacol. 71, 464–482. doi:10.1111/jphp.12984
Felix, M., Guerrero, A., and Carrera-Sánchez, C. (2022). Optimization of multiple W1/O/W2 emulsions processing for suitable stability and encapsulation efficiency. Foods 11, 1367. doi:10.3390/foods11091367
Fernandez-Rodriguez, M. A., Ramos, J., Isa, L., Rodriguez-Valverde, M. A., Cabrerizo-Vilchez, M. A., and Hidalgo-Alvarez, R. (2015). Interfacial activity and contact angle of homogeneous, functionalized, and Janus nanoparticles at the water/decane interface. Langmuir 31 (32), 8818–8823. doi:10.1021/acs.langmuir.5b02137
Frelichowska, J., Bolzinger, M. A., Pelletier, J., Valour, J. P., and Chevalier, Y. (2009). Topical delivery of lipophilic drugs from o/w Pickering emulsions. Int. J. Pharm. 371, 56–63. doi:10.1016/j.ijpharm.2008.12.017
Geffroy, C., Cohen Stuart, M. A., Wong, K., Cabane, B., and Bergeron, V. (2000). Adsorption of nonionic surfactants onto polystyrene: kinetics and reversibility. Langmuir 16, 6422–6430. doi:10.1021/la0000769
Gonzalez Ortiz, D., Pochat-Bohatier, C., Cambedouzou, J., Bechelany, M., and Miele, P. (2020). Current trends in Pickering emulsions: particle morphology and applications. Engineering 6, 468–482. doi:10.1016/j.eng.2019.08.017
Haji, F., Cheon, J., Baek, J., Wang, Q., and Tam, K. C. (2022). Application of Pickering emulsions in probiotic encapsulation- A review. Curr. Res. Food Sci. 5, 1603–1615. doi:10.1016/j.crfs.2022.09.013
Harman, C. L. G., Patel, M. A., Guldin, S., and Davies, G. L. (2019). Recent developments in Pickering emulsions for biomedical applications. Curr. Opin. Colloid & Interface Sci. 39, 173–189. doi:10.1016/j.cocis.2019.01.017
He, Y., Yazhgur, P., Salonen, A., and Langevin, D. (2015). Adsorption-desorption kinetics of surfactants at liquid surfaces. Adv. Colloid Interface Sci. 222, 377–384. doi:10.1016/j.cis.2014.09.002
Jaber, A., Roques-Carmes, T., Marchal, P., Hamieh, T., and Benyahia, L. (2022). Interfacial viscoelastic moduli in a weak gel. J. Colloid Interface Sci. 622, 126–134. doi:10.1016/j.jcis.2022.04.047
Jiang, X., Liu, M., Li, X., Wang, L., Liang, S., and Guo, X. (2021). Effects of surfactant and hydrophobic nanoparticles on the crude oil-water interfacial tension. Energies 14, 6234. doi:10.3390/en14196234
Li, Z., and Yu, D. (2023). Controlled ibuprofen release from Pickering emulsions stabilized by pH-responsive cellulose-based nanofibrils. Int. J. Biol. Macromol. 242, 124942. doi:10.1016/j.ijbiomac.2023.124942
Mao, Q., Li, M., Zhang, S., Zhang, X., He, G., and Zhang, W. (2020). Chitosan-hydrophobic alginate nanocomposites stabilized pH-triggered Pickering emulsion for drug controlled-release. Int. J. Biol. Macromol. 162, 1888–1896. doi:10.1016/j.ijbiomac.2020.08.092
Powell, K. C., and Chauhan, A. (2016). Dynamic interfacial tension and dilational rheology of dispersant Corexit 9500. Colloids Surfaces A Physicochem. Eng. Aspects 497, 352–361. doi:10.1016/j.colsurfa.2016.03.009
Ramos, D. M., Sadtler, V., Marchal, P., Lemaitre, C., Benyahia, L., and Roques-Carmes, T. (2021). Insight into the emulsification process effect on particles distribution in Pickering emulsions: a series of rheological and gravimetric tests. Chem. Eng. Trans. 86, 1291–1296. doi:10.3303/CET2186216
Ramos, D. M., Sadtler, V., Marchal, P., Lemaitre, C., Benyahia, L., and Roques-Carmes, T. (2023b). Properties of non-conventional direct O/W Pickering emulsions stabilized by partially hydrophobic silica particles controlled by rotor-stator or ultrasonic emulsification. Colloids Surfaces A Physicochem. Eng. Aspects 673, 131782. doi:10.1016/j.colsurfa.2023.131782
Ramos, D. M., Sadtler, V., Marchal, P., Lemaitre, C., Niepceron, F., Benyahia, L., et al. (2023a). Particles’ organization in direct oil-in-water and reverse water-in-oil Pickering emulsions. Nanomaterials 13, 371. doi:10.3390/nano13030371
Rangel-Yagui, C. O., Pessoa, A., and Tavares, L. C. (2005). Micellar solubilization of drugs. J. Pharm. Pharm. Sci. 8, 147–165.
Repka, D., Kurillová, A., Murtaja, Y., and Lapcík, L. Y. (2023). Application of physical-chemical approaches for encapsulation of active substances in pharmaceutical and food industries. Foods 12, 2189. doi:10.3390/foods12112189
Roques-Carmes, T., Gigante, A., Commenge, J.-M., and Corbel, S. (2009). Use of surfactants to reduce the driving voltage of switchable optical elements based on electrowetting. Langmuir 25, 12771–12779. doi:10.1021/la900882h
Roques-Carmes, T., Velandia, S. F., Ramos, D., Lemaître, C., Sadtler, V., and Marchal, P. (2022). “Chemical product design methodology applied to Pickering emulsions,” in Advances in chemistry research. Editor J. C. Taylor (New York, NY: Nova Science Publishers, Inc.), Vol. 73, 221–240.
Sharipova, A. A., Aidarova, S. B., Mutaliyeva, B. Z., Babayev, A. A., Issakhov, M., Issayeva, A. B., et al. (2017). The use of polymer and surfactants for the microencapsulation and emulsion stabilization. Colloids Interfaces 1 (1), 3. doi:10.3390/colloids1010003
Simovic, S., Hui, H., Song, Y., Davey, A. K., Rades, T., and Prestidge, C. A. (2010). An oral delivery system for indomethicin engineered from cationic lipid emulsions and silica nanoparticles. J. Control. Release 143, 367–373. doi:10.1016/j.jconrel.2010.01.008
Stauffer, F., Peter, B., Alem, H., Funfschilling, D., Dumas, N., Serra, C., et al. (2019). Polyelectrolytes layer-by-layer surface modification of PDMS microchips for the production of simple O/W and double W/O/W emulsions: from global to localized treatment. Chem. Eng. Process. - Process Intensif. 146, 107685. doi:10.1016/j.cep.2019.107685
Sun, Z., Yan, X., Xiao, Y., Hu, L., Eggersdorfer, M., Chen, D., et al. (2022). Pickering emulsions stabilized by colloidal surfactants: role of solid particles. Particuology 64, 153–163. doi:10.1016/j.partic.2021.06.004
Sy, P. M., Anton, N., Idoux-Gillet, Y., Dieng, S. M., Messaddeq, N., Ennahar, S., et al. (2018). Pickering nano-emulsion as a nanocarrier for pH-triggered drug release. Int. J. Pharm. 549, 299–305. doi:10.1016/j.ijpharm.2018.07.066
Tai, Z., Huang, Y., Zhu, Q., Wu, W., Yi, T., Chen, Z., et al. (2020). Utility of Pickering emulsions in improved oral drug delivery. Drug Discov. Today 25, 2038–2045. doi:10.1016/j.drudis.2020.09.012
Tikekar, R. V., Pan, Y., and Nitin, N. (2013). Fate of curcumin encapsulated in silica nanoparticle stabilized Pickering emulsion during storage and simulated digestion. Food Res. Int. 51, 370–377. doi:10.1016/j.foodres.2012.12.027
Velandia, S. F., Marchal, P., Lemaitre, C., Sadtler, V., and Roques-Carmes, T. (2021). Evaluation of the repartition of the particles in Pickering emulsions in relation with their rheological properties. J. Colloid Interface Sci. 589, 286–297. doi:10.1016/j.jcis.2021.01.005
Velandia, S. F., Marchal, P., Sadtler, V., Arnoux, P., Bonn, D., and Roques-Carmes, T. (2025). Globular proteins as Pickering emulsion stabilizers: particles or surfactants? Colloids Surfaces A Physicochem. Eng. Aspects 704, 135469. doi:10.1016/j.colsurfa.2024.135469
Wang, X., Collot, M., Omran, Z., Vandamme, T. F., Klymchenko, A., and Anton, N. (2020). Further insights into release mechanisms from nano-emulsions assessed by a simple fluorescence-based method. J. Colloid Interface Sci. 578, 768–778. doi:10.1016/j.jcis.2020.06.028
Yaakov, N., Kottakota, C., Mani, K. A., Naftali, S. M., Zelinger, E., Davidovitz, M., et al. (2022). Encapsulation of Bacillus thuringiensis in an inverse Pickering emulsion for pest control applications. Colloids Surfaces B Biointerfaces 213, 112427. doi:10.1016/j.colsurfb.2022.112427
Yan, C., and Kim, S. R. (2024). Microencapsulation for pharmaceutical applications: a Review. ACS Appl. Bio Mater. 7, 692–710. doi:10.1021/acsabm.3c00776
Yan, N., Gray, M. R., and Masliyah, J. H. (2001). On water-in-oil emulsions stabilized by fine solids. Colloids Surfaces A Physicochem. Eng. Aspects 193, 97–107. doi:10.1016/S0927-7757(01)00748-8
Zabot, G. L., Schaefer Rodrigues, F., Polano Ody, L., Vinícius Tres, M., Herrera, E., Palacin, H., et al. (2022). Encapsulation of bioactive compounds for food and agricultural applications. Polymers 14, 4194. doi:10.3390/polym14194194
Zanini, M., Marschelke, C., Svelostav, S. E., Marini, E., Synytska, A., and Isa, L. (2017). Universal emulsion stabilisation from the arrested adsorption rough particles at liquid-liquid interfaces. Nat. Commun. 8, 15701. doi:10.1038/ncomms15701
Keywords: ibuprofen, encapsulation, Pickering emulsion, hydrophobized silica, paraffin oil
Citation: Ramos D, Sapin-Minet A, Marchal P, Parent M, Sadtler V and Roques-Carmes T (2024) Ibuprofen encapsulation inside non-conventional O/W Pickering emulsions stabilized with partially hydrophobized silica. Front. Coat. Dye In. 2:1422260. doi: 10.3389/frcdi.2024.1422260
Received: 23 April 2024; Accepted: 28 October 2024;
Published: 12 November 2024.
Edited by:
K. R. Justin Thomas, Indian Institute of Technology Roorkee, IndiaReviewed by:
Antonio Perazzo, Novaflux, United StatesEduardo Méndez, Universidad de la República, Uruguay
Copyright © 2024 Ramos, Sapin-Minet, Marchal, Parent, Sadtler and Roques-Carmes. This is an open-access article distributed under the terms of the Creative Commons Attribution License (CC BY). The use, distribution or reproduction in other forums is permitted, provided the original author(s) and the copyright owner(s) are credited and that the original publication in this journal is cited, in accordance with accepted academic practice. No use, distribution or reproduction is permitted which does not comply with these terms.
*Correspondence: Thibault Roques-Carmes, dGhpYmF1bHQucm9xdWVzLWNhcm1lc0B1bml2LWxvcnJhaW5lLmZy