- 1Laboratoire des Sciences du Climat et de l’Environnement – Institut Pierre-Simon Laplace (LSCE-IPSL), Université Paris-Saclay, Centre d’Etudes de Saclay, L’Orme des Merisiers, Gif-sur-Yvette, France
- 2Schoool of Arts, Sciences, and Humanities, University of São Paulo, São Paulo, Brazil
- 3Department of Geosciences, Geo- and Environmental Research Center, University of Tübingen, Tubingen, Germany
- 4International Water Research Institute, University Mohammed VI Polytechnic, Benguerir, Morocco
- 5Department of Environment, Agriculture, and Geography, Bishop’s University, Sherbrooke, QC, Canada
- 6Department of Meteorology and Geophysics, University of Vienna, Vienna, Austria
- 7Département de Géographie, Université de Montréal, Montréal, QC, Canada
- 8Environnements et Paléoenvironnements Océaniques et Continentaux (EPOC), Université de Bordeaux, Bordeaux, France
- 9Past Global Changes (PAGES), Bern, Switzerland
- 10Research Center for Climate and Atmosphere, National Research and Innovation Agency (BRIN), Bandung, Indonesia
- 11Laboratoire de Planetologie et Geoscience, UMR6112, Nantes Université, Nantes, France
- 12Climate Change Research Centre, University of New South Wales, Sydney, NSW, Australia
- 13ARC Centre of Excellence for Climate Extremes, Sydney, NSW, Australia
- 14Department of Geosciences, Princeton University, Princeton, NJ, United States
- 15NSF National Center for Atmospheric Research, Climate and Global Dynamics Laboratory, Boulder, CO, United States
- 16Laboratory of Ocean and Atmosphere Studies (LOA), Earth Observation and Geoinformatics Division (DIOTG), National Institute for Space Research (INPE), São Paulo, Brazil
- 17Department of Environmental Sciences, Statistics, and Informatics, Ca’ Foscari University of Venice, Venice, Italy
- 18MARUM – Center for Marine Environmental Sciences, University of Bremen, Bremen, Germany
- 19Woods Hole Oceanographic Institution, Woods Hole, MA, United States
- 20British Antarctic Survey, Cambridge, United Kingdom
- 21NSF National Center for Atmospheric Research, Climate, and Global Dynamics Laboratory, Boulder, CO, United States
Paleoclimate information has played an instrumental role in showing how fast climate can vary and how large these changes can be. It provided the first vivid demonstration of the relationships between atmospheric greenhouse gas concentrations and surface air temperatures, as well as striking representations of climate change impacts and possible feedbacks within the climate system, such as those associated with vegetation or ice sheet changes. Here, a short review of recent advances in paleoclimate studies is provided, with the objective of showing what this information on past climates and environments can bring to research on current and possible future climates. We advocate that (1) paleoclimatic and paleoenvironmental information can be leveraged for narratives about climate change, in particular at the local and regional levels, (2) paleoclimate data is essential for out-of-range tests of climate models, since future climates are also out of the range of recent climate information used for calibrating climate models, (3) paleoclimate data, in particular for the last millennia, is essential for taking multi-centennial and multi-millennial variability into account when describing trends related to anthropogenic forcings and attributing climate change signals, in particular for extreme and rare events, and (4) paleoclimates also provide extremely valuable information for initializing the slow components of climate models. In addition, we show how paleoclimate studies can be beneficial to put recent and future climate change into context and improve our knowledge on key processes. They can both benefit from and contribute to models and knowledge based on the study of recent and future climates.
1 Introduction
Paleoclimate information arises from a wealth of archives, depicting local to global aspects of climate and environmental changes. It has been instrumental to put the current climate into a long-term perspective and assess the role of human activities in the ongoing climate warming (Arias et al., 2021; Figure 1). In particular, analyses of paleorecords have highlighted the link between greenhouse gas atmospheric concentration and climate, provided evidence that there can be multiple equilibrium states of the climate system or in its components and shown that there can be abrupt shifts in the climate system without large changes in external forcing. The strength of paleoclimate reconstructions comes from the fact that some paleoclimate records, such as the CO2, CH4, N2O and mineral dust records from ice cores and isotopic records from many archives, are direct measurements of physical or biogeochemical characteristics of the climate system. Other records, such as floral or faunal records, lake level records, sea level records (Figure 1), directly relate to possible impacts of climate change on the environment, often indicating feedbacks operating within the climate system or thresholds leading to high impact events or tipping points. In addition, archives such as corals and speleothems even allow the documentation of changes on finer scales — interannual, interdecadal— variability for these far-from-present climate states.
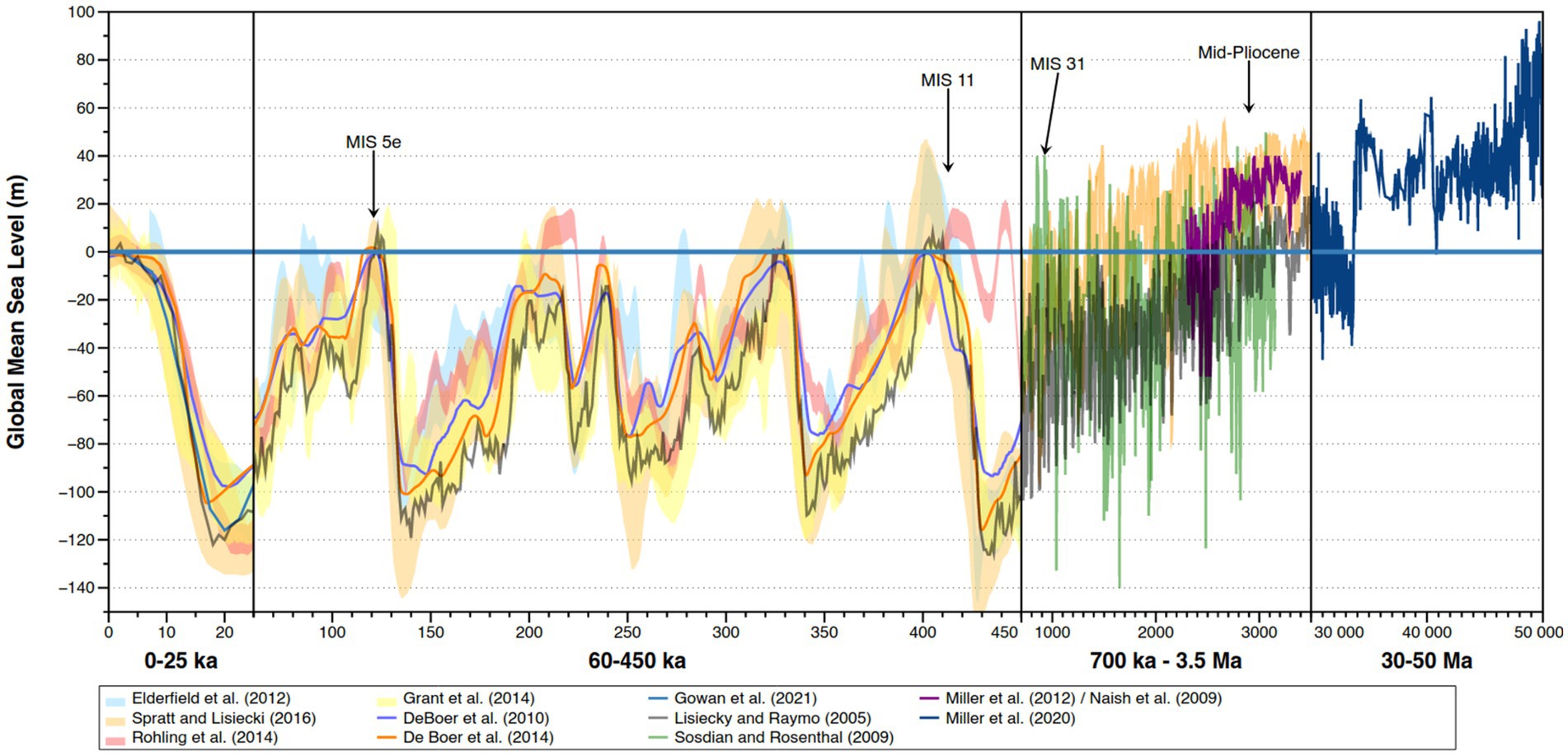
Figure 1. How Earth’s climate has changed, as recorded by global mean sea level, between 50 millions years ago and the present day. Data from: Elderfield et al. (2012), Spratt and Lisiecki (2016), Rohling et al. (2014), Grant et al. (2014), De Boer et al. (2010), De Boer et al. (2014), Gowan et al. (2021), Lisiecki and Raymo (2005), Sosdian and Rosenthal (2009), Miller et al. (2012), Naish et al. (2009), and Miller et al. (2020).
These records combined with climate modeling are fundamental to expand and test our understanding of the climate system. Models of different complexities and resolutions are needed to explore the long term and regional fluctuations of climate and interactions between the different components of the Earth System depicted by paleoclimate and paleoenvironmental records. These records, describing global and regional conditions over land, ocean and ice, are available for numerous periods and offer stringent tests for models used to compute climate projections. This is essential to assess their ability to represent future climate changes larger than the ones that prevailed during the instrumental period (Harrison et al., 2015; Burls and Sagoo, 2022). Some vivid examples of past climate and environmental states different from the present one include past episodes of widespread glaciation, contrasting with periods of scarce or no permanent land ice at the Earth’s surface, episodes of a Sahara covered by lakes and vegetation, that has become an iconic topic within the Paleoclimate Modeling Intercomparison Project (PMIP) to test vegetation and soil feedbacks in climate simulations (Braconnot et al., 2007; Menviel et al., 2021). There are other examples for which the understanding and the representation of the coupling between climate and the cryosphere remains a challenge for climate models, both for past and future climates (Nowicki and Seroussi, 2018). Records of abrupt climate and environmental shifts during the last deglaciation are receiving a lot of attention and inform on the sensitivity of the ocean circulation and heat transport to fresh water fluxes and concomitant changes in other components of the climate system. Taken together, those climate reconstructions, modeling studies and model-data comparisons are highly relevant to society by providing unique information on natural and anthropogenic caused fluctuations of the Earth’s climate and environment. Moreover, these reconstructions are essential for testing and improving the robustness of climate models in simulating the Earth system’s responses to rapid and gradual changes at a societally relevant scale.
Paleoclimate information therefore puts recent and future climate change into context and help us quantify how far human activities are pushing the climate system into unknown new states, and what these new states might look like. This concept note will highlight recent advances, some of which were presented at the 2023 WCRP Open Science Meeting, in depicting and understanding past responses to various external forcings, in terms of feedbacks and multiple time scale variability and abrupt events. From these, we will draw lessons and opportunities for future studies which would strongly benefit from possible collaborations within WCRP core projects and lighthouse activities. Indeed, in our opinion, the next years should foster work on the integration of global, regional and local climate changes on multiple timescales, improve the understanding of the linkages between climate and environmental changes (e.g., ecosystems, hydrosystems, biodiversity), and provide information for risk assessments, including for past humans. There are strong needs and opportunities for exciting work on paleoclimates and paleoenvironments in collaboration with experts on current and future climate change processes, on climate change impacts and on vulnerability and risks. These collaborations are essential for paleoclimate and paleoenvironmental information to deliver lessons, for advancing our knowledge on climate change, and for adaptation and mitigation.
In section 2, we provide a few examples of recent advances in paleoclimate studies (on cold, warm climates and abrupt changes) which relate to several aspects of future climate change. This is of course not intended to be an exhaustive overview, but rather an illustration of the potential of paleoclimate information for anticipating future possible climate and environmental changes. In section 3, we draw perspectives and opportunities for a better integration of research on past, present and future climate and environmental changes. Section 4 highlights the resulting recommendations.
2 Recent advances in paleoclimate studies yielding new perspectives
2.1 Assessing climate sensitivity taking advantage of multiple, cold and warm periods
The multiple constraints from both warm and cold periods have been used to estimate equilibrium climate sensitivity (ECS, i.e., the global mean surface air temperature response to a doubling of CO2 since the preindustrial, at equilibrium) (Sherwood et al., 2020), along other types of evidence. Since then, Zhu et al. (2020, 2022) utilized paleo-data to evaluate the high ECS of the Community Earth System Model version 2 (CESM2), which was 6°C—significantly higher than previous models. This model significantly overestimated global cooling during the Last Glacial Maximum (LGM) and warming during the early Eocene, indicating its high ECS was inconsistent with paleoclimate data. These studies attributed the excessive climate sensitivity of CESM2 to issues in the parameterization of clouds. By collaborating closely with model developers, Zhu et al., 2022 were able to pinpoint and rectify these deficiencies. The revised model shows markedly improved performance in simulating both the LGM and early Eocene climates, and it now exhibits a much-reduced ECS of 3°C, while still performing reliably for the present-day climate (Zhu et al., 2022; Zhu et al., 2024). These improvements will be incorporated into the next generation of CESM. This work continues the tradition of leveraging paleoclimate data to assess and refine climate models, following the spirit of the earlier work of Manabe and Broccoli (1985).
Recent advancements have also enhanced our ability to directly quantify ECS using paleo-data on climate forcing and response. Sherwood et al. (2020) highlighted the critical role of paleoclimate information in constraining ECS and identified the need for improved quantification of paleoclimate forcings—such as that from ice sheets—as well as better understanding the pattern effect and the state dependence of forcings and feedbacks. Building on this foundation, Cooper et al. (2024) developed a novel framework that integrates recent temperature reconstructions of the LGM with a complete, model-based quantification of the LGM radiative forcing (e.g., Zhu and Poulsen, 2021) and the effect of sea surface temperature (SST) patterns on feedbacks. This approach has further refined ECS estimates, demonstrating how collaborative efforts and the application of paleo-data and state-of-the-art methods can advance our understanding of climate sensitivity.
The emergent constraint approach is another valuable method for reducing uncertainties in ECS by leveraging observable paleoclimate data and statistical relationships between these observations and ECS across multiple models. Renoult et al. (2020) employed recent estimates of LGM and Pliocene global mean surface temperatures and a suite of PMIP and PlioMIP (Pliocene Model Intercomparison Project) simulations to constrain ECS. Their emergent constraint approach yielded a tighter upper bound for ECS, with a central estimate of 2.5°C and an uncertainty range of 0.4–4.0°C. Renoult et al. (2023) suggested that increasing the number of model simulations enhances the robustness of these constraints, particularly for models with higher ECS values. They also stressed the need for a deeper understanding of non-CO2 forcings and feedbacks and their state dependency.
2.2 Cold climates
Paleoclimate records provide independent constraints over land and ocean with which to investigate and evaluate models that range in complexity from idealized theoretical models to fully coupled Earth system models. The Last Glacial Maximum (LGM) has been extensively used for such an endeavor, in particular thanks to PMIP (Kageyama et al., 2018; Kageyama et al., 2021b) for the latter type of models. On the other hand, applying simple dynamical models to past climates helps to identify and evaluate fundamental mechanisms of climate change by exploring large changes that exceed those in the short instrumental record of modern climate. For example, consideration of terrestrial and marine temperature during the LGM provides an opportunity to explore the broad applicability of an idealized model for the amplification of warming over land, relative to sea, that links low-latitude humidity and temperature (Byrne and O’Gorman, 2018). In a recent study, proxy records and PMIP4 (PMIP 4th phase) model simulations were used to independently evaluate this terrestrial amplification theory, finding close agreement in the land-sea warming ratio between paleotemperature change since the LGM and future greenhouse gas-driven warming (Seltzer et al., 2023).
2.3 The last interglacial’s cryosphere
The rapid warming and decline in Arctic sea ice over the past 40 years is a hallmark of contemporary climate change. Climate models have struggled to accurately capture this warming and sea ice loss (Notz and the SIMIP Community, 2020), which reduces confidence in future projections (Fox-Kemper et al., 2021). Past warm climates with minimal Arctic sea ice can thus provide valuable insights for the future (e.g., Otto-Bliesner et al., 2017). During the Last Interglacial (LIG), orbital forcing resulted in summertime top-of-atmosphere shortwave radiation in the Arctic being 60–75 Wm−2 greater than during the preindustrial period. This forcing is in nature very different from the increased greenhouse gas concentrations typical of future climate forcings, but Kageyama et al. (2021a) showed a wide range of sea-ice responses both for LIG and higher CO2 forcings, and a clear relationship between the model responses to both forcings. The idea was then that LIG Arctic sea-ice reconstructions could help to evaluate model ability to represent sea-ice loss for climates warmer than today. All PMIP4-CMIP6 models showed a reduction of the seasonal minimum extent, with one model, HadGEM3, simulating a nearly ice-free Arctic during the LIG (with sea ice extent falling below 1 × 106 km2 at its minimum). The near-complete loss of summer sea ice in HadGEM3 was attributed to the model’s advanced representation of melt ponds, shallow pools of water on the surface of Arctic sea ice that significantly influence the absorption and reflection of sunlight (Guarino et al., 2020; Diamond et al., 2021, 2024). The sea-ice reconstructions and ice-core data compiled by Malmierca-Vallet et al. (2018) and Kageyama et al. (2021a) indeed showed evidence of this sea-ice area reduction during the LIG, but were inconclusive about this period being seasonally ice free. The HadGEM3 simulation, however, matched continental temperature records around the Arctic remarkably well (Guarino et al., 2020). Recently, new LIG sea-ice extent and summer temperature datasets (Sime et al., 2023; Vermassen et al., 2023) showed that Arctic warming was closer to 3.7 ± 1.5°C above PI levels, rather than the previously suggested 4–5°C. They also showed that the LIG Arctic climatological minimum sea-ice area ranged from 1.3 to 1.5 × 106 km2, with summers fluctuating between ice-free and near-ice-free conditions, demonstrating the realism of the HadGEM3 simulation, performed with a high ECS, explicit melt pond scheme and supporting the reliability of such a model for future Arctic climate projections. These recent publications therefore provide valuable data for evaluating climate simulations, hence helping model evaluation for the forthcoming AR7 FastTrack experiments (https://wcrp-cmip.org/cmip7/).
Furthermore, the Greenland Ice Sheet is losing mass at an accelerating rate as the climate warms (Church et al., 2013). Although the future surface mass balance of the ice sheet is uncertain and is strongly dependent on the climate trajectory (Aschwanden et al., 2016; Fettweis et al., 2013; Mouginot et al., 2019), this pattern of mass loss will likely continue as surface elevation lowers and air temperatures increase, possibly leading to the substantial retreat of the ice sheet. Models that couple the climate and Greenland Ice Sheet are increasingly being used to project the contribution of this ice sheet to global mean sea level rise during the 21st century and beyond (Muntjewerf et al., 2020). Benchmarking these projections is challenging though with the very short historical record. To better understand climate–ice sheet interactions and inform projections of the future, it is thus helpful to study the behavior of climate ice-sheet interactions during past warm climates in Earth’s history. Ice cores near the Greenland Ice Sheet summit provide evidence that central Greenland remain glaciated through the Last Interglacial (Yau et al., 2016). Sediment cores drilled from the Eirik Drift off southern Greenland indicate vegetation (de Vernal and Hillaire-Marcel, 2008) and a smaller ice sheet (Colville et al., 2011) in southern Greenland. Recent modeling with the CMIP6 Community Earth System Model (CESM2) coupled with an evolving Greenland Ice Sheet model and vegetation model simulated a forest and tundra expansion in Canada and Greenland and Arctic summer warming over land of 4-6°C (Sommers et al., 2021) in agreement with proxy reconstructions (CAPE-Last Interglacial Project Members, 2006; Sime et al., 2023). The Greenland Ice Sheet reached its minimum size at 121.9 thousand years ago, losing an equivalent of 3.0 m of sea level compared to the present day, and then regrew as summer temperatures began to cool. Changes in Arctic vegetation distribution during the Last Interglacial had a strong influence on the climate, and therefore how much the Greenland Ice Sheet retreated. A companion CESM simulation employing static vegetation based on pre-industrial conditions showed a much smaller ice-sheet retreat, highlighting the importance of the changes in high-latitude vegetation distribution for amplifying the ice-sheet response (Sommers et al., 2021).
The West Antarctic Ice Sheet (WAIS) is at risk of rapid large-scale deglaciation («collapse») due to global warming, which could lead to a global eustatic sea-level rise of 3.3 m (Bamber et al., 2009). To assess the likelihood of a WAIS collapse in the future, it is helpful to understand whether, and why, the WAIS has collapsed in the past. The LIG is of particular interest, because (i) its temperatures were close to those projected for the near future, and (ii) it is captured in several Antarctic ice core records (Masson-Delmotte et al., 2011). Stable water isotopes archived in the ice reflect the history of Antarctic surface climate, atmospheric circulation, and oceanic conditions, but it is unclear which isotopic signatures would indicate a collapse of the WAIS during the LIG. Recent modeling studies have addressed this question by comparing simulations of Antarctic climate in which the WAIS has collapsed with control simulations in which the WAIS is intact (e.g., Steig et al., 2015; Holloway et al., 2016; Goursaud et al., 2021; Dütsch et al., 2023; Hutchinson et al., 2024). They found distinct isotopic responses to WAIS collapse at sites near the WAIS relative to sites in interior East Antarctica, including a substantial increase in δ18O at Skytrain Ice Rise, a West Antarctic ice core that was drilled recently (Mulvaney et al., 2021). The measured δ18O in this ice core is increased by approximately half the simulated value in the LIG, suggesting partial WAIS collapse (Wolff et al., 2024). Future ice cores near the WAIS such as Hercules Dome (Fudge et al., 2023), as well as more comprehensive modeling studies, will provide further constraints on the extent of WAIS ice loss during the LIG and help to estimate the likelihood of WAIS collapse in the future. Where possible, models should also account for changes in sea-ice concentrations, as WAIS collapse simulations with LIG greenhouse gas concentrations and orbital forcing alone cannot fully explain the observed δ18O anomalies in Antarctic ice cores.
2.4 Abrupt climate change
Here we use the term “abrupt” to classify a change in a specific component of the climate system that at least apparently occurred quicker than the forcing, shifting the component to a different state (Lenton et al., 2023). This suggests the operation of non-linear processes in that specific component of the climate system. The very possibility of specific components of the climate system to react non-linearly to forcing is of significant concern to society, since our capacity to adapt to climate change depends on time, among other parameters (IPCC, 2022). Research on abrupt climate change greatly benefited from lessons from paleoclimates, since some components of the climate system that may reach a critical threshold within a societally relevant timeframe have done so in the past. This is notably the case for the Atlantic Meridional Overturning Circulation (AMOC) (Henry et al., 2016; Ditlevsen and Ditlevsen, 2023).
Diverse and coherent paleoclimate evidence suggests that AMOC strength abruptly decreased (and recovered) multiple times during the last glacial period, substantially decreasing oceanic heat transport from the South Atlantic to the North Atlantic (Malmierca-Vallet et al., 2023). These pieces of evidence span from direct proxies recording a decrease in deep-water flow velocity (Henry et al., 2016) to indirect proxies showing poor deep-water ventilation (Campos et al., 2020), as well as warmer (colder) SST in the South (North) Atlantic (Santos et al., 2022). Marked decreases in AMOC strength seem to be associated with a surface freshening (i.e., decrease in sea-surface density or seawater salinity) of the high latitudes of the North Atlantic due to iceberg melting (Zhou and McManus, 2024) that were released because of subsurface warming (Max et al., 2022). Importantly, decreases in sea-surface density in the high latitudes of the North Atlantic hampers deep-water formation by disturbing the salt-advection feedback, substantially weakening the AMOC. Ongoing climate change is causing a decrease in sea-surface density in the high latitudes of the North Atlantic through SST warming, increased direct precipitation and Greenland meltwater discharge. These processes may lead to an abrupt decrease in AMOC strength within the 21st century (Ditlevsen and Ditlevsen, 2023), and, in the case of Greenland meltwater discharge, highlight a cascading destabilizing effect between the Greenland Ice Sheet and AMOC strength (Wunderling et al., 2024).
Past decreases in AMOC strength significantly changed tropical precipitation. These changes are particularly well recorded over northeastern South America, where precipitation is strongly controlled by the tropical rain belt. During AMOC slowdowns, northernmost South America experienced a decrease in precipitation, while marked increases in precipitation were recorded over tropical South America to the south of the equator (Zhang et al., 2017; Campos et al., 2019). These changes were synchronous and proportional to the warming (cooling) of the surface South (North) Atlantic due to the decreases in AMOC strength (Mulitza et al., 2017). Recent model experiments suggest that a future AMOC weakening will again decrease precipitation over northernmost South America and increase rainfall over northeastern Brazil (Bellomo et al., 2021; Ben-Yami et al., 2024), increasing the vulnerability of the Amazon Rainforest to climate change (Akabane et al., 2024).
2.5 Oceanic features from Indonesian throughflow circulation
The previous example shows the importance of relating abrupt changes in the atmosphere, cryosphere and the ocean. Next, we report on new research on the strength of an important ocean feature, the Indonesian Throughflow (ITF). ITF acts as the only tropical pathway for exchange between ocean basins in global ocean, especially facilitating the transfer of warm Pacific Ocean water into the Indian Ocean (Susanto and Gordon, 2005; Gordon et al., 2019). The ITF lies in the center of atmospheric deep convection and plays a central role in global climate system (Sprintall et al., 2019). Intensive studies of the modern ITF have explored the main pathway of the ITF at the Makassar Strait (~80% water mass originating from the Pacific Ocean) in more detail than other ITF regions, i.e., Halmahera Sea, Lombok Strait, Ombai Strait, and Timor Sea (Figure 2, Sprintall et al., 2019; Gordon et al., 2019). Indeed, the longest available record of the ITF variability (i.e velocity, volume transport) is for the Makassar Strait, within the Labani Channel (which sill depth is around 680 meters). It is now approximately 13.5 years long (Gordon et al., 2019).
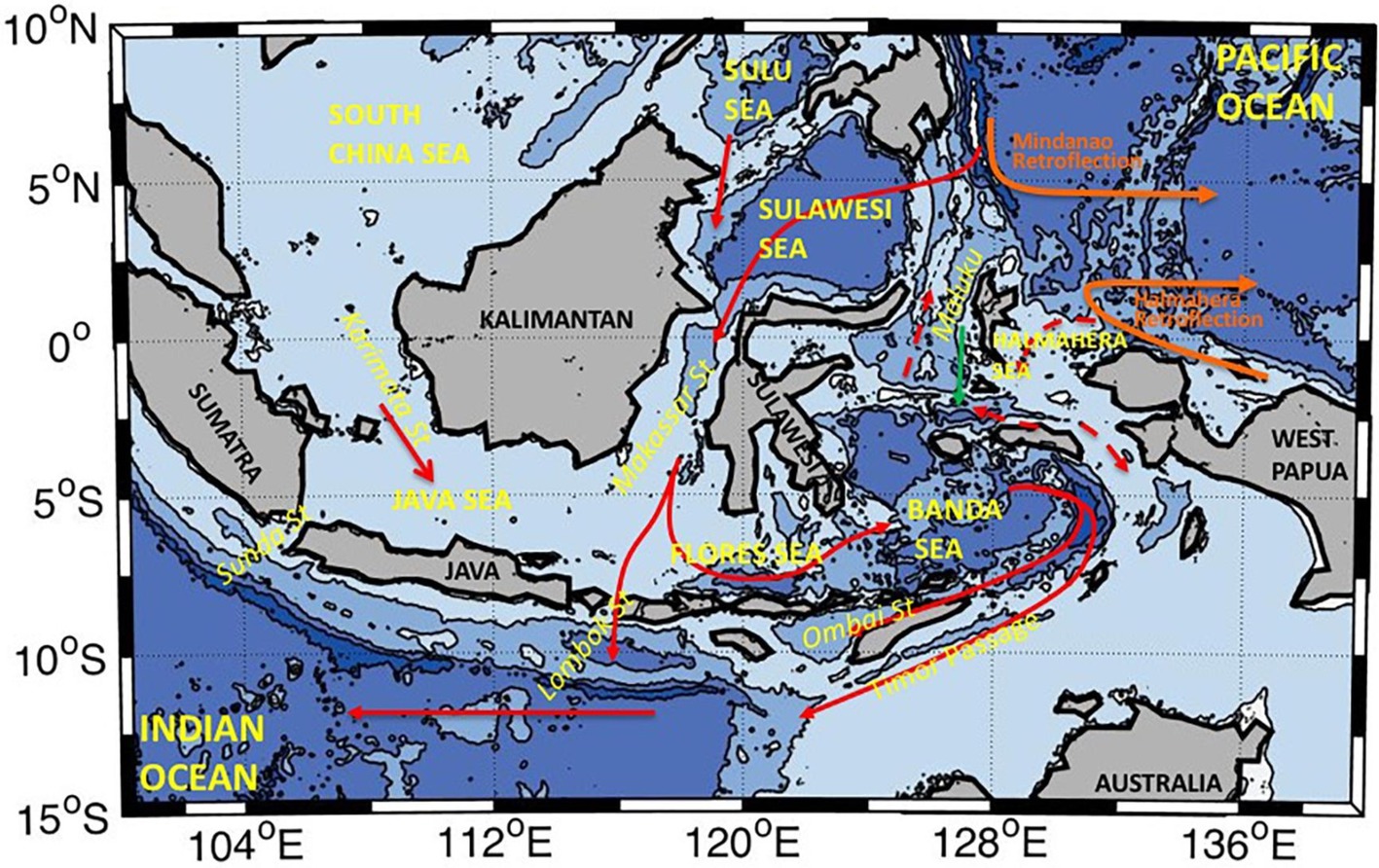
Figure 2. A schematic map of the ITF currents, seas, and basins (Sprintall et al., 2019). Upper ocean current pathway is in red, lower pathway in green. Dashed lines indicate uncertain pathways.
Under future climate warming scenarios, the changes of surface wind stress in response to greenhouse gas forcing are considered too small to drive the projected centennial changes in the ITF transport (Feng et al., 2017; Sen Gupta et al., 2016; Hu et al., 2015), while larger changes are driven by the weakening of AMOC (Sun and Thompson, 2020). The AMOC is a major tipping element in the global climate system (Ditlevsen and Ditlevsen, 2023). Sun and Thompson (2020) highlight the concomitant weakening of the ITF and the AMOC using a reduced gravity model and a CCSM4 4xCO2 experiment. This can explain the ITF weakening simulated for the 21st century in the CMIP6 simulations (Sun and Thompson, 2020). However, the link between the AMOC and ITF shown by model results need data verification. The short time series of ITF measurements are not enough to verify the models. Paleoclimate records at the Indonesian seas could provide valuable longer records of the ITF. Evidence of ITF slowdown during past warm periods would be important to fill the gap of current observations. The last deglaciation, i.e., the period of global warming from the LGM to the Holocene (11,700 years - present), was punctuated by a series of abrupt climate events in the North Atlantic: the cold Heinrich Stadial 1 (HS1), the warm Bölling/Alleröd (B/A), and a second major cooling event, the Younger Dryas (YD). HS1 and YD are the last period of strong disruptions of the AMOC (Broecker et al., 1992; Bond et al., 1992). Existing studies suggest ITF slowdown during these periods of weakened AMOC. However, the causes of this slowdown are debated. Some studies relate the slowdown to El Niño-like wind driven forcing and a southward shift of the ITCZ (Hendrizan et al., 2023; Fan et al., 2018; 2016; Zhang et al., 2018; Ding et al., 2013). Under such conditions, an enhanced Mindanao current may increase the transport of warm and saline north Pacific water eastward into the central and eastern Pacific Ocean, thus less would be leaked westward into the ITF region (Fan et al., 2018, 2013). Other studies relate the ITF slowdown to AMOC changes, such as shown for the future by simple ocean and full climate models (Hendrizan et al., 2017). However, the mechanism relating the ITF and AMOC for those past climate periods still need to be clarified.
2.6 Long-term changes in short-term variability
Past changes in interannual to multidecadal variability can inform on the linkages between this short-term variability and the mean climate and provide evidence of expected changes in the future, despite the fact that the forcing mechanisms are not direct analogs. There has been growing interest on this topic in the last years and new possibilities offered by the fact that (1) progress has been made in the reconstructions of past variability from corals, bivalve shells (e.g., Carré et al., 2021), speleothems (i.e SISAL data base, Atsawawaranunt et al., 2018), tree rings and multi proxy databases (i.e., PAGES 2 k Network, Neukom et al., 2019) (2) the same versions of Earth System Models are used to simulate present future and past climate, with sufficient resolution and complexity to study short term variability and (3) the number of transient simulations with these models is growing. There are therefore new possibilities to analyze short term variability and how it responds to various forcings over a wider range of periods and models.
This is the case for the study of El-Niño Southern Oscillation (ENSO) variability in the tropics. Since the review, by Lu et al. (2018), of the mechanisms explaining the response of ENSO variability to different forcing factors in different climates, along with the missing knowledge to fully understand ENSO dynamics and teleconnections, several analyses of multi-model ensembles simulations have confirmed key mechanisms or brought new results. For instance, Brown et al. (2020) have performed multi model analyses of ENSO variability across different periods using the PMIP4-CMIP6 ensemble of simulations to shed light on key factors affecting ENSO events. Despite different representations of ENSO and mean state biases compared to present day observations, almost all PMIP4-CMIP6 simulations produce a reduction of ENSO variability for the last interglacial and the mid-Holocene simulations, whereas changes in ENSO variability is more diverse for the Last Glacial Maximum or simulations where atmospheric CO2 concentration is increased by 1% per year. It highlights the role of the nature of the forcing (orbital or trace gases and ice sheets) on the ENSO response (Brown et al., 2020). For the mid-Holocene, process studies have also confirmed the role of the changes in seasonality in the development of equatorial Kelvin wave associated with El-Niño events (Luan et al., 2012; Zhang et al., 2022), and the dominant role of the thermocline feedback in the damping of ENSO magnitude in response to mid-Holocene insolation (An and Choi, 2014; Chen et al., 2019; Song and Chen, 2022).
Following the initial work by Emile-Geay et al. (2016), the coral and shell data synthesis covering the Holocene in the Pacific has been extended and provides ground truth estimates of variability and seasonality in different key places in the Pacific Oceans (Carré et al., 2021). These paleoclimate reconstructions of interannual to multidecadal variability also offer the possibility to investigate changes in the diversity of El Niño events (Capotondi et al., 2015). The available literature yields conflicting conclusions on the current and future changes in ENSO flavors, characterized as East or central Pacific events in different climate configurations (Fredriksen et al., 2020; Freund et al., 2020). Thanks to the long-term perspective offered by paleoclimate, it was possible to infer that different, but equivalent methods used to characterize ENSO flavors under modern conditions lead to contradicting results when considering transient Holocene simulations (Abdelkader Di Carlo et al., 2023). This shows the need to be more explicit in the analysis of ENSO diversity and a detailed reassessment of the exact conclusions drawn from the literature. Similar conclusions were raised for the last millennium for the teleconnections with hydrological changes in North America (Luo et al., 2022). Even though additional records would be needed to reduce uncertainties in coral and shell reconstructions, a reduction the ratio of variance between the eastern and central Pacific events along the Holocene is emerging. It is also present in some of the available transient Holocene simulations run with different climate models, however in others, centennial variability masks the long-term trend (Carré et al., 2021).
In addition, changes in teleconnections associated to the major modes of variability such as ENSO in the tropics, the Pacific Decadal Oscillation (PDO) in the Pacific, or the Atlantic Multidecadal Oscillation (AMO) in the Atlantic can be further investigated. Results from transient Holocene simulations highlight the chaotic aspects of ENSO variability and its teleconnections with other regions (Falasca et al., 2020), such as the Indian ocean (Falasca et al., 2022) or Indian and African monsoon (Braconnot et al., 2019; Crétat et al., 2024). Multicentennial to millennium variability in monsoon events emerge from a combination of time scales. Over the Holocene, the interannual to decadal teleconnections between the African and Indian monsoon rains are affected by the long-term changes in the mean state, so that variability increased over India and decreased in Africa. The Indian signal is associated with changes in time of the teleconnection between ENSO and the Indian dipole variabilities. For Africa, the trends have been associated with changes in AMO-Mediterranean variability, and, depending on models, to teleconnections with ENSO (Crétat et al., 2024). Further comparisons with reconstructions are needed to fully evaluate the robustness of these conclusions.
3 Toward a better integration of research on past, present and future climate and environmental changes
3.1 Placing current climate change into the paleo context
More work needs to focus on integrating paleoclimate research into research on contemporary and future climate and environmental change. Investigating past climate offers a unique lens through which to look at anthropogenic climate change, insofar as it is possible to assess the natural boundaries of systems and understand the impacts of abrupt climate change on ecosystems in the past. Although the rate at which CO2 concentrations have increased as a result of human activity in the atmosphere is unprecedented, paleoclimate data reveal various extreme events that have parallels with the contemporary situation. Paleoclimate data can reveal how abrupt changes took place in the past as well as the rate of ecological response to those changes. These insights are at various scales: from understanding spatiotemporal environmental changes more broadly, to assessing the efficacy of adaptive measurements in the past, such as water management systems employed by past societies affected by climate change. The value of paleoclimate data is far-reaching: from modeling, informing policy, as well as acting as narratives to help public understanding of climate science.
Given the importance of paleoclimates for establishing a long-term baseline, or reference point for anthropogenic change, paleoclimate records hold promise in the field of climate attribution and associated work, e.g., insurance studies, where a natural (i.e., pre-human) boundary conditions define what is and is not an extreme event. It is important to identify what is a natural deviation versus what is an anthropogenic signal superimposed on top of it. If attribution of climate extremes is based on observed records, since humans have significantly altered the carbon cycle, then the bigger picture of natural climate variability is lost (Easterling et al., 2016). On the other hand, climate models used alone can lead to overestimations of the attribution of extreme events (for example, Bellprat and Doblas-Reyes, 2016). These authors argue that proper ensemble calibration is needed to represent the probabilities of extreme events. Some paleoclimate records lack the fine temporal resolution for weather events, but more protracted climate events are visible in the paleoclimate record (e.g., Terminal Classic Maya drought, Little Ice Age, etc.) and so can still be useful for establishing baseline climate conditions. For example, drought reconstructions from tree ring records provide essential context to understand the statistical likelihood of modern hydrological observations in southwestern North America, enabling the quantitative attribution of recent multidecadal megadrought conditions to anthropogenic warming (Cook et al., 2010; Williams et al., 2020). Taking the last millennium or the last two millennia as the period of reference, instead of “preindustrial period” as is usual for analysing historical and future climate change, allows to take multi-centennial natural variability as well as the impact of volcanic and solar variability into account. This leads to better evaluation of potential tipping points in the climate system (Michel et al., 2022). This implies that for climate model simulations used for these analyses, a seamless definition of forcings and boundary conditions for the past millennia and for scenarios used for future projections is essential.
Key past climate transitions also document the possible speed of natural climate changes. Transitions associated with Dansgaard-Oeschger events during the last glacial periods, and with the abrupt events of the last deglaciation (HS1, B/A, YD), occur within decades and offer natural examples to study the impact of past abrupt changes occurring as fast as current climate change. Such spontaneous climate shifts are now represented by a few climate models (Malmierca-Vallet et al., 2023, 2024 for a recent review), yielding new perspectives on the representation of tipping points in climate models, and on the study of potential impacts of the climate system crossing these tipping points.
3.2 What can paleoclimate tell us about potential future climate change surprises?
Progress in paleo-reconstruction resolutions, ensemble of simulations and long transient simulations offer the possibility to improve our understanding of the centennial to multicentennial variability in the climate system. Several questions are still pending on the relationship between this variability and external forcing, the role of this variability on the slow changes in the climate mean state and the interaction between this variability and variability at shorter time scales. Analyses of Holocene transient simulations have highlighted that models consistently produce 120-150-year oscillations, which is consistent with estimates (120–130 years) from various proxy records (Askjær et al., 2022). Future progress is tied to the continuous development of methodologies to aggregate different types of records while preserving variability (Essell et al., 2023). Improvements should continue thanks to better data coverage and possibilities to refine sampling intervals. However, several challenges need to be faced, arising from the chaotic nature of this variability, so that new methods need to be developed to be able to compare model results with paleoclimate reconstructions and test if models reproduce the right characteristics of these multi-centennial to millennium events. There is indeed little chance that such events happen at the exact same time in simulations compared to reconstructions, unless an external forcing plays a key role. Also, such a time scale of variability certainly involves modes of variability of the different ocean basins. The challenge is illustrated in Figure 3. It presents the sea surface pattern associated with the first mode of coupled variability between the sea surface temperatures and the African and Indian monsoons at the 50-500-year time scale. It is estimated from two transient experiments of the last 6,000 years with different versions of the IPSL model (Braconnot et al., 2019). Both simulations produce the see-saw pattern in the Atlantic associated to a reduction of the West African monsoon on the 50–500 year time scale, but they have very different patterns in the other ocean basins and over India, highlighting the role of inter-ocean phase differences on teleconnections and continental hydroclimate. A better understanding of these different interactions is needed. It has the potential to help to better understand the sources of climate predictability at the interannual to multidecadal time scale. It is also relevant for the development of targeted indicators to anticipate extreme conditions or extreme events between regions and thus bring new types of constraints to risk assessments.
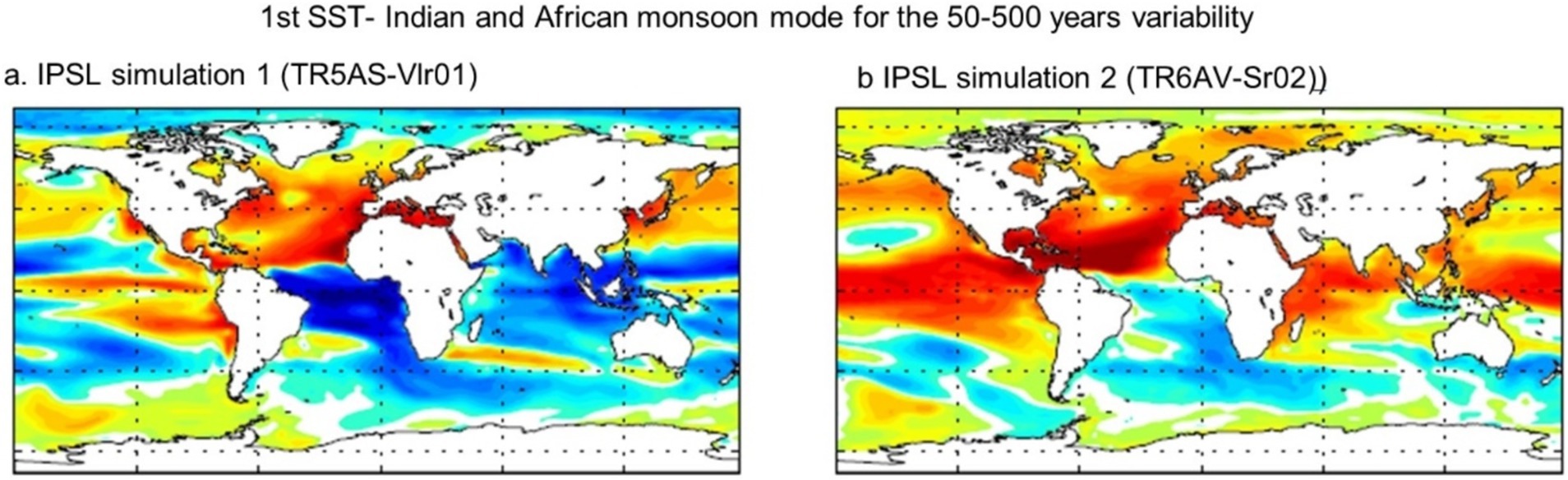
Figure 3. Sea surface temperature pattern associated to the 1st cross variability mode between SST and monsoon precipitation in India and Africa in the 50–500 year band of variability for two simulations of the last 6,000 years with two versions of the IPSL model. Adapted from Braconnot et al. (2019). The names in parentheses correspond to the standard names of the IPSL simulations.
The WCRP Safe Landing Lighthouse Activity has acknowledged the importance of including centennial to millennial variability in the “exploration of the routes to “safe landing” spaces for human and natural systems” (https://www.wcrp-climate.org/safe-landing-climates). It is developing the “What If” Modeling Intercomparison Project (WhatIfMIP), a CMIP7 Community MIP, to understand the global consequences and cascading regional impacts after the future tipping of the Earth system. These storylines of extreme outcomes in future climate settings have perspectives in the paleoclimate records and links to the PMIP.
In recent decades, the Sahel has experienced a significant wetting and re-greening and models indicate this will continue in the future (Seneviratne et al., 2021). Boreal shrubs and trees are expanding farther north in the Arctic and becoming more stressed along their southern margins. Past interglacials can provide a perspective on these changes and their consequences. Pollen analysis indicates a much wetter Sahara and Sahel with wooded grassland and grassland covering this region (Pausata et al., 2020). Pollen and plant macrofossil evidence indicate that boreal forest extended to the Arctic coast along much of North America and Eurasia (CAPE-Last Interglacial Project Members, 2006). Modeling of these interglacials suggest that these vegetation change could alter the future trajectory over land, e.g., Greenland ice sheet and oceans, e.g., ENSO, productivity, sea ice and also extreme events, e.g., tropical cyclones (Pausata et al., 2020; Sommers et al., 2021). Similarly, ice cores and marine sediments reveal that the Greenland ice sheet had a much smaller footprint during the warmer past and potentially completely disappearing over the last 1.1 Myr, a concern for the next century with its current trajectory and projections for the 21st century and beyond. This will impact coastal ecosystems and people, as well as weakening of the AMOC and precipitation patterns globally. WhatIfMIP offers a framework to investigate the global impact of such tipping elements, related to tropical and/or boreal vegetation and to the state of the Greenland ice sheet, once they have tipping under future climate conditions. As such, it uses the knowledge arising from past climates – such states have already occurred in the past – and includes it in our anticipation of potential consequences of the on-going climate change.
3.3 Climate change and early human populations
Present and future climate change arises from human activities, and its foreseen impacts on many aspects of human activities are spreading on virtually every territories and economic sectors. Early human populations also underwent large, and sometime fast, climate and environmental changes, raising the question of the impact of these on the populations (e.g., Burke et al., 2021). In the following, we give further examples of how current research activities highlighting possible links between climate changes and early human populations.
The Sahara Desert, currently one of the most inhospitable environments on Earth, has experienced periods of enhanced precipitation that supported prehistoric human populations. This dramatic climate shift, driven by changes in Earth’s orbital patterns, created favorable conditions for early human populations, providing rich habitats and migration corridors. Relevant information about the timing and sources of moisture for the Green Sahara, as well as their implications for habitability can only be retrieved from natural archives across the Sahara. In Northwest Africa, the available paleoclimate data highlight the east–west geographical extent of the Green Sahara and show that millennial-scale North Atlantic cooling events (Heinrich events) consistently resulted in drier conditions (Sha et al., 2019; Ait Brahim et al., 2023). The study demonstrates that increased westerly-originating winter precipitation and northward shift of the African summer monsoon could both result in favorable environmental conditions in North Africa. Comparing paleoclimate data with local archaeological sequences reveals abrupt climate deterioration and a decline in human density in Northwest Africa during the MIS 5–4 transition, suggesting climate-forced dispersals of populations with potential implications for migration pathways into Eurasia.
There have been numerous recent studies contributing to the development of a proxy for human population change in the past, to be able to link socio-environmental systems. Faecal stanols have been quantified in numerous studies, (D’Anjou et al., 2012; Zocatelli et al., 2017; White et al., 2018; Li et al., 2024), to reconstruct human population change. These molecules can be combined with other biomarkers, such as bile acids, which help improve source attribution. These studies are some of the first attempts to quantify human response to environmental change in the past. In the context of Mesoamerica, faecal stanols have been applied to several lake sediment cores to reconstruct population change in the context of climatic and other environmental changes. The lowland Maya are thought to have abandoned a number of their cities as part of a profound societal transformation, sometimes referred to as a collapse, hypothesized to have been the result of a series of severe droughts. Keenan et al. (2021) reveal population decline because of these regional droughts. However, using carbon and hydrogen isotope analyses of plant waxes applied to the same core, at a local scale no drought appears to have been present. This reveals the complex interplay between climate change in the past and the mechanisms of “collapse” i.e. the impact of climate change elsewhere. Additional analyses of proxies for biomass burning and land use change more generally, help tease apart the nuances of these mechanisms, and both complements, and are complemented by, archaeological data. These indicate that the relationship between population size and land use varied over time, with a transition from generally more intense fire use and C4 plant agriculture during the earlier period of occupation, with a reduction in large-scale fire use over time, suggesting a general change of land use strategy as population increased and possibly as climate varied. Novel analyses of stanols have also revealed insights into degradation of aquatic ecosystems as a result of human presence, e.g., eutrophication in the context of environmental changes (Wogau et al., 2023; Birkett et al., 2023). Going forward, our understanding of human response to environmental changes in the past will benefit from multi-proxy studies applied at a larger spatial resolution in order to understand regional responses and drivers.
3.4 Combining models and data
There are examples of paleoclimate records used to train and test climate models (e.g., Burls and Sagoo, 2022). A first aspect is to rule out potential runaway behaviors, such as reported for a feedback involving temperature, aerosols and vegetation in the LGM state by Hopcroft and Valdes, 2015. A second one is to leverage knowledge on global mean surface temperatures for key cold and warm periods to better constrain processes controlling equilibrium climate sensitivity, as in the work by Zhu et al. (2020, 2022, 2024) summarized in section 2.3.
Models and data have also been combined to get a physically consistent description of the state of the climate system, based on methods of data assimilation. This has been performed for the LGM (Tierney et al., 2020) and the Last Millennium (Jebri and Khodri, 2023). This integration must consider the various limitations of paleoclimate data, which can vary in both temporal and spatial resolution, and can often be more qualitative in nature. In these applications, as well as for model-data comparisons, it is important to take the uncertainties in paleoclimate reconstructions into account. These can arise from numerous sources including measurements, calibration, seasonality and chronology. Further collaboration between the paleoclimate reconstructions and modeling communities would help place current and future climate change into the paleo context. Climate models integrating the computation of isotopes used for climate reconstructions or, more generally, of paleoclimate indicators, are also of great value in this exercice (Tierney et al., 2020), as is preliminary work on standardization of comparisons of climate proxy data- e.g. between the signal of δ2H of plant waxes and δ18O of speleothems. These paleodata assimilation procedures open new avenues in comprehensive descriptions of different climate states (in the case of the LGM) or evolution (in the case of the last millennium). In the latter case, it also provides improved initial conditions for climate models to be used for the historical and future climate simulations.
4 Recommendations
Paleoclimate records remain the basis of our knowledge and allows testing our understanding of the climate system. Paleodata syntheses are particularly useful to test global models, considering not only physical climate feedbacks, but also different aspects of environmental feedbacks arising from carbon or geochemical cycles, or changes in ecosystems (e.g., vegetation, water).
Assessment of uncertainties in reconstructions and syntheses is particularly important for this benchmarking exercise, as well as for paleodata assimilation in climate models. This includes the potential re-examination of initial measurements given new information on any particular record. Data syntheses will be particularly useful to study climate transitions (e.g., the last deglaciation; Ivanovic et al., 2016). Several challenges still need to be faced to refine chronologies and be able to provide different types of indicators on a common scale. In addition, understanding how climate signals are recorded in different archives is necessary to better constrain the climate information provided by these different archives (e.g., ice cores, marine sediment cores, speleothems). In addition to global reconstructions, systemic approaches at the regional to local scale are relevant for impact studies and improved understanding of past vulnerability and resilience to climate change, extremes or abrupt events.
Paleoclimate modeling is based on many types of models, from conceptual to those including the maximum number of processes or using the finest possible resolution. Knowledge has progressed thanks to this diversity: conceptual models were developed to understand large-scale climate processes and feedbacks and the existence of multiple states (e.g., Alvarez-Solas et al., 2010); models including representation of climate indicators (e.g., isotopes, tracers, vegetation) help deciphering the measurements in climate archives; models used for climate projections, generally at rather fine resolution and/or a large number of processes, have to be tested compared to paleodata for climates widely different from the current one. In turn, these can be used to improve our understanding of fine (temporal and spatial) scale climate change. The same mutual benefit approach can be followed with theoretical or conceptual models (e.g., to explain the land sea warming ratio in climate changes, cf. section 2.2). It is fundamental to maintain and develop approaches based on this diversity of models, and stay open to new developments. Statistical models, among which those based on machine learning (ML), have been used for downscaling for a long time (e.g., Latombe et al., 2018) but machine learning has also recently allowed for very fast progress in the field of weather forecast, and could yield new types of climate models. For instance, statistical emulators could replace computationally expensive model parts. This raises large interest for computing long term climate changes, including new processes, and running large ensembles thanks to which changes in variability and extremes can be quantified, as well as parametric and forcing uncertainties. ML could also be used for the representation of climate indicators such as isotopes (Wider et al., 2023). Results of PMIP have shown that a model representing present day climate accurately does not necessarily represent properly past climate changes. We therefore strongly recommend that paleoclimate is seen as a stringent test for these ML-based models, developed using large datasets from reanalyses, before they are used to investigate future changes.
Recent advances in paleoclimate data assimilation and in the use of paleodata at the tuning stage of a climate model show some of the multiple advantages of combining models and paleorecords. Paleodata assimilation yields a physically consistent state of the climate system, as close as possible from the known reconstructions. Analyses of the resulting simulations can also shed light on the potential impact of climate extremes, and climate variability, on the recorded signal. In doing so, one has to remember the strong dependency of the result to the single climate model used for the work, in particular far from data sites. Performing paleodata assimilation with several climate models on a key period would therefore be worth doing, in order to quantify the uncertainty related to the chosen model in addition to the one related to uncertainty in the paleo-records themselves.
Developing a consistent framework for the study of past, current and future climate changes and their impacts, at large scales but also regional ones, is therefore essential to build trustworthy tools to anticipate our future. Paleoclimate can help building this trust by testing our understanding and our tools against paleodata. In the coming years, paleoclimates will also be highly relevant to test regional modeling methodologies (based on regional or high-resolution dynamical models or on statistical methods) that are being applied for studying current and future climate change. Such integrated approached will strongly benefit from the framework developed by WCRP, in particular ESMO, Safe Landing Climates and Rifs. For the latter, local to regional paleoclimate information is highly relevant to build narratives on the range of climates that have naturally occurred over a region.
Author contributions
MK: Conceptualization, Supervision, Writing – original draft, Writing – review & editing. PB: Conceptualization, Data curation, Resources, Visualization, Writing – original draft, Writing – review & editing. CC: Conceptualization, Writing – original draft, Writing – review & editing. KR: Conceptualization, Writing – original draft, Writing – review & editing. YA: Conceptualization, Resources, Writing – original draft, Writing – review & editing. MD: Resources, Writing – original draft, Writing – review & editing. BG: Conceptualization, Investigation, Resources, Writing – original draft, Writing – review & editing. AH: Conceptualization, Writing – original draft, Writing – review & editing. M-FL: Conceptualization, Writing – original draft, Writing – review & editing. MH: Conceptualization, Resources, Writing – original draft, Writing – review & editing. KM: Validation, Writing – original draft, Writing – review & editing. PM: Validation, Writing – original draft, Writing – review & editing. BO-B: Conceptualization, Writing – original draft, Writing – review & editing. LP: Writing – original draft, Writing – review & editing. AR: Resources, Visualization, Writing – original draft, Writing – review & editing. AS: Writing – original draft, Writing – review & editing. LS: Conceptualization, Writing – original draft, Writing – review & editing. JZ: Conceptualization, Writing – original draft, Writing – review & editing.
Funding
The author(s) declare that financial support was received for the research, authorship, and/or publication of this article. MK and PB acknowledge the support from the CLIMERI-France research infrastructure as well Agence Nationale de la Recherche - France 2030 as part of the PEPR TRACCS programme under grant number ANR-22-EXTR-0001. CMC acknowledges the financial support from FAPESP (grants 2018/15123-4 and 2019/24349-9) and CNPq (grant 312458/2020-7). JZ and BLO-B acknowledge the support by the National Center for Atmospheric Research (NCAR), which is a major facility sponsored by the National Science Foundation (NSF) under Cooperative Agreement No.1852977. The CESM project was supported primarily by the National Science Foundation (NSF). Computing and data storage resources, including the Cheyenne supercomputer (doi: 10.5065/D6RX99HX), were provided by the Computational and Information Systems Laboratory (CISL) at NCAR. The Past Global Changes (PAGES) project receives support primarily from the Swiss Academy of Sciences and the Chinese Academy of Sciences. KR acknowledges funding through the Deutsche Forschungsgemeinschaft (STACY, grant no. 395588486) and the federal Ministry of Education and Research of Germany (PalMod III, grant nos 01LP2311C, 01LP2310A). Louise Sime is supported by the funding from NERC: The Sensitivity of the West Antarctic Ice Sheet to +2C: SWAIS2C. NE/X009386/1; and Assessing ocean-forced, marine-terminating glacier change in Greenland during climatic warm periods and its impact on marine productivity: KANG-GLAC NE/V006509/1. AR acknowledges funding from the European Research Council (ERC) under the European Union’s Horizon 2020 research and innovation programme (grant agreement n. 802414).
Acknowledgments
The authors thank Lin Chen, from NUIST, and the reviewers along with the editor, for their positive and constructive comments and swift handling of the manuscript.
Conflict of interest
The authors declare that the research was conducted in the absence of any commercial or financial relationships that could be construed as a potential conflict of interest.
Publisher’s note
All claims expressed in this article are solely those of the authors and do not necessarily represent those of their affiliated organizations, or those of the publisher, the editors and the reviewers. Any product that may be evaluated in this article, or claim that may be made by its manufacturer, is not guaranteed or endorsed by the publisher.
References
Abdelkader Di Carlo, I., Braconnot, P., Carré, M., Elliot, M., and Marti, O. (2023). Different methods in assessing El Niño flavors Lead to opposite results. Geophys. Res. Lett. 50:e2023GL104558. doi: 10.1029/2023GL104558
Ait Brahim, Y., Sha, L., Wassenburg, J. A., Azennoud, K., Cheng, H., Cruz, F. W., et al. (2023). The spatiotemporal extent of the green Sahara during the last glacial period. iScience 26:107018. doi: 10.1016/j.isci.2023.107018
Akabane, T. K., Chiessi, C. M., Hirota, M., Bouimetarhan, I., Prange, M., Mulitza, S., et al. (2024). Weaker Atlantic overturning circulation increases the vulnerability of northern Amazon forests. Nat. Geosci. doi: 10.1038/s41561-024-01578-z
Alvarez-Solas, J., Charbit, S., Ritz, C., Paillard, D., Ramstein, G., and Dumas, C. (2010). Links between ocean temperature and iceberg discharge during Heinrich events. Nat. Geosci. 3, 122–126. doi: 10.1038/ngeo752
An, S.-I., and Choi, J. (2014). Mid-Holocene tropical Pacific climate state, annual cycle, and ENSO in PMIP2 and PMIP3. Clim. Dyn. 43, 957–970. doi: 10.1007/s00382-013-1880-z
Arias, P. A., Bellouin, N., Coppola, E., Jones, R. G., Krinner, G., Marotzke, J., et al. (2021). “Technical summary” in Climate change 2021: The physical science basis. Contribution of working group I to the sixth assessment report of the intergovernmental panel on climate change. eds. V. Masson-Delmotte, P. Zhai, A. Pirani, S. L. Connors, C. Péan, and S. Berger, et al. (Cambridge, United Kingdom and New York, NY, USA: Cambridge University Press), 33–144.
Aschwanden, A., Fahnestock, M. A., and Truffer, M. (2016). Complex Greenland outlet glacier flow captured. Nat. Commun. 7:10524. doi: 10.1038/ncomms10524
Askjær, T. G., Zhang, Q., Schenk, F., Ljungqvist, F. C., Lu, Z., Brierley, C. M., et al. (2022). Multi-centennial Holocene climate variability in proxy records and transient model simulations. Quat. Sci. Rev. 296:107801. doi: 10.1016/j.quascirev.2022.107801
Atsawawaranunt, K., Comas-Bru, L., Amirnezhad Mozhdehi, S., Deininger, M., Harrison, S. P., Baker, A., et al. (2018). The SISAL database: a global resource to document oxygen and carbon isotope records from speleothems. Earth Syst. Sci. Data 10, 1687–1713. doi: 10.5194/essd-10-1687-2018
Bamber, J. L., Riva, R. E. M., Vermeersen, B. L. A., and LeBrocq, A. M. (2009). Reassessment of the potential sea-level rise from a collapse of the West Antarctic ice sheet. Science 324, 901–903. doi: 10.1126/science.1169335
Bellomo, K., Angeloni, M., Corti, S., and Von Hardenberg, J. (2021). Future climate change shaped by inter-model differences in Atlantic meridional overturning circulation response. Nat. Commun. 12:3659. doi: 10.1038/s41467-021-24015-w
Bellprat, O., and Doblas-Reyes, F. (2016). Attribution of extreme weather and climate events overestimated by unreliable climate simulations. Geophys. Res. Lett. 43, 2158–2164. doi: 10.1002/2015GL067189
Ben-Yami, M., Good, P., Jackson, L. C., Crucifix, M., Hu, A., Saenko, O., et al. (2024). Impacts of AMOC collapse on monsoon rainfall: a multi-model comparison. Earth’s Future 12:e2023EF003959. doi: 10.1029/2023EF003959
Birkett, B. A., Obrist-Farner, J., Rice, P. M., Parker, W. G., Douglas, P. M. J., Berke, M. A., et al. (2023). Preclassic environmental degradation of Lake Petén Itzá, Guatemala, by the early Maya of Nixtun-Ch’ich’. Commun. Earth Environ. 4:59. doi: 10.1038/s43247-023-00726-4
Bond, G., Heinrich, H., Broecker, W., Labeyrie, L., McManus, J., Andrews, J., et al. (1992). Evidence for massive discharges of icebergs into the North Atlantic Ocean during the last glacial period. Nature 360, 245–249. doi: 10.1038/360245a0
Braconnot, P., Crétat, J., Marti, O., Balkanski, Y., Caubel, A., Cozic, A., et al. (2019). Impact of multiscale variability on last 6,000 years Indian and West African monsoon rain. Geophys. Res. Lett. 46, 14021–14029. doi: 10.1029/2019GL084797
Braconnot, P., Otto-Bliesner, B., Harrison, S., Joussaume, S., Peterchmitt, J.-Y., Abe-Ouchi, A., et al. (2007). Results of PMIP2 coupled simulations of the mid-Holocene and last glacial maximum – part 1: experiments and large-scale features. Clim. Past 3, 261–277. doi: 10.5194/cp-3-261-2007
Broecker, W., Bond, G., Klas, M., Clark, E., and McManus, J. (1992). Origin of the northern Atlantic’s Heinrich events. Clim. Dyn. 6, 265–273. doi: 10.1007/BF00193540
Brown, J. R., Brierley, C. M., An, S.-I., Guarino, M.-V., Stevenson, S., Williams, C. J. R., et al. (2020). Comparison of past and future simulations of ENSO in CMIP5/PMIP3 and CMIP6/PMIP4 models. Clim. Past 16, 1777–1805. doi: 10.5194/cp-16-1777-2020
Burke, A., Peros, M. C., Wren, C. D., Pausata, F. S. R., Riel-Salvatore, J., Moine, O., et al. (2021). The archaeology of climate change: the case for cultural diversity. Proc. Natl. Acad. Sci. USA 118:e2108537118. doi: 10.1073/pnas.2108537118
Burls, N., and Sagoo, N. (2022). Increasingly sophisticated climate models need the out-of-sample tests paleoclimates provide. J. Adv. Model Earth Syst. 14:e2022MS003389. doi: 10.1029/2022MS003389
Byrne, M. P., and O’Gorman, P. A. (2018). Trends in continental temperature and humidity directly linked to ocean warming. Proc. Natl. Acad. Sci. USA 115, 4863–4868. doi: 10.1073/pnas.1722312115
Campos, M. C., Chiessi, C. M., Prange, M., Mulitza, S., Kuhnert, H., Paul, A., et al. (2019). A new mechanism for millennial scale positive precipitation anomalies over tropical South America. Quat. Sci. Rev. 225:105990. doi: 10.1016/j.quascirev.2019.105990
Campos, M. C., Chiessi, C. M., Venancio, I. M., Pinho, T. M. L., Crivellari, S., Kuhnert, H., et al. (2020). Constraining millennial-scale changes in northern component water ventilation in the Western tropical South Atlantic. Paleoceanog Paleoclimatol 35:e2020PA003876. doi: 10.1029/2020PA003876
CAPE-Last Interglacial Project Members (2006). Last interglacial Arctic warmth confirms polar amplification of climate change. Quat. Sci. Rev. 25, 1383–1400. doi: 10.1016/j.quascirev.2006.01.033
Capotondi, A., Wittenberg, A. T., Newman, M., Di Lorenzo, E., Yu, J.-Y., Braconnot, P., et al. (2015). Understanding ENSO Diversity. Bull. Am. Meteorol. Soc. 96, 921–938. doi: 10.1175/BAMS-D-13-00117.1
Carré, M., Braconnot, P., Elliot, M., d’Agostino, R., Schurer, A., Shi, X., et al. (2021). High-resolution marine data and transient simulations support orbital forcing of ENSO amplitude since the mid-Holocene. Quat. Sci. Rev. 268:107125. doi: 10.1016/j.quascirev.2021.107125
Chen, L., Zheng, W., and Braconnot, P. (2019). Towards understanding the suppressed ENSO activity during mid-Holocene in PMIP2 and PMIP3 simulations. Clim. Dyn. 53, 1095–1110. doi: 10.1007/s00382-019-04637-z
Church, J. A., Clark, P. U., Cazenave, A., Gregory, J. M., Jevrejeva, S., Levermann, A., et al. (2013) in Sea Level Change. In: Climate Change 2013: The Physical Science Basis. Contribution of Working Group I to the Fifth Assessment Report of the Intergovernmental Panel on Climate Change. eds. T. F. Stocker, D. Qin, G.-K. Plattner, M. Tignor, S. K. Allen, and J. Boschung, et al. (Cambridge, United Kingdom and New York, NY, USA: Cambridge University Press).
Colville, E. J., Carlson, A. E., Beard, B. L., Hatfield, R. G., Stoner, J. S., Reyes, A. V., et al. (2011). Sr-Nd-Pb isotope evidence for ice-sheet presence on southern Greenland during the last interglacial. Science 333, 620–623. doi: 10.1126/science.1204673
Cook, E. R., Seager, R., Heim, R. R., Vose, R. S., Herweijer, C., and Woodhouse, C. (2010). Megadroughts in North America: placing IPCC projections of hydroclimatic change in a long-term palaeoclimate context. J Quater Sci 25, 48–61. doi: 10.1002/jqs.1303
Cooper, V. T., Armour, K. C., Hakim, G. J., Tierney, J. E., Osman, M. B., Proistosescu, C., et al. (2024). Last glacial maximum pattern effects reduce climate sensitivity estimates. Sci. Adv. 10:eadk9461. doi: 10.1126/sciadv.adk9461
Crétat, J., Harrison, S. P., Braconnot, P., d’Agostino, R., Jungclaus, J., Lohmann, G., et al. (2024). Orbitally forced and internal changes in West African rainfall interannual-to-decadal variability for the last 6000 years. Clim. Dyn. 62, 2301–2316. doi: 10.1007/s00382-023-07023-y
D’Anjou, R. M., Bradley, R. S., Balascio, N. L., and Finkelstein, D. B. (2012). Climate impacts on human settlement and agricultural activities in northern Norway revealed through sediment biogeochemistry. Proc. Natl. Acad. Sci. USA 109, 20332–20337. doi: 10.1073/pnas.1212730109
De Boer, B., Lourens, L. J., and Van De Wal, R. S. W. (2014). Persistent 400,000-year variability of Antarctic ice volume and the carbon cycle is revealed throughout the Plio-Pleistocene. Nat. Commun. 5:2999. doi: 10.1038/ncomms3999
De Boer, B., Van De Wal, R. S. W., Bintanja, R., Lourens, L. J., and Tuenter, E. (2010). Cenozoic global ice-volume and temperature simulations with 1-D ice-sheet models forced by benthic δ 18 O records. Ann. Glaciol. 51, 23–33. doi: 10.3189/172756410791392736
De Vernal, A., and Hillaire-Marcel, C. (2008). Natural variability of Greenland climate, vegetation, and ice volume during the past million years. Science 320, 1622–1625. doi: 10.1126/science.1153929
Diamond, R., Schroeder, D., Sime, L. C., Ridley, J., and Feltham, D. (2024). The significance of the melt-pond scheme in a CMIP6 global climate model. J. Clim. 37, 249–268. doi: 10.1175/JCLI-D-22-0902.1
Diamond, R., Sime, L. C., Schroeder, D., and Guarino, M.-V. (2021). The contribution of melt ponds to enhanced Arctic Sea-ice melt during the last interglacial. Cryosphere 15, 5099–5114. doi: 10.5194/tc-15-5099-2021
Ding, X., Bassinot, F., Guichard, F., and Fang, N. Q. (2013). Indonesian Throughflow and monsoon activity records in the Timor Sea since the last glacial maximum. Mar. Micropaleontol. 101, 115–126. doi: 10.1016/j.marmicro.2013.02.003
Ditlevsen, P., and Ditlevsen, S. (2023). Warning of a forthcoming collapse of the Atlantic meridional overturning circulation. Nat. Commun. 14:4254. doi: 10.1038/s41467-023-39810-w
Dütsch, M., Steig, E. J., Blossey, P. N., and Pauling, A. G. (2023). Response of water isotopes in precipitation to a collapse of the West Antarctic ice sheet in high-resolution simulations with the weather research and forecasting model. J. Clim. 36, 5417–5430. doi: 10.1175/JCLI-D-22-0647.1
Easterling, D. R., Kunkel, K. E., Wehner, M. F., and Sun, L. (2016). Detection and attribution of climate extremes in the observed record. Weather Climate Extremes 11, 17–27. doi: 10.1016/j.wace.2016.01.001
Elderfield, H., Ferretti, P., Greaves, M., Crowhurst, S., McCave, I. N., Hodell, D., et al. (2012). Evolution of ocean temperature and ice volume through the mid-Pleistocene climate transition. Science 337, 704–709. doi: 10.1126/science.1221294
Emile-Geay, J., Cobb, K. M., Carré, M., Braconnot, P., Leloup, J., Zhou, Y., et al. (2016). Links between tropical Pacific seasonal, interannual and orbital variability during the Holocene. Nat. Geosci. 9, 168–173. doi: 10.1038/ngeo2608
Essell, H., Krusic, P. J., Esper, J., Wagner, S., Braconnot, P., Jungclaus, J., et al. (2023). A frequency-optimised temperature record for the Holocene. Environ. Res. Lett. 18:114022. doi: 10.1088/1748-9326/ad0065
Falasca, F., Crétat, J., Bracco, A., Braconnot, P., and Marti, O. (2022). Climate change in the indo-Pacific basin from mid- to late Holocene. Clim. Dyn. 59, 753–766. doi: 10.1007/s00382-022-06153-z
Falasca, F., Crétat, J., Braconnot, P., and Bracco, A. (2020). Spatiotemporal complexity and time-dependent networks in sea surface temperature from mid- to late Holocene. Eur. Phys. J. Plus 135:392. doi: 10.1140/epjp/s13360-020-00403-x
Fan, W., Jian, Z., Bassinot, F., and Chu, Z. (2013). Holocene centennial-scale changes of the Indonesian and South China Sea throughflows: evidences from the Makassar Strait. Glob. Planet. Chang. 111, 111–117. doi: 10.1016/j.gloplacha.2013.08.017
Fan, W., Jian, Z., Chu, Z., Dang, H., Wang, Y., Bassinot, F., et al. (2018). Variability of the Indonesian Throughflow in the Makassar Strait over the last 30 ka. Sci. Rep. 8:5678. doi: 10.1038/s41598-018-24055-1
Feng, M., Zhang, X., Sloyan, B., and Chamberlain, M. (2017). Contribution of the deep ocean to the centennial changes of the Indonesian Throughflow. Geophys. Res. Lett. 44, 2859–2867. doi: 10.1002/2017GL072577
Fettweis, X., Franco, B., Tedesco, M., Van Angelen, J. H., Lenaerts, J. T. M., Van Den Broeke, M. R., et al. (2013). Estimating the Greenland ice sheet surface mass balance contribution to future sea level rise using the regional atmospheric climate model MAR. Cryosphere 7, 469–489. doi: 10.5194/tc-7-469-2013
Fox-Kemper, B., Hewitt, H. T., Xiao, C., Aðalgeirsdóttir, G., Drijfhout, S. S., Edwards, T. L., et al. (2021). “Ocean, cryosphere and sea level change” in Climate change 2021: The physical science basis. Contribution of working group I to the sixth assessment report of the intergovernmental panel on climate change. eds. V. Masson-Delmotte, P. Zhai, A. Pirani, S. L. Connors, C. Péan, and S. Berger, et al. (Cambridge, United Kingdom and New York, NY, USA: Cambridge University Press), 1211–1362.
Fredriksen, H., Berner, J., Subramanian, A. C., and Capotondi, A. (2020). How does El Niño–southern oscillation change under global warming—a first look at CMIP6. Geophys. Res. Lett. 47:e2020GL090640. doi: 10.1029/2020GL090640
Freund, M. B., Brown, J. R., Henley, B. J., Karoly, D. J., and Brown, J. N. (2020). Warming patterns affect El Niño diversity in CMIP5 and CMIP6 models. J. Clim. 33, 8237–8260. doi: 10.1175/JCLI-D-19-0890.1
Fudge, T. J., Hills, B. H., Horlings, A. N., Holschuh, N., Christian, J. E., Davidge, L., et al. (2023). A site for deep ice coring at West Hercules dome: results from ground-based geophysics and modeling. J. Glaciol. 69, 538–550. doi: 10.1017/jog.2022.80
Gordon, A. L., Napitu, A., Huber, B. A., Gruenburg, L. K., Pujiana, K., Agustiadi, T., et al. (2019). Makassar Strait Throughflow seasonal and interannual variability: An overview. JGR Oceans 124, 3724–3736. doi: 10.1029/2018JC014502
Goursaud, S., Holloway, M., Sime, L., Wolff, E., Valdes, P., Steig, E. J., et al. (2021). Antarctic ice sheet elevation impacts on water isotope records during the last interglacial. Geophys. Res. Lett. 48:e2020GL091412. doi: 10.1029/2020GL091412
Gowan, E. J., Zhang, X., Khosravi, S., Rovere, A., Stocchi, P., Hughes, A. L. C., et al. (2021). A new global ice sheet reconstruction for the past 80 000 years. Nat. Commun. 12:1199. doi: 10.1038/s41467-021-21469-w
Grant, K. M., Rohling, E. J., Ramsey, C. B., Cheng, H., Edwards, R. L., Florindo, F., et al. (2014). Sea-level variability over five glacial cycles. Nat. Commun. 5:5076. doi: 10.1038/ncomms6076
Guarino, M.-V., Sime, L. C., Schröeder, D., Malmierca-Vallet, I., Rosenblum, E., Ringer, M., et al. (2020). Sea-ice-free Arctic during the last interglacial supports fast future loss. Nat. Clim. Chang. 10, 928–932. doi: 10.1038/s41558-020-0865-2
Harrison, S. P., Bartlein, P. J., Izumi, K., Li, G., Annan, J., Hargreaves, J., et al. (2015). Evaluation of CMIP5 palaeo-simulations to improve climate projections. Nature Clim Change 5, 735–743. doi: 10.1038/nclimate2649
Hendrizan, M., Kuhnt, W., and Holbourn, A. (2017). Variability of Indonesian Throughflow and Borneo runoff during the last 14 kyr. Paleoceanography 32, 1054–1069. doi: 10.1002/2016PA003030
Hendrizan, M., Kuhnt, W., Holbourn, A., Cahyarini, S. Y., and Ningsih, N. S. (2023). Kalimantan hydroclimate variability since the last glacial period. Int J Earth Sci 112, 615–629. doi: 10.1007/s00531-022-02266-2
Henry, L. G., McManus, J. F., Curry, W. B., Roberts, N. L., Piotrowski, A. M., and Keigwin, L. D. (2016). North Atlantic Ocean circulation and abrupt climate change during the last glaciation. Science 353, 470–474. doi: 10.1126/science.aaf5529
Holloway, M. D., Sime, L. C., Singarayer, J. S., Tindall, J. C., Bunch, P., and Valdes, P. J. (2016). Antarctic last interglacial isotope peak in response to sea ice retreat not ice-sheet collapse. Nat. Commun. 7:12293. doi: 10.1038/ncomms12293
Hopcroft, P. O., and Valdes, P. J. (2015). Last glacial maximum constraints on the earth system model HadGEM2-ES. Clim. Dyn. 45, 1657–1672. doi: 10.1007/s00382-014-2421-0
Hu, D., Wu, L., Cai, W., Sen Gupta, A., Ganachaud, A., Qiu, B., et al. (2015). Pacific western boundary currents and their roles in climate. Nature 522, 299–308. doi: 10.1038/nature14504
Hutchinson, D. K., Menviel, L., Meissner, K. J., and Hogg, A. M. C. (2024). East Antarctic warming forced by ice loss during the last interglacial. Nat. Commun. 15:1026. doi: 10.1038/s41467-024-45501-x
IPCC (2022) in Climate change 2022: impacts, adaptation, and vulnerability. Contribution of working group II to the sixth assessment report of the intergovernmental panel on climate change. eds. H.-O. Pörtner, D. C. Roberts, M. Tignor, E. S. Poloczanska, K. Mintenbeck, and A. Alegría, et al. (Cambridge, UK and New York, NY, USA: Cambridge University Press), 3056.
Ivanovic, R. F., Gregoire, L. J., Kageyama, M., Roche, D. M., Valdes, P. J., Burke, A., et al. (2016). Transient climate simulations of the deglaciation 21–9 thousand years before present (version 1) – PMIP4 Core experiment design and boundary conditions. Geosci. Model Dev. 9, 2563–2587. doi: 10.5194/gmd-9-2563-2016
Jebri, B., and Khodri, M. (2023). Large ensemble particle filter for spatial climate reconstructions using a linear inverse model. J Adv Model Earth Syst 15:e2022MS003094. doi: 10.1029/2022MS003094
Kageyama, M., Braconnot, P., Harrison, S. P., Haywood, A. M., Jungclaus, J. H., Otto-Bliesner, B. L., et al. (2018). The PMIP4 contribution to CMIP6 – part 1: overview and over-arching analysis plan. Geosci. Model Dev. 11, 1033–1057. doi: 10.5194/gmd-11-1033-2018
Kageyama, M., Harrison, S. P., Kapsch, M.-L., Lofverstrom, M., Lora, J. M., Mikolajewicz, U., et al. (2021a). The PMIP4 last glacial maximum experiments: preliminary results and comparison with the PMIP3 simulations. Clim. Past 17, 1065–1089. doi: 10.5194/cp-17-1065-2021
Kageyama, M., Sime, L. C., Sicard, M., Guarino, M.-V., De Vernal, A., Stein, R., et al. (2021b). A multi-model CMIP6-PMIP4 study of Arctic Sea ice at 127 ka: sea ice data compilation and model differences. Clim. Past 17, 37–62. doi: 10.5194/cp-17-37-2021
Keenan, B., Imfeld, A., Johnston, K., Breckenridge, A., Gélinas, Y., and Douglas, P. M. J. (2021). Molecular evidence for human population change associated with climate events in the Maya lowlands. Quat. Sci. Rev. 258:106904. doi: 10.1016/j.quascirev.2021.106904
Latombe, G., Burke, A., Vrac, M., Levavasseur, G., Dumas, C., Kageyama, M., et al. (2018). Comparison of spatial downscaling methods of general circulation model results to study climate variability during the last glacial maximum. Geosci. Model Dev. 11, 2563–2579. doi: 10.5194/gmd-11-2563-2018
Lenton, T. M., Armstrong McKay, D. I., Loriani, S., Abrams, J. F., Lade, S. J., Donges, J. F., et al. (Eds.) (2023). The Global Tipping Points Report 2023. Exeter, UK: University of Exeter. Available at: https://global-tipping-points.org/resources-gtp/.
Li, X., Liu, S., Ji, K., Hou, X., Yuan, K., Hou, J., et al. (2024). Late Holocene human population change revealed by fecal stanol records and its response to environmental evolution at Xiada co on the western Tibetan plateau. Palaeogeogr. Palaeoclimatol. Palaeoecol. 636:111993. doi: 10.1016/j.palaeo.2023.111993
Lisiecki, L. E., and Raymo, M. E. (2005). A Pliocene-Pleistocene stack of 57 globally distributed benthic δ 18 O records. Paleoceanography 20:2004PA001071. doi: 10.1029/2004PA001071
Lu, Z., Liu, Z., Zhu, J., and Cobb, K. M. (2018). A review of paleo El Niño-southern oscillation. Atmos. 9:130. doi: 10.3390/atmos9040130
Luan, Y., Braconnot, P., Yu, Y., Zheng, W., and Marti, O. (2012). Early and mid-Holocene climate in the tropical Pacific: seasonal cycle and interannual variability induced by insolation changes. Clim. Past 8, 1093–1108. doi: 10.5194/cp-8-1093-2012
Luo, X., Dee, S., Stevenson, S., Okumura, Y., Steiger, N., and Parsons, L. (2022). Last millennium ENSO diversity and north American teleconnections: new insights from paleoclimate data assimilation. Paleoceanog Paleoclimatol 37:e2021PA004283. doi: 10.1029/2021PA004283
Malmierca-Vallet, I., and Sime, L. C.and the D–O community members. (2023). Dansgaard–Oeschger events in climate models: review and baseline marine isotope stage 3 (MIS3) protocol. Clim. Past 19, 915–942. doi: 10.5194/cp-19-915-2023
Malmierca-Vallet, I., Sime, L. C., Tindall, J. C., Capron, E., Valdes, P. J., Vinther, B. M., et al. (2018). Simulating the last interglacial Greenland stable water isotope peak: the role of Arctic Sea ice changes. Quat. Sci. Rev. 198, 1–14. doi: 10.1016/j.quascirev.2018.07.027
Malmierca-Vallet, I., Sime, L. C., Valdes, P. J., Klockmann, M., Vettoretti, G., and Slattery, J. (2024). The impact of CO 2 and climate state on whether Dansgaard–Oeschger type oscillations occur in climate models. Geophys. Res. Lett. 51:e2024GL110068. doi: 10.1029/2024GL110068
Manabe, S., and Broccoli, A. J. (1985). A comparison of climate model sensitivity with data from the last glacial maximum. J. Atmos. Sci. 42, 2643–2651. doi: 10.1175/1520-0469(1985)042<2643:ACOCMS>2.0.CO;2
Masson-Delmotte, V., Buiron, D., Ekaykin, A., Frezzotti, M., Gallée, H., Jouzel, J., et al. (2011). A comparison of the present and last interglacial periods in six Antarctic ice cores. Clim. Past 7, 397–423. doi: 10.5194/cp-7-397-2011
Max, L., Nürnberg, D., Chiessi, C. M., Lenz, M. M., and Mulitza, S. (2022). Subsurface Ocean warming preceded Heinrich events. Nat. Commun. 13:4217. doi: 10.1038/s41467-022-31754-x
Menviel, L., Govin, A., Avenas, A., Meissner, K. J., Grant, K. M., and Tzedakis, P. C. (2021). Drivers of the evolution and amplitude of African humid periods. Commun Earth Environ 2:237. doi: 10.1038/s43247-021-00309-1
Michel, S. L. L., Swingedouw, D., Ortega, P., Gastineau, G., Mignot, J., McCarthy, G., et al. (2022). Early warning signal for a tipping point suggested by a millennial Atlantic multidecadal variability reconstruction. Nat. Commun. 13:5176. doi: 10.1038/s41467-022-32704-3
Miller, K. G., Browning, J. V., Schmelz, W. J., Kopp, R. E., Mountain, G. S., and Wright, J. D. (2020). Cenozoic Sea-level and cryospheric evolution from deep-sea geochemical and continental margin records. Sci. Adv. 6:eaaz1346. doi: 10.1126/sciadv.aaz1346
Miller, K. G., Wright, J. D., Browning, J. V., Kulpecz, A., Kominz, M., Naish, T. R., et al. (2012). High tide of the warm Pliocene: implications of global sea level for Antarctic deglaciation. Geology 40, 407–410. doi: 10.1130/G32869.1
Mouginot, J., Rignot, E., Bjørk, A. A., Van Den Broeke, M., Millan, R., Morlighem, M., et al. (2019). Forty-six years of Greenland ice sheet mass balance from 1972 to 2018. Proc. Natl. Acad. Sci. USA 116, 9239–9244. doi: 10.1073/pnas.1904242116
Mulitza, S., Chiessi, C. M., Schefuß, E., Lippold, J., Wichmann, D., Antz, B., et al. (2017). Synchronous and proportional deglacial changes in Atlantic meridional overturning and northeast Brazilian precipitation. Paleoceanography 32, 622–633. doi: 10.1002/2017PA003084
Mulvaney, R., Rix, J., Polfrey, S., Grieman, M., Martìn, C., Nehrbass-Ahles, C., et al. (2021). Ice drilling on Skytrain ice rise and Sherman Island, Antarctica. Ann. Glaciol. 62, 311–323. doi: 10.1017/aog.2021.7
Muntjewerf, L., Petrini, M., Vizcaino, M., Ernani Da Silva, C., Sellevold, R., Scherrenberg, M. D. W., et al. (2020). Greenland ice sheet contribution to 21st Century Sea level rise as simulated by the coupled CESM2.1-CISM2.1. Geophys. Res. Lett. 47:e2019GL086836. doi: 10.1029/2019GL086836
Naish, T., Powell, R., Levy, R., Wilson, G., Scherer, R., Talarico, F., et al. (2009). Obliquity-paced Pliocene West Antarctic ice sheet oscillations. Nature 458, 322–328. doi: 10.1038/nature07867
Neukom, R., Steiger, N., Gómez-Navarro, J. J., Wang, J., and Werner, J. P. (2019). No evidence for globally coherent warm and cold periods over the preindustrial common era. Nature 571, 550–554. doi: 10.1038/s41586-019-1401-2
Notz, D.SIMIP Community (2020). Arctic Sea ice in CMIP6. Geophys. Res. Lett. 47:e2019GL086749. doi: 10.1029/2019GL086749
Nowicki, S., and Seroussi, H.. (2018). Projections of future sea level contributions from the Greenland and Antarctic Ice Sheets: Challenges beyond dynamical ice sheet modeling. Oceanography 31, doi: 10.5670/oceanog.2018.216
Otto-Bliesner, B. L., Braconnot, P., Harrison, S. P., Lunt, D. J., Abe-Ouchi, A., Albani, S., et al. (2017). The PMIP4 contribution to CMIP6 – part 2: two interglacials, scientific objective and experimental design for Holocene and last interglacial simulations. Geosci. Model Dev. 10, 3979–4003. doi: 10.5194/gmd-10-3979-2017
Pausata, F. S. R., Gaetani, M., Messori, G., Berg, A., Maia De Souza, D., Sage, R. F., et al. (2020). The greening of the Sahara: past changes and future implications. One Earth 2, 235–250. doi: 10.1016/j.oneear.2020.03.002
Renoult, M., Annan, J. D., Hargreaves, J. C., Sagoo, N., Flynn, C., Kapsch, M.-L., et al. (2020). A Bayesian framework for emergent constraints: case studies of climate sensitivity with PMIP. Clim. Past 16, 1715–1735. doi: 10.5194/cp-16-1715-2020
Renoult, M., Sagoo, N., Zhu, J., and Mauritsen, T. (2023). Causes of the weak emergent constraint on climate sensitivity at the last glacial maximum. Clim. Past 19, 323–356. doi: 10.5194/cp-19-323-2023
Rohling, E. J., Foster, G. L., Grant, K. M., Marino, G., Roberts, A. P., Tamisiea, M. E., et al. (2014). Sea-level and deep-sea-temperature variability over the past 5.3 million years. Nature 508, 477–482. doi: 10.1038/nature13230
Santos, T. P., Shimizu, M. H., Nascimento, R. A., Venancio, I. M., Campos, M. C., Portilho-Ramos, R. C., et al. (2022). A data-model perspective on the Brazilian margin surface warming from the last glacial maximum to the Holocene. Quat. Sci. Rev. 286:107557. doi: 10.1016/j.quascirev.2022.107557
Seltzer, A. M., Blard, P.-H., Sherwood, S. C., and Kageyama, M. (2023). Terrestrial amplification of past, present, and future climate change. Sci. Adv. 9:eadf8119. doi: 10.1126/sciadv.adf8119
Seneviratne, S. I., Zhang, X., Adnan, M., Badi, W., Dereczynski, C., Di Luca, A., et al. (2021) in Weather and Climate Extreme Events in a Changing Climate. In Climate Change 2021: The Physical Science Basis. Contribution of Working Group I to the Sixth Assessment Report of the Intergovernmental Panel on Climate Change. eds. V. Masson-Delmotte, P. Zhai, A. Pirani, S. L. Connors, C. Péan, and S. Berger, et al. (Cambridge, United Kingdom and New York, NY, USA: Cambridge University Press), 1513–1766. doi: 10.1017/9781009157896.013
Sen Gupta, A., McGregor, S., van Sebille, E., Ganachaud, A., Brown, J. N., and Santoso, A. (2016). Future changes to the Indonesian Throughflow and Pacific circulation: The differing role of wind and deep circulation changes. Geophys. Res. Lett. 43, 1669–1678. doi: 10.1002/2016GL067757
Sha, L., Ait Brahim, Y., Wassenburg, J. A., Yin, J., Peros, M., Cruz, F. W., et al. (2019). How far north did the African monsoon fringe expand during the African humid period? Insights from southwest Moroccan speleothems. Geophys. Res. Lett. 46, 14093–14102. doi: 10.1029/2019GL084879
Sherwood, S. C., Webb, M. J., Annan, J. D., Armour, K. C., Forster, P. M., Hargreaves, J. C., et al. (2020). An assessment of Earth’s climate sensitivity using multiple lines of evidence. Rev. Geophys. 58:e2019RG000678. doi: 10.1029/2019RG000678
Sime, L. C., Sivankutty, R., Vallet-Malmierca, I., De Boer, A. M., and Sicard, M. (2023). Summer surface air temperature proxies point to near-sea-ice-free conditions in the Arctic at 127 ka. Clim. Past 19, 883–900. doi: 10.5194/cp-19-883-2023
Sommers, A. N., Otto-Bliesner, B. L., Lipscomb, W. H., Lofverstrom, M., Shafer, S. L., Bartlein, P. J., et al. (2021). Retreat and regrowth of the Greenland ice sheet during the last interglacial as simulated by the CESM2-CISM2 coupled climate–ice sheet model. Paleoceanog Paleoclimatol 36:e2021PA004272. doi: 10.1029/2021PA004272
Song, M.-E., and Chen, L. (2022). Responses of tropical background state and ENSO behaviors to mid-Holocene forcing simulated by PMIP3 and PMIP4 models. Front. Earth Sci. 10:853577. doi: 10.3389/feart.2022.853577
Sosdian, S., and Rosenthal, Y. (2009). Deep-Sea temperature and ice volume changes across the Pliocene-Pleistocene climate transitions. Science 325, 306–310. doi: 10.1126/science.1169938
Spratt, R. M., and Lisiecki, L. E. (2016). A Late Pleistocene sea level stack. Clim. Past 12, 1079–1092. doi: 10.5194/cp-12-1079-2016
Sprintall, J., Gordon, A. L., Wijffels, S. E., Feng, M., Hu, S., Koch-Larrouy, A., et al. (2019). Detecting change in the Indonesian seas. Front. Mar. Sci. 6:257. doi: 10.3389/fmars.2019.00257
Steig, E. J., Huybers, K., Singh, H. A., Steiger, N. J., Ding, Q., Frierson, D. M. W., et al. (2015). Influence of West Antarctic ice sheet collapse on Antarctic surface climate. Geophys. Res. Lett. 42, 4862–4868. doi: 10.1002/2015GL063861
Sun, S., and Thompson, A. F. (2020). Centennial changes in the Indonesian Throughflow connected to the Atlantic meridional overturning circulation: the Ocean’s transient Conveyor Belt. Geophys. Res. Lett. 47:e2020GL090615. doi: 10.1029/2020GL090615
Susanto, R. D., and Gordon, A. L. (2005). Velocity and transport of the Makassar Strait throughflow. J. Geophys. Res. 110:2004JC002425. doi: 10.1029/2004JC002425
Tierney, J. E., Zhu, J., King, J., Malevich, S. B., Hakim, G. J., and Poulsen, C. J. (2020). Glacial cooling and climate sensitivity revisited. Nature 584, 569–573. doi: 10.1038/s41586-020-2617-x
Vermassen, F., O’Regan, M., De Boer, A., Schenk, F., Razmjooei, M., West, G., et al. (2023). A seasonally ice-free Arctic Ocean during the last interglacial. Nat. Geosci. 16, 723–729. doi: 10.1038/s41561-023-01227-x
White, A. J., Stevens, L. R., Lorenzi, V., Munoz, S. E., Lipo, C. P., and Schroeder, S. (2018). An evaluation of fecal stanols as indicators of population change at Cahokia, Illinois. J. Archaeol. Sci. 93, 129–134. doi: 10.1016/j.jas.2018.03.009
Wider, J., Kruse, J., Weitzel, N., Bühler, J. C., Köthe, U., and Rehfeld, K. (2023). Towards learned emulation of interannual water isotopologue variations in General Circulation Models. Environmental Data Science. 2:e35. doi: 10.1017/eds.2023.29
Williams, A. P., Cook, E. R., Smerdon, J. E., Cook, B. I., Abatzoglou, J. T., Bolles, K., et al. (2020). Large contribution from anthropogenic warming to an emerging north American megadrought. Science 368, 314–318. doi: 10.1126/science.aaz9600
Wogau, K. H., Keenan, B., Arz, H. W., and Böhnel, H. N. (2023). Paleoenvironmental study of the late Preclassic period in the northern Mesoamerican frontier. The Holocene 33, 1291–1303. doi: 10.1177/09596836231185828
Wolff, E., Mulvaney, R., Grieman, M., Hoffmann, H., Humby, J., Nehrbass-Ahles, C., et al. (2024). The Ronne Ice Shelf survived the last interglacial. Nature, accepted.
Wunderling, N., Von Der Heydt, A. S., Aksenov, Y., Barker, S., Bastiaansen, R., Brovkin, V., et al. (2024). Climate tipping point interactions and cascades: a review. Earth Syst. Dynam. 15, 41–74. doi: 10.5194/esd-15-41-2024
Yau, A. M., Bender, M. L., Robinson, A., and Brook, E. J. (2016). Reconstructing the last interglacial at summit, Greenland: insights from GISP2. Proc. Natl. Acad. Sci. USA 113, 9710–9715. doi: 10.1073/pnas.1524766113
Zhang, X., Atwood, A. R., Nag, B., and Cobb, K. M. (2022). The tropical Pacific annual cycle and ENSO in PMIP4 simulations of the mid-Holocene. JGR Oceans 127:e2021JC017587. doi: 10.1029/2021JC017587
Zhang, Y., Chiessi, C. M., Mulitza, S., Sawakuchi, A. O., Häggi, C., Zabel, M., et al. (2017). Different precipitation patterns across tropical South America during Heinrich and Dansgaard-Oeschger stadials. Quat. Sci. Rev. 177, 1–9. doi: 10.1016/j.quascirev.2017.10.012
Zhang, P., Xu, J., Schröder, J. F., Holbourn, A., Kuhnt, W., Kochhann, K. G. D., et al. (2018). Variability of the Indonesian Throughflow thermal profile over the last 25-kyr: a perspective from the southern Makassar Strait. Glob. Planet. Chang. 169, 214–223. doi: 10.1016/j.gloplacha.2018.08.003
Zhou, Y., and McManus, J. F. (2024). Heinrich event ice discharge and the fate of the Atlantic meridional overturning circulation. Science 384, 983–986. doi: 10.1126/science.adh8369
Zhu, J., Otto-Bliesner, B. L., Brady, E. C., Gettelman, A., Bacmeister, J. T., Neale, R. B., et al. (2022). LGM paleoclimate constraints inform cloud parameterizations and equilibrium climate sensitivity in CESM2. J Adv Model Earth Syst 14:e2021MS002776. doi: 10.1029/2021MS002776
Zhu, J., and Poulsen, C. J. (2021). Last glacial maximum (LGM) climate forcing and ocean dynamical feedback and their implications for estimating climate sensitivity. Clim. Past 17, 253–267. doi: 10.5194/cp-17-253-2021
Zhu, J., Poulsen, C. J., and Otto-Bliesner, B. L. (2020). High climate sensitivity in CMIP6 model not supported by paleoclimate. Nat. Clim. Chang. 10, 378–379. doi: 10.1038/s41558-020-0764-6
Zhu, J., Poulsen, C. J., and Otto-Bliesner, B. L. (2024). Modeling past hothouse climates as a means for assessing earth system models and improving the understanding of warm climates. Annu. Rev. Earth Planet. Sci. 52, 351–378. doi: 10.1146/annurev-earth-032320-100333
Zocatelli, R., Lavrieux, M., Guillemot, T., Chassiot, L., Le Milbeau, C., and Jacob, J. (2017). Fecal biomarker imprints as indicators of past human land uses: source distinction and preservation potential in archaeological and natural archives. J. Archaeol. Sci. 81, 79–89. doi: 10.1016/j.jas.2017.03.010
Keywords: climate modeling, paleoclimate reconstructions, paleoenvironment reconstructions, future climate, climate change impacts
Citation: Kageyama M, Braconnot P, Chiessi CM, Rehfeld K, Ait Brahim Y, Dütsch M, Gwinneth B, Hou A, Loutre M-F, Hendrizan M, Meissner K, Mongwe P, Otto-Bliesner B, Pezzi LP, Rovere A, Seltzer A, Sime L and Zhu J (2024) Lessons from paleoclimates for recent and future climate change: opportunities and insights. Front. Clim. 6:1511997. doi: 10.3389/fclim.2024.1511997
Edited by:
Matthew Collins, University of Exeter, United KingdomReviewed by:
Xuguang Sun, Nanjing University, ChinaLin Chen, Nanjing University of Information Science and Technology, China
Copyright © 2024 Kageyama, Braconnot, Chiessi, Rehfeld, Ait Brahim, Dütsch, Gwinneth, Hou, Loutre, Hendrizan, Meissner, Mongwe, Otto-Bliesner, Pezzi, Rovere, Seltzer, Sime and Zhu. This is an open-access article distributed under the terms of the Creative Commons Attribution License (CC BY). The use, distribution or reproduction in other forums is permitted, provided the original author(s) and the copyright owner(s) are credited and that the original publication in this journal is cited, in accordance with accepted academic practice. No use, distribution or reproduction is permitted which does not comply with these terms.
*Correspondence: Masa Kageyama, bWFzYS5rYWdleWFtYUBsc2NlLmlwc2wuZnI=