- 1Indian Institute of Tropical Meteorology, Ministry of Earth Sciences, Pune, India
- 2Krea University, Sri City, India
- 3Faculty of Environmental Earth Science, Hokkaido University, Sapporo, Japan
- 4Department of Environmental Science, School of Science and Engineering, Ateneo de Manila University, Quezon City, Philippines
- 5Fenner School of Environment and Society, Australian National University, Canberra, ACT, Australia
- 6Indian Institute of Geomagnetism, Mumbai, India
- 7School of Environmental Science, Jawaharlal Nehru University, New Delhi, India
- 8International Centre for Integrated Mountain Development, Kathmandu, Nepal
- 9School of Earth and Environmental Sciences, Seoul National University, Seoul, Republic of Korea
- 10Interdisciplinary Program in Artificial Intelligence, Seoul National University, Seoul, Republic of Korea
- 11Manila Observatory, Ateneo de Manila University Campus, Quezon City, Philippines
- 12First Institute of Oceanography, Ministry of Natural Resources, Qingdao, China
Anthropogenic climate change has led to rapid and widespread changes in the atmosphere, land, ocean, cryosphere, and biosphere, leading to more pronounced weather and climate extremes globally. Recent IPCC reports have highlighted that the probability of compound extreme events, which can amplify risk, has risen in multiple regions. However, significant gaps remain in our understanding of the drivers and mechanisms behind these events. This concept paper discusses compound events in the Asian region in the context of its unique and diverse geographical settings, and regional climatic features including the seasonal monsoons. Notably, Asia is the world’s most disaster-affected region due to weather, climate, and water-related hazards. Therefore, an integrated understanding of how climate change will impact compound events in this region is essential for effective forewarning and risk mitigation. This paper analyzes three typologies of compound events in the Asian region, illustrating their regional complexity and potential linkages to climate change. The first typology pertains to compound floods, for example, the devastating floods in the Indus River Basin and adjoining Western Himalayas during 2022 caused by the combined effects of heavy monsoon rainfall, intense pre-monsoon heatwaves, glacier melt, and modes of climate variability. The second typology relates to compound heatwave-drought events that have prominently manifested in East and South Asia, and are linked to large-scale drivers of the land-atmosphere–ocean coupled system and local feedbacks. The third typology relates to marine extremes involving the compounding effects of ocean warming, sea-level rise, marine heatwaves, and intensifying tropical cyclones. We identify key knowledge gaps in understanding and predicting compound events over the Asian region and discuss advances required in science and technology to address these gaps. We also provide recommendations for the effective utilization of climate information towards improving early warning systems and disaster risk reduction.
1 Introduction
The Earth’s global mean surface temperature has risen by about 1.2 to 1.3°C since pre-industrial times (Met Office, 2024; Forster et al., 2023). The planet’s warming has been unequivocally attributed to human activities by the Intergovernmental Panel on Climate Change (IPCC)‘s Sixth Assessment Report (AR6; Masson-Delmotte et al., 2021). Warming has been accompanied by increasing trends in weather and climate extremes, such as heatwaves, heavy precipitation, droughts, and tropical cyclones (TCs; Arias et al., 2021).
The threats posed by rising extremes have pushed our planet into an era of increasingly complex, intersecting climate risks (IPCC, 2023). The AR6 asserts with high confidence that multiple, interrelated hazards—both climatic and non-climatic—will increasingly co-occur, thereby amplifying overall risks and precipitating cascading impacts across sectors and regions (Pörtner et al., 2022b; Seneviratne et al., 2021). This assumes greater significance for developing countries given the higher vulnerability in their socio-economic contexts (IPCC, 2022).
One of the emerging concerns in the context of climate change is the occurrence of compound extreme events, where multiple climatic drivers and hazards interact to produce more severe impacts as compared to those caused by hazards occurring in isolation (Aghakouchak et al., 2020; Zscheischler et al., 2020). For instance, a heatwave combined with a drought can create extreme stress on water resources and agriculture, while heavy rainfall on saturated soils can lead to floods of greater intensity. The interactions among drivers make such events more challenging to predict and manage, often resulting in amplified impacts. The AR6 has assessed, with high confidence, an increase in the incidence of compound events with warming (IPCC, 2021: Summary for Policymakers; Seneviratne et al., 2021).
A recent example of a compound event is the extensive flooding incident that occurred in Pakistan in August 2022 following unprecedented rainfall. This compound event turned out to be one of the world’s most catastrophic disasters, which displaced over 30 million people, caused more than 1,000 deaths and economic losses exceeding USD 30 billion with reconstruction costs anticipated to surpass USD 16 billion (World Bank, 2022; Wyns, 2022). Subsequent analyses suggest that this disaster emerged from a confluence of drivers that combined to exact damages far greater than what each driver may have done in isolation (Mallapaty, 2022; Hong et al., 2023; Otto et al., 2023; Nanditha et al., 2023).
While heavily precipitating monsoon systems from the south and east provided an important trigger for the Pakistan 2022 flood event, the intertwined processes that can facilitate compound flooding in the region are couched in Asia’s diverse geographical setting, including the elevated Tibetan Plateau, glaciated high mountains, dry continental regions to the north and west, and a complex coastline bordered by the Indian and Pacific Oceans (see Qiu, 2008; Rasmussen and Houze, 2012; Salinger et al., 2014; Shaw et al., 2022; Mallapaty, 2022). Complex interactions involving large-scale monsoon and mid-latitude circulation patterns, climate drivers such as La Niña, Indian Ocean Dipole (IOD), and local to regional feedback processes (e.g., land-surface feedback, orographic uplift, cryosphere dynamics, convective organization, etc) create conditions favorable for compound weather and climate extremes in this region (Sillmann et al., 2017; Krishnan et al., 2020). The resulting compound events can produce severe impacts, including property damage, loss of life, displacement of communities, and significant economic losses, which many parts of Asia are already experiencing (Dhara and Krishnan, 2020).
In fact, some of the world’s most disaster-affected regions due to weather, climate, and water-related hazards are situated in Asia, and large parts of the continent are on the frontlines of rising climate risk because of high exposure to climate hazards and socio-economic vulnerabilities (Atwii et al., 2022). However, the lack of long-term observational data over the high mountainous regions (Bohner, 2006) and challenges in representing regional climatic processes, scale interactions, and realistic tropical sea surface temperature (SST) gradients in climate model introduce uncertainties in understanding, predicting compound events as Asia’s climate evolves under the influence of anthropogenic climate change (Wang et al., 2004; Lee and Cha, 2020; Hoell et al., 2023).
The 2024 Kigali Declaration, a key output of the 2023 World Climate Research Programme (WCRP) Open Science Conference, calls for feasible but transformative solutions to address the complex risks arising from climate change impacts (World Climate Reserach Programme (WCRP), 2024); however, such solutions need to be grounded on robust evidence and information. Complex extremes and compound events emerged as one of the key issues discussed at the conference. Challenges include predictability, with special attention given to how extreme events may morph into compound events and the underlying drivers or feedback mechanisms of these processes; coordinated collaboration (i.e., among climate scientists, the climate services community, impact modelers, decision-makers, and communication experts) to make full use of the best available information towards reducing risk; and the disproportionality of risk, especially in the Global South, in an era of climate inequity and injustice (World Climate Reserach Programme (WCRP), 2024).
The Pakistan flood event is a stark example of the risks posed by compounded hazards in Asia and highlights the necessity to enhance our understanding and management of such events (Otto et al., 2023). However, studies on compound hazards in Asia are still severely limited. In alignment with the Kigali Declaration, this concept paper addresses the imperative of understanding compound extremes in the Asian context.
Our objectives are threefold:
1. To analyse key typologies of compound events relevant to Asia. Specifically, we focus on three typologies: (1) compound flooding events, (2) compound heatwave-drought events, and (3) compound marine extremes. We discuss each of them by means of event(s) representative of that typology, as well as the precursors, processes, and teleconnections that tend to precondition and sustain them;
2. To identify prevailing knowledge gaps that impede our ability to forecast these events; and
3. To propose strategies to address these knowledge gaps, towards improving forecasting and risk management.
The paper is structured as follows. Section 1.1 discusses major features of Asia’s climate and synthesizes key assessments, such as the AR6, pertaining to regional changes in extremes. Section 2 focuses on the Asian region, discussing the three typologies of compounded extremes (Sections 2.1 to 2.3) and identifying knowledge gaps. Section 3 offers recommendations aimed at bridging these gaps and improving assessments of impacts and risk.
1.1 Key features of regional climate and climate change in Asia
Asia exhibits a complex climate system profoundly influenced by its unique physical geography and strong interactions among the land, atmosphere, biosphere, ocean, and cryosphere. The Himalayan topography, regional mountain ranges together with the elevated Tibetan Plateau and surrounding oceanic regions exert dominant control on the South and East Asian monsoons—which are key components of the global climate system (Yanai et al., 1992; Yanai and Li, 1994; Boos and Kuang, 2010; Krishnan et al., 2019, 2020). However, significant portions of the continent, such as Central Asia and Siberia, which lie outside the monsoon region exhibit markedly different climatic conditions, ranging from the arid climates of vast deserts to the permafrost regions of Siberia (see Sharma et al., 2019; Bolch et al., 2019). Interannual and decadal scale variations in the South and East Asian monsoon rainfall are known to have links to modes of climate variability, such as El Niño Southern Oscillation (ENSO) and the Indian Ocean Dipole (IOD), Pacific Decadal Variability (PDV; IPCC AR6, 2021, Technical Annex).
From the perspective of climate change, the IPCC AR6 has assessed that the mean surface temperature and heat extremes have increased across Asia, as have marine heatwaves in the surrounding oceanic regions. The AR6 also assessed that the tendency for an increase in monsoon precipitation over South and East Asia due to warming from GHG emissions has been counteracted by anthropogenic aerosol cooling during the twentieth century (Masson-Delmotte et al., 2021). With continued warming, monsoon precipitation over South Asia and East Asia is projected to increase during the 21st century, with increased variability (see Wang et al., 2021; Masson-Delmotte et al., 2021).
Daily precipitation extremes have increased in parts of East Asia, leading to more frequent and intense landslides in some mountain areas. South East Asia has been affected by fewer but more extreme tropical cyclones (Wang et al., 2021; IPCC AR6, 2021).
Interactions with the cryosphere are particularly pronounced in Asia, where extensive glaciers play a vital role (Bolch et al., 2019). The meltwater from these cryospheric reserves feeds many of Asia’s major river systems, particularly in West Asia (Scott et al., 2019). The albedo associated with snow and ice cover exerts strong influence on regional energy balance, with climate change markedly impacting the Himalayan cryosphere and subsequently affecting the discharge in surrounding river systems and basins (Bolch et al., 2019).
Several areas in the Hindukush-Karakoram-Himalayas have exhibited a decline in snowfall and retreating glaciers in recent decades (Sabin et al., 2020; IPCC AR6, 2021), but parts of the high-elevation Karakoram Himalayas have experienced increased wintertime precipitation (see Kapnick et al., 2014; Forsythe et al., 2017; Bolch et al., 2019; Krishnan et al., 2019; Farinotti et al., 2020; and references therein).
Glacial retreat and permafrost thawing are evident across the Tibetan Plateau and North Asia. Concurrently, relative sea levels around Asia are rising faster than the global average, leading to losses in coastal areas (Bolch et al., 2019; IPCC AR6, 2021).
2 Compound events with focus on Asia
Most weather- and climate-related events are not isolated but strongly coupled. For instance, droughts and heat waves often co-occur. In June 2023, anomalously high temperatures were witnessed in parts of India, when monsoon precipitation was substantially lower than the long-term average (IMD, 2023). Rainfall deficits lead to dry soils, which impede evaporative cooling of the surface and cause increased warming (Chiang et al., 2018). Thus, the co-occurrence of heatwaves and droughts can be termed a compound event.
Chapter 11 of the IPCC AR6 WG1 broadly defined compound events as the combination of multiple drivers and/or hazards contributing to societal or environmental risk based on Zscheischler et al. (2020). In this context, “drivers” refer to meteorological processes or variables that initiate climatic events. “Hazards” are the direct physical phenomena that result from these drivers, including floods and heatwaves, which act as immediate precursors to potentially negative impacts on society and the environment.
Compound events have been classified into preconditioned events, where a weather-driven or climate-driven precondition aggravates the impacts of a hazard; multivariate events, where multiple drivers and/or hazards lead to an impact; temporally compounding events, where a succession of hazards leads to an impact; and spatially compounding events, where hazards in multiple connected locations cause an aggregated impact (Zscheischler et al., 2020). The effective impacts of compound or concatenated extremes, whether causally related or not, can be much higher than the sum of individual extremes alone.
Extreme and especially compound extreme events pose severe risks across the board—food, water, the economy, infrastructure, and energy (Pörtner et al., 2022b). For example, a prolonged heatwave compounded by drought conditions could devastate agriculture (Tripathy and Mishra, 2023; Dhara and Koll, 2023) and ecosystems (Allen et al., 2010). Water scarcity not only threatens drinking water supplies but also has ramifications for hydropower energy generation (Eyer and Wichman, 2018). Similarly, the compounded effects of heavy rainfall and landslides could damage critical infrastructure like roads and bridges, hindering emergency responses and long-term recovery efforts (Haque et al., 2019; Dave et al., 2021; Zhu et al., 2021). This can also disrupt supply chains, leading to economic losses and impacting jobs (Becker et al., 2018).
Developing countries face a greater risk from weather and climate extremes because of higher exposure and vulnerability (Pörtner et al., 2022b). According to the AR6, the largest absolute number of people displaced by extreme weather each year occurs in South, Southeast and East Asia (Pörtner et al., 2022a). South Asia, in particular, is assessed as one of the hotspots of high human vulnerability (Pörtner et al., 2022b).
Given the complexity of compound events, we have adopted the strategy of focusing on three typologies of compound extreme events in Asia. In each case, we identify potential meteorological / climate drivers of an observed impact and the interactions between them. Finally, we identify knowledge gaps that must be addressed to improve future forecasting.
2.1 Compound flooding
In this section, we discuss the typology of compound flooding in Asia caused by the cascading impacts of climate change. Physical processes driving compound flooding are inherently complex and vary widely across events. The purpose of this discussion, however, is not to give an exhaustive list of such events in Asia. Instead, we give a detailed account of one specific high impact event in Asia to highlight how compound flooding in this region can emerge from complex interactions among Earth system components. We then briefly discuss similar events in the wider Asian context before turning to the key knowledge gaps that hinder our understanding of the processes involved, and our ability to predict these events.
The case study we analyze is the extreme flooding that occurred in Pakistan in August 2022, which was one of the largest disasters of recent years.
2.1.1 The 2022 Pakistan floods as a compound event
The proximate cause of the 2022 Pakistan floods was the extreme rainfall in August 2022 (Nanditha et al., 2023; Annamalai, 2024; https://cdpc.pmd.gov.pk). However, subsequent analysis has revealed a more nuanced picture of how multiple interactive drivers and hazards exacerbated this disaster (Otto et al., 2023).
2.1.1.1 Local preconditioning
Intense summer heatwaves during March–May significantly elevated temperatures across Pakistan, accelerating glacial melt in the northern mountainous region and increasing streamflow in the upper tributaries of the Indus river basin (Mallapaty, 2022). Concurrently, intense land surface heating appears to have promoted low-pressure development over Pakistan (Otto et al., 2023) while unusually warm north Arabian Sea SSTs led to moisture build-up over the region (Doi et al., 2024; Luo et al., 2024). In addition, extraordinarily high vapor transport over the Arabian Sea during 2022 favored the intensification and lifespan of westward propagating monsoon low pressure systems (You et al., 2024; Otto et al., 2023). These drivers combined to induce early, heavy, and extreme episodes of monsoon rainfall.
Furthermore, prolonged rainfall over several weeks caused soil moisture to saturate, markedly increasing runoff and the likelihood of subsequent flooding (Nanditha et al., 2023; see also Wasko and Nathan, 2019). In other words, these events preconditioned flooding in response to extreme rainfall.
2.1.1.2 Temporal and spatial compounding
A sequence of drivers and hazards occurred in close succession in and around the Pakistan region between March and August 2022 and amplified the subsequent impacts. Prolonged heatwaves during March–May, enhanced glacier melt in subsequent months, sustained rainfall in July–August, followed by extreme rainfall during August 16–24 comprised temporally compounding events (Mallapaty, 2022; Hong et al., 2023). Furthermore, the widespread nature of precipitation concurrently affected multiple provinces such as Sindh and Balochistan, and resulted in aggregated impacts. Therefore, the temporal compounding involving multiple drivers/hazards in succession was accompanied by spatial compounding of the same hazard (sustained rainfall) experienced simultaneously by vast regions. When extreme rainfall events occurred during 16–24 August 2022, driven by large moisture transport from the Arabian Sea, much of it fell on already saturated soils, severely magnifying the subsequent flooding (Nanditha et al., 2023).
2.1.1.3 Remote influence
Extreme rainfall events in the Asian monsoon region are known to have links to global atmospheric teleconnection patterns through dynamical tropical-extratropical couplings (Boers et al., 2019). Here, it is worth mentioning that several major flood events in Pakistan including the 2010 and 2022 events, have coincided with La Niña conditions in the tropical Pacific (see Mujumdar et al., 2012). Persistent large-scale atmospheric circulation anomalies during La Niña episodes characterized by westward shifts in the West Pacific Subtropical High and atmospheric blocking in the mid-latitude jet stream over Europe and Russia (Mujumdar et al., 2012; Lau and Kim, 2012; Martius et al., 2013; Ullah and Shouting, 2013; Otto et al., 2023; Hong et al., 2023; Zhang et al., 2024). The large-scale atmospheric circulation anomalies together with warm SST anomalies in the tropical West Pacific and Indian Oceans during La Niña events enhance northward and westward transport of moisture fluxes into the Indo-Pak region and promote deep moist convection and increased rainfall (Priya et al., 2015; Hong et al., 2023).
2.1.1.4 Cascading impacts in remote regions
Intense rainfall events over South and West Asia can potentially trigger multiple disasters in other parts of Asia, through a series of interconnected drivers that can amplify the severity of disasters (Malik et al., 2012; Tang et al., 2023; Hong et al., 2023; Zhang et al., 2024). For example, the record-breaking heatwaves in the Yangtze River Valley in July and August 2022 appear to have emerged from modifications in the large-scale atmospheric circulation and the downstream Rossby wave train, which were induced by latent heating associated with the extreme rainfall in the Indus basin (Tang et al., 2023; Hong et al., 2023; Zhang et al., 2024). Additionally, these studies have suggested that the triple-dip La Niña (2020–2022) played a role in setting up these conditions by forcing a westward extension of the Western Pacific Subtropical High and reinforcing the atmospheric circulation patterns that led to the extreme heatwaves. In short, compound drivers can produce multiple impacts in widely separated regions through strong dynamical interactions. The frequency and intensity of such cascading events are expected to amplify in a warming world (Seneviratne et al., 2021). It is therefore essential to develop effective multi-hazard early warning systems to mitigate the increasing risk from future compound extreme events (UNISDR, 2015; see also Sec. 3.1.5).
The major processes, interactions, feedbacks and teleconnections that played a role in the Indus River Valley compound flooding and the Yangtze River valley heatwave-drought events are schematically illustrated in Figure 1.
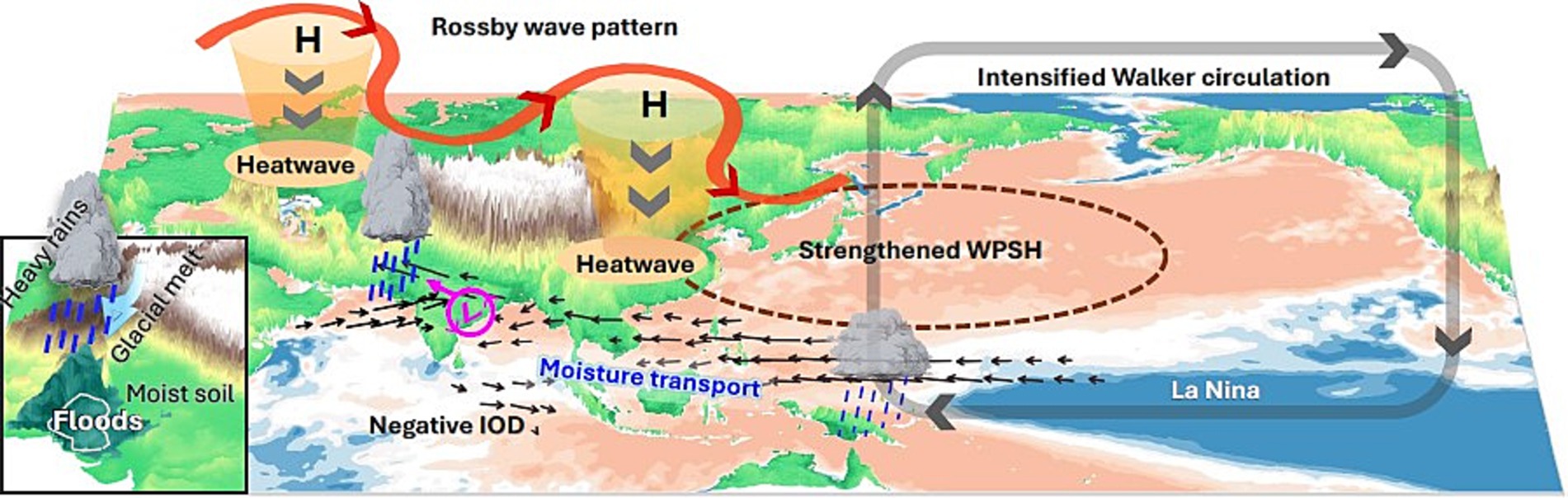
Figure 1. Schematic of the 2022 Pakistan flood event: local pre-conditioning, remote influence and its cascading impacts. The inset shows the local processes, glacier melt (thick blue-white arrow) from Northwest Himalaya, heavy rainfall, and moist soil (dark green shading) causing floods over Pakistan (white contour). The large-scale features include intense moisture transport (black arrows) from Tropical Pacific and Arabian Sea induced by an intense La Niña, a negative IOD, and an unusually warm Arabian Sea. Westward propagating low pressure system (magenta circle with “L”) further aided the moisture influx. “H” indicates upper level high associated with the atmospheric teleconnection pattern causing heatwaves over Europe, and China during the same period.
2.1.2 The wider Asian context
2.1.2.1 East Asia
The far-field effects of the Indo-Pacific SST anomalies on monsoon precipitation are not limited to South Asia, but extend to areas in the Far East. For example, precipitation associated with the Meiyu in China, Baiu in Japan, and Changma in Korea, all of which are important constituents of the East Asian Summer Monsoon, were substantially enhanced during June and July 2020 (Park et al., 2021a). Heavy precipitation events also occurred in South Korea as they were associated with an enhanced and prolonged East Asian summer monsoon (Park et al., 2021b). While persistent La Niña conditions prevailed from 2020 through 2022, the prolonged enhancement of the East Asian summer monsoon rainfall during June and July 2020 was also influenced by the Indian Ocean SST distribution, which caused a southwestward extension of the subtropical high and enhanced moisture transport (Takaya et al., 2020). In fact, the triple-dip La Niña from 2020 through 2022 (Li et al., 2023) was preceded by an extreme positive Indian Ocean Dipole (IOD) in 2019 (Ratna et al., 2021) that caused record-breaking extreme precipitation over the Kyushu Island in Japan in July 2020 (Horinouchi et al., 2021), as well as extreme monsoon precipitation over western India (Ayantika et al., 2024; Krishnan et al., 2024) that lasted for several days over both locations. The long-lasting nature of precipitation over the Kyushu Island during 2019 resulted from the combined effects of the overall shift of the Baiu subtropical front and a particular phase of the wave train of the Silk-road teleconnection (Horinouchi et al., 2021). These studies suggest that the heavy precipitation events in East Asia can result from temporally compounded events across multiple spatial scales.
Studies of other major events such as the catastrophic precipitation event in Zhengzhou City, Henan Province of China, in July 2021 (Zhang Q. et al., 2023), and in Seoul, South Korea in August 2022 (Park et al., 2024), and in Beijing of China around 31 July 2023 (Li et al., 2024) have concluded that these events emerged from multi-scale dynamical processes. These highlight that, as with the 2022 Pakistan event, extreme precipitation events in East Asia emerge from a confluence of multiple drivers, both local and remote.
Surface measurements show that extreme precipitation events have become more frequent and intense over the past 60 years (Do et al., 2023). There is no question that the increase in extreme precipitation in East Asia is due to anthropogenic warming. However, it cannot be explained simply by increasing moisture in a warming climate, because extreme precipitation in the tropics and monsoon regions is tied to the organization of mesoscale convective systems, embedded in synoptic and large-scale atmospheric circulations (Houze, 2004; Liu, 2011; Roca et al., 2014; Roca and Fiolleau, 2020; Ayantika et al., 2024; Krishnan et al., 2024). This multiscale nature makes quantitative attribution of long-term precipitation trends difficult.
2.1.2.2 High Mountain Asia
The discussion on extreme precipitation and compound flooding in Asia is incomplete without considering the unique hazards posed by Glacial Lake Outburst Floods (GLOFs) in the unique and fragile High Mountain regions of Asia. GLOFs represent a significant threat in mountainous regions, where increased melt from glaciers due to warming leads to the formation and expansion of glacial lakes (ICIMOD, 2023). The resulting GLOFs can have devastating impacts on downstream populations.
Formation of glacial lakes due to increased melting of glaciers in a warming climate and their subsequent expansion represents a major flooding hazard that can result from GLOFs, threatening large populations in High Mountains Asia (HMA; Bajracharya et al., 2007; Taylor et al., 2023; Zhang T. et al., 2023; Shrestha et al., 2023; Dubey et al., 2024). For example, the breaching of the South Lhonak Glacial lake in Sikkim, India, in early October 2023 killed more than 40 people and left 75 reported missing, while washing away many bridges and roads (Chettri, 2023). GLOF risk is expected to increase in the future (ICIMOD, 2023), also increasing the potential for transboundary events with cross-border impacts, e.g., a glacial lake may lie within the borders of one country, but the main impact of a GLOF event may be across the border in another country. For example, the 2016 GLOF in the Bhote Koshi River that occurred when a moraine-dammed lake burst in the Zhangzangbo River basin, a primary tributary of the Poiqu/Bhote Koshi River in China that substantially damaged the 45 MW Upper Bhote Koshi Hydropower project in Nepal (Bruen et al., 2017). Detailed investigations are needed to understand the mechanisms that cause breaches in glacial lakes, which can help in developing early warning systems (Sattar et al., 2019) particularly in transboundary basins.
The Melamchi disaster in Nepal’s Sindhupalchowk district in June 2021 illustrates the complex, cascading nature of such events. High rainfall, GLOFs, landslides, and landslide dam outburst floods combined to amplify the disaster’s scale, killing at least five people, with 20 people reported missing, and also causing substantial economic losses (Maharjan et al., 2021; Adhikari et al., 2023). These complex cascading disasters are becoming more common in the Hindu Kush Himalayan region (Zhang T. et al., 2023). It is crucial to integrate the study of GLOFs into the broader context of extreme precipitation and compound flooding events to develop comprehensive mitigation strategies.
2.1.3 Key knowledge gaps
Some of the key knowledge gaps pertaining to climate change induced compound flooding in the Asian region are presented below.
2.1.3.1 Challenges in modeling and attributing extreme precipitation/flooding events to climate change
Extreme event attribution aims to elucidate the link between global climate change, extreme weather events, and loss and damage due to anthropogenic climate change; this allows disentangling natural drivers of extreme weather from human-induced climate change (see Clarke et al., 2022). However, several challenges hinder our ability to attribute specific extreme events to anthropogenic climate change.
A significant challenge is the lack of high-quality, high-resolution historical and real-time data for soil moisture, precipitation, and other variables in many parts of Asia. The lack of high-quality data and long-term climate records in many parts of Asia hinders accurate assessment of past extreme events, climate model validation, and the ability to distinguish natural variability from human-induced changes (Clarke et al., 2022; Otto, 2017).
Another challenge is the limitations of climate models. A study by Doi et al. (2024) on the failure of models to forecast the extreme Pakistan rainfall events in 2022 found that one of the reasons for the failure was that models did not correctly simulate the anomalously high Arabian Sea SSTs that persisted during the summer. In addition, current climate models face major challenges in accurately simulating the magnitude, location and timing of extremely heavy precipitation events, especially convective systems critical in monsoon regions (Stevens et al., 2024).
2.1.3.2 Challenges related to the complex spatial and temporal variability of the Asian monsoon
A major source of uncertainty in the attribution of extreme precipitation events in the Asian monsoon region is that these events are strongly influenced both by internal dynamics, and by large-scale teleconnections (Boers et al., 2019). The Asian monsoon rainfall exhibits complex spatial and temporal variability due to strong links with modes of natural variability like ENSO and IOD (IPCC AR6, 2021, Technical Annex). On the one hand, these linkages contribute significant interannual variability to monsoon precipitation, with El Niño events generally leading to weaker monsoon rainfall and La Niña events enhancing it. This temporal variability is further influenced by the Pacific Decadal Variability (PDV), which affects the monsoon’s strength and precipitation patterns over decadal timescales.
On the other hand, a deeper question that arises is how these modes of variability might themselves respond to climate change—a scientific issue that is not well understood. Several state-of-the-art climate models show a weakening of the Pacific Walker circulation and a El Niño-like SST response to global warming (Vecchi and Soden, 2007; Collins et al., 2013). However, more recent studies suggest that the response of the mean state of the tropical ocean–atmosphere coupled system to global warming is more likely to produce a La Niña-like regime in the Pacific with stronger zonal SST gradient (i.e., warmer west and colder east) and intensified atmospheric Walker circulation (Seager et al., 2019; Douville et al., 2021; Kalik et al., 2024). This, in turn, raises the question about the role of climate change in shaping the evolution of specific La Niña events such as 2010, 2022 and subsequently their impacts on extreme monsoon precipitation events over the Asian region.
Uncertainty also persists in historical trends in midlatitude circulation patterns and the relative influence of forced and natural variability (Francis et al., 2017; Francis, 2017; Cohen et al., 2020) posing challenges to projecting future circulation change (Shepherd, 2014; Kornhuber and Tamarin-Brodsky, 2021).
2.1.3.3 Challenges due to inadequate data coverage
Sparse historical data collection in remote regions, such as the Himalayan ranges, limits the ability to understand the complex processes driving extreme events. Large observational spread, particularly in in-situ, satellite, and gridded precipitation datasets over the Third Pole and Pakistan region, impedes the evaluation of km-scale model simulations thus limiting advancements in understanding the hydroclimate of this critical region (Collier et al., 2024). Additionally, there is a paucity of in situ observations over many regions due to sparse station network (Shrestha et al., 2015), which hampers the understanding and prediction of extremes. High-resolution, real-time precipitation, and soil moisture datasets are essential for accurate forecasting and risk management but are unavailable in many regions of Asia. There is also a lack of functional early warning systems in transboundary basins.
2.2 Compound heatwave-drought events
Heatwaves are another class of climate extremes pertinent to Asia. In 2018, East Asia experienced an unusually hot summer (Tao and Zhang, 2019; Shimpo et al., 2019; Ha et al., 2020). Daily temperatures reached 37.3°C in Shenyang, Northeast China; 41.1°C in Kumagaya, north of Tokyo, Japan; and 41.0°C in Hongcheon, South Korea. The heatwave in South Korea broke the country’s historical record, resulting in 4,526 heat-related illnesses and 48 heat-related deaths (Ha et al., 2020). The heatwave in eastern Japan, which followed heavy rainfall in the western part of the country, also set the highest temperature record (Shimpo et al., 2019).
Similar heatwaves have also been observed in South Asia, affecting millions in the region (Aadhar and Mishra, 2023 and references therein). In the 2022 pre-monsoon season, a highly anomalous heatwave occurred as described in section 2.1.1 (Aadhar and Mishra, 2023). It affected about 60% of South Asia over a five-week period from late February to April 2022, with temperatures nearly 4°C above normal over a wide area (Aadhar and Mishra, 2023).
In Southeast Asia, observations from the 1980s to 2010s indicate significant upward trends in the frequency and duration of heatwaves over most areas, as regional temperatures have increased and influenced by El Niño events (Dong et al., 2021; Li, 2020; Li et al., 2022). Furthermore, heatwaves defined by daily minimum temperatures have high increasing trends, which can have serious impacts on mortality rates (Li, 2020; Li et al., 2022).
2.2.1 Compound heatwave-drought: trends and projections
Heatwaves often occur concurrently with droughts, leading to compound heatwave-drought events (CHDEs). The CHDEs are more disastrous than standalone heatwave events (Wang et al., 2020; Zscheischler et al., 2020). For instance, they can contribute to the onset of wildfires and rapidly developing droughts, exacerbating the impacts on ecosystems, agriculture, and water resources.
Wang et al. (2020) performed an attribution study to conclude that the rise in anthropogenic greenhouse gas emission has significantly driven the frequency and intensity of summertime compound hot extremes across the Northern Hemisphere from 1960 to 2012. They further projected an approximate eightfold increase in the frequency and a threefold increase in the intensity of these extremes by 2,100 under unabated greenhouse gas emissions. This increase in compound hot extremes has profound implications for future population exposure, with a four to eightfold increase in exposure projected by the end of the century. Asia has also witnessed a rising trend in compound dry extremes with East, South, and Southeast Asia identified as hotspots (Abella and Ahn, 2024).
2.2.1.1 East Asia
CHDEs in East Asia have become more frequent during the last few decades, especially over northern East Asia (Kong et al., 2020; Yu and Zhai, 2020; Seo and Ha, 2022). Kong et al. (2020) highlighted a significant increase in the frequency of concurrent drought and heatwave events in Eastern China, particularly noting that these events are more common in the north and south of the region. The positive dependence between droughts and heatwaves has exacerbated the occurrence of these compound events, primarily driven by the increase in heatwave occurrences. Yu and Zhai (2020) reported that CHDEs in northern East Asia have become more frequent, widespread, and persistent since the 1990s. Seo and Ha (2022) attributed the rising CHDE occurrences to enhanced land-atmosphere interactions through strengthened soil moisture-temperature coupling. They found that persistent soil moisture deficits have amplified surface warming and intensified heatwaves, particularly over the past two decades.
Reconstructions of heatwaves and soil moisture for the past 260 years by Zhang et al. (2020) showed an abrupt climate shift towards a hotter and drier climate over inner East Asia in the 1990s. They suggested that the magnitude of recent CHDEs is unprecedented over the past quarter of a millennium, highlighting the severity of the current climate crisis.
These findings collectively highlight the critical need for enhanced understanding and proactive management of CHDEs to mitigate their adverse impacts on agriculture, ecosystems, and human health in the region.
2.2.1.2 South Asia
In the South Asian context, CHDEs have also shown an increasing trend in India. Sharma and Mujumdar (2017) have identified an alarming increase in the concurrent occurrence of meteorological droughts and heatwaves across India, particularly since the 1980s. Guntu and Agarwal (2021) revealed that the frequency of CHDEs in India has increased by 1–3 events per decade from 1977 to 2019 compared to the base period of 1951–1976. This increase is particularly notable in north-central India, western India, north-eastern India, and along the south-eastern coastlines. A study by Ganguli (2023) has found that urban areas across India have witnessed a significant increase in compound hot and dry spells, with a median 6-fold amplification in their joint frequencies relative to the expected annual number of local (univariate) 50-year severe heatwave episodes.
Mishra et al. (2020) analysed climate projections under the high-emission scenario and reported a 1.5-fold increase in the frequency of concurrent hot and dry extremes in India by the end of the 21st century, despite an overall increase in projected precipitation Mishra et al. (2020). However, this paradoxical increase in CHDEs, despite the projected precipitation rise, is not well understood. For example, Ganeshi et al. (2023) analysed the impact of soil moisture perturbations on temperature extremes over India for the historical period (1951–2010) and future (2051–2100) under a 4 K warming scenario and reported that more than 70% area of the Indian landmass experienced significant changes in the characteristics of temperature extremes due to soil moisture perturbations. Ganeshi et al. (2023) noted that the impact of soil moisture perturbations is particularly large over north-central India, a regional hotspot for strong soil moisture -temperature coupling, where precipitation and soil moisture anomalies can significantly revamp the frequency, duration and intensity of extreme temperatures by modulating surface energy partitioning, evapotranspiration and soil moisture memory. Clearly further studies are warranted to better understand the future behaviour of CHDEs over the South Asian region, especially in view of the projected increase in the regional monsoon precipitation during the 21st century, with increased variability (Wang et al., 2021; Masson-Delmotte et al., 2021).
2.2.1.3 Southeast Asia
Global-scale analysis has identified Southeast Asia, among other regions in Asia, where warm season CHDEs have become more severe and more frequent, associated with a significant increase in temperature (Hao et al., 2018; Hao et al., 2022). However, there is currently very limited regional-scale analysis of CHDEs in this area. Abella and Ahn (2024) estimate an approximate return period of less than 2 years for CHDE over mainland Southeast Asia.
2.2.2 Drivers and mechanisms of CHDEs
The mechanisms behind the occurrence and change in CHDEs over the Asian continent are still not fully understood. East Asian CHDEs are often influenced by the anticyclonic circulation anomalies which are modulated by zonally propagating Rossby waves originating from Eurasia, such as the Silk Road pattern (Zhang and Zhou, 2015; Noh et al., 2021). In addition to large-scale atmospheric circulation patterns, local SST and soil moisture anomalies also play a role in the development of CHDEs (Ni et al., 2024, and references therein).
As addressed above, and in other studies on CHDEs (Schumacher et al., 2022; Hao and Singh, 2020), the role of soil moisture-temperature coupling has been identified as an important driver of CHDEs over India (Rajeev and Mishra, 2022; Guntu et al., 2023; Ganeshi et al., 2023) and northern East Asia (Seo and Ha, 2022). Soil moisture depletion—pre-conditioned by precipitation deficits—reduces evaporative cooling, creating a feedback loop that amplifies both hot and dry conditions. Guntu and Agarwal (2023) reported that low soil moisture is responsible for 55–65% of CHDE occurrences in India.
Studies have emphasized the key role of land-atmosphere feedbacks in the intensification and propagation of CHDEs (Miralles et al., 2019; Wang et al., 2020). Miralles et al. (2019) highlighted that the surface energy partitioning shifts towards sensible heat flux as soil moisture decreases, leading to higher temperatures. In addition, they point to ecosystem-specific responses to drought and heatwaves. Forests, for instance, may initially buffer against extreme temperatures due to their deeper root systems and higher water use efficiency, but they can also contribute to prolonged drought conditions by maintaining high transpiration rates until soil moisture is severely depleted. This complex interplay between different land cover types and their respective feedbacks on the atmosphere is crucial for understanding the full dynamics of CHDEs. The interaction of these feedbacks with large-scale atmospheric patterns and local anomalies forms a complex system that is still being unravelled.
2.2.3 Key knowledge gaps
Given that climate extremes in East Asian and South Asian countries share common characteristics, regional collaborations are essential for effectively addressing these challenges. Such collaborations require unrestricted access to regional datasets across borders. As highlighted by Zhang Q. et al. (2023), only a few percent of surface observations are currently shared with the global system. This limited data sharing hinders the ability of researchers to fully understand the mechanisms underpinning CHDE occurrence, and their trends in the region.
One of the primary data gaps is the lack of high-resolution in-situ observations, which are crucial for capturing the detailed spatio-temporal evolution of localized temperature and precipitation extremes (Li et al., 2022). High-resolution gridded datasets, such as APHRODITE (Asian Precipitation—Highly-Resolved Observational Data Integration Towards Evaluation; Yatagai et al., 2012), need to be updated and extended to provide more comprehensive coverage. Furthermore, there is a significant need for long-term temperature, precipitation, and soil moisture data to improve the monitoring and prediction of CHDEs. Unrestricted access to satellite datasets is also essential.
Several scientific gaps also need to be addressed to improve our understanding and prediction of CHDEs. The driving mechanisms of CHDEs are still not well understood, and models often fail to accurately represent the complex land-atmosphere feedbacks that drive these extremes (Miralles et al., 2019). For instance, the representation of vegetation responses to drought conditions, such as stomatal conductance and root depth variability, is often oversimplified in models (Kala et al., 2016). This would limit the efficacy of models, for instance, in capturing the implications of land-use change, such as deforestation and urbanization, for CHDE occurrence. Future changes in East and South Asian compound extreme events remain uncertain, adding to the complexity of preparing for these events. Moreover, current operational models struggle to predict extreme events accurately on subseasonal-to-seasonal timescales (Kong et al., 2020; Domeisen et al., 2022).
Miralles et al. (2019) also highlight the importance of understanding “teleconnected land–atmospheric feedbacks” and event self-propagation, in which extremes in one region can trigger extremes in another region through atmospheric interactions. This concept of self-propagation adds another layer of complexity to predicting and managing CHDEs, as it requires a comprehensive understanding of both local and remote feedback mechanisms. For example, the drying of soil and vegetation in one region can reduce moisture availability for downwind areas, leading to drought conditions in those regions. This is another example of “cascading impacts in remote regions” alluded to in Section 2.1.
These limitations in observations and models highlight the need for integrated and enhanced observational and modeling approaches that can improve understanding of processes underpinning CHDEs, and capture the full spatial and temporal extent of these extreme events.
2.3 Compound ocean events
Compound ocean events emerging from interactions between long term warming, marine heatwaves (MHW), tropical cyclones (TC), and extreme sea levels (ESL) are becoming increasingly common in the tropical Indian Ocean, threatening marine ecosystems and coastal communities.
In May 2020, a strong MHW, with sea surface temperature anomalies >2.5°C, occurred in the Bay of Bengal, facilitating the rapid intensification of the tropical cyclone Amphan (Rathore et al., 2022). The cyclone-induced storm surges led to extreme sea levels and extreme precipitation, resulting in a compound flooding event, inundating the coastal districts of India and Bangladesh, causing deaths and displacement along with widespread environmental and agricultural damages (Basheer Ahammed and Pandey, 2021). Understanding these compound ocean extremes is therefore crucial for predicting and mitigating the risks associated with climate change.
2.3.1 Context: changes in the ocean state
The tropical Indian Ocean, due to its geographic configuration and the influence of seasonally reversing monsoon winds, is one of the fastest-warming ocean basins globally, with a basin-wide surface warming rate of 0.12°C per decade since 1950 (Alory and Meyers, 2009; Swapna et al., 2020; Roxy et al., 2020, 2024). This accelerated warming has amplified multiple hazards, including extreme monsoon rainfall (Krishnan et al., 2015; Roxy et al., 2017), severe tropical cyclones, and extreme sea levels. In addition, marine heatwaves (MHWs), which are periods of hot oceanic temperatures above the 90th percentile for at least 5 days, in the tropical Indian Ocean are amplifying the intensity of tropical cyclones, resulting in devastating impacts in coastal areas (Rathore et al., 2022).
A consequence of the accelerated warming of the tropical Indian Ocean is the rapid increase in thermosteric sea level in the basin (Srinivasu et al., 2017; Swapna et al., 2017), which is estimated to have risen at a rate of about 5.5 mm yr.−1 between 2013 and 2020 (Sreeraj et al., 2022)—about 15% higher than the rate of global mean sea level rise (WMO, 2022).
Sea level variations in the Indian Ocean are modulated by modes of climate variability like El Niño Southern Oscillation (ENSO), Pacific Decadal Oscillation (PDO), Indian Ocean Dipole (IOD), and also decadal variability in the strength of monsoon winds and associated ocean heat transport, etc. (Swapna et al., 2017, 2020; Jyoti et al., 2019). Global and Indian Ocean sea levels are projected to increase further with continued ocean warming (Fox-Kemper et al., 2021).
2.3.2 Compound events associated with marine extremes
The rapid warming of the Indian Ocean has resulted in several cascading and compound extremes. The combined effects of rapid ocean warming and increase in MHWs in the tropical Indian Ocean during the past four decades (Saranya et al., 2022; Chatterjee et al., 2022) have resulted in compounding impacts on coral bleaching and marine ecosystems (Collins et al., 2019; Smith et al., 2023), modulation of the seasonal monsoon rainfall (Saranya et al., 2022), and intensification of TCs (Murakami et al., 2017; Swapna et al., 2017, 2022; Balaji et al., 2018; Deshpande et al., 2021), which are experiencing rapid intensification in recent times (Singh et al., 2021; Rathore et al., 2022). These trends are projected to persist with future warming, with TC intensity, including wind speeds, rainfall rates, and storm surge, projected to amplify (Masson-Delmotte et al., 2021).
Mawren and Hermes (2022) found that, over the southwest Indian Ocean, a high percentage of pre-existing MHWs help strengthen tropical cyclones as they pass over the MHW region but an even higher percentage of the cyclones tend to weaken or end the MHWs after they both co-occur. In the western North Pacific and the Atlantic basins, Choi et al. (2024) found that the maximum lifetime intensity of tropical cyclones formed during MHWs was 35.4% stronger, and also that enhanced latent heat flux during MHWs led to higher precipitation. Interactions between MHWs and TCs are, therefore, complex and can be classified as multivariate compound events, where the combination of drivers results in greater risks than would occur from individual hazards alone (Zscheischler et al., 2020).
Storm surges associated with intense TCs in combination with sea level rise and astronomical tides, can generate extreme sea levels (ESL)—a form of compounded ocean events that can exacerbate the severity of coastal flooding. For example, storm surge of the extremely severe cyclonic storm Tauktae in the Arabian Sea in 2021 led to ESL conditions along India’s west coast (Sreeraj et al., 2022). Studies have reported increased frequency and intensity of ESL globally (Vousdoukas et al., 2018; Kirezci et al., 2020; Tebaldi and Debeire, 2021). In the Indian Ocean context, Sreeraj et al. (2022) found that there has been a 2–3 fold increase in ESL occurrence since 1970, with higher risk along the Arabian Sea coastline and the Indian Ocean Islands, which is primarily attributable to rising mean sea level. The IPCC AR6 has assessed that ESL events that previously occurred once in 100 years could occur annually or more frequently by the end of this century under all scenarios.
TCs can also be associated with other forms of compounded events such as compound precipitation-wind extremes and compound flooding. Rajeev and Mishra (2023) analysed 94 TCs making landfall over India between 1981 and 2021 and found an increase in TCs exhibiting compound extremes during the most recent decade (2011–2021). Additionally, they found that the spatial extent of regions affected by these extremes has expanded significantly, heightening the risk to coastal infrastructure and human lives. Another study has highlighted higher flood risk from cyclones during the post-monsoon season in India, when soil moisture levels are higher (Rajeev and Mishra, 2022), representing a preconditioned compound event, where the antecedent soil moisture conditions amplify the flooding impact of the TC.
2.3.3 Key knowledge gaps
Despite advances in our understanding of individual marine extremes, the interactions between MHWs, tropical cyclones, and ESL events remain understudied. Forecasting of compound ocean extremes is still at a nascent stage.
2.3.3.1 Challenges in process-understanding and modeling
Cyclone track forecasts have improved remarkably in past decades (Zhao et al., 2017), however, the forecast of their intensity, intensification rates, and maximum intensity remains a big challenge with slow progress during the past few decades. Models are also significantly limited in their ability to simulate local convection and mesoscale convective systems (Ridder et al., 2021).
There is sparse research on the impacts of MHWs in the Indian Ocean (Saranya et al., 2022), and comprehensive studies are needed to better understand their interactions with TCs. The representation of complex topography and ocean dynamics along the Indian Ocean coastline is not well captured in models posing challenges to studies of coastal sea level rise.
2.3.3.2 Challenges due to inadequate data coverage
While observing and understanding the state of the Indian Ocean and its influence on climate and maritime resources is of critical importance to the populous nations that rim its border, major gaps in the Indian Ocean Observing System have affected the monitoring and forecasting of regional climate in recent times (Sprintall et al., 2024). Moreover, the lack of long-term, high-frequency data on marine physical and biogeochemical variables has been a major limitation for quantifying heat exchange and ecosystem processes in the Indian Ocean and associated compound extremes (Talley et al., 2016; Roemmich et al., 2019; Sprintall et al., 2024).
3 Recommendations
Given the challenges posed by compound events, recommendations need to consider both (1) strategies to advance the science of compound events (i.e., for addressing deficiencies in data and understanding), and (2) strategies for enhancing the practice of science toward the goal of translating cutting-edge science and technology towards useful and robust climate information to underpin more effective risk management interventions.
We elaborate on our key recommendations below, with a summary given in Figure 2.
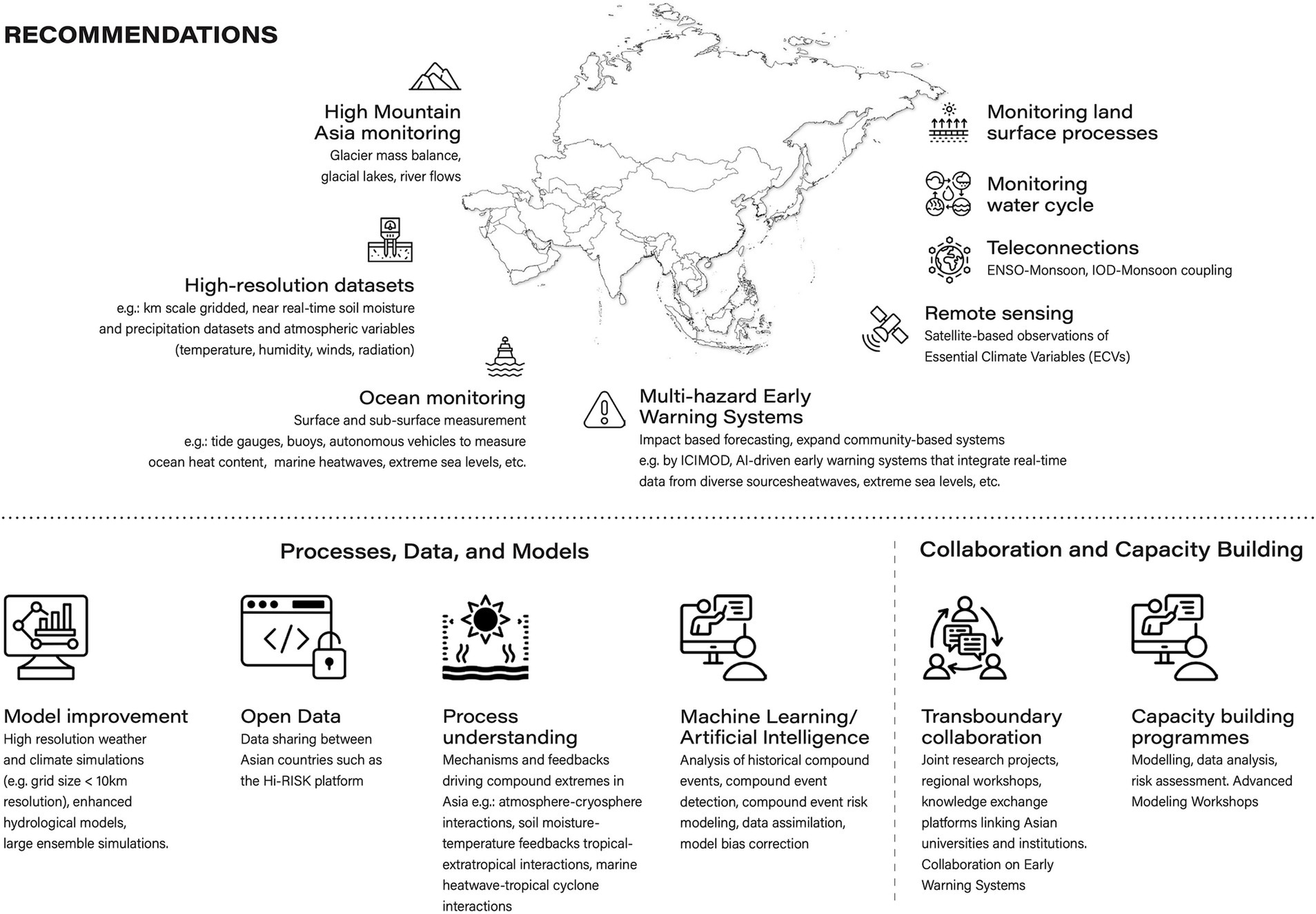
Figure 2. Recommendations to address scientific and data gaps to improve extreme and compound event forecasting in the Asian context. Schematic by Tejas AP.
3.1 Advancing scientific understanding
In an important paper, Emanuel (2020) suggested the need for a balanced approach of using models, observations and theory in order to advance research in atmospheres, oceans and climate. This concept paper recognizes and appreciates the value of the aforementioned approach, and asserts that an integrated Earth System approach is necessary towards addressing the key knowledge gaps pertaining to compound weather and climate extremes. With this perspective, it is essential to develop a strategy for monitoring, quantifying, and forecasting compound extremes in the Asian context. Recent recommendations from the climate modeling community (Stammer et al., 2024) emphasize the need for integrated approaches combining Earth system modeling, data assimilation (DA), and machine learning (ML) to improve our understanding of complex climate processes.
The first step would be to prepare a list of Essential Climate Variables (ECVs) relevant to this region. In this connection, there are already ongoing efforts to develop ECV inventories for the globe, and across Asia, using satellite measurements and in-situ observations. For example, https://gcos.wmo.int/en/essential-climate-variables/table, https://nices.nrsc.gov.in/ecv/ecv.php, https://earth.jaxa.jp/en/earthview/2020/11/30/2961/index.html. Monitoring ECVs and improving forecasts will require several advances in data collection and sharing, process understanding, and model improvement as we detail below.
3.1.1 Enhance observational networks
To improve our understanding of compound events, it is crucial to expand and enhance observational networks across various domains.
3.1.1.1 Asian land region
In High Mountain Asia, it is essential to improve earth system observations by enhancing real-time monitoring of glacier mass balance, glacier lakes, surface temperature, precipitation, mesoscale convective systems, atmospheric and land surface variables, river flows, and water availability.
Continuous monitoring of the spatio-temporal evolution of precipitation, surface temperature, soil moisture, glaciers and mesoscale convective systems, etc., using satellites, radars and ground-based observational networks together with high resolution weather and climate models are critical for improving forecasts of compound flood and drought events.
In order to improve the predictability of the Asian hydroclimatological system, it is essential to understand the physical processes, land-atmosphere interactions, and multiscale interactions associated with the diverse hydroclimatic conditions of the Asian continent through field campaigns, satellite observations, and high resolution model experiments (Terao et al., 2023).
3.1.1.2 Oceanic region
Enhancement of observational networks in the ocean context includes the following: (1) Continuous monitoring of sea level along the Indian Ocean coastline with increased density of GPS co-located tide gauges for accurate and high-resolution measurements of mean and extreme sea levels (2) Augmenting and strengthening the network of in situ moorings such as the Research Moored Array for African–Asian–Australian Monsoon Analysis and Prediction (RAMA) and Ocean Moored buoy Network for the Northern Indian Ocean (OMNI), needed to continuously monitor the ocean subsurface and evolution of marine heatwaves (MHWs) at high temporal resolution in the Indian Ocean. This observing system should include deploying more subsurface floats and autonomous vehicles equipped with temperature, salinity, and current sensors. (3) Continuous monitoring of ocean biogeochemistry, coastal circulation, hydrology and freshwater fluxes from land in order to assess the impacts of compound extremes on ocean and coastal ecosystems.
The implementation of low-cost buoy technology, as described by Liu et al. (2022), may dramatically reduce the cost of ocean observation. Lastly, there is a need to expand hazard data collection and real-time access across all domains to support comprehensive monitoring and early warning systems.
3.1.2 Advances in theory
Advancing theoretical understanding of compound events is crucial for improving our ability to predict and mitigate their impacts. In particular, it is essential to develop a hierarchy of models of varying complexities that can facilitate predictive understanding of how global climate change can alter the atmosphere–ocean-land-cryosphere coupled system, particularly the large-scale tropical Walker and Hadley circulations, the Asian monsoon circulation, large-scale SST gradients and organization of tropical convective systems, which are central to monsoon precipitation extremes over Asia (Held, 2005; Seager et al., 2019; Douville et al., 2021; Kalik et al., 2024; Krishnan et al., 2024).
Moreover, focused research on the mechanisms driving compound events, including interactions between MHWs, tropical cyclones, and extreme sea levels, is necessary in the Asian region. Increased research efforts are needed to develop a mechanistic understanding of compound events, focusing on the interactions between different climate processes and drivers, such as tropical-extratropical interactions, soil moisture-temperature feedbacks, and atmosphere-cryosphere interactions in the Third Pole (see Figure 1).
A key area requiring attention is the understanding of wave effects on air-sea fluxes and their role in the intensification of tropical cyclones (e.g., Zhao et al., 2017, 2022). Air-sea fluxes are turbulent processes that play a crucial role in the forecasting and prediction of weather and climate extremes. However, our scientific understanding of air-sea fluxes is quite limited. Scientific experiments and improvements in physical process-based parametrizations are needed to address this knowledge gap.
3.1.3 Improve modeling capabilities
Advancing modeling capabilities is crucial for better understanding and predicting compound events. Current generation climate models can represent extreme weather and climate events at large to regional scales, but capturing extremes at highly local scales remains a challenge (Ummenhofer and Meehl, 2017; Williams et al., 2024). High resolution climate models with improved representation of moisture fluxes and diurnally varying SST can significantly improve large-scale circulation and extremes (Haarsma et al., 2016; Bao et al., 2020; Li et al., 2024; Song et al., 2024) and have been shown to reduce systematic biases in climate models (Moreno-Chamarro et al., 2022).
Over the Asian region, increasing model resolution improves the spatial distribution and intensity of monsoon rainfall (Sabin et al., 2013; Krishnan et al., 2015; You and Ting, 2023). As moist physical processes play a major role in modulating tropical climate, a realistic representation of interactions between clouds and large-scale circulation is necessary to accurately predict and project hydroclimatic extremes over South and East Asia. This can be achieved through climate simulations using global storm-resolving/K-scale climate models (Bony et al., 2015; Satoh et al., 2019; Takahashi et al., 2020).
Recent studies have shown that K-scale models that resolve small-scale orography and cloud processes can generate realistic simulations of circulation, ITCZ, equatorial waves, Asian monsoon, tropical cyclones, intense precipitation characteristics, meso-scale eddies and Arctic sea ice leads (van Niekerk et al., 2018; Judt and Rios-Berrios, 2021; Rackow et al., 2024; Takasuka et al., 2024). Greenhouse gas warming simulations using storm-resolving models provide enhanced regional information on projected changes in tropical cyclones, extremes, and hydroclimatic teleconnection patterns (Moon et al., 2024).
Approaches to understand and project compound extremes over the Asian region should include climate-scale simulations using ultra-high resolution models for present and future climatic states. Projects like Horizon 2020 Next Generation Earth Modelling Systems (nextGEMS), Flagship Pilot Study (CORDEX-FPS) Convection-Permitting Third Pole (CPTP; Prein et al., 2023), “Ocean to climate Seamless forecasting System (OSF)” under UN Ocean Decade, and Earth Visualization Engine (Stevens et al., 2024) can reliably quantify how climate will change on a global to local scale and transform our understanding of local hazards and their impacts.
In addition to high-resolution simulations, there is a need to enhance hydrological models to accurately represent the interactions between different components of the water cycle, which are critical for predicting compound flooding events. Ocean models, particularly for improving MHW predictions, also require enhancement.
The development of new generation tropical cyclone models with improved air-sea flux representations is crucial. Coupling surface waves into Tropical Cyclone Models can pave a new way to improve the forecasting ability of TCs (Zhao et al., 2022; Babanin, 2023). Creating integrated models that couple atmospheric, oceanic, surface wave, and land surface processes will better simulate compound events. This includes improving the representation of soil moisture-temperature coupling and air-sea fluxes in models.
To better quantify uncertainties and improve the predictability of compound events, increased use of large ensemble simulations is recommended. This approach helps in understanding the range of possible future outcomes under different climate scenarios. Improving subseasonal-to-seasonal forecasting capabilities for extreme events is also crucial, involving the development of better initialization techniques and data assimilation methods to enhance model accuracy.
Accelerating efforts towards generating Earth System Reanalysis datasets, including energy, water, carbon, and biogeochemical cycles, will also help support Earth system model improvement (Stammer et al., 2024).
3.1.4 Use of AI/ML
Artificial Intelligence (AI) and Machine Learning (ML) methods offer transformative potential in understanding and predicting compound extreme events. These advanced computational techniques can process vast amounts of high-resolution data from various sources to identify patterns that might go unnoticed with traditional methods (McGovern et al., 2017). By leveraging these capabilities, AI and ML can potentially help create more accurate forecasting models for anticipating the occurrence and impact of compound extreme events (Huntingford et al., 2019; Kochkov et al., 2024).
AI/ML techniques excel at integrating diverse datasets—ranging from satellite imagery and reanalysis, to historical climate records—making them ideal for tackling the complex, interconnected nature of compound extremes (Karpatne et al., 2019). This holistic approach allows for the identification of correlations and interactions between different climate variables, providing a deeper understanding of the triggers and propagation of extreme events (Zscheischler et al., 2018).
The application of AI in climate science also enables the development of more sophisticated early warning systems. These systems can help provide timely alerts to communities and decision-makers, potentially saving lives and reducing economic losses (Shi et al., 2015). Furthermore, AI-driven simulations can help in scenario planning, allowing policymakers to explore the potential outcomes of different climate adaptation strategies and their effectiveness in mitigating the impacts of compound extremes (Ise and Oba, 2019).
However, the success of AI/ML applications in this field heavily depends on the quality and quantity of available data. Policymakers should prioritize investments in high-resolution data collection systems, particularly for soil moisture, oceanographic conditions, and atmospheric variables (AghaKouchak et al., 2020). This data infrastructure is crucial for training robust AI models and improving the accuracy of predictions.
In conclusion, the integration of AI and ML techniques into climate science represents a significant opportunity to enhance our understanding and prediction of compound extreme events. Policymakers can leverage these insights to craft more comprehensive climate action plans, emphasizing the importance of data availability and technological innovation in improving preparedness strategies and building resilience against the growing threats posed by climate change (ECMWF, 2021).
3.1.5 Early warning systems with multi-hazard impact based forecasts
Strengthening early warning systems (EWS) for compound events is critical for effective disaster risk reduction, particularly in regions frequently affected by multiple hazards such as those in Asia (e.g., Sahoo and Bhaskaran, 2018). To achieve this, it is essential to strengthen Multi-Hazard Early Warning Systems (MHEWS) at both national and regional levels. This involves enhancing cross-border data sharing and technical cooperation through regional initiatives like the Regional Integrated Multi-Hazard Early Warning System for Africa and Asia (RIMES), which supports member countries in establishing and maintaining robust MHEWS (UNDRR and WMO, 2023). In the Asian context, Bangladesh’s Cyclone Preparedness Programme (Haque et al., 2022) and Japan’s J-Alert emergency broadcast system are examples of successfully implemented EWS.
Impact-Based Forecasting can provide more actionable warnings, going beyond traditional hazard predictions by forecasting potential impacts, enabling decision-makers and communities to take preemptive actions (UNDRR and WMO, 2023; van den Hurk et al., 2023). Integrating “compound thinking,” i.e., the awareness, consideration, and improved representation of compound impact-drivers, into impact-based forecasting models can significantly improve risk assessments and preparedness strategies (van den Hurk et al., 2023; Stalhandske et al., 2024).
Making early warning systems more people-centered, and embedding community-based disaster preparedness activities within MHEWS, can empower local populations to respond swiftly and effectively to early warnings (Aguirre-Ayerbe et al., 2020; Trogrlić et al., 2022). This entails ensuring that warning messages are communicated in clear, understandable terms in the local language, tailored to local contexts and engaging local stakeholders in the design and implementation of EWS (Shrestha et al., 2021).
Expanding the use of advanced technology and data sharing is also crucial. Leveraging satellite technology, mobile networks, and internet-based platforms can enhance real-time hazard data dissemination and early warning delivery, reaching even the most remote areas (UNDRR and WMO, 2023). Integrating high-resolution observational data with advanced statistical, dynamical, and machine-learning-based modeling techniques can improve the accuracy and lead time of forecasts, making early warnings more reliable and actionable (Guntu and Agarwal, 2023).
Furthermore, to ensure the effectiveness and sustainability of MHEWS, it is important to develop strong risk governance mechanisms to facilitate better integration and management of MHEWS, enhancing their coverage and functionality (UNDRR and WMO, 2023).
Investing in capacity-building and education is recommended to enhance the understanding and utilization of EWS. Training personnel in modern hazard detection and forecasting tools, alongside educating the public on appropriate responses to warnings, ensures that EWS are effectively used and maintained. Practitioners should also be trained to manage compound events, improving their ability to interpret complex, multi-hazard scenarios (UNDRR and WMO, 2023; van den Hurk et al., 2023).
3.2 Strategies for enhancing the practice of science
Strategies to enhance the practice of science span initiatives within and across the scientific community, as well as innovations that reach beyond the traditional boundaries of the scientific community. Examples of the former include transboundary data-sharing and collaborations, and cross-disciplinal capacity building. Examples of the latter involve stakeholder engagement and inclusive decision-making, which includes the design and implementation of multi-hazard early warning systems, and risk assessment and management of compound events.
3.2.1 Improve data sharing and access
Promote open data policies to facilitate the sharing of high-resolution datasets across borders, particularly in Asia. This includes encouraging countries to contribute their observational data to global repositories. For instance, access of hydrographic data over coastal regions, particularly the Exclusive Economic Zones (EEZ), where data is not currently shared, is important for understanding coastal compound extremes. Regional collaborative platforms can also be developed, e.g., through meteorological and oceanic agencies, to enable this transboundary data-sharing and cooperation. These platforms should include data from surface observations, radar, satellite, and other remote sensing technologies. In this way, researchers can better collaborate on the causes, impacts, and potential solutions to increasing compound events, including transboundary impacts due to teleconnected feedbacks leading to propagating or cascading compound events. This enhanced cooperation will ultimately lead to more effective strategies for mitigating climate risks.
An example of a regional platform is HiRisk,1 a consortium of researchers mainly from Mountain Asia working on the specific challenges of the region, including producing and evaluating open access datasets relevant to risk assessment. A publication arising from this consortium that is relevant to compound events is that of Zhong et al. (2024) on the cascading process of rock and ice avalanches. This consortium framework can be reviewed and potentially adapted for other parts of Asia.
3.2.2 Regional collaborations
We need to explore mechanisms to better initiate, cultivate and sustain joint or collaborative research projects among Asian countries to study compound events. These projects should focus on data sharing, model development, and capacity building. Regional workshops and conferences can play a vital role in facilitating knowledge exchange and networking among scientists, policymakers, and practitioners. These events should also aim to identify research priorities and promote best practices for addressing compound events.
3.2.3 Interdisciplinary capacity-building
Training programs for researchers, meteorologists, and disaster management professionals need to be more deliberately developed to enhance their understanding of compound events and improve their technical skills (Dhara and Krishnan, 2022). These programs should cover advanced climate modeling techniques, data analysis, and early warning system implementation. A key feature would be the orientation of researchers in other relevant fields on the nexus of compounding events which might be beyond the boundaries of their respective specializations. As seen in the earlier case studies, compound events require an interdisciplinary approach—e.g., collaboration among meteorologists, oceanographers, hydrologists, climatologists, earth scientists—given that it is the interaction of hazards in space and/or time which enhances the potential for harm rather than the nature of the individual events (Ridder et al., 2022). The Kigali Declaration calls on the research community to invest in long-term education and skills development across Asia, which should promote “equal visibility, voice, and access to opportunity to early career scientists, marginalized scientists, and historically disadvantaged scientific communities (Section 2; World Climate Reserach Programme (WCRP), 2024).”
3.2.4 Transdisciplinary capacity-building and risk assessment
Beyond capacity-building and collaboration within the research community, a key concern is how to evolve and institutionalize mechanisms to effectively engage policy-makers, other sectoral representatives and the broader public in the co-design and co-production of research and actionable knowledge (Kigali Declaration, Section 2). A transdisciplinary approach brings together these diverse actors to co-create knowledge and develop solutions that are relevant to the local context. Collaboration and communication with these various actors are essential in effectively integrating perspectives, co-creating knowledge and implementing solutions (Alcántara-Ayala et al., 2024). In the case of compound extremes, an immediate potential application, as mentioned previously, is in strengthening multi-hazard early warning systems. However, research (e.g., the work by Ponce de Leon, 2020, 2021a, 2021b, 2023 on Typhoon Haiyan) has shown that improved science is not enough—leaders, decision-makers and other stakeholders receive, perceive and hence act on hazard and risk information in different ways. Hence, cooperation is necessary on different fronts, and with different stakeholders, to advance more holistic responses to mitigate the risks of compound extremes.
Recent research with European stakeholders has identified key challenges in implementing multi-hazard risk assessment and management (Trogrlić et al., 2024). These include governance issues (fragmented responsibilities, lack of coordination), knowledge gaps about multi-hazard interactions, limitations in existing risk management approaches, difficulties in translating science to practice, and data scarcity. The study highlights that these challenges are interconnected and cannot be tackled in isolation, and emphasizes the importance of engaging stakeholders early in the process to understand local contexts and constraints, while developing approaches that can bridge science-policy-practice divides. As an example of an ongoing initiative, the Coordinated Regional Climate Downscaling Experiment (CORDEX) Southeast Asia, a collaborative regional network of climate researchers, aims to increase engagement with local stakeholders in their current project, “Climatic hazard Assessment to enhance Resilience against climate Extremes for Southeast Asian megacities (CARE for SEA megacities),” funded by the Asia-Pacific Network for Global Change Research (APN). Priority hazards were identified in consultation with the local stakeholders at the beginning of the project to ideally increase the usefulness and relevance of the climate information that will be produced.2
Developing cooperation among stakeholders will require building capacities for transdisciplinary paradigms and approaches on all sides. In the case of compound events, information from the ground—e.g., structures and infrastructures that influence the cascade or co-occurrence of hazards, drivers of vulnerability—are vital for determining acceptable risk thresholds. On the one hand, scientists and researchers need to learn how to facilitate meaningful community inputs into the research process, and build long-term relationships with communities they serve. This will enable the provision of context-relevant information (Section 3, Kigali Declaration). On the other hand, practitioners, professionals and other community members need to articulate their experiences of events on the ground, and subsequently, their needs and priorities, desired information, and decision-making paradigms (i.e., their criteria for what makes information actionable in the context of multi-hazard and/or compound hazard risk assessments). Initiatives such as the My Climate Risk Lighthouse Activity3 precisely encourage a bottom-up approach which starts with the exploration and characterization of the decision-making context and hence, corresponding modeling and data needs, complementary to the typical top-down approach of constructing climate information and downscaling to the local level. Policy-makers need to facilitate mechanisms to bring marginalized and vulnerable groups to the table, to meaningfully influence decision-making and planning discussions and processes. For example, community-based early warning systems, such as those promoted by ICIMOD,4 espouse a “people-centered approach” with tools and plans “managed by and for communities.”
Most existing risk assessment frameworks or methods, including those conducted at a local level, e.g., as part of climate action plans, consider the impact of one hazard at a time (AghaKouchak et al., 2020) given its associated exposure and vulnerability factors. Thus, the consideration of compound events will require investigation into potential interactions of vulnerability drivers as well in the overall assessment of risk. Simpson et al. (2021), for example, propose new frameworks for increasing complexities of risk, such as with multiple interacting drivers within determinants of risk, appropriate for compound events, and interacting risks. Such approaches need to be piloted and made more mainstream in the Asian region.
In the Asian context, there are examples of risk assessments such as vulnerability assessment for the Indian coasts which have experienced multiple stresses from global climate change and human intervention (Sudha Rani et al., 2015). In particular, the stresses due to sea-level rise, coastal erosion, frequent extreme events and saltwater encroachment in the Indian coasts, have necessitated the development of coastal risk assessments which are essential for developing strategies to reduce coastal risks, protect coastal ecosystems and livelihoods and also improve management of coastal resources (Sudha Rani et al., 2015). While current methodologies for developing coastal vulnerability assessments are based on satellite remote sensing data, conventional data from ground observations and field reconnaissance survey and geographical information systems (GIS; Sudha Rani et al., 2015), the use of high-resolution observations, ML and Earth System reanalysis (Stammer et al., 2024) offers a promising opportunity to enhance risk assessments at local scales with increased granularity.
In addition, risk analysis in the context of compound events will need to consider policy and development decisions that not only influence vulnerability but may aggravate the hazard, e.g., urbanization, which enhances flooding during compound rainfall and storm surge events (due to increased impermeable surfaces that decrease soil drainage), and which enhances the severity of heat waves (due to the urban heat island effect). Innovative risk management approaches are needed to guard against potentially maladaptive responses to enhanced extremes, such as in the case of larger dams built to address potential droughts but which lead to wide-scale land use/land cover change affecting local climate, in addition to GHG emissions from the construction process and loss of terrestrial carbon sinks (AghaKouchak et al., 2020).
The potential feedback mechanisms between anthropogenic factors and the evolution of risk due to compound events require a systems approach, integrating improvements in modeling with bottom-up mechanisms and sources of knowledge (Rodrigues and Shepherd, 2022). A transdisciplinary approach (e.g., through co-production strategies) that engages stakeholders on the ground across all sectors is required to better understand the experience of hazards and the drivers of risk at a community level (Kigali Declaration, Section 3). Given the diverse cultures and contexts in the Asian region, coupled with the limits of model resolution, a grassroots or bottom-up approach, drawing from traditional local or indigenous knowledge (Petzold et al., 2020), can complement the “top-down” / high-level science, and help address the challenges brought about by heterogeneity of the Asian region.
Author contributions
RK: Conceptualization, Project administration, Supervision, Writing – original draft. CD: Conceptualization, Project administration, Supervision, Writing – original draft, Visualization. TH: Writing – original draft. AD: Writing – original draft. MS: Writing – original draft. PS: Writing – original draft. MR: Writing – original draft. S-WS: Writing – original draft. DA: Writing – original draft, Visualization. FC: Writing – original draft. FQ: Writing – original draft. CG: Writing – original draft.
Funding
The author(s) declare that financial support was received for the research, authorship, and/or publication of this article. SWS was supported by Institute of Information & communications Technology Planning & Evaluation (IITP) grant funded by the Korea government (MSIT) [NO.RS-2021-II211343, Artificial Intelligence Graduate School Program (Seoul National University)]. Qiao F. was supported by National Natural Science Foundation of China under grant No. 41821004. CKGG was partially supported by the Australian Government through the Australian Research Council (Discovery Project DP230101280).
Acknowledgments
We sincerely thank Manmeet Singh for providing inputs on the use of AI/ML for compound event forecasting. We are grateful to Tejas AP for preparing Figure 2 of this manuscript.
Conflict of interest
The authors declare that the research was conducted in the absence of any commercial or financial relationships that could be construed as a potential conflict of interest.
The author(s) declared that they were an editorial board member of Frontiers, at the time of submission. This had no impact on the peer review process and the final decision.
Generative AI statement
The author(s) declare that no Generative AI was used in the creation of this manuscript.
Publisher’s note
All claims expressed in this article are solely those of the authors and do not necessarily represent those of their affiliated organizations, or those of the publisher, the editors and the reviewers. Any product that may be evaluated in this article, or claim that may be made by its manufacturer, is not guaranteed or endorsed by the publisher.
Footnotes
2. ^https://cordex.org/2024/01/16/cordex-south-east-asia-inception-workshop-and-stakeholder-consultation/
References
Aadhar, S., and Mishra, V. (2023). The 2022 mega heatwave in South Asia in the observed and projected future climate. Environ. Res. Lett. 18:104011. doi: 10.1088/1748-9326/acf778
Abella, D. J., and Ahn, K.-H. (2024). Investigating the spatial and temporal characteristics of compound dry hazard occurrences across the pan-Asian region. Weather Clim. Extremes 44:100669. doi: 10.1016/j.wace.2024.100669
Adhikari, T. R., Baniya, B., Tang, Q., Talchabhadel, R., Gouli, M. R., Budhathoki, B. R., et al. (2023). Evaluation of post extreme floods in high mountain region: a case study of the Melamchi flood 2021 at the Koshi river basin in Nepal. Nat. Hazar. Res. 3, 437–446. doi: 10.1016/j.nhres.2023.07.001
AghaKouchak, A., Chiang, F., Huning, L. S., Love, C. A., Mallakpour, I., Mazdiyasni, O., et al. (2020). Climate extremes and compound hazards in a warming world. Annu. Rev. Earth Planet. Sci. 48, 519–548. doi: 10.1146/annurev-earth-071719-055228
Aguirre-Ayerbe, I., Merino, M., Aye, S. L., Dissanayake, R., Shadiya, F., and Lopez, C. M. (2020). An evaluation of availability and adequacy of multi-Hazard early warning Systems in Asian Countries: a baseline study. Int. J. Disaster Risk Reduct. 49:101749. doi: 10.1016/j.ijdrr.2020.101749
Alcántara-Ayala, I, Vogel, C, Kotani, M, Mooney, C, Singh Shrestha, M, and Leal de Moraes, OL (2024). “Towards pathways to sustainable futures: the role of transdisciplinary approaches to weather, climate, water and related environmental and social sciences”. In: United in science 2024, World Meteorological Organization. Available at: https://wmo.int/publication-series/united-science-2024 (Accessed November 5, 2024).
Allen, C. D., Macalady, A. K., Chenchouni, H., Bachelet, D., McDowell, N., Vennetier, M., et al. (2010). A global overview of drought and heat-induced tree mortality reveals emerging climate change risks for forests. For. Ecol. Manag. 259, 660–684. doi: 10.1016/j.foreco.2009.09.001
Alory, G., and Meyers, G. (2009). Warming of the upper equatorial Indian Ocean and changes in the heat budget (1960–99). J. Clim. 22, 93–113. doi: 10.1175/2008JCLI2330.1
Annamalai, H. (2024). Unprecedented monsoon precipitation over Southwest Pakistan in 2022: regional processes in moistening the climatological heat low. Q. J. R. Meteorol. Soc. 150, 4391–4416. doi: 10.1002/qj.4821
Arias, P. A., Bellouin, N., Coppola, E., Jones, R. G., Krinner, G., Marotzke, J., et al. (2021). “Technical summary” in Climate change 2021: The physical science basis. Contribution of working group I to the sixth assessment report of the intergovernmental panel on climate change. eds. V. Masson-Delmotte, P. Zhai, A. Pirani, S. L. Connors, C. Péan, and S. Berger, et al. (Cambridge, United Kingdom and New York, NY, USA: Cambridge University Press), 33–144.
Atwii, F, Sandvik, K, Kirch, L, Paragi, B, Radtke, K, Schneider, S, et al. (2022). World risk report 2022. Bündnis Entwicklung Hilft. Available at: https://weltrisikobericht.de/wp-content/uploads/2022/09/WorldRiskReport-2022_Online.pdf
Ayantika, D. C., Sumit, K. M., Krishnan, R., Vellore, R. K., and Guhathakurta, P. (2024). A mechanistic investigation into the unusual intensification of rainfall over Western India during the 2019 summer monsoon. Atmos. Res. 299:107209. doi: 10.1016/j.atmosres.2023.107209
Babanin, A. V. (2023). Ocean waves in large-scale air-sea weather and climate systems. J. Geophys. Res. Oceans 128:e2023JC019633. doi: 10.1029/2023JC019633
Bajracharya, S. R., Mool, P. K., and Shrestha, B. R. (2007). Impact of climate change on himalayan glaciers and glacial lakes - case studies on GLOF and associated hazards in Nepal and Bhutan. Kathmandu, Nepal: ICIMOD, 1–127.
Balaji, M., Chakraborty, A., and Mandal, M. (2018). Changes in tropical cyclone activity in North Indian Ocean during satellite era (1981–2014). Int. J. Climatol. 38, 2819–2837. doi: 10.1002/joc.5463
Bao, Y., Song, Z., and Qiao, F. (2020). FIO-ESM version 2.0: model description and evaluation. J. Geophys. Res. Oceans 125:e2019JC016036. doi: 10.1029/2019JC016036
Basheer Ahammed, K. K., and Pandey, A. C. (2021). Characterization and impact assessment of super cyclonic storm AMPHAN in the Indian subcontinent through space borne observations. Ocean Coast. Manag. 205:105532. doi: 10.1016/j.ocecoaman.2021.105532
Becker, A., Ng, A. K. Y., McEvoy, D., and Mullett, J. (2018). Implications of climate change for shipping: ports and supply chains. WIREs Clim. Change 9:e508. doi: 10.1002/wcc.508
Boers, N., Goswami, B., Rheinwalt, A., Bookhagen, B., Hoskins, B., and Kurths, J. (2019). Complex networks reveal global pattern of extreme-rainfall teleconnections. Nature 566, 373–377. doi: 10.1038/s41586-018-0872-x
Bohner, J. (2006). General climatic controls and topoclimatic variations in central and high Asia. Boreas 35, 279–295. doi: 10.1080/03009480500456073
Bolch, T., Joseph, M. S., Shiyin, L., Farooq, M. A., Yang, G., Stephan, G., et al. (2019). “Status and change of the cryosphere in the extended Hindu Kush Himalaya region” in The Hindu Kush Himalaya assessment. eds. P. Wester, A. Mishra, A. Mukherji, and A. Shrestha (Cham: Springer).
Bony, S., Stevens, B., Frierson, D. M. W., Jakob, C., Kageyama, M., Pincus, R., et al. (2015). Clouds, circulation and climate sensitivity. Nat. Geosci. 8, 261–268. doi: 10.1038/ngeo2398
Boos, W. R., and Kuang, Z. (2010). Dominant control of the south Asian monsoon by orographic insulation versus plateau heating. Nature 463:8707. doi: 10.1038/nature08707
Bruen, M., Witnik, J., and Sthapit, B. (2017). Surviving three natural disasters: lessons learned at upper Bhote Koshi in. Nepal Hydro Rev. 36.
Chatterjee, A., Anil, G., and Shenoy, L. R. (2022). Marine heatwaves in the Arabian Sea. Ocean Sci. 18, 639–657. doi: 10.5194/os-18-639-2022
Chettri, Mona (2023). “Who Is Responsible for Sikkim’s Glacial Lake Outburst Flood?” Frontline. Available at: https://frontline.thehindu.com/environment/article67453490.ece (Accessed November 5, 2024).
Chiang, F., Mazdiyasni, O., and AghaKouchak, A. (2018). Amplified warming of droughts in southern United States in observations and model simulations. Science. Advances 4:eaat2380. doi: 10.1126/sciadv.aat2380
Choi, H. Y., Park, M. S., Kim, H. S., and Lee, S. (2024). Marine heatwave events strengthen the intensity of tropical cyclones. Commun. Earth Environ. 5:69. doi: 10.1038/s43247-024-01239-4
Clarke, B., Otto, F., Stuart-Smith, R., and Harrington, L. (2022). Extreme weather impacts of climate change: An attribution perspective. Environ. Res. Clim. 1:012001. doi: 10.1088/2752-5295/ac6e7d
Cohen, J., Zhang, X., Francis, J., Jung, T., Kwok, R., Overland, J., et al. (2020). Divergent consensuses on Arctic amplification influence on Midlatitude severe winter weather. Nat. Clim. Chang. 10, 20–29. doi: 10.1038/s41558-019-0662-y
Collier, E., Ban, N., Richter, N., Ahrens, B., Chen, D., Chen, X., et al. (2024). The first ensemble of kilometer-scale simulations of a hydrological year over the third pole. Clim. Dyn. 62, 7501–7518. doi: 10.1007/s00382-024-07291-2
Collins, M., Knutti, R., Arblaster, J., Dufresne, J.-L., Fichefet, T., Friedlingstein, P., et al. (2013). Long-term climate change: Projections, commitments and irreversibility. Cambridge, UK: Cambridge University Press, 1029–1136.
Collins, M., Sutherland, M., Bouwer, L., Cheong, S.-M., Frölicher, T., Combes, H. J. D., et al. (2019). “Extremes, abrupt changes and managing risks” in IPCC special report on oceans and cryosphere in a changing climate. eds. Portner, et al. (Cambridge, UK and New York, NY, USA: Cambridge University Press), 589–655.
Dave, R., Subramanian, S. S., and Bhatia, U. (2021). Extreme precipitation induced concurrent events trigger prolonged disruptions in regional road networks. Environ. Res. Lett. 16:104050. doi: 10.1088/1748-9326/ac2d67
Deshpande, M., Singh, V. K., Ganadhi, M. K., Roxy, M. K., Emmanuel, R., and Kumar, U. (2021). Changing status of tropical cyclones over the North Indian Ocean. Clim. Dyn. 57, 3545–3567. doi: 10.1007/s00382-021-05880-z
Dhara, C., and Koll, R. M. (2023). How a warming Pacific Ocean and climate change will impact agriculture. Nat. India. doi: 10.1038/d44151-023-00093-1
Dhara, C., and Krishnan, R. (2020). “Possible climate change impacts and policy-relevant messages” in Assessment of climate change over the Indian region (Singapore: Springer Singapore), 223–226.
Dhara, Chirag, and Krishnan, R. (2022). “Advancing south Asian monsoon climate change projections: challenges and opportunities.” J. Indian Geophys. Union 26, 245–257.
Do, H. S., Kim, J., Cha, E.-J., Chang, E. C., Son, S. W., and Lee, G. (2023). Long-term change of summer mean and extreme precipitations in Korea and East Asia. Int. J. Climatol. 43, 3476–3492. doi: 10.1002/joc.8039
Doi, T., Behera, S. K., and Yamagata, T. (2024). Seasonal predictability of the extreme Pakistani rainfall of 2022 possible contributions from the northern coastal Arabian Sea temperature. NPJ Clim. Atmos. Sci. 7:13. doi: 10.1038/s41612-023-00557-2
Domeisen, D. I. V., White, C. J., Afargan-Gerstman, H., Muñoz, A. G., Janiga, M. A., Vitart, F., et al. (2022). Advances in the subseasonal prediction of extreme events: relevant case studies across the globe. Bull. Am. Meteorol. Soc. 103, E1473–E1501. doi: 10.1175/BAMS-D-20-0221.1
Dong, Z., Wang, L., Sun, Y., Hu, T., Limsakul, A., Singhruck, P., et al. (2021). Heatwaves in Southeast Asia and their changes in a warmer world. Earth’s Future 9:7. doi: 10.1029/2021EF001992
Douville, H., Raghavan, K., Renwick, J. A., Allan, R. P., Arias, P. A., Barlow, M., et al. (2021, 2021). “Water cycle changes” in Climate change 2021: The physical science basis. Contribution of working group I to the sixth assessment report of the intergovernmental panel on climate change. eds. V. Masson-Delmotte, P. Zhai, A. Pirani, S. L. Connors, C. Péan, and S. Berger, et al. (Cambridge, United Kingdom and New York, NY, USA: Cambridge University Press), 1055–1210.
Dubey, S., Sattar, A., Gupta, V., Goyal, M. K., Haritashya, V. K., and Kargel, J. S. (2024). Transboundary hazard and downstream impact of glacial lakes in Hindu-Kush Karakoram Himalayas. Sci. Total Environ. 914:169758. doi: 10.1016/j.scitotenv.2023.169758
Emanuel, K. (2020). The relevance of theory for contemporary research in atmospheres, oceans, and climate. AGU Adv. 1:129. doi: 10.1029/2019AV000129
Eyer, J., and Wichman, C. J. (2018). Does water scarcity shift the electricity generation Mix toward fossil fuels? Empirical evidence from the United States. J. Environ. Econ. Manag. 87, 224–241. doi: 10.1016/j.jeem.2017.07.002
Farinotti,, Immerzeel, W. W., de Kok, R. J., Quincey, D. J., and Dehecq, A. (2020). Manifestations and mechanisms of the Karakoram glacier anomaly. Nat. Geosci. 13, 8–16. doi: 10.1038/s41561-019-0513-5
Forster, P. M., Smith, C. J., Walsh, T., Lamb, W. F., Lamboll, R., Hauser, M., et al. (2023). Indicators of global climate change 2022: annual update of large-scale indicators of the state of the climate system and human influence. Earth Syst. Sci. Data 15, 2295–2327. doi: 10.5194/essd-15-2295-2023
Forsythe, N., Fowler, H. J., Li, X.-F., Blenkinsop, S., and Pritchard, D. (2017). Karakoram temperature and glacial melt driven by regional atmospheric circulation variability. Nat. Clim. Chang. 7, 664–670. doi: 10.1038/nclimate3361
Fox-Kemper, B., Hewitt, H. T., Xiao, C., Aðalgeirsdóttir, G., Drijfhout, S. S., Edwards, T. L., et al. (2021). “Ocean, cryosphere and sea level change” in Climate change 2021: The physical science basis. Contribution of working group I to the sixth assessment report of the intergovernmental panel on climate change. eds. V. Masson Delmotte, P. Zhai, A. Pirani, S. L. Connors, C. Péan, and S. Berger, et al. (Cambridge, United Kingdom and New York, NY, USA: Cambridge University Press).
Francis, J. A. (2017). Why are Arctic linkages to extreme weather still up in the air? Bull. Am. Meteorol. Soc. 98, 2551–2557. doi: 10.1175/BAMS-D-17-0006.1
Francis, J. A., Vavrus, S. J., and Cohen, J. (2017). Amplified Arctic warming and mid-latitude weather: New perspectives on emerging connections. WIREs Clim. Change 8:e474. doi: 10.1002/wcc.474
Ganeshi, N. G., Mujumdar, M., Takaya, Y., Goswami, M. G., Singh, B. B., Krishnan, R., et al. (2023). Soil moisture revamps the temperature extremes in a warming climate over India. Npj climate and atmospheric. Science 6:12. doi: 10.1038/s41612-023-00334-1
Ganguli, P. (2023). Amplified risk of compound heat stress-dry spells in urban India. Clim. Dyn. 60, 1061–1078. doi: 10.1007/s00382-022-06324-y
Guntu, R. K., and Agarwal, A. (2021). Disentangling increasing compound extremes at regional scale during Indian summer monsoon. Sci. Rep. 11:16447. doi: 10.1038/s41598-021-95775-0
Guntu, R. K., and Agarwal, A. (2023). Improving the predictability of compound dry and hot extremes through complexity science. Environ. Res. Lett. 18:124048. doi: 10.1088/1748-9326/ad0c0c
Guntu, R. K., Merz, B., and Agarwal, A. (2023). Increased likelihood of compound dry and hot extremes in India. Atmos. Res. 290:6789. doi: 10.1016/j.atmosres.2023.106789
Ha, K. J., Yeo, J. H., Seo, Y. W., Chung, E. S., Moon, J. Y., Feng, X., et al. (2020). What caused the extraordinarily hot 2018 summer in Korea? Kishō shūshi 98, 153–167. doi: 10.2151/jmsj.2020-009
Haarsma, R. J., Roberts, M. J., Vidale, P. L., Senior, C. A., Bellucci, A., Bao, Q., et al. (2016). High resolution model intercomparison project (HighResMIP v1.0) for CMIP6. Geosci. Model Dev. Discuss. 9, 4185–4208. doi: 10.5194/gmd-9-4185-2016
Hao, Z., Hao, F., Singh, V. P., and Zhang, X. (2018). Changes in the severity of compound drought and hot extremes over global land areas. Environ. Res. Lett. 13:124022. doi: 10.1088/1748-9326/aaee96
Hao, Z., Hao, F., Xia, Y., Feng, S., Sun, C., Zhang, X., et al. (2022). Compound droughts and hot extremes: characteristics, drivers, changes, and impacts. Earth Sci. Rev. 235:104241. doi: 10.1016/j.earscirev.2022.104241
Hao, Z., and Singh, V. P. (2020). Compound events under global warming: a dependence perspective. J. Hydrol. Eng. 25:03120001. doi: 10.1061/(ASCE)HE.1943-5584.0001991
Haque, U., da Silva, P. F., Devoli, G., Pilz, J., Zhao, B., Khaloua, A., et al. (2019). The human cost of global warming: deadly landslides and their triggers (1995–2014). Sci. Total Environ. 682, 673–684. doi: 10.1016/j.scitotenv.2019.03.415
Haque, A., Haider, D., Rahman, M. S., Kabir, L., and Lejano, R. P. (2022). Building resilience from the grassroots: the cyclone preparedness programme at 50. Int. J. Environ. Res. Public Health 19:14503. doi: 10.3390/ijerph192114503
Held, I. M. (2005). The gap between simulation and understanding in climate Modeling. Bull. Am. Meteorol. Soc. 86, 1609–1614. doi: 10.1175/BAMS-86-11-1609
Hoell, A., Robinson, R., Agel, L., Barlow, M., Breeden, M., Eischeid, J., et al. (2023). Changes to Middle East and Southwest Asia compound drought and heat since 1999. J. Clim. 37, 269–287. doi: 10.1175/JCLI-D-23-0194.1
Hong, C.-C., Huang, A.-Y., Hsu, H.-H., Tseng, W.-L., Mong-Ming, L., and Chang, C.-C. (2023). Causes of 2022 Pakistan flooding and its linkage with China and Europe heatwaves. NPJ Clim. Atmos. Sci. 6:163. doi: 10.1038/s41612-023-00492-2
Horinouchi, T., Kosaka, Y., Nakamigawa, H., Sattar, A., Gupta, V., Goyal, M. K., et al. (2021). Moisture supply, jet, and silk-road wave train associated with the prolonged heavy rainfall in Kyushu, Japan in early July 2020. SOLA 17B, 1–8. doi: 10.2151/sola.2021-019
Houze, R. A. (2004). Mesoscale convective systems. Rev. Geophys. 42, 1–43. doi: 10.1029/2004RG000150
Huntingford, C., Jeffers, E. S., Bonsall, M. B., Christensen, H. M., Lees, T., and Yang, H. (2019). Machine learning and artificial intelligence to aid climate change research and preparedness. Environ. Res. Lett. 14:124007. doi: 10.1088/1748-9326/ab4e55
ICIMOD (2023) in Water, ice, society, and ecosystems in the Hindu Kush Himalaya: An outlook. eds. P. Wester, S. Chaudhary, N. Chettri, M. Jackson, A. Maharjan, and S. Nepal, et al. (Kathmandu, Nepal: ICIMOD).
IPCC (2021). “Summary for policymakers” in Climate change 2021: The physical science basis. Contribution of working group I to the sixth assessment report of the intergovernmental panel on climate change. eds. V. P. Masson Delmotte, A. P. Zhai, S. L. Connors, C. Péan, S. Berger, and N. Caud, et al. (Cambridge, United Kingdom and New York, NY, USA: Cambridge University Press), 3–32. (Accessed September 5, 2024).
IPCC (2022). “Summary for policymakers.” In Climate change 2022 – Impacts, adaptation and vulnerability: Working group II contribution to the sixth assessment report of the intergovernmental panel on climate change, 1st ed. Cambridge, UK and New York, NY, USA: Cambridge University Press, (2022).
IPCC (2023). “Summary for policymakers.” In Climate change 2023 – Synthesis Report. Contribution of Working Groups I, II and III to the Sixth Assessment Report of the Intergovernmental Panel on Climate Change [Core Writing Team, H. Lee and J. Romero (eds.)]. Geneva, Switzerland: IPCC.
IPCC AR6 (2021). IPCC AR6 Regional Fact Sheet – Asia. Available at: https://www.ipcc.ch/report/ar6/wg1/downloads/factsheets/IPCC_AR6_WGI_Regional_Fact_Sheet_Asia.pdf.
Ise, T., and Oba, Y. (2019). Forecasting climatic trends using neural networks: An experimental study using global historical data. Front. Robot. AI 6:32. doi: 10.3389/frobt.2019.00032
Judt, F., and Rios-Berrios, R. (2021). Resolved convection improves the representation of equatorial waves and tropical rainfall variability in a global nonhydrostatic model. Geophys. Res. Lett. 48:e2021GL093265. doi: 10.1029/2021GL093265
Jyoti, J., Swapna, P., Krishnan, R., and Naidu, C. V. (2019). Pacific modulation of accelerated South Indian Ocean Sea level rise during the early 21st century. Clim. Dyn. 53:2019. doi: 10.1007/s00382-019-04795-0
Kala, J., De Kauwe, M. G., Pitman, A. J., Medlyn, B. E., Wang, Y.-P., Lorenz, R., et al. (2016). Impact of the representation of stomatal conductance on model projections of heatwave intensity. Sci. Rep. 6:23418. doi: 10.1038/srep23418
Kalik, V, Krishnan, R, Ayantika, DC, Swapna, P, Singh, M, Sandeep, N, et al. (2024): Understanding the response of tropical overturning circulations to greenhouse gas and aerosol forcing. Environ. Res. Clim. 3, 1–20.
Kapnick, S. B., Delworth, T. L., Ashfaq, M., Malyshev, S., and Milly, P. C. D. (2014). Snowfall less sensitive to warming in Karakoram than in Himalayas due to a unique seasonal cycle. Nat. Geosci. 7, 834–840. doi: 10.1038/ngeo2269
Karpatne, A., Ebert-Uphoff, I., Ravela, S., Babaie, H. A., and Kumar, V. (2019). Machine learning for the geosciences: challenges and opportunities. IEEE Trans. Knowl. Data Eng. 31, 1544–1554. doi: 10.1109/TKDE.2018.2861006
Kirezci, E., Young, I. R., Ranasinghe, R., Muis, S., Nicholls, R. J., Lincke, D., et al. (2020). Projections of global-scale extreme sea levels and resulting episodic coastal flooding over the 21st century. Sci. Rep. 10:11629. doi: 10.1038/s41598-020-67736-6
Kochkov, D., Yuval, J., Langmore, I., Norgaard, P., Smith, J., Mooers, G., et al. (2024). Neural general circulation models for weather and climate. Nature 632, 1060–1066. doi: 10.1038/s41586-024-07744-y
Kong, Q., Guerreiro, S. B., Blenkinsop, S., Li, X. F., and Fowler, H. J. (2020). Increases in summertime concurrent drought and heatwave in eastern China. Weather Clim. Extremes 28:100242. doi: 10.1016/j.wace.2019.100242
Kornhuber, K., and Tamarin-Brodsky, T. (2021). Future changes in northern hemisphere summer weather persistence linked to projected Arctic warming. Geophys. Res. Lett. 48, no. 4:e2020GL091603. doi: 10.1029/2020GL091603
Krishnan, R., Gnanaseelan, C., Sanjay, J., Swapna, P., Chirag Dhara, T. P., Sabin, J. J., et al. (2020). Introduction to climate change over the Indian region. Assessment of climate change over the Indian region. Singapore: Springer Singapore.
Krishnan, R., Sabin, T. P., Vellore, R., Mujumdar, M., Sanjay, J., Goswami, B. N., et al. (2015). Deciphering the desiccation trend of the south Asian monsoon hydroclimate in a warming world. Clim. Dyn. 47, 1007–1027. doi: 10.1007/s00382-015-2886-5
Krishnan, R., Vishisth, Kalik, Ayantika, D.C., Kumar, Sumit, and Vellore, R.K. (2024): Climate change and monsoon rainstorms. Monograph on severe storms: Anatomy, early warning systems and aftermath in changing climate scenarios -Editors: Someshwar Das and Wei-Kuo Tao, Singapore: Springer Nature (In Press).
Krishnan, R., Shrestha, A. B., Ren, G., Rajbhandari, R., Saeed, S., Sanjay, J., et al. (2019). “Unravelling climate change in the Hindu Kush Himalaya: rapid warming in the mountains and increasing extremes” in The Hindu Kush Himalaya Assessment: Mountains, Climate Change, Sustainability and People. eds. P. Wester, A. Mishra, A. Mukherji, and A. Shrestha (Cham: Springer). doi: 10.1007/978-3-319-92288-1_3
Lau, W. K. M., and Kim, K.-M. (2012). The 2010 Pakistan flood and Russian heat wave: teleconnection of Hydrometeorological extremes. J. Hydrometeorol. 13, 392–403. doi: 10.1175/JHM-D-11-016.1
Lee, D.-K., and Cha, D.-H. (2020). Regional climate Modeling for Asia. Geosci. Lett. 7:13. doi: 10.1186/s40562-020-00162-8
Li, X.-X. (2020). Heat wave trends in Southeast Asia during 1979-2018: the impact of humidity. Sci. Total Environ. 721:137664. doi: 10.1016/j.scitotenv.2020.137664
Li, M., Cao, Z., Gordon, A. L., Zheng, F., and Wang, D. (2023). Roles of the indo-Pacific subsurface kelvin waves and volume transport in prolonging the triple-dip 2020–2023 La Nina. Environ. Res. Lett. 18:104043. doi: 10.1088/1748-9326/acfcce
Li, X.-X., Yuan, C., and Hang, J. (2022). Heat wavetrends in Southeast Asia: comparison of results from observation and reanalysis data. Geophys. Res. Lett. 49:e2021GL097151. doi: 10.1029/2021GL097151
Li, S., Zhao, B., Ma, S., Yin, X., Ji, D., and Qiao, F. (2024). Effects of sea spray on extreme precipitation forecasting: a case study in Beijing of China. Geophys. Res. Lett. 51:e2024GL109923. doi: 10.1029/2024GL109923
Liu, C. (2011). Rainfall contributions from precipitation systems with different sizes, convective intensities, and durations over the tropics and subtropics. J. Hydrometeorol. 12, 394–412. doi: 10.1175/2010JHM1320.1
Liu, Y., Li, M., Liu, Y., Wang, Y., Jiang, S., Wang, Y., et al. (2022). Real-time precise measurements of ocean surface waves using GNSS variometric approach. Int. J. Appl. Earth Obs. Geoinf. 115:103125. doi: 10.1016/j.jag.2022.103125
Luo, Y., Liu, L., Zhang, Y., Mehmood, S., Yang, Y., Yang, G., et al. (2024). The impact of Tropical Sea surface temperature on extreme precipitation in Pakistan during the summer of 2022. Environ. Res. Lett. 19:044012. doi: 10.1088/1748-9326/ad2f77
Maharjan, S. B., Steiner, J. F., Shrestha, A. B., Maharjan, A., Nepal, S., Shrestha, M. S., et al. (2021). The Melamchi flood disaster. Cascading hazard and the need for multi hazard risk management. ICIMOD.
Malik, N., Bookhagen, B., Marwan, N., and Kurths, J. (2012). Analysis of spatial and temporal extreme monsoonal rainfall over South Asia using complex networks. Clim. Dyn. 39, 971–987. doi: 10.1007/s00382-011-1156-4
Mallapaty, S. (2022). Why are Pakistan’s floods so extreme this year? Nature. doi: 10.1038/d41586-022-04502-w
Martius, O., Sodemann, H., Joos, H., Pfahl, S., Winschall, A., Croci-Maspoli, M., et al. (2013). The role of upper-level dynamics and surface processes for the Pakistan flood of July 2010. Q. J. R. Meteorol. Soc. 139, 1780–1797. doi: 10.1002/qj.2082
Masson-Delmotte, V., Zhai, P., Pirani, A., Connors, S. L., Péan, C., Berger, S., et al. (2021). “Climate change 2021: the physical science basis” in Contribution of working group I to the sixth assessment report of the intergovernmental panel on climate change. (Cambridge, United Kingdom and New York, NY, USA: Cambridge University Press).
Mawren, D. J., and Hermes, C. J. C. R. (2022). Marine heat waves and tropical cyclones - two devastating types of coastal hazard in South-Eastern Africa. Estuar. Coast. Shelf Sci. 277:108056.doi: 10.1016/j.ecss.2022.108056
McGovern, A., Elmore, K. L., Gagne, D. J., Haupt, S. E., Karstens, C. D., Lagerquist, R., et al. (2017). Using artificial intelligence to improve real-time decision-making for high-impact weather. Bull. Am. Meteorol. Soc. 98, 2073–2090. doi: 10.1175/BAMS-D-16-0123.1
Met Office (2024). “Indicators of global warming.” Climate Dashboard. Available at: https://climate.metoffice.cloud/current_warming.html (Accessed November 5, 2024).
Miralles, D. G., Gentine, P., Seneviratne, S. I., and Teuling, A. J. (2019). Land–atmospheric feedbacks during droughts and heatwaves: state of the science and current challenges. Ann. N. Y. Acad. Sci. 1436, 19–35. doi: 10.1111/nyas.13912
Mishra, V., Thirumalai, K., Singh, D., and Aadhar, S. (2020). Future exacerbation of hot and dry summer monsoon extremes in India. NPJ Clim. Atmos. Sci. 3, 1–9. doi: 10.1038/s41612-020-0113-5
Moon, J.-Y., Streffing, J., Lee, S.-S., Semmler, T., Andrés-Martínez, M., Chen, J., et al. (2024). Earth's future climate and its variability simulated at 9 km global resolution. EGUsphere, 1–46. doi: 10.5194/egusphere-2024-2491
Moreno-Chamarro, E., Caron, L. P., Loosveldt Tomas, S., Vegas-Regidor, J., Gutjahr, O., Moine, M. P., et al. (2022). Impact of increased resolution on long-standing biases in HighResMIP-PRIMAVERA climate models. Geosci. Model Dev. 15, 269–289. doi: 10.5194/gmd-15-269-2022
Mujumdar, M., Preethi, B., Sabin, T. P., Ashok, K., Saeed, S., Pai, D. S., et al. (2012). The Asian summer monsoon response to the La Niña event of 2010. Meteorol. Appl. 19, 216–225. doi: 10.1002/met.1301
Murakami, H., Vecchi, G. A., and Underwood, S. (2017). Increasing frequency of extremely severe cyclonic storms over the Arabian Sea. Nat. Clim. Chang. 7, 885–889. doi: 10.1038/s41558-017-0008-6
Nanditha, J. S., Kushwaha, A. P., Singh, R., Malik, I., Solanki, H., Chuphal, D. S., et al. (2023). The Pakistan flood of august 2022: causes and implications. Earth’s. Earths Fut. 11:e2022EF003230. doi: 10.1029/2022EF003230
Ni, Y., Qiu, B., Miao, X., Li, L., Chen, J., Tian, X., et al. (2024). Shift of soil moisture-temperature coupling exacerbated 2022 compound hot-dry event in eastern China. Environ. Res. Lett. 19:014059. doi: 10.1088/1748-9326/ad178c
Noh, E., Kim, J., Jun, S. Y., Cha, D. H., Park, M. S., Kim, J. H., et al. (2021). The role of the Pacific-Japan pattern in extreme heatwaves over Korea and Japan. Geophys. Res. Lett. 48:e2021GL093990. doi: 10.1029/2021GL093990
Otto, F. E. L. (2017). Attribution of weather and climate events. Annu. Rev. Environ. Resour. 42, 627–646. doi: 10.1146/annurev-environ-102016-060847
Otto, F. E. L., Zachariah, M., Saeed, F., Siddiqi, A., Kamil, S., Haris Mushtaq, T., et al. (2023). Climate change increased extreme monsoon rainfall, flooding highly vulnerable communities in Pakistan. Environ. Res. Clim. 2:025001. doi: 10.1088/2752-5295/acbfd5
Park, C., Kang, M. J., Hwang, J., Cho, H-O., Kim, S., and Son, S-W. (2024). Multiscale drivers of catastrophic heavy rainfall event in early august 2022 in South Korea.
Park, C., Son, S.-W., and Kim, H. (2021a). Distinct features of atmospheric rivers in the early versus late east Asian summer monsoon and their impacts on monsoon rainfall. J. Geophys. Res. Atmos. 126:e2020JD033537. doi: 10.1029/2020JD033537
Park, C., Son, S. W., Kim, H., Ham, Y. G., Kim, J., Cha, D. H., et al. (2021b). Record-breaking summer rainfall in South Korea in 2020: synoptic characteristics and the role of large-scale circulations. Mon. Weather Rev. 149, 3085–3100. doi: 10.1175/MWR-D-21-0051.1
Petzold, J., Andrews, N., Ford, J. D., Hedemann, C., and Postigo, J. C. (2020). Indigenous knowledge on climate change adaptation: a global evidence map of academic literature. Environ. Res. Lett. 15:113007. doi: 10.1088/1748-9326/abb330
Ponce de Leon, I. Z. (2020). Of warnings and waiting: An examination of the path of information for two communities hit by typhoon Haiyan. J. Risk Res. 23, 598–612. doi: 10.1080/13669877.2019.1592212
Ponce de Leon, I. Z. (2021a). A portrait of contrasts in disaster risk response: a post-Haiyan study of Coron, Philippines. Weather Clim. Soc. 13, 511–523. doi: 10.1175/WCAS-D-20-0093.1
Ponce de Leon, I. Z. (2021b). The purok system of San Francisco, Camotes: a communication perspective of community-based Haiyan response. Int. J. Disaster Risk Reduct. 61:102379. doi: 10.1016/j.ijdrr.2021.102379
Ponce de Leon, I. Z. (2023). Good Neighbors, good response: Roxas, Capiz post-Haiyan. J. Int. Crisis Risk Commun. Res. 6, 1–32. doi: 10.30658/jicrcr.6.1.1
Pörtner, H.-O., Roberts, D. C., Adams, H., Adelekan, I., Rita Adrian, C. A., Aldunce, P., et al. (2022a). “Technical summary” in Climate change 2022: Impacts, adaptation and vulnerability. Contribution of working group II to the sixth assessment report of the intergovernmental panel on climate change. eds. H.-O. Pörtner, D. C. Roberts, and M. M. B. Tignor, et al. (Cambridge, United Kingdom and New York, NY, USA: Cambridge University Press).
Pörtner, H.-O., Roberts, D. C., MMB, T., Alegría, A., Craig, M., Langsdorf, S., et al. (2022b). “Summary for policymakers” in Climate change 2022: Impacts, adaptation and vulnerability. Contribution of working group II to the sixth assessment report of the intergovernmental panel on climate change (Cambridge, United Kingdom and New York, NY, USA: Cambridge University Press).
Prein, A. F., Ban, N., Ou, T., Tang, J., Sakaguchi, K., Collier, E., et al. (2023). Towards ensemble-based Kilometer-scale climate simulations over the third pole region. Clim. Dyn. 60, 4055–4081. doi: 10.1007/s00382-022-06543-3
Priya, P., Milind Mujumdar, T. P., Sabin, P. T., and Krishnan, R. (2015). Impacts of indo-Pacific Sea surface temperature anomalies on the summer monsoon circulation and heavy precipitation over Northwest India–Pakistan region during 2010. J. Clim. 28, no. 9, 3714–3730. doi: 10.1175/JCLI-D-14-00595.1
Rackow, T., Pedruzo-Bagazgoitia, X., Becker, T., Milinski, S., Sandu, I., Aguridan, R., et al. (2024). Multi-year simulations at kilometre scale with the integrated forecasting system coupled to FESOM2.5/NEMOv3.4. EGUsphere, 1–59. doi: 10.5194/egusphere-2024-913
Rajeev, A., and Mishra, V. (2022). On the causes of tropical cyclone driven floods in India. Weather Clim. Extremes 36:100432. doi: 10.1016/j.wace.2022.100432
Rajeev, A., and Mishra, V. (2023). Increasing risk of compound wind and precipitation extremes due to tropical cyclones in India. Environ. Res. Clim. 2:021004. doi: 10.1088/2752-5295/accf2e
Rasmussen, K. L., and Houze, R. A. (2012). A flash-flooding storm at the steep edge of high terrain: disaster in the Himalayas. Bull. Am. Meteorol. Soc. 93, 1714–1724. doi: 10.1175/BAMS-D-11-00236-1
Rathore, S., Goyal, R., Jangir, B., Ummenhofer, C. C., Feng, M., and Mishra, M. (2022). Interactions between a marine heatwave and tropical cyclone Amphan in the bay of Bengal in 2020. Front. Clim. 4:861477. doi: 10.3389/fclim.2022.861477
Ratna, S. B., Cherchi, A., Osborn, T. J., Joshi, M., and Uppara, U. (2021). The extreme positive Indian Ocean dipole of 2019 and associated Indian summer monsoon rainfall response. Geophys. Res. Lett. 48:9147. doi: 10.1029/2020GL091497
Ridder, N. N., Pitman, A. J., and Ukkola, A. M. (2021). Do CMIP6 climate models simulate global or regional compound events Skillfully? Geophys. Res. Lett. 48:e2020GL091152. doi: 10.1029/2020GL091152
Ridder, N. N., Ukkola, A. M., Pitman, A. J., and Perkins-Kirkpatrick, S. E. (2022). Increased occurrence of high impact compound events under climate change. NPJ Clim. Atmos. Sci. 5:3. doi: 10.1038/s41612-021-00224-4
Roca, R., Aublanc, J., Chambon, P., Fiolleau, T., and Viltard, N. (2014). Robust observational quantification of the contribution of mesoscale convective systems to rainfall in the tropics. J. Clim. 27, 4952–4958. doi: 10.1175/JCLI-D-13-00628.1
Roca, R., and Fiolleau, T. (2020). Extreme precipitation in the tropics is closely associated with long-lived convective systems. Commun. Earth Environ. 1:18. doi: 10.1038/s43247-020-00015-4
Rodrigues, R. R., and Shepherd, T. G. (2022). Small is beautiful: climate-change science as if people mattered. PNAS Nexus 1:pgac009. doi: 10.1093/pnasnexus/pgac009
Roemmich, D., Alford, M. H., Claustre, H., Johnson, K., King, B., Moum, J., et al. (2019). On the future of Argo: a global, full-depth, multi-disciplinary Array. Front. Mar. Sci. 6:439. doi: 10.3389/fmars.2019.00439
Roxy, M. K., Ghosh, S., Pathak, A., Athulya, R., Mujumdar, M., Murtugudde, R., et al. (2017). ). Threefold rise in widespread extreme rain events over Central India. Nat. Commun. 8:708. doi: 10.1038/s41467-017-00744-9
Roxy, M. K., Gnanaseelan, C., Parekh, A., Chowdary, J. S., Singh, S., Modi, A., et al. (2020). Indian Ocean warming.Assessment of climate change over the Indian region. Singapore: Springer Singapore.
Roxy, M. K., Saranya, J. S., Modi, A., Anusree, A., Cai, W., Resplandy, L., et al. (2024). “Chapter 20: future projections for the tropical Indian Ocean” in The Indian Ocean and its role in the global climate system. eds. C. C. Ummenhofer and R. R. Hood (Elsevier), 469–482.
Sabin, T. P., Krishnan, R., Ghattas, J., Denvil, S., Dufresne, J.-L., Hourdin, F., et al. (2013). High resolution simulation of the south Asian monsoon using a variable resolution global climate model. Clim. Dyn. 41, 173–94. doi: 10.1007/s00382-012-1658-8
Sabin, T. P., Krishnan, R., Vellore, R., Priya, P., Borgaonkar, H. P., Singh, B. B., et al. (2020). climate change over the Himalayas. Singapore: Springer Singapore.
Sahoo, B., and Bhaskaran, P. K. (2018). Multi-Hazard risk assessment of coastal vulnerability from tropical cyclones – a GIS based approach for the Odisha coast. J. Environ. Manag. 206, 1166–1178. doi: 10.1016/j.jenvman.2017.10.075
Salinger, M. J., Shrestha, M. L., Ailikun, W. D., McGregor, J. L., and Wang, S. (2014). “Climate in Asia and the Pacific: climate variability and change” in Climate in Asia and the Pacific: Security, society and sustainability. eds. M. Manton and L. A. Stevenson (Dordrecht: Springer Netherlands), 17–57.
Saranya, J. S., Roxy, M. K., Dasgupta, P., and Anand, A. (2022). Genesis and trends in marine heatwaves over the tropical Indian Ocean and their interaction with the Indian summer monsoon. J. Geophys. Res. Oceans 127:e2021JC017427. doi: 10.1029/2021JC017427
Satoh, M., Stevens, B., Judt, F., Khairoutdinov, M., Lin, S. J., Putman, W. M., et al. (2019). Global cloud-resolving models. Curr. Clim. Chang. Rep. 5, 172–184. doi: 10.1007/s40641-019-00131-0
Sattar, A., Goswami, A., and Kulkarni, A. V. (2019). Hydrodynamic moraine-breach Modeling and outburst flood routing - a Hazard assessment of the south Lhonak Lake, Sikkim. Sci. Total Environ. 668, 362–378. doi: 10.1016/j.scitotenv.2019.02.388
Schumacher, D. L., Hauser, M., and Seneviratne, S. I. (2022). Drivers and mechanisms of the 2021 Pacific northwest heatwave. Earth’s Future 10:e2022EF002967. doi: 10.1029/2022EF002967
Scott, C. A., Zhang, F., Mukherji, A., Immerzeel, W., Mustafa, D., and Bharati, L. (2019). “Water in the Hindu Kush Himalaya” in The Hindu Kush Himalaya assessment. eds. P. Wester, A. Mishra, A. Mukherji, and A. Shrestha (Cham: Springer).
Seager, R., Cane, M. A., Henderson, N., Lee, D., Abernathey, R., and Zhang, H. (2019). Strengthening tropical Pacific zonal sea surface temperature gradient consistent with rising greenhouse gases. Nat. Clim. Chang. 9, 517–522. doi: 10.1038/s41558-019-0505-x
Seneviratne, S. I., Zhang, X., Adnan, M., Badi, W., Dereczynski, C., Luca, A. D., et al. (2021). “Weather and climate extreme events in a changing climate” in Climate change 2021: The physical science basis. Contribution of working group I to the sixth assessment report of the intergovernmental panel on climate change. eds. V. Masson-Delmotte, P. Zhai, and A. Pirani, et al. (Cambridge, United Kingdom and New York, NY, USA: Cambridge University Press), 1513–1766.
Seo, Y. W., and Ha, K. J. (2022). Changes in land-atmosphere coupling increase compound drought and heatwaves over northern East Asia. NPJ Clim. Atmos. Sci. 5:100. doi: 10.1038/s41612-022-00325-8
Sharma, S., and Mujumdar, P. (2017). Increasing frequency and spatial extent of concurrent meteorological droughts and heatwaves in India. Sci. Rep. 7:15582. doi: 10.1038/s41598-017-15896-3
Sharma, E., Molden, D., Rahman, A., Khatiwada, Y. R., Zhang, L., Singh, S., et al. (2019). “Introduction to the Hindu Kush Himalaya assessment” in The Hindu Kush Himalaya assessment. eds. P. Wester, A. Mishra, A. Mukherji, and A. Shrestha (Cham: Springer).
Shaw, R., Luo, Y., Cheong, T. S., Halim, S. A., Chaturvedi, S., Hashizume, M., et al. (2022). “Asia” in Climate change 2022: Impacts, adaptation and vulnerability. Contribution of working group II to the sixth assessment report of the intergovernmental panel on climate change. eds. H.-O. Pörtner, D. C. Roberts, M. M. B. Tignor, E. S. Poloczanska, K. Mintenbeck, and A. Alegría, et al. (Cambridge, United Kingdom and New York, NY, USA: Cambridge University Press).
Shepherd, T. (2014). Atmospheric circulation as a source of uncertainty in climate change projections. Nat. Geosci. 7, 703–708. doi: 10.1038/ngeo2253
Shi, X., et al. (2015). Convolutional LSTM network: a machine learning approach for precipitation nowcasting. Adv. Neural Inf. Proces. Syst. 28, 1–9.
Shimpo, A., Takemura, K., Wakamatsu, S., Togawa, H., Mochizuki, Y., Takekawa, M., et al. (2019). Primary factors behind the heavy rain event of July 2018 and the subsequent heat wave in Japan. SOLA 15A, 13–18. doi: 10.2151/sola.15A-003
Shrestha, M. S., Grabs, W. E., and Khadgi, V. R. (2015). Establishment of a regional flood information system in the Hindu Kush Himalayas: challenges and opportunities. Int. J. Water Resour. D. 31, 238–252. doi: 10.1080/07900627.2015.1023891
Shrestha, M. S., Gurung, M. B., Khadgi, V. R., Wagle, N., Banarjee, S., Sherchan, U., et al. (2021). The last mile: flood risk communication for better preparedness in Nepal. Int. J. Disaster Risk Reduct. 56:102118. doi: 10.1016/j.ijdrr.2021.102118
Shrestha, F., Steiner, J. F., Shrestha, R., Dhungel, Y., Joshi, S. P., Inglis, S., et al. (2023). A comprehensive and version-controlled database of glacial lake outburst floods in High Mountain Asia. Earth Syst. Sci. Data 15, 3941–3961. doi: 10.5194/essd-15-3941-2023
Sillmann, J., Thorarinsdottir, T., Keenlyside, N., Schaller, N., Alexander, L. V., Hegerl, G., et al. (2017). Understanding, Modeling and predicting weather and climate extremes: challenges and opportunities. Weather Clim. Extremes 18, 65–74. doi: 10.1016/j.wace.2017.10.003
Simpson, N. P., Mach, K. J., Constable, A., Hess, J., Hogarth, R., Howden, M., et al. (2021). A framework for complex climate change risk assessment. One Earth 4, 489–501. doi: 10.1016/j.oneear.2021.03.005
Singh, V. K., Roxy, M. K., and Deshpande, M. (2021). Role of warm ocean conditions and the MJO in the genesis and intensification of extremely severe cyclone Fani. Sci. Rep. 11:3607. doi: 10.1038/s41598-021-82680-9
Smith, K. E., Burrows, M. T., Hobday, A. J., King, N. G., Moore, P. J., Sen Gupta, A., et al. (2023). Biological impacts of marine heatwaves. Annu. Rev. Mar. Sci. 15, 119–145. doi: 10.1146/annurev-marine-032122-121437
Song, Y., Yang, X., Bao, Y., Qiao, F., and Song, Z. (2024). Role of strong sea surface temperature diurnal variation in triggering the summer monsoon onset over the bay of Bengal in a climate model. Geophys. Res. Lett. 51:e2023GL108015. doi: 10.1029/2023GL108015
Sprintall, J., Nagura, M., Hermes, J., Roxy, M. K., McPhaden, M. J., Rao, E. P. R., et al. (2024). COVID impacts cause critical gaps in the Indian Ocean observing system. Bull. Am. Meteorol. Soc. 105, E725–E741. doi: 10.1175/BAMS-D-22-0270.1
Sreeraj, P., Swapna, P., Krishnan, R., Nidheesh, A. G., and Sandeep, N. (2022). Extreme Sea level rise along the Indian Ocean coastline: observations and 21st century projections. Environ. Res. Lett. 17:114016. doi: 10.1088/1748-9326/ac97f5
Srinivasu, U., Ravichandran, M., Han, W., Sivareddy, S., Rahman, H., Li, Y., et al. (2017). Causes for the reversal of North Indian Ocean decadal sea level trend in recent two decades. Clim. Dyn. 49, 3887–3904. doi: 10.1007/s00382-017-3551-y
Stalhandske, Z., Steinmann, C. B., Meiler, S., Sauer, I. J., Vogt, T., Bresch, D. N., et al. (2024). Global multi-Hazard risk assessment in a changing climate. Sci. Rep. 14:5875. doi: 10.1038/s41598-024-55775-2
Stammer, D., Amrhein, D. E., Balmaseda, M. A., Bertino, L., Bonavita, M., Buontempo, C., et al. (2024). Earth system reanalysis in support of climate model improvements. Bull. Am. Meteorol. Soc. 105, E1399–E1406. doi: 10.1175/BAMS-D-24-0110.1
Stevens, B., Adami, S., Ali, T., Anzt, H., Aslan, Z., Attinger, S., et al. (2024). Earth virtualization engines (EVE). Earth Syst. Sci. Data 16, 2113–2122. doi: 10.5194/essd-16-2113-2024
Sudha Rani, N. N. V., Satyanarayana, A. N. V., and Bhaskaran, P. K. (2015). Coastal vulnerability assessment studies over India: a review. Nat. Hazards 77, 405–428. doi: 10.1007/s11069-015-1597-x
Swapna, P., Jyoti, J., Krishnan, R., Sandeep, N., and Griffies, S. M. (2017). Multidecadal weakening of Indian summer monsoon circulation induces an increasing northern Indian Ocean Sea level. Geophys. Res. Lett. 44, 10560–10572. doi: 10.1002/2017GL074706
Swapna, P., Ravichandran, M., Nidheesh, G., Jyoti, J., Sandeep, N. S., Deepa, J. S., et al. (2020). “Sea-Level Rise” in Assessment of climate change over the Indian region eds. R. Krishnan, J. Gnanaseelan, S. C. Mujumdar, M. Kulkarni, and A. Supriyo Chakraborty. (Singapore: Springer Singapore).
Swapna, P., Sreeraj, P., Narayanasetti, S., Jyoti, J., Krishnan, R., Prajeesh, A. G., et al. (2022). Increasing frequency of extremely severe cyclonic storms in the North Indian Ocean by anthropogenic warming and southwest monsoon weakening. Geophys. Res. Lett. 49:e2021GL094650. doi: 10.1029/2021GL094650
Takahashi, H. G., Kamizawa, N., Nasuno, T., Yamada, Y., Kodama and, C., Sugimoto, S., et al. (2020). Response of the Asian summer monsoon precipitation to global warming in a high-resolution global nonhydrostatic model. J. Clim. 33, 8147–8164. doi: 10.1175/JCLI-D-19-0824.1
Takasuka, D., Kodama, C., Suematsu, T., Ohno, T., Yamada, Y., Seiki, T., et al. (2024). How can we improve the seamless representation of climatological statistics and weather toward reliable global K-scale climate simulations? J. Adv. Model. Earth Syst. 16:e2023MS003701. doi: 10.1029/2023MS003701
Takaya, Y., Ishikawa, I., Kobayashi, C., Endo, H., and Ose, T. (2020). Enhanced Meiyu-Baiu rainfall in early summer 2020: aftermath of the 2019 super IOD event. Geophys. Res. Lett. 47:e2020GL090671. doi: 10.1029/2020GL090671
Talley, L. D., Feely, R. A., Sloyan, B. M., Wanninkhof, R., Baringer, M. O., Bullister, J. L., et al. (2016). Changes in ocean heat, carbon content, and ventilation: a review of the first decade of GO-SHIP global repeat hydrography. Annu. Rev. Mar. Sci. 8, 185–215. doi: 10.1146/annurev-marine-052915-100829
Tang, S., Qiao, S., Wang, B., Liu, F., Feng, T., Yang, J., et al. (2023). Linkages of unprecedented 2022 Yangtze River valley heatwaves to Pakistan flood and triple-dip La Niña. NPJ Clim. Atmos. Sci. 6, 1–8. doi: 10.1038/s41612-023-00386-3
Tao, P., and Zhang, Y. (2019). Large-scale circulation features associated with the heat wave over Northeast China in summer 2018. Atmos. Ocean. Sci. Lett. 12, 254–260. doi: 10.1080/16742834.2019.1610326
Taylor, C., Robinson, T. R., Dunning, S., Carr, J. R., and Westoby, M. (2023). Glacial lake outburst floods threaten millions globally. Nat. Commun. 14:487. doi: 10.1038/s41467-023-36033-x
Tebaldi, C., and Debeire, K., and co-authors (2021). Climate model projections from the scenario model Intercomparison project (ScenarioMIP) of CMIP6, Earth Syst. Dynam., 12, 253–293, doi: 10.5194/esd-12-253-2021
Terao, T., Kanae, S., Fujinami, H., Someshwar Das, A. P., Dimri, S. D., Fujita, K., et al. (2023). AsiaPEX: challenges and prospects in Asian precipitation research. Bull. Am. Meteorol. Soc. 104, E884–E908. doi: 10.1175/BAMS-D-20-0220.1
Tripathy, K. P., and Mishra, A. K. (2023). How unusual is the 2022 European compound drought and heatwave event? Geophys. Res. Lett. 50:e2023GL105453. doi: 10.1029/2023GL105453
Trogrlić, Š., Robert, K. R., Ciurean, R. L., Gottardo, S., Torresan, S., Daloz, A. S., et al. (2024). Challenges in assessing and managing multi-Hazard risks: a European stakeholders perspective. Environ. Sci. Pol. 157:103774. doi: 10.1016/j.envsci.2024.103774
Trogrlić, Š., Robert, M., van den Homberg, M., Budimir, C. M. Q., Sneddon, A., and Golding, B. (2022). “Early warning systems and their role in disaster risk reduction” in Towards the “perfect” weather warning: Bridging disciplinary gaps through partnership and communication. ed. B. Golding (Cham: Springer International Publishing), 11–46.
Ullah, K., and Shouting, G. (2013). A diagnostic study of convective environment leading to heavy rainfall during the summer monsoon 2010 over Pakistan. Atmos. Res. 120–121, 226–239. doi: 10.1016/j.atmosres.2012.08.021
Ummenhofer, C. C., and Meehl, G. A. (2017). Extreme weather and climate events with ecological relevance: a review. Philos. Trans. R. Soc. B Biol. Sci. 372:20160135. doi: 10.1098/rstb.2016.0135
UNISDR (2015). “Sendai framework for disaster risk reduction 2015–2030.” United Nations Office for Disaster Risk Reduction (UNISDR). Available at: https://www.preventionweb.net/files/43291_sendaiframeworkfordrren.pdf (Accessed September 5, 2024).
van den Hurk, B. J. J. M., White, C. J., Ramos, A. M., Ward, P. J., Martius, O., Olbert, I., et al. (2023). Consideration of compound drivers and impacts in the disaster risk reduction cycle. iScience 26:6030. doi: 10.1016/j.isci.2023.106030
van Niekerk, A., Sandu, I., and Vosper, S. B. (2018). The circulation response to resolved versus parametrized orographic drag over complex mountain terrains. J. Adv. Model. Earth Syst. 10, 2527–2547. doi: 10.1029/2018MS001417
Vecchi, G. A., and Soden, B. J. (2007). Global warming and the weakening of the tropical circulation. J. Clim. 20, 4316–4340. doi: 10.1175/JCLI4258.1
Vousdoukas, M. I., Mentaschi, L., Voukouvalas, E., Verlaan, M., Jevrejeva, S., Jackson, L. P., et al. (2018). Global probabilistic projections of Extreme Sea levels show intensification of coastal flood Hazard. Nat. Commun. 9:2360. doi: 10.1038/s41467-018-04692-w
Wang, B., Biasutti, M., Byrne, M. P., Castro, C., Chang, C. P., Cook, K., et al. (2021). Monsoons climate change assessment. Bull. Am. Meteorol. Soc. 102, E1–E19. doi: 10.1175/BAMS-D-19-0335.1
Wang, J., Chen, Y., Tett, S. F., Yan, Z., Zhai, P., Feng, J., et al. (2020). Anthropogenically-driven increases in the risks of summertime compound hot extremes. Nat. Commun. 11:528. doi: 10.1038/s41467-019-14233-8
Wang, Y., Ruby Leung, L., McGREGOR, J. L., Lee, D.-K., Wang, W.-C., Ding, Y., et al. (2004). Regional climate Modeling: Progress, challenges, and prospects. J. Meteorol. Soc. Japan Ser. II 82, 1599–1628. doi: 10.2151/jmsj.82.1599
Wasko, C., and Nathan, R. (2019). Influence of changes in rainfall and soil moisture on trends in flooding. J. Hydrol. 575, 432–441. doi: 10.1016/j.jhydrol.2019.05.054
Williams, E., Funk, C., Peterson, P., and Tuholske, C. (2024). High resolution climate change observations and projections for the evaluation of heat-related extremes. Sci. Data 11:261. doi: 10.1038/s41597-024-03074-w
World Bank (2022). Pakistan: Flood damages and economic losses over USD 30 billion and reconstruction needs over USD 16 billion - New assessment. Washington, USA: World Bank.
World Climate Reserach Programme (WCRP) (2024). Kigali declaration: climate science for a sustainable future for all. 01/2024. WCRP Open Science Conference, 23–27 October 2023. Kigali, Rwanda. January 2024.
Wyns, A. (2022). Disaster diplomacy in the wake of the 2022 Pakistan floods. Lancet Planet. Health 6, e855–e856. doi: 10.1016/S2542-5196(22)00240-6
Yanai, M. H., and Li, C. (1994). Mechanism of heating and the boundary layer over the Tibetan plateau. Mon. Weather Rev. 122, 305–323. doi: 10.1175/1520-0493(1994)122<0305:MOHATB>2.0.CO;2
Yanai, M., Li, C., and Song, Z. (1992). Seasonal heating of the Tibetan plateau and its effects on the evolution of the Asian summer monsoon. J. Meteorol. Soc. Japan Ser. II 70, 319–351. doi: 10.2151/jmsj1965.70.1B_319
Yatagai, A., Kamiguchi, K., Arakawa, O., Hamada, A., Yasutomi, N., and Kitoh, A. (2012). APHRODITE: constructing a long-term daily gridded precipitation dataset for Asia based on a dense network of rain gauges. Bull. Am. Meteorol. Soc. 93, 1401–1415. doi: 10.1175/BAMS-D-11-00122.1
You, Y., and Ting, M. (2023). Improved performance of high-resolution climate models in simulating Asian monsoon rainfall extremes. Geophys. Res. Lett. 50:e2022GL100827. doi: 10.1029/2022GL100827
You, Y., Ting, M., and Biasutti, M. (2024). Climate warming contributes to the record-shattering 2022 Pakistan rainfall. NPJ Clim. Atmos. Sci. 7:89. doi: 10.1038/s41612-024-00630-4
Yu, R., and Zhai, P. (2020). More frequent and widespread persistent compound drought and heat event observed in China. Sci. Rep. 10:14576. doi: 10.1038/s41598-020-71312-3
Zhang, P., Jeong, J. H., Yoon, J. H., Kim, H., Wang, S. Y. S., Linderholm, H. W., et al. (2020). Abrupt shift to hotter and drier climate over inner East Asia beyond the tipping point. Science 370, 1095–1099. doi: 10.1126/science.abb3368
Zhang, Q., Li, R., Sun, J., Lu, F., Xu, J., and Zhang, F. (2023). A review of research on the record-breaking precipitation event in Henan Province, China, July 2021. Adv. Atmos. Sci. 40, 1485–1500. doi: 10.1007/s00376-023-2360-y
Zhang, T., Wang, W., An, B., and Wei, L. (2023). Enhanced glacial activity threatens numerous communities and infrastructure in the third pole. Nat. Commun. 14:8250. doi: 10.1038/s41467-023-44123-z
Zhang, P., Wang, B., Wu, Z., Jin, R., and Cao, C. (2024). Intensified gradient La Nina and extratropical thermal patterns drive the 2022 east and south Asian “seesaw” extremes. Npj climate and atmospheric. Science 7:47. doi: 10.1038/s41612-024-00597-2
Zhang, L., and Zhou, T. (2015). Drought over East Asia: a review. J. Clim. 28, 3375–3399. doi: 10.1175/JCLI-D-14-00259.1
Zhao, B., Qiao, F., Cavaleri, L., Wang, G., Bertotti, L., and Liu, L. (2017). Sensitivity of typhoon modeling to surface waves and rainfall. J. Geophys. Res. Oceans 122:2262. doi: 10.1002/2016JC012262
Zhao, B., Wang, G., Zhang, J. A., Liu, L., Liu, J., Xu, J., et al. (2022). The effects of ocean surface waves on tropical cyclone intensity: numerical simulations using a regional atmosphere-ocean-wave coupled model. J. Geophys. Res. Oceans 127:e2022JC019015. doi: 10.1029/2022JC019015
Zhong, Y., Allen, S. K., Zheng, G., Liu, Q., and Stoffel, M. (2024). Large rock and ice avalanches frequently produce cascading processes in High Mountain Asia. Geomorphology 449:109048. doi: 10.1016/j.geomorph.2023.109048
Zhu, D., Song, K., Jingchao, M., Huang, H., Hongjian, D., Xiazhuang, F., et al. (2021). Effect of climate change induced extreme precipitation on landslide activity in the three gorges reservoir, China. Bull. Eng. Geol. Environ. 80, 781–794. doi: 10.1007/s10064-020-01948-y
Zscheischler, J., Martius, O., Westra, S., Bevacqua, E., Raymond, C., Horton, R. M., et al. (2020). A typology of compound weather and climate events. Nat. Rev. Earth Environ. 1, 333–347. doi: 10.1038/s43017-020-0060-z
Keywords: compound events, risk, Asia, early warning systems, knowledge gaps, compound flooding, compound heatwave-drought, compound marine extremes
Citation: Krishnan R, Dhara C, Horinouchi T, Gotangco Gonzales CK, Dimri AP, Shrestha MS, Swapna P, Roxy MK, Son S-W, Ayantika DC, Cruz FAT and Qiao F (2025) Compound weather and climate extremes in the Asian region: science-informed recommendations for policy. Front. Clim. 6:1504475. doi: 10.3389/fclim.2024.1504475
Edited by:
Matthew Collins, University of Exeter, United KingdomReviewed by:
Geli Wang, Chinese Academy of Sciences (CAS), ChinaGoran Trbic, University of Banjaluka, Bosnia and Herzegovina
Lili Li, West Yunnan University of Applied Sciences, China
Copyright © 2025 Krishnan, Dhara, Horinouchi, Gotangco Gonzales, Dimri, Shrestha, Swapna, Roxy, Son, Ayantika, Cruz and Qiao. This is an open-access article distributed under the terms of the Creative Commons Attribution License (CC BY). The use, distribution or reproduction in other forums is permitted, provided the original author(s) and the copyright owner(s) are credited and that the original publication in this journal is cited, in accordance with accepted academic practice. No use, distribution or reproduction is permitted which does not comply with these terms.
*Correspondence: Chirag Dhara, Y2hpcmFnLmRoYXJhQGtyZWEuZWR1Lmlu