- 1Oden Institute for Computational Engineering and Sciences, University of Texas at Austin, Austin, TX, United States
- 2Scripps Institution of Oceanography, University of San Diego, San Diego, CA, United States
- 3Department of Applied Mathematics and Theoretical Physics, University of Cambridge, Cambridge, United Kingdom
- 4Department of Earth, Atmospheric, and Planetary Sciences, Massachusetts Institute of Technology, Cambridge, MA, United States
- 5South Atlantic Environmental Research Institute, Stanley, Falkland Islands
- 6Jackson School of Geosciences, University of Texas at Austin, Austin, TX, United States
- 7Your Ocean Consulting, LLC, Knoxville, TN, United States
- 8Department of Oceanography, University of Hawaii at Manoa, Honolulu, HI, United States
- 9FRB-CESAB, Fondation pour la Recherche sur la Biodiversité – Centre de Synthèse et d'Analyse sur la Biodiversité, Montpellier, France
- 10Posgrado en Ciencias Biológicas, Universidad Nacional Autónoma de México, Mexico City, Mexico
- 11Pinngortitaleriffik Greenland Institute of Natural Resources, Greenland Climate Research Centre, Nuuk, Greenland
- 12Institute for Marine and Antarctic Studies, Centre for Marine Socioecology, University of Tasmania, Hobart, TAS, Australia
- 13Department of Earth System Science, University of California, Irvine, Irvine, CA, United States
- 14Instituto de Ciencias del Mar y Limnología, Universidad Nacional Autonoma de Mexico, Mexico City, Mexico
- 15Department of Nutrition, T.H. Chan School of Public Health, Harvard University, Boston, MA, United States
- 16Department of Earth, Environmental, and Planetary Sciences and Division of Applied Mathematics, Brown University, Providence, RI, United States
- 17Department of Biological Sciences, University of Bergen and Bjerknes Centre for Climate Research, Bergen, Norway
- 18Gloucester Marine Genomics Institute, Gloucester, MA, United States
- 19National Oceanography Centre, Southampton, United Kingdom
- 20Independent Researcher, London, United Kingdom
- 21School of Marine Science and Policy, University of Delaware, Lewes, DE, United States
- 22Minderoo-UWA Deep-Sea Research Centre, School of Biological Sciences and UWA Oceans Institute, University of Western Australia, Perth, WA, Australia
- 23Abdelmalek Essaadi University, Tétouan, Morocco
- 24Oxford University Museum of Natural History, University of Oxford, Oxford, United Kingdom
- 25Nekton Foundation, Oxford, United Kingdom
- 26The Biodiversity Consultancy, Cambridge, United Kingdom
- 27CEREGE, Aix Marseille University, CNRS, IRD, INRAE, College de France, Aix-en-Provence, France
- 28Earth System Science Interdisciplinary Center, University of Maryland, College Park, MD, United States
Introduction: A defining aspect of the Intergovernmental Panel on Climate Change (IPCC) assessment reports (AR) is a formal uncertainty language framework that emphasizes higher certainty issues across the reports, especially in the executive summaries and short summaries for policymakers. As a result, potentially significant risks involving understudied components of the climate system are shielded from view.
Methods: Here we seek to address this in the latest, sixth assessment report (AR6) for one such component—the deep ocean—by summarizing major uncertainties (based on discussions of low confidence issues or gaps) regarding its role in our changing climate system. The goal is to identify key research priorities to improve IPCC confidence levels in deep ocean systems and facilitate the dissemination of IPCC results regarding potentially high impact deep ocean processes to decision-makers. This will accelerate improvement of global climate projections and aid in informing efforts to mitigate climate change impacts. An analysis of 3,000 pages across the six selected AR6 reports revealed 219 major science gaps related to the deep ocean. These were categorized by climate stressor and nature of impacts.
Results: Half of these are biological science gaps, primarily surrounding our understanding of changes in ocean ecosystems, fisheries, and primary productivity. The remaining science gaps are related to uncertainties in the physical (32%) and biogeochemical (15%) ocean states and processes. Model deficiencies are the leading cited cause of low certainty in the physical ocean and ice states, whereas causes of biological uncertainties are most often attributed to limited studies and observations or conflicting results.
Discussion: Key areas for coordinated effort within the deep ocean observing and modeling community have emerged, which will improve confidence in the deep ocean state and its ongoing changes for the next assessment report. This list of key “known unknowns” includes meridional overturning circulation, ocean deoxygenation and acidification, primary production, food supply and the ocean carbon cycle, climate change impacts on ocean ecosystems and fisheries, and ocean-based climate interventions. From these findings, we offer recommendations for AR7 to avoid omitting low confidence-high risk changes in the climate system.
1 Importance of the deep sea
The deep ocean is defined within the Intergovernmental Panel on Climate Change (IPCC) Special Report on Ocean and Cryosphere in a Changing Climate (SROCC, IPCC, 2019) as the region below 200 m depth, marking both the end of the shallow shelf seas and the depth at which photosynthesis takes place (Bindoff et al., 2019). Below this depth critical ecological changes and biogeochemical transformations occur (Bindoff et al., 2019). The deep ocean plays a pivotal role in our climate system owing to its vast reservoir capacities and residence times, hosting the majority of total ocean carbon (~37 k Gt, Friedlingstein et al., 2023), which in turn exceeds the quantity of carbon contained in the atmosphere and connected upper ocean reservoir (by factor ∼50) and in plants and soils (by factor ∼20). To date the ocean has taken up ∼30% of anthropogenic CO2 (Sabine et al., 2004; Khatiwala et al., 2009, 2013; Gruber et al., 2019a; Crisp et al., 2022; Friedlingstein et al., 2023). The ocean has also served as the dominant reservoir for heat produced by the earth’s energy imbalance resulting from anthropogenic changes in atmospheric composition. Since 1971, observations indicate that 90% of this heat has been absorbed by the ocean (with approximately half of this anthropogenic heat residing below 700 m depth; von Schuckmann et al., 2023), significantly slowing transient global warming on land (Drake et al., 2021). The deep ocean accounts for 95% of Earth’s habitable space and supports a plethora of unique ecosystems (Ramirez-Llodra et al., 2010) including those likely to have hosted the development of life on the planet (Baross and Hoffman, 1985; Martin et al., 2008). It also serves as a vast repository for biodiversity (Snelgrove and Smith, 2002) and genetic resources (Harden-Davies, 2017) and could provide important nutritional assets for tackling increasing threats to global food security (Campanya-Llovet et al., 2017; Gatto et al., 2023). The total economic value of these resources and other ecosystem processes and services provided by the global deep sea (Folkersen et al., 2018; Turner et al., 2019) has been estimated to amount to 267 billion US dollars per year (Ottaviani, 2020). This estimate does not account for the immense cultural and social significance of the deep sea for people all around the world, which is beyond quantification.
Despite this tremendous importance, the deep sea remains largely unexplored owing to logistical and technological challenges and high costs. Approximately 80% of the seafloor has not been mapped at resolution higher than 1 km (Mayer et al., 2018) and it is predicted that millions of deep ocean species remain to be discovered (Mora et al., 2011). Huge uncertainty surrounds our understanding of fundamental physical, chemical, and biological aspects of the deep ocean, including its baseline state and variability, characteristic processes, sensitivities, and vulnerabilities. There is, however, mounting evidence of significant and concerning changes underway, including a weakening of the ocean carbon sink (Muller et al., 2023), widespread deep ocean deoxygenation (Stramma et al., 2010; Oschlies et al., 2018) and acidification (Lauvset et al., 2020; Ma et al., 2023), reduction of food supply to depths (Smith et al., 2006) and resulting degradation of deep ocean ecosystems (Ruhl and Smith, 2004; Smith et al., 2009, 2013; Sweetman et al., 2018). As extra stresses arrive with expanding oil and gas extraction, trawling, as well as potential deep-seabed mining and ocean-based climate interventions in the future—all with highly uncertain impacts and interactions (Mengerink et al., 2014; Sweetman et al., 2018; Levin et al., 2023)—there is an urgent need to identify and close scientific gaps related to deep ocean climate change and inform policy that can ensure effective management and mitigate serious harm (Amon et al., 2022a). This study seeks to synthesize these science gaps as highlighted across the latest assessment reports of the IPCC. The reports are chosen as the basis for our synthesis since they represent the most extensive survey of peer reviewed literature (and other quality-assured sources) from the global research community, returning the most comprehensive assessment of present and future climate change, its causes, potential impacts and response options.
2 The IPCC AR6 and its uncertainty characterization
The Sixth Assessment Report (AR6) was produced during the latest IPCC assessment cycle (2015–2023). It comprises three Working Group (WG) contributions, a Methodology Report, three Special Reports, and a Synthesis. The WG Reports provide a holistic assessment of scientific, technical and socioeconomic aspects of climate change: Working Group I (WGI, IPCC, 2021a) assesses the physical science basis, Working Group II (WGII IPCC, 2022a) assesses climate change impacts and adaptations/vulnerabilities of the natural world and human societies, and Working Group III (WGIII, IPCC, 2022b) assesses mitigation options. The three cross-WG Special Reports provide more focused assessments of global warming of 1.5°C (SR15, IPCC, 2018), the ocean and cryosphere in a changing climate (SROCC, IPCC, 2019) and climate change and land (SRCCL, IPCC, 2021c). The Methodology Report provides updated guidelines for greenhouse gas inventories, and the Synthesis Report (SYR, IPCC, 2021b) integrates findings across all reports in the cycle.
The IPCC reports are informed by thousands of peer-reviewed publications (and other quality-assured sources) and assessed by expert author teams exceeding 200 members per WG. As a result, they provide the ultimate scientific basis to inform policy and sustainable development goals. To facilitate this, each chapter body—typically comprising a few hundred pages—is condensed into a 3 page Executive Summary (ES), and each report—typically comprising a few thousand pages—is condensed into a 30 page high-level Summary for Policy Makers (SPM). The SPMs are unique in being subject to line-by-line review until agreement is reached by all participating governments.
A critical component of IPCC Assessment Reports is a formal uncertainty language framework, typeset in italics and aimed at ensuring coherent characterization and communication of uncertainty across various working groups, reports and chapters. This framework was first introduced during the production of the third assessment report (Moss and Schneider, 2000) and was most recently revised by Mastrandrea et al. (2010) for the fifth assessment report (AR5). This latest framework, also employed for AR6, provides three scales to communicate the degree of certainty in findings: (1) evidence/agreement, (2) confidence, and (3) likelihood, with relations between the scales described in the Supplementary material. Their adoption has been assessed for the ESs and SPMs in AR4 and AR5 (Mach et al., 2017), and for the AR6 Special Reports (Janzwood, 2020). These studies find that the latest author guidelines have improved balanced judgments of certainty across disciplines, with a particularly notable increase in the use of confidence terms (Mach et al., 2017; Janzwood, 2020), the scale accounting for the majority of uncertainty language use across almost all chapters. Furthermore, the proportion of very low and low confidence statements has increased significantly since AR4, following explicit guidelines for authors to communicate a wide range of possible outcomes, not only high certainty findings (Mastrandrea et al., 2010). This calibrated language aids identification of key knowns and major science gaps. While the former can be communicated easily to policymakers, identifying science gaps is essential for recognizing current limits on evidence-based decision making. Our study focuses on collating deep ocean science gaps associated with very low and low confidence statements across the AR6 to highlight critical research needs.
3 Underrepresentation of uncertain issues in policy considerations
Despite the improvements in communicating the assessment conclusions cited above, many challenges persist. The reports have been shown to focus on what is known with medium to very high confidence and deemphasize uncertain findings, with this skewness further exacerbated in the ESs and SPMs. Mach et al. (2017) show that for AR5 ∼ 65% of confidence designations are very high or high confidence, ∼30% are medium confidence, and only ∼5% are low or very low confidence across all ESs and SPMs. Janzwood (2020) identifies a similar proportional language use in the SROCC ESs and SPM and demonstrates the drop off in very low and low confidence statements from the chapter bodies to the more widely-read summaries. Expanding upon Janzwood (2020) evaluation, we show that a similar reporting skew is evident in all of the AR6 literature that we reviewed for our meta-analysis (Figure 1), including the three WG reports. This skew has severe implications for understudied components of the climate system such as the deep ocean, which is beset by uncertainty due to challenges outlined above. The result is that—despite its immense importance for global climate, ecology, and society—the deep sea is chronically underrepresented in policy considerations and critical research needs are overlooked (Levin, 2021). This highlights current limits on evidence-based decision making and motivates our study with the objectives outlined below.
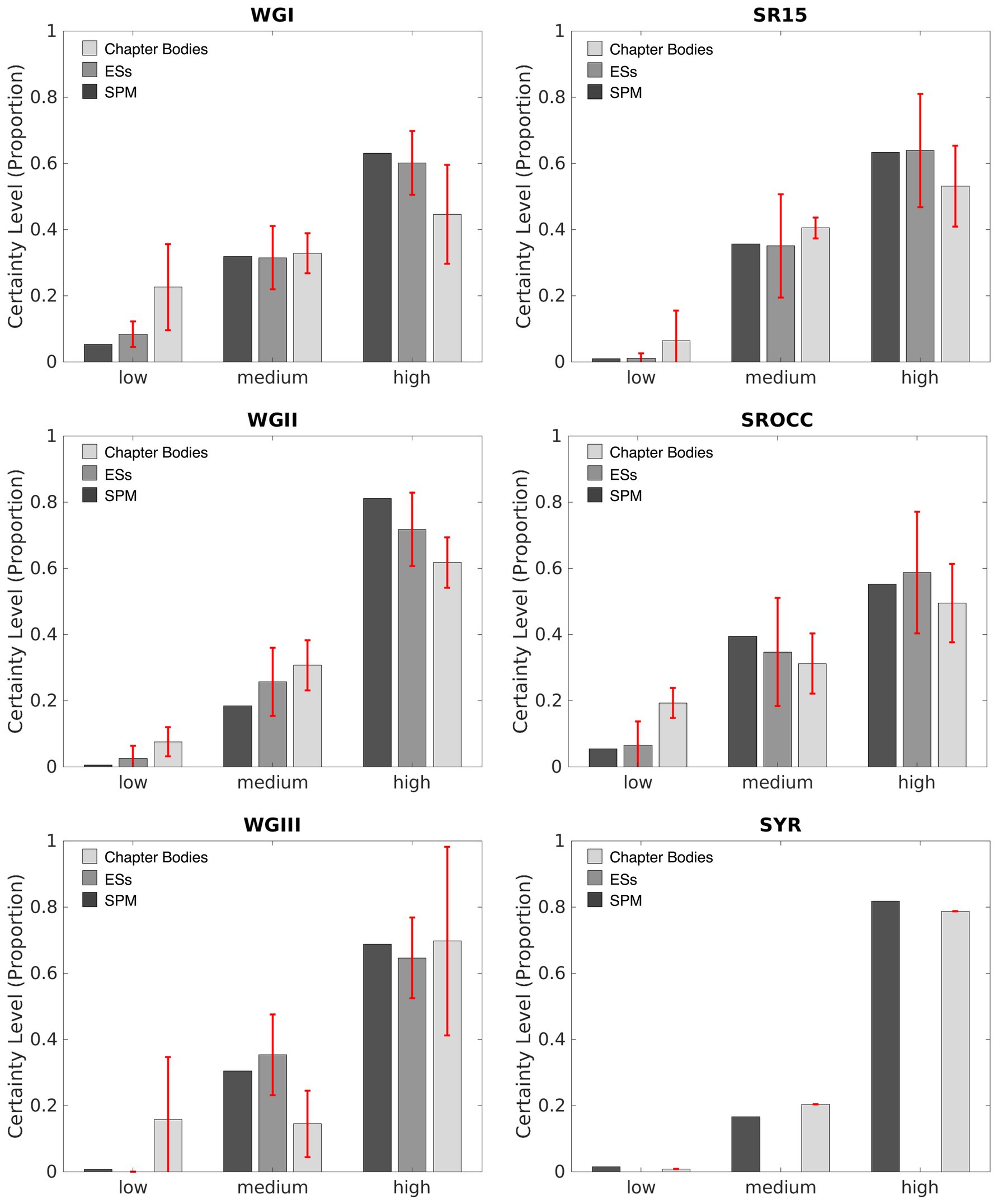
Figure 1. Summary of proportional use of certainty levels within the chapter bodies, executive summaries (ESs) and summary for policy makers (SPM) for each of the six AR6 reports reviewed in our meta-analysis. Following Mach et al. (2017) “certainty” terminology is used to group confidence levels: low certainty combines very low and low confidence, medium certainty combines medium-low and medium confidence, and high certainty combines high and very high confidence. Bars plotted for the chapter bodies and ESs are averages of those shown in Supplementary Figure S1, with the standard deviation indicated in red. Note there is no ES for the Synthesis Report (SYR). High certainty is emphasized across all sections of every report. The proportion of high and medium certainty increases from the chapter bodies to the ESs and SPMs and there is an accompanying particularly severe drop off in low certainty statements in the ESs and SPMs. The total counts for low, medium, and high certainty for each chapter were obtained using a combination of text-based searches, for text within each chapter body, and optical character recognition (OCR) software to isolate language within figures and image-based tables. References and leading introductory text (i.e., introducing the calibrated language framework) were omitted.
4 Study objectives
In this study, we seek to extract major science gaps related to the deep ocean across the IPCC AR6 literature. Our principal aims are to:
i. Identify key research priorities to improve IPCC confidence levels in deep ocean systems, and
ii. encourage better representation and increased visibility of potentially high impact yet uncertain deep ocean processes in the communication of IPCC results to policymakers.
Addressing these aims will provide valuable guidance for expanding the global ocean observing system, ensure accelerated improvement of climate projections, and support well-informed climate change mitigation efforts. An important product accompanying this paper is a table of deep ocean science gaps collated from the IPCC AR6 reports (Supplementary Table 1). Our intention is that this resource can be used by researchers and observing programs to determine where they may best contribute their expertise and resources. The remainder of the paper is organized as follows. In section 5 we describe our approach for assessing major deep ocean science gaps across the AR6 reports. We present the results of our meta-analysis in section 6. Our results include an assessment of deep ocean recognition in the SPMs, a comprehensive list of major deep ocean related science gaps across the AR6 (provided as SI), quantification of the major reasons for low confidence in these areas, and identification of the main physical/biogeochemical/biological variables impacting—or being impacted by—low confidence in other fields. Our results also include a deeper discussion of seven areas of major uncertainty identified by our meta-analysis: the overturning circulation, the ocean carbon cycle, primary production and food supply, ocean deoxygenation and acidification, climate change impacts on ocean ecosystems and fisheries, and ocean based climate interventions. Recommendations for future research and reporting are given in sections 7 and 8. The limitations of our study are discussed in section 9 and a summary is given in section 10.
5 Methods
The IPCC formal language framework offers an objective approach to identify major deep ocean related gaps in understanding across scientific, technical, and socioeconomic spheres. As discussed above, the latest guidelines have increased the use of the three uncertainty language scales (section 2), although some confusion remains around their relation and appropriate use (Janzwood, 2020). We start by briefly discussing these issues, justifying our decision to focus on identifying science gaps expressed using very low and low confidence statements in the IPCC literature. We then outline our data collection method, which involves a group (25 of the authors of this manuscript) of scientists with deep ocean expertise performing a meta-analysis of 6 selected IPCC reports to collate and categorize these statements, along with those presented in dedicated knowledge gaps sections.
5.1 A focus on AR6 “confidence” statements
IPCC author guidelines for calibrated language assignments are summarized in the SI with recent critiques of the framework presented by Mach et al. (2017) and Janzwood (2020). We chose to identify major science gaps across the AR6 literature by focusing on the use of the confidence language scale, collating statements of very low confidence and low confidence related to the deep ocean. Following Mach et al. (2017) we will refer to these two categories together as “low certainty” below. The guidelines encourage that these terms “should be reserved for areas of major concern and the reason for their presentation should be carefully explained” (Mastrandrea et al., 2010), so our focus allows us to elevate major deep ocean science gaps that have been deemed to be of major concern by the IPCC expert authors. In doing so, we also avoid ambiguities in relating across language scales (see Supplementary material).
5.2 Data collection method
Discussion of the ocean is presented in six key documents published between 2021 and 2023: WGI, WGII, WGIII, SR15, SROCC, and SYR. All low certainty issues related to activity on land within these reports were omitted in this study and the SRCCL was not included in our meta-analysis because of its focus on land-based processes. Although terrestrial systems may impact the deep ocean, especially over long timescales, we focused on science gaps where this connection is direct, allowing us to achieve our objectives. After compiling a list of ocean-relevant chapters across these six reports, study participants were sought from members of the DOOS (Deep Ocean Observing Strategy, Smith et al., 2023) and DOSI (Deep Ocean Stewardship, DOSI, 2018) communities. Participants were requested to review at least 2 chapters as either the “primary” or “secondary” assessor. Participants were only allowed to serve as primary assessors for topics in which they had reasonable knowledge and expertise. This requirement was relaxed for secondary assessors, who were tasked with verifying that information was correctly reported by the primary assessor. To perform a comprehensive gaps assessment, primary assessors were instructed to compile low certainty issues for which deep ocean relevance was both explicit and implicit within each of their chapter(s). For example, many low certainty issues reported occur in the near-surface ocean but propagate via physical/biogeochemical/biological pathways to generate significant uncertainty below 200 m depth. A key element of our meta-analysis was to characterize both the cause (“stressor”) and ramification (“impact”) of each low certainty statement and categorize the reason for low agreement and/or limited evidence (e.g., model disagreement, lack of observations) where possible. For example, the relevance of the deep ocean may be as the stressor of uncertainty (e.g., deep ocean warming generating low confidence in ice sheet and sea level projections) or the impact (e.g., low confidence in Antarctic Bottom Water trends owing to the uncertain role of sea ice variations). The following information was requested for each deep ocean relevant low certainty statement:
• section number and title,
• summary of the low certainty statement,
• topic of the underlying cause (“stressor”) and ramification (“impact”) of low certainty (Table 1)
• reason for low certainty,
• assessor comments, including extra description or discussion of deep ocean relevance where needed.
To obtain meaningful quantitative information, primary assessors were instructed to report multiple low certainty statements discussing a single issue in the same section only once. Statements made in AR6 referencing results from earlier assessment report cycles were omitted.
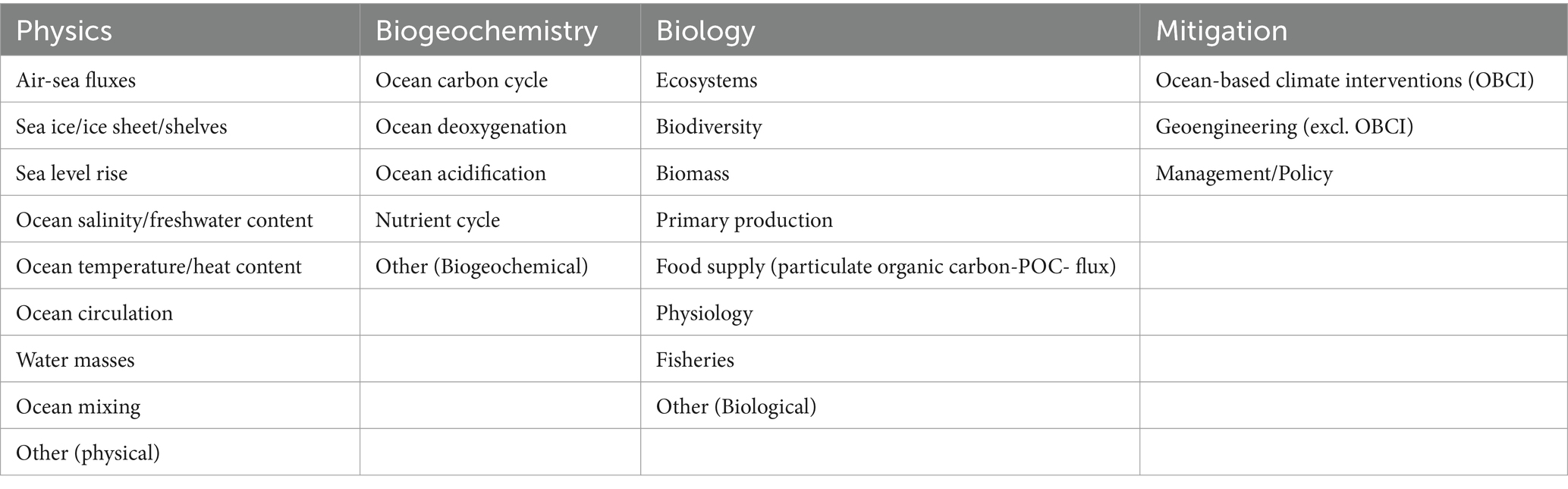
Table 1. Specific subjects used to categorize the topic of the deep ocean relevant low certainty (very low confidence and low confidence) statements in the AR6 reports, organized by discipline. Where possible, subjects were specified for both the driver of low confidence in the deep ocean (i.e., the “stressor”) and the field/process characterized by low certainty as a result (i.e., the “impact”). As an example, consider the following statement from chapter 3 of SR15: “Some ecosystems, such as those from bathyal areas (i.e., 200–3,000 m below the surface), are likely to undergo very large reductions in pH by the year 2100...yet evidence of how deep-water ecosystems will respond is currently limited despite the potential planetary importance of these areas (low to medium confidence)”. In this case the stressor (ocean acidification) and impact (ecosystems) can easily be identified. For a large proportion of science gaps, this is not the case. For the following example from chapter 3 of WGI the impact can be identified (ocean circulation) but there is no explicit stressor: “estimates of AMOC since at least 1950...suggest the AMOC weakened through the 20th century (low confidence)”.
A potential problem in focusing a science gaps assessment only on low certainty statements is that some threshold level of evidence is required for even this designation (see Supplementary material). As a result, IPCC expert authors do not have the opportunity to discuss grossly under-constrained issues with this language. Recognizing that these issues may have major potential importance for climate, dedicated sections on knowledge gaps are included at the end of many chapters. Assessors were also instructed to report all relevant gaps from these sections. For the remainder of the paper, we will refer to all collated issues collectively as “science gaps.” Finally, for the 6 reports surveyed, we collated all discussion of the deep ocean from the SPMs (including text without accompanying expressions of confidence). Comparing this information to all identified science gaps illustrates which deep ocean changes and processes are not shaping policy, media reporting, and public knowledge and perception of climate change.
6 Results: IPCC AR6 deep ocean science gaps
In total, we reviewed approximately 3,000 pages across the six selected AR6 reports and identified 219 major science gaps related to the deep ocean. All 219 gaps are listed in Supplementary Table 1, where the issues collated from the dedicated knowledge gaps sections—representing those associated with major structural deficiencies in climate science research—are highlighted in pink. In this section, we first discuss recognition of the deep ocean in the SPMs, before assessing the principal stressors and impacts of deep ocean uncertainty across the reports. We then discuss the main underlying causes and highlight areas where research needs are great and collaboration should be increased.
6.1 Deep ocean recognition in the summaries for policy makers
Discussion of the deep ocean in the SPMs (Table 2) is almost entirely limited to general qualitative statements of high and very high confidence changes in globally averaged or integrated quantities. Almost all SPMs (WGI, WGII, SROCC and SYR) recognize that the global ocean has absorbed a significant fraction of anthropogenic heat and CO2 and has, as a result, undergone large scale warming, acidification, and deoxygenation. These changes, accompanied by altered ocean circulation, reduced vertical mixing and fluxes of organic carbon (SROCC SPM), are recognized to be irreversible and accompanied by substantial damages to deep ocean marine ecosystems, adversely affecting biodiversity, food production, and ecosystem services (WGII, SROCC, and SYR SPMs). It is recognized that thermal expansion of the warming ocean explains 50% of sea level rise over the last 50 years (although recent estimates indicate this contribution has decreased to 33% since 2005 with accelerating contributions from land ice, Hamlington et al., 2020 and references therein) and that sea level rise will continue on centennial to millennial timescales as a response to deep ocean warming (WGI, SROCC and SYR SPMs). Although we are already committed to many physical and biogeochemical changes over the early 21st century (WGI and SROCC SPMs), it is recognized that limiting global warming to 1.5°C would reduce detrimental impacts on ocean ecosystems and their services (SR15 SPM), with the opportunity to further reduce vulnerability to ongoing climate change with targeted management of deep-ocean areas (WGII and SYR SPMs). In contrast, marine carbon dioxide removal (mCDR) strategies, such as ocean fertilization, may exacerbate existing biogeochemical changes and further perturb deep ocean ecosystems (WGIII SPM). Any discussion of specific deep-ocean regions, ecosystems, or species within the SPMs is limited to the SROCC, which highlights harmful effects of physical and biogeochemical changes on cold-water coral and abyssal plain ecosystems and includes the only issue communicated with low confidence. The omission of other deep-sea ecosystems (e.g., seamounts, hydrothermal vents, methane seeps, sponge reefs, canyons, pelagic zones including oxygen minimum zones, trenches) is caused by large uncertainties associated with specific region/ecosystem/species change.
6.2 Stressors and impacts of deep ocean uncertainty
Stressors and impacts of major deep ocean uncertainty are summarized in Figure 2, with their connections shown in Figure 3. Common connections (i.e., largest values in the Figure 3 heat map) are generally interpreted as specific interactions in great need of investigation to improve confidence in the next IPCC assessment cycle. For 45% of the identified science gaps, the main stressor is unspecified (Figure 2A), meaning that the specifics of the research problem requiring attention are less clear. For the remaining 55% of the identified science gaps, only 5% are explicitly linked to uncertainties in mitigation efforts that will be undertaken, mainly those involving ocean-based climate interventions (OBCI) (Figure 2A). This low proportion is a reflection of the relative paucity of mCDR research (compared to other themes listed) to date. It does not serve as a true indicator of the level of research need in this area, which is significantly elevated by the increasing appetite to consider these measures in the policy community (section 6.4.7). Physical, biogeochemical and biological variables are the stressors underpinning approximately 35%, 12%, and 2% respectively, of the remaining gaps (Figure 2A). These proportions for identified links reflect causal connections in the climate system, allowing the propagation of uncertainty from physical to biogeochemical and biological states (Figure 3). Uncertainties in ocean temperature/heat content are the dominant physical stressor, underpinning numerous science gaps related to deep ocean ecosystems and fisheries (Figure 3). Air-sea fluxes (mainly of momentum, Supplementary Table 1), ocean circulation (mainly associated with overturning and upwelling, Supplementary Table 1), and sea ice/ice shelf/ice sheet processes are also important stressors (Figure 3). Uncertainties in ocean acidification account for approximately half of the biogeochemical stressors, with negative consequences for projections of deep ocean ecosystems and the physiological responses of deep ocean species to climate change (Figure 3).
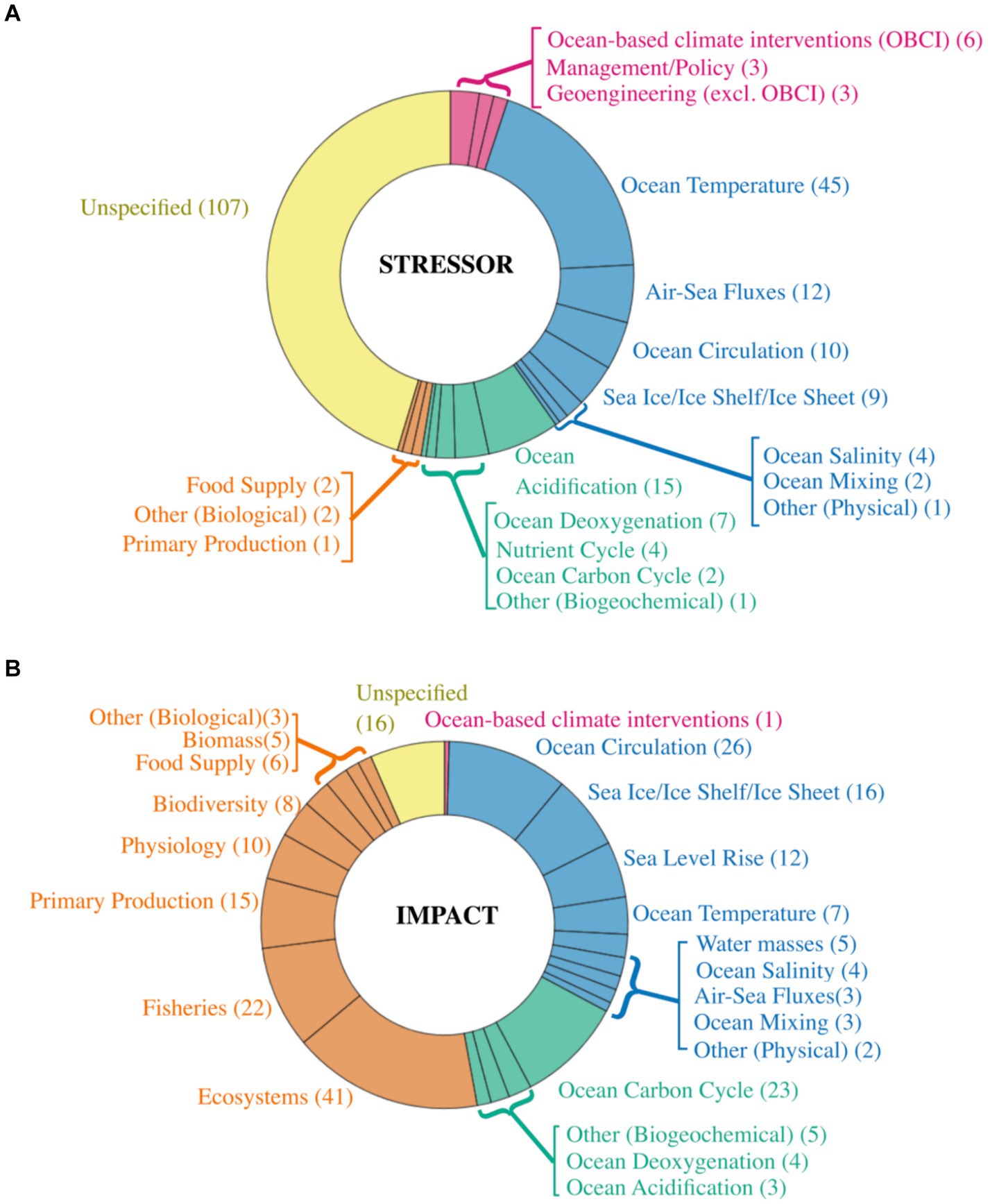
Figure 2. Category of (A) underlying cause (“stressor”) and (B) ramification (“impact”) of low certainty associated with the 219 deep ocean science gaps identified in the IPCC AR6 meta-analysis. In both cases total counts exceed 219 since each gap may be listed with more than one stressor or impact. Colors group mitigation efforts (pink), physical (blue), biogeochemical (green), and biological (orange) topics. “Unspecified” stressors and impacts are shown in yellow and may be discernible from a broader literature review but are not explicitly referenced in the AR6 reports surveyed.
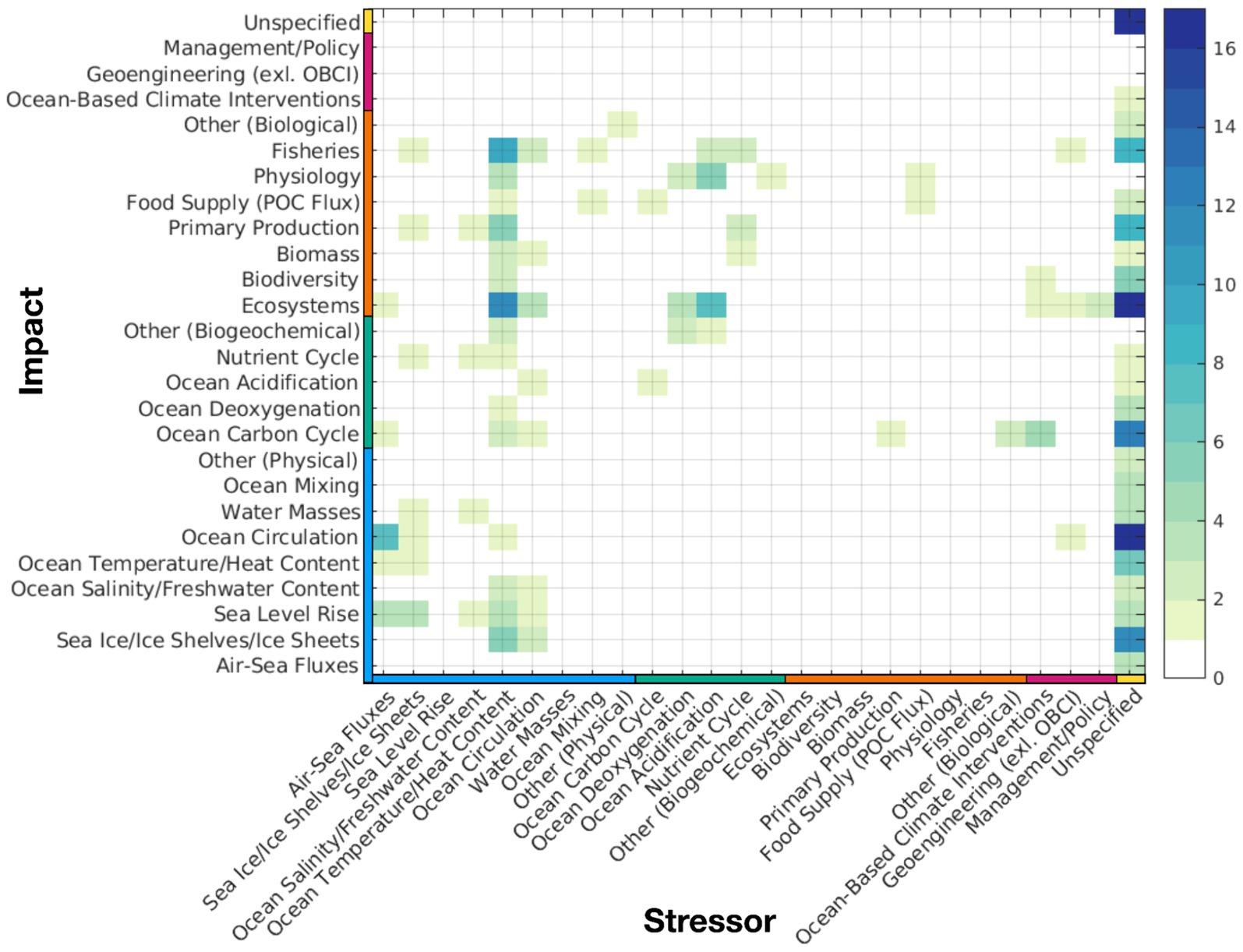
Figure 3. Heat map showing association of stressors (i.e., underlying cause) and impacts of low certainty for the deep ocean science gaps identified in our IPCC AR6 meta-analysis. Colors in the heat map indicate the number of times an association between stressor and impact was identified. Colors along the axes are used consistently from Figure 2 to delineate physical (blue), biogeochemical (green), and biological (orange) stressors/impacts, and those related to climate mitigation efforts (pink). The rightmost column tallies unspecified stressors for all impacts shown on the y-axis. Summing this heat map in the y and x directions yields the counts shown in Figures 2A,B, respectively.
As a result of the uncertainty propagation described above, almost one half of the identified science gaps are associated with uncertain consequences for the biological state (Figure 2B). These biological science gaps primarily surround our understanding of ongoing and anticipated changes in ocean ecosystems, fisheries, and primary productivity (Figure 3). For the remaining science gaps, 32% and 15% are associated with uncertain impacts on the physical and biogeochemical states, respectively. Major physical unknowns are associated with the changing ocean circulation (mainly the overturning component, Supplementary Table 1), sea ice/ice shelf/ice sheet variations, and sea level rise. Many science gaps related to sea level rise are an impact of the low certainty in ocean heat uptake and redistribution in the present climate system and in the future under different emissions scenarios or maximum warming targets (Supplementary Table 1). The majority of identified biogeochemical science gaps surround the ocean carbon cycle (Figure 2B). Finally, 7% of the identified science gaps do not mention specific impacts (Figure 2B), meaning that the implications for policy are not clear. These gaps are also missing acknowledgement of specific stressors (Figure 3, upper right corner) and are associated with high-level descriptions of broad climate change impacts (e.g., uncertain projections of risk under a range of climate and development pathways, Supplementary Table 1).
It is important to note that all science gaps are presented with an equal weight in Figures 2, 3, but that those harvested from the dedicated “knowledge gaps” sections of the surveyed chapters may encompass an entire field of research. As mentioned above, this is the case for half of the science gaps surrounding mitigation efforts which, as a result, have a misleadingly low count in Figures 2, 3. Many biological gaps are also taken from “knowledge gaps” sections (Supplementary Table 1). The implication is that the severe need to improve the knowledge base around biological responses to climate change is underrepresented in Figure 2B.
6.3 Origins of deep ocean uncertainty
We now inspect the origins of uncertainty for deep ocean science gaps (Figure 4) to identify key areas for priority action by the deep ocean community. Model deficiencies are the leading cited cause of low certainty in the physical ocean and ice states. In contrast, biological uncertainties in the AR6 reports are primarily attributed to limited studies, conflicting results, and limited observations. For approximately half of the science gaps identified, however, the causes of uncertainty are not referenced. As such, it is not possible to make broad declarative statements on best ways to improve confidence in the deep ocean system. Instead, we investigated a few major areas of low certainty, discussing associated gaps and their origins in more detail. The report in which each gap is presented is given in parentheses below, with full details given in Supplementary Table 1. Suggested avenues for closure of these gaps will be given in section 7.
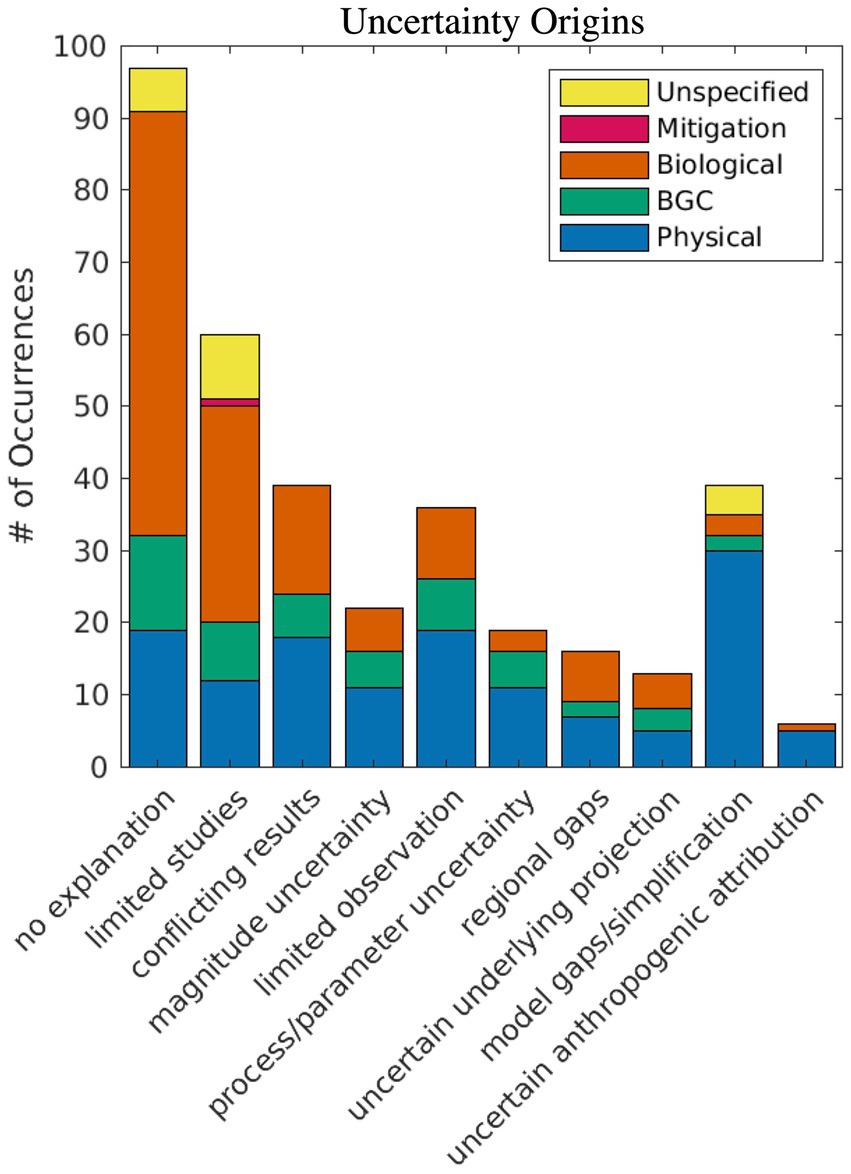
Figure 4. Origins of uncertainty associated with the deep ocean science gaps identified in the IPCC AR6 meta-analysis. Colors indicate the area in which uncertainty is manifested (see Figure 2B). The total count exceeds the number of science gaps identified (219) since each of these may arise from multiple causes listed on the x-axis. The number of occurrences corresponds directly to the number of science gaps for the “no explanation” count only. Where “no explanation” is given, the roots of low certainty may be discernible from a broader literature review, but are not explicitly referenced in the AR6 reports surveyed.
6.4 A spotlight on major areas of low confidence
6.4.1 Meridional overturning circulation
Major areas of uncertainty in the physical ocean state are related to changes in the ocean’s meridional overturning circulation (MOC) in the Atlantic and Southern Oceans. The MOC is of fundamental importance for partitioning heat and climatically-important gasses between the atmosphere and ocean, creating sequestration timescales on the order of centuries to millennia (e.g., Gebbie and Huybers, 2012). The MOC thus shapes the long-term climate response to ongoing emissions and offers the potential for climate mitigation via synthetic enhancement of CO2 uptake in high latitude deep water mass formation regions (e.g., Sarmiento et al., 2010; Bach et al., 2023). Transport estimates are sparse, however, and model spread is large, leading to low confidence in ongoing MOC changes and their potential reversibility (WGI and SROCC). Lack of direct observations of the Southern Ocean MOC in particular is cited as “a critical weakness in sustained observations of the global ocean” (SROCC). Numerous science gaps identified in the meta-analysis surround future projections of the Southern Ocean sea ice state, water mass transformations and overturning, and sensitivity to changing wind forcing. A noteworthy AR6 conclusion regarding the Atlantic MOC (AMOC) is that confidence has decreased relative to AR5 regarding the AMOC state over the 20th century. Low agreement in reconstructed and simulated variability has increased uncertainty in the occurrence of any significant weakening trend (WGI and WGII). Large uncertainty also surrounds any causal connection between anthropogenic forcing and AMOC weakening, with the transport estimates from the AMOC arrays being too short to disentangle natural and anthropogenic external forcing, and internal variability (WGI, WGII, and SR15). While ensemble projections agree on the likelihood of future AMOC weakening, there is significant spread in the projected timing and magnitude over the coming century (WGI).
6.4.2 Ocean carbon cycle
Although the deep ocean is a major carbon reservoir and has absorbed a significant fraction of anthropogenic CO2 (section 1), major observational gaps prevent rigorous quantification of temporal and spatial gradients describing deep ocean carbon change and the underlying mechanisms (SROCC), and processes linking regional flux variations to global climate (WGI). As mentioned above, data paucity in the Southern Ocean—the region dominating global CO2 uptake and its decadal variability—is particularly problematic (WGI), compounded by challenges modeling local ocean circulation and sea ice distribution (SROCC and WGI). These uncertainties mean that drivers of the temporary slowdown (hiatus) of the global ocean CO2 sink during the 1990s, to which the Southern Ocean makes an observable contribution, remain unresolved (WGI). Models also diverge on the net impact of intensifying and shifting westerlies and increased freshwater fluxes on the Southern Ocean overturning and CO2 sink over the coming century (WGI). Additionally, many major science gaps reported across the AR6 are associated with the ocean’s biological carbon pump (BCP). There is a lack of agreement not only for the amplitude but also the expected sign of the BCP response to physical and biogeochemical changes underway, including ongoing anthropogenic CO2 and heat uptake, changing stratification, ventilation, and nutrient supply (WGI and SROCC). BCP projections are plagued by persistent uncertainties in regional net primary production (NPP, see below), which are propagated along all export and sequestration pathways of the BCP, from POC fluxes away from the surface to carbon burial in ocean sediments (WGI and SROCC) and a host of ramifications for marine food webs in between. Idealized parameterizations and limited constraints in biogeochemical models lead to low certainty in the effect of rising temperature and decreasing oxygen concentrations on ecological shifts, biomass production and microbial activity, leading to low confidence in projections of biological carbon storage and biological contributions to carbon export (SROCC).
6.4.3 Primary production and food supply
Biological systems in the deep sea are intricately linked to the surface ocean through the vertical movement of marine life (e.g., the daily vertical migration of many zooplankton and micronekton-crustaceans, cephalopods and mesopelagic fishes) as well as sinking of particulate organic matter, or “marine snow” (e.g., dead phytoplankton, feces, etc). As such, changes in primary productivity in the surface ocean can have large impacts on the food supply in the deep sea via export. Numerous gaps in the SROCC and WGII surrounded uncertain changes in primary production caused by observational gaps and a limited understanding of dominant drivers. These deficiencies prevent identification and attribution of ongoing changes in timing, distribution, composition, and net production in response to ongoing warming, changing stratification, circulation and sea ice distribution (WGI, WGII, SROCC and SR15). These gaps also lead to significant spread in regional NPP projections over the coming century (WGII and SROCC), which has increased from AR5 to AR6, despite improved agreement in NPP changes over the historic period. There is also large uncertainty in how these changes will impact zooplankton biomass and propagate through the food web (WGII). Lastly, it is unclear how these changes will impact POC flux to the deep ocean and what effects a decreased food supply will have on deep ocean communities.
6.4.4 Ocean deoxygenation and acidification
Despite high certainty that climate change has caused large-scale ocean deoxygenation (Table 2), an important conclusion from the AR6 is the lack of sufficiently dense direct measurements of O2 to monitor spatial gradients and rates of change (SROCC), understand deoxygenation as a causal forcing for the expansion of oxygen minimum zones (SR15), and track mechanisms underpinning ongoing changes in the ocean carbon cycle (SROCC). Model disagreement in simulating O2 evolution over the coming century is also a key issue, resulting in significant uncertainty in anticipated regional trends, feedbacks, and impacts on natural and human systems (SROCC). Specifically, major science gaps surround the evolutionary adaptation and potential vulnerability of numerous species to decreasing oxygen, including fish, sponges and corals (WGII and SROCC), and the likelihood that ocean deoxygenation may accelerate global warming via enhanced ocean bacterial production and surface emissions of nitrous oxide (N2O, a potent greenhouse gas), despite increased stratification (WGI).
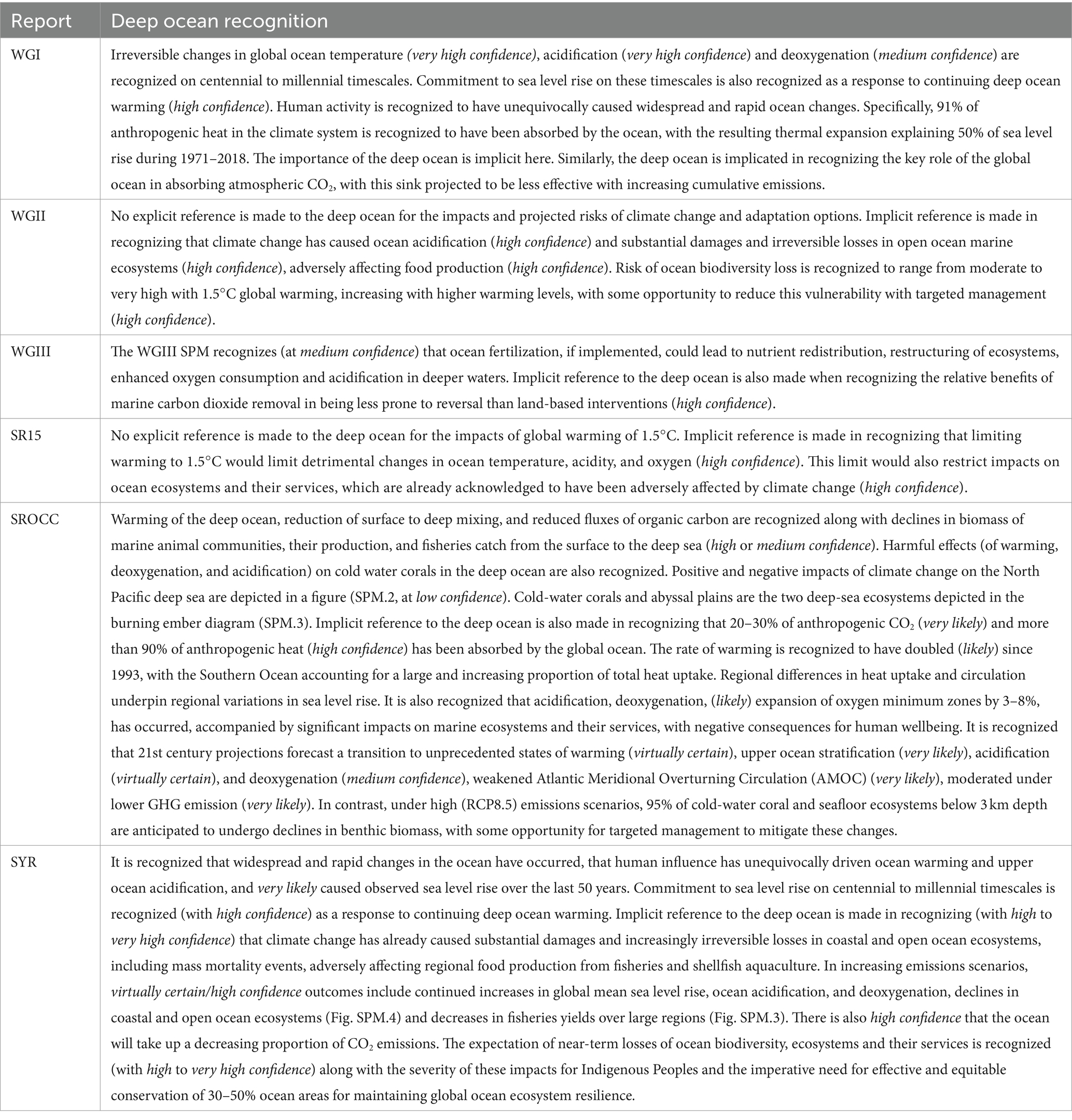
Table 2. Recognition of the deep ocean in the summaries for policy makers (SPMs) from the six reports of the IPCC AR6 reviewed in this study: Working group I “Climate Change 2021: the Physical Science Basis” (WGI, IPCC, 2021a), Working Group II “Climate Change 2022: Impacts, Adaptation and Vulnerability” (WGII, IPCC, 2022a), Working Group III “Mitigation of Climate Change” (WGIII, IPCC, 2022b), Climate Change 2023: Synthesis Report (SYR, IPCC, 2021b), Special Report on Global Warming of 1.5°C (SR15, IPCC, 2021b), and Special Report on the Ocean and Cryosphere in a Changing Climate (SROCC, IPCC, 2019). This includes both explicit mentions of deep regions as well as discussion considered relevant to the deep ocean. Note that with the exception of a burning ember diagram in the SROCC, all discussion is limited to higher certainty outcomes.
Similarly, although there is high certainty that the ocean has undergone widespread acidification in recent decades that will continue over the coming century (WGI, SROCC), major science gaps surround the ramifications of changing seawater chemistry for both natural and human systems (WGII, SROCC, SR15). Uncertainty begins at the air-sea interface and increases with depth due to dependence on poorly constrained ventilation changes and pathways which also show a large spread across future projections (WGI). Following CO2 uptake, there are uncertainties in the anticipated changing ionic composition of seawater (SR15) with poor constraints on past changes from sediment proxies (WGI). Although the detrimental impact of decreasing pH, carbonate ion (CO32−) and aragonite saturation state (Ωarag) on ocean organisms forming calciferous shells and skeletons is well established, uncertainties surround the extent of dissolution of cold-water coral habitats (SROCC), expected changes in species growth rate (SROCC), and resulting reductions in POC flux and abyssal food supply. The largest pH declines are anticipated for polar oceans (SROCC) but many science gaps surround expected changes in species distribution in response to degraded growth and recruitment at high latitudes (SROCC, WGII), and subsequent impacts on seafood supply (WG1, SR15).
6.4.5 Climate change impacts on ocean ecosystems
A large proportion of low certainty designations in the AR6 describe unknown impacts of climate related stressors—including ocean warming, acidification, deoxygenation, and declining food supply—on deep ocean ecosystems, including biodiversity, community composition and species’ habitat ranges. Potential degradation of many important and biodiverse deep-sea habitats, including seamounts, cold-water coral reefs, slopes, and abyssal plains, are recognized (SROCC), along with uncertain impacts of changing gyre (WGI) and eastern boundary upwelling systems (WGII) on local ecology. Many science gaps surround the vulnerability and extinction risk or evolutionary adaptability of various species, particularly those at high latitudes (WGII and SROCC) and deep-water corals (WGII and SROCC). Importantly, since temperatures are more stable in the deep ocean compared to epipelagic systems (0–200 m depth), deep-sea organisms may have narrower thermal envelopes and lower adaptive capabilities, which could be further exacerbated by their slow growth rates. Many science gaps surrounding climate change impacts on ocean ecosystems result from the severe paucity of deep-ocean biological data, as illustrated by the hypothesis that most species in the deep sea are yet to be discovered. We lack present-day baseline information on biodiversity, ecosystem biomass, food web structure, and the BCP, which limits our capacity to quantify change and fully understand vulnerability and risk (SROCC). These science gaps also limit our ability to reliably simulate deep sea biological processes and interactions in earth system models.
6.4.6 Climate change impacts on fisheries
Deep-sea commercial fisheries target a range of invertebrates and fish. Although poleward expansion and redistribution of species is anticipated in response to ocean warming, large uncertainties surround estimated habitat range shifts (WGII, SROCC, SR1.5) and impacts on food security. There is also low confidence in the extent of fishery productivity declines in low and mid-latitudes where warming and/or increased stratification will decrease primary production. The additive responses of fisheries and climate-related stressors (warming, acidification, and deoxygenation) on fish distributions and biomass emerge as low confidence in WGII. Similarly, the SROCC documents projected changes to deep-sea environments within the jurisdictions of various Regional Fisheries Management Organizations, but there is limited information about impacts on fish biomass or catch. Additionally, there is uncertainty in the magnitude of change in fish size and biomass in the deep scattering layer with increased warming and changes in POC flux to the deep ocean, which fuels deep food webs, impacting animal body size and overall fisheries catch.
6.4.7 Ocean-based climate interventions
Several reports within AR6 (SR15, WGIII, SYR) state that active CDR will be required alongside decarbonization to limit global warming to within the Paris Agreement’s targets and mitigate the worst intergenerational impacts of climate change. Due to the large reservoir capacities and long residence timescales of the deep ocean, attention is being turned to mCDR, including both biotic (e.g., macroalgae cultivation and sinking, ocean iron fertilization, artificial upwelling, and crop and wood waste disposal) and abiotic (e.g., ocean alkalinity enhancement, electrochemical mCDR) approaches. These methodologies are now under intense investigation fuelled by industrial, philanthropic and government interest. Their discussion in AR6 is largely restricted to the knowledge gaps section of WGIII and SR15, highlighting that this emerging field has largely lacked critical research infrastructure to date. Here it is noted that mCDR has not been comprehensively analyzed in integrated assessment approaches or accounting for interactions (synergies and trade-offs) between different mCDR methods employed together. Critically, the deep sea is the carbon repository for most mCDR technologies. However, impacts on deep-ocean environments, processes and ecosystems have yet to be assessed. The AR6 also acknowledges that mCDR technologies are neither ready to be deployed at scale nor regulated in the absence of the necessary frameworks (SR15).
7 Recommendations for future research
In section 6.5 we highlighted seven major areas of deep ocean uncertainty. Below we offer actionable recommendations for tackling uncertainty in each area.
7.1 Meridional overturning circulation
Key recommendations for improving observation-based estimates of MOC include addressing uncertainties in existing measurements at the AMOC arrays (e.g., transport calculation errors and instrument drifts; Frajka-Williams et al., 2018, Danabasoglu et al., 2021), and better integrating these data with independent observing networks (e.g., Biogeochemical (BGC)-Argo, Deep Argo, SWOT) and the physical and biogeochemical quantities they monitor (e.g., heat, carbon, oxygen, nutrients). Developing sustainable, long term monitoring strategies is an important goal that demands tighter collaboration between observing, modeling, and data assimilation communities to conduct extensive observing system design experiments (Brown et al., 2024). Common frameworks for model-data comparisons are essential for this task and can be obtained via data assimilation (e.g., Nguyen et al., 2021), detailed metric evaluation (e.g., Danabasoglu et al., 2021) and model intercomparison projects (MIPs). Past MIPs have isolated the impacts of atmospheric forcing (Danabasoglu et al., 2014, 2016) and model resolution choices (Roberts et al., 2020) and new MIPs have been proposed to determine best representation of overflows and expose differences in Lagrangian pathways (Jackson et al., 2023). We emphasize that the latter would allow for better appreciation of the zonal asymmetries (Tamsitt et al., 2017; Gruber et al., 2019b) and distinct dynamical regimes masked within the 2D overturning metric (Yung et al., 2022; Youngs and Flierl, 2023), which is essential for understanding ocean variability and its climate impacts (Wunsch and Heimbach, 2012). Detailed intercomparison of water mass transformation budgets would further elucidate bias origins and help pinpoint where additional observational constraints or process studies are required (Drake et al., 2024). Without these investments, common model deficiencies, including excessive deep convection in the Subpolar North Atlantic, excessive mixing in the overflows, a spuriously shallow depth of maximum overturning and weak northward heat transport in the Atlantic (Roberts et al., 2020; Weijer et al., 2020) will persist through AR7. We advocate for coordinated effort between model developers, observational oceanographers, theoreticians and process experts to improve model representation of high latitude water mass transformations and follow Jackson et al. (2023) in highlighting that US CLIVAR’s Climate Process Team (Subramanian et al., 2016) would offer an effective mechanism to organize across multiple modeling teams and research agencies to accelerate progress in this area.
7.2 Ocean carbon cycle
Constraining the global ocean carbon sink is a societal and environmental challenge, integrating physical changes in ocean circulation, sea ice, and air-sea exchange, as well as biogeochemical and biological dynamics of intertwined carbon, macronutrient, and micronutrient cycles. These drivers feed into carbon cycle variability and trends in primary production, export fluxes, and remineralization, ultimately determining the sequestration of carbon in the deep ocean and exchange of CO2 with the atmosphere. Discrepancies of up to 30% currently exist between globally-integrated ocean carbon sink estimates derived from air-sea CO2 flux products based on surface-ocean pCO2 observations, accumulation of carbon in the ocean’s interior based on dissolved inorganic carbon (DIC) observations, and ocean biogeochemistry models (Friedlingstein et al., 2023). Complexity of carbon cycle models has increased significantly, along with the number of free parameters, thus limited observations and conflicting results put future progress in constraining the ocean carbon variability and novel parameterization development on an unsteady scientific footing. Biogeochemical and ecosystem state estimates (e.g., Carroll et al., 2020, 2022) show promise in closing the carbon budget “gap,” within the constraints (and assumptions) of a particular model framework, but it is yet to be seen whether climate-driven trends in the ocean carbon sink may be captured for the “right” reasons.
Specific areas that need further research cover the breadth of the ocean carbon cycle. An essential goal is to achieve a comprehensive understanding of the variability, trends, and drivers of the atmosphere–ocean flux of CO2 and improve representation of air-sea gas exchange in models. Current (CMIP6) models are limited in parameterizing gas transfer velocity as a function of 10 m wind speed (Orr et al., 2017), therefore omitting the effects of surface roughness and bubble-mediated transfer (e.g., Woolf, 2005; Fangohr and Woolf, 2007; Deike and Melville, 2018; Reichl and Deike, 2020). These processes can alter CO2 fluxes by 20–30%, particularly in stormy regions and locations with tight coupling between the surface and deep ocean such as the North Atlantic, North Pacific and the Southern Ocean. Future work should address these omissions and also further interrogate how sea ice variations impact air-sea carbon fluxes through opposing effects of capping and light attenuation (e.g., Gupta et al., 2020).
There is an urgent need for large-scale, frequently-repeating observations of ocean carbon, for which BGC-Argo floats equipped with pH sensors are ideally suited (Addey, 2022). An expanded BGC-Argo array has the potential to provide complete seasonal and annual cycles of pCO2 derived from pH throughout the upper 2 km of the global ocean (Williams et al., 2017). These data would be complemented by leveraging Saildrones and other uncrewed surface vehicles to collect observations at the atmosphere–ocean interface (e.g., Nickford et al., 2022) and by augmenting existing moored arrays (e.g., OSNAP/RAPID) with BGC sensors to better understand how changes in large-scale overturning regulate air-sea carbon exchange and sequestration (Atamanchuk et al., 2021). Development of robust algorithms and/or platforms for sampling under sea ice should also be pursued. Following the suggestion that observed variations in the Southern Ocean carbon sink can be attributed to sampling heterogeneity (Gloege et al., 2021), we emphasize the importance of increasing funding and expanding research capacity in the Global South to address data paucity in this region of strong carbon uptake.
The gravitational, mixed-layer, eddy subduction, seasonal lipid and migrant (or active) pumps contributing to the total BCP (Boyd et al., 2019) are all characterized by significant uncertainty. The gravitational pump—associated with particle production and sinking—dominates the total vertical carbon flux, with an amplitude estimated to be equal to that of the remaining pumps combined (Boyd et al., 2019). Many studies have attempted to address uncertainty in various aspects of this pump (particle export, sinking, and remineralization) though scrutiny of relatively simple mathematical functions (e.g., the “Martin Curve” power law; Martin et al., 1987; Henson et al., 2012; Gloege et al., 2021, Wilson et al., 2019; Lauderdale and Cael, 2021) or use more sophisticated models to account for additional (but incompletely constrained) processes that shape “marine snow,” including density and ballast effects, aggregation/disaggregation, lability, and bacterial degradation rates. Additional observations from sediment traps would provide valuable insight into dominant carbon exporters. These observations would be complemented by leveraging novel technologies and autonomous platforms that combine different sensors (e.g., active acoustics, optics, eDNA, etc.) to enable better characterization of sinking particle processes.
Following the gravitational pump, the migrant pump is estimated to drive the second largest carbon export rates and is important for penetrating deeper below the surface to contribute to long term sequestration (Boyd et al., 2019). This pump is driven by the diurnal vertical migration of zooplankton and micronekton (Longhurst et al., 1990; Archibald et al., 2019; Saba et al., 2021) and is poorly represented in climate models (e.g., Usbeck et al., 2003; Orr et al., 2017). The contribution of specific migratory groups (e.g., mesopelagic fishes, squids, crustaceans, and jellyfish) to the movement of carbon is poorly constrained due to large uncertainties in life history, bioenergetics, biomasses, community compositions, and migration dynamics and controls (Caiger et al., 2021; Saba et al., 2021; Kaartvedt et al., 2012; Irigoien et al., 2014; Proud et al., 2019; Klevjer et al., 2016; McMonagle et al., 2023). Filling these gaps requires a combination of novel technology [e.g., acoustics (either from shipboard ADCP or AUVs), eDNA], video imagery data (which could be coupled with AI), and net sampling, as each approach can bias against specific faunal groups. Better estimating the contribution of mesopelagic fishes is a key target for future research, since their large biomass (1–16 billion metric tons; Kaartvedt et al., 2012; Irigoien et al., 2014; Proud et al., 2019) suggests they likely make the dominant contribution to the migrant pump (15–40% of the total carbon flux; Davison et al., 2013; Cavan et al., 2019; McMonagle et al., 2023). The eddy subduction pump (Omand et al., 2015; Freilich et al., 2024) is also associated with a strong and efficient carbon export to the mid-depth ocean (Boyd et al., 2019). Coincident observations of both biology and physics at high spatial and temporal resolution are required to capture the small-scale episodic nature of this flux. Strategic deployment of additional BGC-Argo floats (e.g., in undersampled regions of high eddy kinetic energy, Llort et al., 2018) will be critical to reduce uncertainties in the eddy subduction pump and estimate its importance on the global scale.
7.3 Ocean deoxygenation and acidification
Although previous work has investigated effective sampling of temperature using a Deep Argo Array (Johnson et al., 2015) and shown the value of these acquisitions in data assimilating systems (Gasparin et al., 2020), a sampling protocol for deep oxygen on Deep Argo floats has yet to undergo rigorous design. Key questions to answer include determining suitable parking depths to assign for monitoring changing water mass characteristics (Racape et al., 2019) while minimizing drift outside of deep ocean regions (Zilberman et al., 2020), suitable 4,000-meter and 6,000-meter capable float populations, and necessary profile density for monitoring key space–time variability. Consideration of oxygen as a 10th planetary boundary (Rose et al., 2024) and development of a global ocean oxygen database and atlas (GO2-DAT, Gregoire et al., 2021) emphasize the critical need for global deep ocean oxygen data. Similarly, several studies have explored the value of upper ocean pH measurements for constraining regional air-sea CO2 fluxes (Lenton et al., 2006; Majkut et al., 2014) but suitable network characteristics for monitoring global acidification variability and the effects of a changing carbonate saturation state remain unexplored.
BGC-Argo floats are equipped with sensors for the direct measurement of pH and O2 from 0 to 2000 m, providing an unprecedented opportunity to revolutionize our understanding of ocean deoxygenation, carbon uptake and acidification (Roemmich et al., 2019). We recommend extensive collaboration between observing and modeling groups to inform their global expansion. Recommended approaches include assessments of decorrelation length scales, Observing System (Simulation) Experiments, and identification of distinct biogeochemical provinces (Sayre et al., 2017) to determine suitable distribution of assets (see Biogeochemical-Argo Planning Group, 2016, and references therein). To avoid shortcomings inherent in using limited observational data sets or any single imperfect model in isolation, we recommend a diversified approach to offer robust guidance on the design of fit-for-purpose global deoxygenation and acidification observing systems that remain effective with ongoing change, and demonstrate the value of these investments to funding agencies. Integrating these observations with those from fixed networks (e.g., cabled observatories, benthic landers) collecting biological data will be important for connecting ecosystem changes to large-scale environmental variability. These observations will improve our understanding of the metabolic costs and benefits of inhabiting an increasingly acidic (e.g., Figuerola et al., 2021) and deoxygenated (e.g., Zakem et al., 2020, 2021) ocean, and reduce uncertainty in future production of potent greenhouse gasses (e.g., N2O).
7.4 Climate change impacts on primary production, food supply, ocean ecosystems, and fisheries
There are intricate links between the many biological components of the deep ocean and the sea surface. While primary production occurs in the euphotic zone, it fuels most oceanic food webs. A better understanding of regional changes in primary production is therefore critical to predicting changes in animal abundance and biomass and carbon uptake via the BCP. Examining climate change impacts on deep ocean biology first requires baseline data. Several initiatives are currently working toward obtaining this, including Seabed2030 (Mayer et al., 2018), Challenger 150 (Howell et al., 2020), and the Ocean Census (Rogers et al., 2023). Seabed2030 aims to map the entirety of the ocean floor to high resolution (minimum grid cell size 800 × 800 m below 5,750 m depth increasing to 100 × 100 m above 1,500 m depth) within this decade and will provide detailed maps of benthic habitats. Challenger150 and the Ocean Census are initiatives aiming to expand deep-sea biological data, create best practices for collecting and reporting biodiversity data, build capacity for deep-sea research, and accelerate the discovery of ocean life.
While long-term biological sampling programs exist for epipelagic systems in few regions of the world’s ocean (e.g., California Cooperative Oceanic Fisheries Investigations; Continuous Plankton Recorder Survey in Plymouth, UK; Bermuda Atlantic Time Series in the Sargasso Sea), long-term deep ocean time series are very limited worldwide (Larkin et al., 2010). Support for programs monitoring deep ocean biodiversity and ecosystems has grown over time. Some of these programs (e.g., Station M in the northeast Pacific or Porcupine Abyssal Plain Sustained Observatory in the northeast Atlantic) provide invaluable insights into connections between the surface and the deep sea (Hartman et al., 2021; Smith et al., 2023; Messié et al., 2023) furthering our understanding of carbon cycling and the response of deep ocean biology and ecosystems to climate stressors. Maintaining and expanding these networks are critical efforts that will be aided by leveraging recent advances in low-cost deep technology. Resilient video platforms comprising off-the-shelf products (Dominguez-Carrió et al., 2021) and emerging eDNA-based approaches (Thomsen and Willerslev, 2015; Yang et al., 2024) are notable examples of new technologies enabling accurate large-scale surveys of deep ocean biodiversity and new species discovery.
There is large uncertainty in estimates of deep-sea biomass and projected changes under different climate scenarios. For deep-sea benthos, additional work from marine ecology scientists is needed to minimize bias and enhance precision of estimating biomass from marine imagery (Durden et al., 2016; Benoist et al., 2019) and further collaborative work with compute vision scientists to automate annotation techniques (Borremans et al., 2024). Such shortfalls, together with lack of fundamental ecological knowledge of deep-sea benthic fauna, are limiting the predictive power of models forecasting reductions in seafloor biomass in response to climate change-induced reductions of POC fluxes to the deep (Jones et al., 2014; Yool et al., 2017). Uncertainty also has important implications for fisheries. Some studies have suggested that mesopelagic biomass is large and will increase with warming (Proud et al., 2019), which has attracted more commercial interest in mesopelagic fisheries (Fjeld et al., 2023), while others have suggested a decrease with warming (Ariza et al., 2022). Future studies that quantify mesopelagic biomass and the varied migration patterns of mesopelagic organisms are needed to inform the management of current and potential future fisheries resources. Conversely, fishing in the deep sea has historically led to rapid and sustained declines in species abundance (Clark et al., 2016) and potential uptake in mining activities may lead to decade-long disturbances to communities and their composition (Gollner et al., 2017; Drazen et al., 2020). Improved modeling of impending economic activities in relation to changing ocean conditions, species redistributions, and life histories is also needed to facilitate projections for deep-sea fish, habitats, and fisheries (FAO, 2019; Levin et al., 2020). For example, improved understanding is needed of how hypoxia, warming and acidification affect fisheries resources, via avoidance, shoaling, deepening or competitive advantage via tolerance. This also has implications for the carbon cycle (Lutz et al., 2018; Saba et al., 2021).
7.5 Ocean-based climate interventions
There is an essential and urgent need to expand and improve an unbiased knowledge base around mCDR to evaluate both positive and negative potential outcomes over different spatial and temporal scales, develop monitoring strategies, quantify uncertainties, and inform deployment decisions and policy (Levin et al., 2023). Since the deep ocean is characterized by low energy supply, typically cold and stable conditions, and organisms with narrow thermal envelopes, slow growth rates and lower adaptive capabilities, OBCI impacts on the deep ocean demand targeted investigation (Levin et al., 2023). AR7 planning includes an Expert Meeting and Methodology Report on CDR technologies and carbon capture utilization and storage. We recommend that including deep-ocean processes, altered functions, unintended consequences, and feedbacks of mCDR in this report will be critical. Potential deep ocean impacts of different OBCI strategies are detailed in Levin et al. (2023), offering valuable guidance on specific questions to address. In addition, closing all other gaps outlined above will significantly advance our ability to evaluate mCDR efficacy, assess additionality, and determine desirability. A recent National Academies report (NASEM, 2022) offered a first-order attempt at prioritizing various technologies for research based on factors including estimated efficacy, durability, scalability, environmental risk, and monitoring potential. These findings encourage that the research community’s efforts around mCDR should be focused on exploring the efficacy and impacts of iron fertilization, seaweed cultivation, and ocean alkalinity enhancement.
8 Recommendations for future reporting
The science gaps presented here demonstrate that low confidence surrounds numerous acutely important and policy relevant issues in the deep sea. With the current IPCC reporting protocol, however, these issues are shielded from policy makers and public view as the high-level summaries that attract the attention of both policy makers and the media focus largely on high certainty claims (Figure 1). This focus reflects the established reporting culture among IPCC authors, with many viewing the primary purpose of IPCC assessments as the communication of robust, high certainty findings (Janzwood, 2020). Levin (2021) has called attention to the potential pitfalls of this culture, particularly for the deep ocean, noting the IPCC’s failure to highlight vulnerability and risk in areas where research is largely absent and proposing inverse assessment reports to summarize low certainty issues of potentially high impact. We reiterate that this would expose where policy cannot be supported by an adequate evidence base and provide valuable direction for researchers and funding agencies. We recognize, however, that this is a challenging proposal to implement given the requirement for all SPMs to undergo line-by-line approval by all participating governments. For this reason, we also outline actionable recommendations below that do not require significant changes to the current reporting system and could be implemented for the IPCC’s 7th Assessment Report (AR7) that is currently underway (completion due 2029).
In section 6.2 we showed that specific information around the underlying cause (“stressor”) and ramification (“impact”) is missing for many science gaps identified in the AR6. The result is that the associated risk, research needs, and policy implications are obscured for these themes. The latest author guidelines state that “the reason for [the] presentation [of low confidence] should be carefully explained” (Mastrandrea et al., 2010) and we encourage that this is more strongly emphasized for the next assessment cycle. We also encourage the mandatory inclusion of a dedicated knowledge gaps section or appendix for every IPCC chapter, to highlight major structural deficiencies in climate research. These could be accompanied by a risk assessment of each science gap to enhance the visibility of understudied components with potentially substantial repercussions for the climate system. While recognizing that authors are subject to strict word count limits, we stress that these areas merit attention and that it would be highly beneficial to clearly communicate where research needs are most profound. Furthermore, since the deep ocean was implicitly implicated in many of the science gaps identified in this study, we also encourage that explicitly referring to the deep ocean in future reporting would help to make this important environment more visible. These recommendations are also relevant for other international and intergovernmental assessments employing qualitative confidence frameworks, such as the Intergovernmental Science-Policy Platform on Biodiversity and Ecosystem Services (IPBES, 2019), and the UN General Assembly’s World Ocean Assessment III, to avoid undervaluing emerging ocean themes and issues of great potential importance that have yet to be investigated in detail.
Finally, we note that indigenous and local knowledge that has not been disseminated through peer reviewed literature is largely missing from the reports (Carmona et al., 2023) and as a result is not represented in our meta-analysis. Similarly, we recognize the particularly striking absence of social science knowledge gaps (e.g., technological advances, policy making, public perception of the deep sea, generational considerations) from our results, which we attribute to this area being largely overlooked by the reports. The IPCC reports are significantly shaped by author training, disciplinary background, organizational affiliation, and geographical location and expertise. These factors result in a framing that continues to predominantly reflect the perspective of natural science scholars from the Global North (Tandon, 2023), a perspective that is further refined by additional inequities within this cohort (De Vos et al., 2023). Including marginalized voices in the process would improve the identification of science gaps and help highlight high impact gaps. For instance, accurate risk assessments require identifying stressor-impact connections across social and ecological domains (Wassénius and Crona, 2022). Integrated assessments as proposed in our recommendations would considerably expand the current approach for how and by whom science gaps are identified and assessed, demanding insights from a diversity of knowledge holders. We recommend that greater investment to promote diversity, equity and inclusivity in the deep ocean space (De Vos et al., 2023), and engage social science experts (beyond economists, Maxwell et al., 2022), scholars from the Global South, and indigenous knowledge holders (Carmona et al., 2023) in the co-production of IPCC reports will be essential for the next assessment cycle. This will ensure a more balanced and comprehensive assessment of how communities are experiencing and responding to climate change, and thus support the development of improved adaptation strategies and increased resilience.
9 Study limitations
The IPCC AR6 was chosen as the foundation of this study since it offers the most recent and extensive summary of peer reviewed literature from across the global research community addressing all aspects of climate change. It also allowed for objective identification of major science gaps across all six reviewed reports via the IPCC formal certainty language framework and the inclusion of dedicated knowledge gaps sections. We note, however, that the latter were not mandatory for IPCC authors and are present for less than half of the AR6 chapters reviewed. Although the IPCC’s formal language framework is designed to allow consistent communication of certainty across topics and research methodologies, inconsistent use has been reported within earlier IPCC assessment cycles (Adler and Hirsch Hadom, 2014; Mach et al., 2017) and differing author group preferences are also detectable, albeit to a lesser extent, in AR6 (see Supplementary Figure S1 and also Janzwood, 2020), which may have led to omissions from our meta-analysis. Furthermore, we acknowledge that while our choice to focus on confidence designations helped avoid ambiguities and retain some degree of objectivity in deciding which science gaps are major (section 5), this choice may have led to omission of gaps expressed with low agreement/limited evidence or very low/low likelihood.
Our decision to enroll two assessors per chapter aimed to ensure the correct identification and categorization of all deep ocean related science gaps. Accidental omissions may, however, still have occurred. This is most likely where low certainty is referenced in the legend of a figure and may not have been detectable in the scan for the low confidence string. Similarly, subjective evaluation was required to determine which major science gaps were deep sea relevant (in the absence of an explicit reference) and different individual perspectives may have led to further omissions. Acknowledging couplings and nonlinearities in the climate system and allowing for sufficiently long timescales, many omitted issues—notably those involving terrestrial and ice sheet processes—are arguably relevant to the deep sea. Our rationale was to retain science gaps with direct implications for future deep ocean observing and modeling efforts.
We acknowledge that the deep ocean is defined differently within the research community, and some may dispute the 200 m depth delineation used here. It is common to find discussions of the deep ocean referencing depths >1000 m (e.g., Hoegh-Guldberg et al., 2014) or 2000 m (e.g., Smith et al., 2009). Differing choices may reflect discipline or specific research topic/region norms (e.g., the depth at which the southward deep limb of the AMOC is found), and limits of particular observational capabilities (e.g., the maximum depth of BGC and Core Argo arrays). Our choice is adopted from the SROCC (IPCC, 2019) and has relevance from physical, biogeochemical and biological perspectives. This definition is also adopted by the Deep Ocean Observing Strategy (DOOS, Smith et al., 2023), Deep-Ocean Stewardship Initiative (DOSI, 2018), and Ocean Discovery League (Bell et al., 2022). These global scientific networks have demonstrated that consistent use of this nomenclature aids discussion of observational assets and coordination of research activities essential for tracking capacity development and accelerating generation and use of deep ocean data during the United Nations (UN) Ocean Decade (e.g., Bell et al., 2022, 2023; Amon et al., 2022b). Finally, we emphasize that the 219 science gaps identified in our study—and the 7 major areas of deep ocean uncertainty highlighted in section 6.4 - represent only the “known unknowns”. There may be more critical deep ocean risks and vulnerabilities we have yet to discover.
10 Summary
The deep-sea is a critically important and under-explored component of the Earth system, currently undergoing substantial changes in response to anthropogenic greenhouse gas emissions and exposed to emerging anthropogenic stresses from expanding resource extraction and climate interventions. Many policy and regulatory decisions are under development that will determine the fate of the deep ocean and its ecosystems for generations to come. Examples include decisions about hydrocarbon extraction, mineral mining from the deep-seabed to facilitate electrification of transport systems, managing bottom trawling to avoid ecosystem damage and carbon release, protecting 30% of the ocean to conserve biodiversity, sharing benefits from marine genetic resources, and regulating CO2 injections, biomass sinking, and iron additions (as pollutants). Advancing understanding of the MOC, the ocean carbon cycle and biological pump, deoxygenation and acidification, as well as the impacts of changes in these on ecosystems and fisheries will greatly inform ongoing decision making in these areas.
Given the importance of ocean science to ocean policy making, the UN has declared 2021–2030 the UN Decade of Ocean Science for Sustainable Development. Ten major challenges have been selected for focusing Decade actions, many of which are pertinent to this study, including expanding ocean observing, exploring ocean-based solutions to climate change, and protecting biodiversity. The meta-analysis of the IPCC AR6 literature presented in this study offers direction for approaching these challenges by identifying major deep ocean science gaps that must be addressed to support science-based decision making around climate change. Many of the major gaps identified surround interactions of multiple physical, biogeochemical and biological stressors, culminating in potentially major impacts on deep ocean ecosystems (and their services) and the ongoing regulatory capacity of the deep ocean in the Earth’s climate system. This highlights the urgent need for a new era of extensive collaboration in an increasingly diverse, equitable and inclusive ocean space, to exchange information and tools across research disciplines, co-design and implement an effective deep ocean observing system, and develop skillful numerical models for trustworthy predictions informing mitigation and management efforts.
Data availability statement
The original contributions presented in the study are included in the article/Supplementary material, further inquiries can be directed to the corresponding author/s.
Author contributions
HP: Conceptualization, Data curation, Formal analysis, Investigation, Methodology, Visualization, Writing – original draft. EH: Conceptualization, Data curation, Investigation, Methodology, Writing – original draft. LL: Conceptualization, Data curation, Investigation, Methodology, Writing – original draft, Funding acquisition. LC: Conceptualization, Data curation, Investigation, Methodology, Writing – original draft. JL: Investigation, Writing – original draft. JG: Investigation, Writing – original draft. KJ: Formal analysis, Writing – review & editing. PH: Conceptualization, Funding acquisition, Writing – review & editing. LS: Conceptualization, Funding acquisition, Writing – review & editing. CA: Investigation, Writing – review & editing. PA: Investigation, Writing – review & editing. SA: Investigation, Writing – review & editing. NB: Investigation, Writing – review & editing. HD: Investigation, Writing – review & editing. EE: Investigation, Writing – review & editing. LE: Investigation, Writing – review & editing. MF: Investigation, Writing – review & editing. NG: Investigation, Writing – review & editing. FG: Investigation, Writing – review & editing. MH: Investigation, Writing – review & editing. DJ: Investigation, Writing – review & editing. SJ: Investigation, Writing – review & editing. XL: Investigation, Writing – review & editing. PM: Investigation, Writing – review & editing. OSa: Investigation, Writing – review & editing. PS: Investigation, Writing – review & editing. OSu: Investigation, Writing – review & editing. DT: Investigation, Writing – review & editing.
Funding
The author(s) declare that financial support was received for the research, authorship, and/or publication of this article. HP, EH, LC, LL, LS, and PH were funded by the NSF AccelNet program, Award #2114717 and its associated subcontracts including subaward #308056–0001. HP received additional funding with DT from NSF Award #2242743. DT was supported by the Cooperative Institute for Climate, Ocean, and Ecosystem Studies (CISESS) at the University of Maryland/ESSIC. DJ was funded through the UK Natural Environment Research Council Climate Linked Atlantic Sector Science project (NERC reference NE/R015953/1). JML was supported by NASA Carbon Cycle Sciences Award #80NSSC22K0153. MJH was supported by a grant from OceanX—Dalio Philanthropies (award ID 22-08846). NDG was supported by a grant from the Research Council of Norway (HypOnFjordFish project number: 301077). SJ was self-funded. SA was supported by CONAHCYT (CVU: 698614). MAF was supported by a Scripps Institution of Oceanography postdoctoral fellowship.
Acknowledgments
We thank all IPCC expert author groups for volunteering their time and expertise to produce the AR6 reports used in this study. We are grateful to the DOOS and DOSI communities for helpful feedback over the course of this study, and to two reviewers, Detelina Ivanova and Anna Pirani, for providing thoughtful and constructive comments that significantly improved the paper. The optical character recognition software used in this study is available at https://cloud.google.com/document-ai.
Conflict of interest
LS was employed by the Your Ocean Consulting, LLC. PS was employed by The Biodiversity Consultancy.
The remaining authors declare that the research was conducted in the absence of any commercial or financial relationships that could be construed as a potential conflict of interest.
The author(s) declared that they were an editorial board member of Frontiers, at the time of submission. This had no impact on the peer review process and the final decision.
Publisher’s note
All claims expressed in this article are solely those of the authors and do not necessarily represent those of their affiliated organizations, or those of the publisher, the editors and the reviewers. Any product that may be evaluated in this article, or claim that may be made by its manufacturer, is not guaranteed or endorsed by the publisher.
Supplementary material
The Supplementary material for this article can be found online at: https://www.frontiersin.org/articles/10.3389/fclim.2024.1445694/full#supplementary-material
References
Addey, C. I. (2022). Using biogeochemical Argo floats to understand ocean carbon and oxygen dynamics. Nat. Rev. Earth Environ. 3:739. doi: 10.1038/s43017-022-00341-5
Adler, C., and Hirsch Hadom, G. (2014). The IPCC and treatment of uncertainties: topics and sources of dissensus. Wiley Interdiscip. Rev. Clim. Chang. 5, 663–676. doi: 10.1002/wcc.297
Amon, D., Gollner, S., Morato, T., Smith, C., Chen, C., Christiansen, S., et al. (2022a). Assessment of scientific gaps related to the effective environmental management of deep-seabed mining. Mar. Policy 138:105006. doi: 10.1016/j.marpol.2022.105006
Amon, D., Rotjan, R. D., Kennedy, B. R. C., Alleng, G., Anta, R., Aram, E., et al. (2022b). My deep sea, my backyard: a pilot study to build capacity for global deep-ocean exploration and research. Phil. Trans. R. Soc. 377:20210121. doi: 10.1098/rstb.2021.0121
Archibald, K., Siegel, D. A., and Doney, S. C. (2019). Modeling the impact of zooplankton diel vertical migration on the carbon export flux of the biological pump. Glob. Biogeochem. Cycles 33, 181–199. doi: 10.1029/2018GB005983
Ariza, A., Lengaigne, M., Menkes, C., Lebourges-Dhaussy, A., Receveur, A., Gorgues, T., et al. (2022). Global decline of pelagic fauna in a warmer ocean. Nat. Clim. Chang. 12, 928–934. doi: 10.1038/s41558-022-01479-2
Atamanchuk, D., Palter, J., Palevsky, H., Le Bras, I., Koelling, J., and Nicholson, D. (2021). Linking oxygen and carbon uptake with the meridional overturning circulation using a transport mooring array. Oceanography 34:4. doi: 10.5670/oceanog.2021.supplement.02-03
Bach, L. T., Tamsitt, V., Baldry, K., McGee, J., Laurenceau-Cornec, E. C., Strzepek, R. F., et al. (2023). Identifying the most (cost-)efficient regions for CO2 removal with Iron fertilization in the Southern Ocean. Glob. Biogeochem. Cycles 37:e2023GB007754. doi: 10.1029/2023GB007754
Baross, J., and Hoffman, S. (1985). Submarine hydrothermal vents and associated gradient environments 598 as sites for the origin and evolution of life. Orig. Life Evol. Biosph. 15, 327–345. doi: 10.1007/BF01808177
Bell, K. L. C., Chow, J. S., Hope, A., Quinzin, M. C., Cantner, K. A., Amon, D. J., et al. (2022). Low-cost, deep-sea imaging and analysis tools for deep-sea exploration: a collaborative design study. Front. Mar. Sci. 9:873700. doi: 10.3389/fmars.2022.873700
Bell, K., Quinzin, M. C., Amon, D., Poulton, S., Hope, A., Sarti, O., et al. (2023). Exposing inequities in 601 deep-sea exploration and research: results of the 2022 global deep-sea capacity assessment. Front. Mar. Sci. 10:1217227. doi: 10.3389/fmars.2023.1217227
Benoist, N. M., Bett, B. J., Morris, K. J., and Ruhl, H. A. (2019). A generalised volumetric method to estimate the biomass of photographically surveyed benthic megafauna. Prog. Oceanogr. 178:102188. doi: 10.1016/j.pocean.2019.102188
Bindoff, N. L., Cheung, W. W., Kairo, J. G., Arístegui, J., Guinder, V. A., Hallberg, R., et al. (2019). “Changing ocean, marine ecosystems, and dependent communities” in IPCC Special Report on the Ocean and Cryosphere in a Changing Climate. eds. H. O. Portner, D. Roberts, V. Masson-Delmotte, and P. Zhai (Cambridge: Cambridge University Press).
Biogeochemical-Argo Planning Group (2016). “Biogeochemical-argo science and implementation plan,” in The scientific rationale, design and implementation plan for a biogeochemical-argo float array. Eds K. Johnson and H. Claustre (France: IFREMER). Available at: https://archimer.ifremer.fr/doc/00355/46601/46508.pdf (Accessed March 6, 2024).
Borremans, C., Durden, J., Schoening, T., Curtis, E., Adams, L., Albu, A. B., et al. (2024). Report on the marine imaging workshop 2022. Res. Ideas Outcomes 10:e119782. doi: 10.3897/rio.10.e119782
Boyd, P. W., Claustre, H., Levy, M., Siegel, D. A., and Weber, T. (2019). Multi-faceted particle pumps drive carbon sequestration in the ocean. Nature 568, 327–335. doi: 10.1038/s41586-019-1098-2
Brown, P., Chassignet, E., Danabasoglu, G., de Young, B., Frajka-Williams, E., Foukal, N., et al. (2024). Workshop report: Meeting AMOC observation needs in a changing climate. Boulder, CO: US CLIVAR.
Caiger, P. E., Lefebve, L. S., and Llopiz, J. K. (2021). Growth and reproduction in mesopelagic fishes: a literature synthesis. ICES J. Marine Sci. 78, 765–781. doi: 10.1093/icesjms/fsaa247
Campanya-Llovet, N., Snelgrove, P., and Parrish, C. (2017). Rethinking the importance of food quality in marine benthic food webs. Prog. Oceanogr. 156, 240–251. doi: 10.1016/j.pocean.2017.07.006
Carmona, R., Reed, G., Thorsell, S., Dorough, D. S., MacDonald, J. P., Rai, T. B., et al. (2023). Analysing engagement with indigenous peoples in the intergovernmental panel on climate Change’s sixth assessment report. NPJ Clim. Action 2:29. doi: 10.1038/s44168-023-00048-3
Carroll, D., Menemenlis, D., Adkins, J. F., Bowman, K. W., Brix, H., Dutkiewicz, S., et al. (2020). The ECCO-Darwin data-assimilative global ocean biogeochemistry model: estimates of seasonal to multidecadal surface ocean pCO2 and air-sea CO2 flux. J. Adv. Modeling Earth Syst. 12:e2019MS001888. doi: 10.1029/2019MS001888
Carroll, D., Menemenlis, D., Dutkiewicz, S., Lauderdale, J. M., Adkins, J. F., Bowman, K. W., et al. (2022). Attribution of space-time variability in global-ocean dissolved inorganic carbon. Glob. Biogeochem. Cycles 36:e2021GB007162. doi: 10.1029/2021GB007162
Cavan, E. L., Laurenceau-Cornec, E. C., Bressac, M., and Boyd, P. W. (2019). Exploring the ecology of the mesopelagic biological pump. Prog. Oceanogr. 176:102125. doi: 10.1016/j.pocean.2019.102125
Clark, M. R., Althaus, F., Schlacher, T. A., Williams, A., Bowden, D. A., and Rowden, A. A. (2016). The impacts of deep-sea fisheries on benthic communities: a review. ICES J. Mar. Sci. 73, i51–i69. doi: 10.1093/icesjms/fsv123
Crisp, D., Dolman, H., Tanhua, T., McKinley, G. A., Hauck, J., Bastos, A., et al. (2022). How well do we understand the land-ocean-atmosphere carbon cycle? Rev. Geophys. 60:e2021RG000736. doi: 10.1029/2021RG000736
Danabasoglu, G., Castruccio, F. S., Small, R. J., Tomas, R., Frajka-Williams, E., and Lankhorst, M. (2021). Revisiting AMOC transport estimates from observations and models. Geophys. Res. Lett. 48:e2021GL093045. doi: 10.1029/2021GL093045
Danabasoglu, G., Yeager, S. G., Bailey, D., Behrens, E., Bentsen, M., Bi, D., et al. (2014). North Atlantic simulations in Coordinated Ocean-ice reference experiments phase II (CORE-II). Part I: mean states. Ocean Modeling 73, 76–107. doi: 10.1016/j.ocemod.2013.10.005
Danabasoglu, G., Yeager, S. G., Kim, W. M., Behrens, E., Bentsen, M., Bi, D., et al. (2016). North Atlantic simulations in Coordinated Ocean-ice reference experiments phase II (CORE-II). Part II: inter-annual to decadal variability. Ocean Modeling 97, 65–90. doi: 10.1016/j.ocemod.2015.11.007
Davison, P. C., Checkley, D. M., Koslow, J. A., and Barlow, J. (2013). Carbon export mediated by mesopelagic fishes in the northeast Pacific Ocean. Progress Oceanogr. 116, 14–30. doi: 10.1016/j.pocean.2013.05.013
De Vos, A., Cambronero-Solano, S., Mangubhai, S., Nefdt, L., Woodall, L. C., and Stefanoudis, P. V. (2023). Towards equity and justice in ocean sciences. NPJ Ocean Sustain. 2:25. doi: 10.1038/s44183-023-00028-4
Deike, L., and Melville, W. K. (2018). Gas transfer by breaking waves. Geophys. Res. Lett. 45, 10482–10492. doi: 10.1029/2018GL078758
Dominguez-Carrió, C., Fontes, J., and Morato, T. (2021). A cost-effective video system for a rapid appraisal of deep-sea benthic habitats: The Azor drift-cam. Methods Ecol Evol. 12, 1379–1388. doi: 10.1111/2041-210X.13617
DOSI (2018). Deep-sea fundamentals. Available at: https://dosi-project.org/wp-content/uploads/2018/05/009-Policy-Brief-3-Deep-sea-Fundamentals-DOSI-V3.pdf (Accessed March 1 2024).
Drake, H. F., Bailey, S., Dussin, R., Griffies, S. M., Krasting, J. P., MacGilchrist, G. A., et al. (2024). Water mass transformation budgets in finite-volume generalized vertical coordinate ocean models. JAMES.
Drake, H. F., Rivest, R. L., Edelman, A., and Deutch, J. (2021). A simple model for assessing climate control trade-offs and responding to unanticipated climate outcomes. Environ. Res. Lett. 16:104012. doi: 10.1088/1748-9326/ac243e
Drazen, J. C., Smith, C. R., Gjerde, K. M., Haddock, S. H. D., Carter, G. S., Choy, C. A., et al. (2020). Opinion: midwater ecosystems must be considered when evaluating environmental risks of deep-sea mining. PNAS 117, 17455–17460. doi: 10.1073/pnas.2011914117
Durden, J. M., Bett, B. J., Horton, T., Serpell-Stevens, A., Morris, K. J., Billett, D. S., et al. (2016). Improving the estimation of deep-sea megabenthos biomass: dimension to wet weight conversions for abyssal invertebrates. Mar. Ecol. Prog. Ser. 552, 71–79. doi: 10.3354/meps11769
Fangohr, S., and Woolf, D. K. (2007). Application of new parameterizations of gas transfer velocity and their impact on regional and global marine CO2 budgets. J. Mar. Syst. 66, 195–203. doi: 10.1016/j.jmarsys.2006.01.012
FAO (2019). “Deep-ocean climate change impacts on habitat, fish and fisheries,” in FAO fisheries and aquaculture technical paper no. 638. eds. L. Levin, M. Baker, and A. Thompson (Rome: FAO). 186 pp. Licence: CC BY-NC-SA 3.0 IGO.
Figuerola, B., Hancock, A. M., Bax, N., Cummings, V. J., Downey, R., Griffiths, H. J., et al. (2021). A review and meta-analysis of potential impacts of ocean acidification on marine calcifiers from the Southern ocean. Front. Mar. Sci. 8:584445. doi: 10.3389/fmars.2021.584445
Fjeld, K., Tiller, R., Grimaldo, E., Grimsmo, L., and Standal, I.-B. (2023). Mesopelagics–New gold rush or castle in the sky? Mar. Policy 147:105359. doi: 10.1016/j.marpol.2022.105359
Folkersen, M. V., Fleming, C. M., and Hasan, S. (2018). The economic value of the deep sea: a systematic review and meta-analysis. Mar. Policy 94, 71–80. doi: 10.1016/j.marpol.2018.05.003
Frajka-Williams, E., Lankhorst, M., Koelling, J., and Send, U. (2018). Coherent circulation changes in the deep North Atlantic from 16°N and 26°N transport arrays. J. Geophys. Res. 123, 3427–3443. doi: 10.1029/2018JC013949
Freilich, M. A., Poirier, C., Dever, M., Alou-Font, E., Allen, J., Cabornero, A., et al. (2024). 3D intrusions transport active surface microbial assemblages to the dark ocean. Proc. Natl. Acad. Sci. 121:e2319937121. doi: 10.1073/pnas.2319937121
Friedlingstein, P., O’Sullivan, M., Jones, M. W., Andrew, R. M., Bakker, D. C. E., Hauck, J., et al. (2023). Global carbon budget 2023. Earth Syst. Sci. Data 15, 5301–5369. doi: 10.5194/essd-15-5301-2023
Gasparin, F., Hamon, M., Remy, E., and Le Traon, P.-Y. (2020). How deep Argo will improve the Deep Ocean in an ocean reanalysis. J. Clim. 33, 77–94. doi: 10.1175/JCLI-D-19-0208.1
Gatto, A., Sadik-Zada, E., Ozbek, S., Kieu, H., and Huynh, N. (2023). Deep-sea fisheries as resilient bioeconomic systems for food and nutrition security and sustainable development. Resour. Conserv. Recycl. 197:106907. doi: 10.1016/j.resconrec.2023.106907
Gebbie, G., and Huybers, P. (2012). The mean age of ocean waters inferred from radiocarbon observations: sensitivity to surface sources and accounting for mixing histories. J. Phys. Oceanogr. 42, 291–305. doi: 10.1175/JPO-D-11-043.1
Gloege, L., McKinley, G. A., Landschützer, P., Fay, A. R., Frölicher, T. L., Fyfe, J. C., et al. (2021). Quantifying errors in observationally based estimates of ocean carbon sink variability. Glob. Biogeochem. Cycles 35:e2020GB006788. doi: 10.1029/2020GB006788
Gollner, S., Kaiser, S., Menzel, L., Jones, D. O. B., Brown, A., Mestre, N. C., et al. (2017). Resilience of benthic deep-sea fauna to mining activities. Mar. Environ. Res. 129, 76–101. doi: 10.1016/j.marenvres.2017.04.010
Gregoire, M., Garcon, V., Garcia, H., Breitburg, D., Isensee, K., Oschlies, A., et al. (2021). A global ocean oxygen database and atlas for assessing and predicting deoxygenation and ocean health in the open and coastal ocean. Front. Mar. Sci. 8:724913. doi: 10.3389/fmars.2021.724913
Gruber, N., Clement, D., Carter, B. R., Feely, R. A., van Heuven, S., Hoppema, M., et al. (2019a). The oceanic sink for anthropogenic CO2 from 1994 to 2007. Science 363, 1193–1199. doi: 10.1126/science.aau5153
Gruber, N., Landschutzer, P., and Lovenduski, N. (2019b). The variable Southern Ocean carbon sink. Ann. Rev. Marine Sci. 11, 159–186. doi: 10.1146/annurev-marine-121916-063407
Gupta, M., Follows, M. J., and Lauderdale, J. M. (2020). The effect of Antarctic Sea ice on Southern Ocean carbon outgassing: capping versus light attenuation. Glob. Biogeochem. Cycles 34:e2019GB006489. doi: 10.1029/2019GB006489
Hamlington, B. D., Gardner, A. S., Ivins, E., Lenaerts, J. T. M., Reager, J. T., Trossman, D. S., et al. (2020). Understanding of contemporary regional sea-level change and the implications for the future. Rev. Geophys. 58:e2019RG000672. doi: 10.1029/2019RG000672
Harden-Davies, H. (2017). Deep-sea genetic resources: new frontiers for science and stewardship in areas beyond national jurisdiction. Deep-Sea Res. Part 137, 504–513. doi: 10.1016/j.dsr2.2016.05.005
Hartman, S. E., Bett, B. J., Durden, J. M., Henson, S. A., Iversen, M., Jeffreys, R. M., et al. (2021). Enduring science: three decades of observing the Northeast Atlantic from the porcupine abyssal plain sustained observatory (PAP-SO). Prog. Oceanogr. 191:102508. doi: 10.1016/j.pocean.2020.102508
Henson, S. A., Sanders, R., and Madsen, E. (2012). Global patterns in efficiency of particulate organic carbon export and transfer to the deep ocean, Global Biogeochem. Cycles, 26:GB1028. doi: 10.1029/2011GB004099
Hoegh-Guldberg, O., Cai, R., Poloczanska, E., Brewer, P., Sundby, S., Hilmi, K., et al. (2014). “The ocean” in Climate change 2014: impacts, adaptation, and vulnerability. Part B: regional aspects. contribution of working group ii to the fifth assessment report of the intergovernmental panel on climate change. eds. V. Barros, C. Field, D. Dokken, M. Mastrandrea, K. Mach, and T. Bilir (Cambridge: Cambridge University Press), 1655–1731.
Howell, K. L., Hilário, A., Allcock, A. L., Bailey, D. M., Baker, M., Clark, M. R., et al. (2020). A blueprint for an inclusive, global deep-sea ocean decade field program. Front. Mar. Sci. 7:999. doi: 10.3389/fmars.2020.584861
IPBES (2019). Global assessment report on biodiversity and ecosystem services of the Intergovernmental Science-Policy Platform on Biodiversity and Ecosystem Services. eds. E. S. Brondizio, J. Settele, S. Díaz, and H. T. Ngo (Bonn, Germany: IPBES secretariat), 1148. doi: 10.5281/zenodo.3831673
IPCC (2018). Global Warming of 1.5°C. An IPCC Special Report on the impacts of global warming of 1.5°C above pre-industrial levels and related global greenhouse gas emission pathways, in the context of strengthening the global response to the threat of climate change, sustainable development, and efforts to eradicate poverty. Cambridge: Cambridge University Press.
IPCC (2019). IPCC special report on the ocean and cryosphere in a changing climate. Cambridge: Cambridge University Press.
IPCC (2021a). Climate change 2021: The physical science basis. Contribution of working group I to the sixth assessment report of the intergovernmental panel on climate change. Cambridge: Cambridge University Press.
IPCC (2021b). Climate change 2023: synthesis report. Contribution of working groups I, II and III to the sixth assessment report of the intergovernmental panel on climate change. Geneva: IPCC.
IPCC (2021c). Climate change and land: an IPCC special report on climate change, desertification, land degradation, sustainable land management, food security, and greenhouse gas fluxes in terrestrial ecosystems. Cambridge: Cambridge University Press.
IPCC (2022a). Climate change 2022: impacts, adaptation and vulnerability. Contribution of working group II to the sixth assessment report of the intergovernmental panel on climate change. Cambridge: Cambridge University Press.
IPCC (2022b). Climate change 2022: mitigation of climate change. Contribution of working group III to the sixth assessment report of the intergovernmental panel on climate change. Cambridge: Cambridge University Press.
Irigoien, X., Klevjer, T. A., Røstad, A., Martinez, U., Boyra, G., Acuña, J. L., et al. (2014). Large mesopelagic fishes biomass and trophic efficiency in the open ocean. Nat. Commun. 5:3271. doi: 10.1038/ncomms4271
Jackson, L. C., Hewitt, H. T., Bruciaferri, D., Calvert, D., Graham, T., Guiavarch, C., et al. (2023). Challenges simulating the amoc in climate models. Philos. Trans. R. Soc. A Math. Phys. Eng. Sci. 381:20220187. doi: 10.1098/rsta.2022.0187
Janzwood, S. (2020). Confident, likely, or both? The implementation of the uncertainty language framework in the IPCC special reports. Clim. Chang. 162, 1655–1675. doi: 10.1007/s10584-020-02746-x
Johnson, G., Lyman, J. M., and Purkey, S. G. (2015). Informing deep Argo Array design using Argo and full-depth hydrographic section data. J. Atmos. Ocean. Technol. 32, 2187–2198. doi: 10.1175/JTECH-D-15-0139.1
Jones, D. O., Yool, A., Wei, C. L., Henson, S. A., Ruhl, H. A., Watson, R. A., et al. (2014). Global reductions in seafloor biomass in response to climate change. Glob. Chang. Biol. 20, 1861–1872. doi: 10.1111/gcb.12480
Kaartvedt, S., Staby, A., and Aksnes, D. L. (2012). Efficient trawl avoidance by mesopelagic fishes causes large underestimation of their biomass. Mar. Ecol. Prog. Ser. 456, 1–6. doi: 10.3354/meps09785
Khatiwala, S., Primeau, F., and Hall, T. (2009). Reconstruction of the history of anthropogenic CO2 concentrations in the ocean. Nature 462, 346–349. doi: 10.1038/nature08526
Khatiwala, S., Tanhua, T., Mikaloff Fletcher, S., Gerber, M., Doney, S. C., Graven, H. D., et al. (2013). Global ocean storage of anthropogenic carbon. Biogeosciences 10, 2169–2191. doi: 10.5194/bg-10-2169-2013
Klevjer, T. A., Irigoien, X., Røstad, A., Fraile-Nuez, E., Benítez-Barrios, V. M., and Kaartvedt, S. (2016). Large scale patterns in vertical distribution and behaviour of mesopelagic scattering layers. Sci. Rep. 6:19873.
Lauderdale, J. M., and Cael, B. B. (2021). Impact of remineralization profile shape on the air-sea carbon balance. Geophys. Res. Lett 48:e2020GL091746. doi: 10.1029/2020GL091746
Lauvset, S. K., Carter, B. R., Perez, F. F., Jiang, L.-Q., Feely, R. A., Velo, A., et al. (2020). Processes driving global interior ocean pH distribution. Glob. Biogeochem. Cycles 34:e2019GB006229. doi: 10.1029/2019GB006229
Larkin, K., Ruhl, H. A., Bagley, P., Benn, A., Bett, B. J., Billet, D. S. M., et al. (2010). Benthic biology time-series in the deep sea: Indicators of change, OceanObs’09 - Community White Paper. Available at: http://www.oceanobs09.net/
Lenton, A., Matear, R. J., and Tilbrook, B. (2006). Design of an observational strategy for quantifying the Southern ocean uptake of CO2. Glob. Biogeochem. Cycles 20:2620. doi: 10.1029/2005GB002620
Levin, L. (2021). IPCC and the deep sea: a case for deeper knowledge. Front. Clim. 3:720755. doi: 10.3389/fclim.2021.720755
Levin, L., Alfaro-Lucas, J., Colaco, A., Cordes, E., Craik, N., Danovaro, R., et al. (2023). Deep-sea impacts of climate interventions. Science 379, 978–981. doi: 10.1126/science.ade7521
Levin, L. A., Wei, C.-L., Dunn, D. C., Amon, D. J., Ashford, O. S., Cheung, W. W. L., et al. (2020). Climate change considerations are fundamental to management of deep-sea resource extraction. Glob. Chang. Biol. 26, 4664–4678. doi: 10.1111/gcb.15223
Llort, J., Langlais, C., Matear, R., Moreau, S., Lenton, A., and Strutton, P. G. (2018). Evaluating southern ocean carbon eddy-pump from biogeochemical Argo floats. J. Geophys. Res. Oceans 123, 971–984. doi: 10.1002/2017JC012861
Longhurst, A. R., Bedo, A. W., Harrison, W. G., Head, E. J. H., and Sameoto, D. D. (1990). Vertical flux of respiratory carbon by oceanic diel migrant biota. Deep Sea Res. Part A 37, 685–694. doi: 10.1016/0198-0149(90)90098-G
Lutz, S., Pearson, H., Vatter, J., Bhakta, D., Freeman, P., and Field, C. (2018). Oceanic blue carbon - how marine life can help to combat climate change. Available at: https://www.grida.no/publications/417
Ma, D., Gregor, L., and Gruber, N. (2023). Four decades of trends and drivers of global surface ocean acidification. Glob. Biogeochem. Cycles 37:e2023GB007765. doi: 10.1029/2023GB007765
Mach, K. J., Mastrandrea, M. D., Freeman, P. T., and Field, C. B. (2017). Unleashing expert judgment in assessment. Glob. Environ. Chang. 44, 1–14. doi: 10.1016/j.gloenvcha.2017.02.005
Majkut, J., Carter, B., Frolicher, T., Dufour, C., Rodgers, K., and Sarmiento, J. (2014). An observing system simulation for southern ocean carbon dioxide uptake. Phil. Trans. R. Soc. A 372:20130046. doi: 10.1098/rsta.2013.0046
Martin, J. H., Knauer, G. A., Karl, D. M., and Broenkow, W. W. (1987). VERTEX: carbon cycling in the northeast Pacific. Deep Sea Research Part A. Pap 34, 267–285.
Martin, W., Baross, J., Kelley, D., and Russell, M. (2008). Hydrothermal vents and the origin of life. Nat. Rev. Microbiol. 6, 805–814. doi: 10.1038/nrmicro1991
Mastrandrea, M., Field, C., Stocker, T., Edenhofer, O., Ebi, K., Frame, D., et al. (2010). Guidance note for lead authors of the IPCC fifth assessment report on consistent treatment of uncertainties. Geneva: Intergovernmental Panel on Climate Change (IPCC).
Maxwell, K., Eisenhauer, E., and Lustig, A. (2022). Toward Coequality of the social sciences in the National Climate Assessment. Weather Climate, and Society. 14, 1217–1229. doi: 10.1175/WCAS-D-21-0157.1
Mayer, L., Jakobsson, M., Allen, G., Dorschel, B., Falconer, R., Ferrini, V., et al. (2018). The Nippon foundation—GEBCO seabed 2030 project: the quest to see the World’s oceans completely mapped by 2030. Geosciences 8:63. doi: 10.3390/geosciences8020063
McMonagle, H., Llopiz, J. K., Hilborn, R., and Essington, T. E. (2023). High uncertainty in fish bioenergetics impedes precision of fish-mediated carbon transport estimates into the ocean’s twilight zone. Prog. Oceanogr 217:103078. doi: 10.1016/j.pocean.2023.103078
Mengerink, K., Van Dover, C., Ardron, J., Baker, M., Escobar-Briones, E., Gjerde, K., et al. (2014). A call for deep-ocean stewardship. Science 344, 696–698. doi: 10.1126/science.1251458
Messié, M., and Sherlock, R E., Huffard, C.L., and Pennington, J.T., Choy, C.A., Michisaki, R.P, et al. (2023). Coastal upwelling drives ecosystem temporal variability from the surface to the abyssal seafloor. Proceedings of the National Academy of Sciences, 120:e2214567120. doi: 10.1073/pnas.2214567120
Mora, C., Tittensor, D., Adl, S., Simpson, A., and Worm, B. (2011). How many species are there on earth and in the ocean? PLoS Biol. 9:e1001127. doi: 10.1371/journal.pbio.1001127
Moss, R., and Schneider, S. (2000). Uncertainties in the IPCC TAR: Recommendations to lead authors for more consistent assessment and reporting. Geneva: World Meteorological Organization, 33–51.
Muller, J., Gruber, N., Carter, B., Feely, R., Ishii, M., Lange, N., et al. (2023). Decadal trends in the oceanic storage of anthropogenic carbon from 1994 to 2014. AGU Adv. 4:e2023AV000875. doi: 10.1029/2023AV000875
NASEM (2022). A research strategy for ocean-based carbon dioxide removal and sequestration. Washington, DC: National Academies of Sciences, Engineering, and Medicine.
Nguyen, A. T., Pillar, H., Ocana, V., Bigdeli, A., Smith, T. A., and Heimbach, P. (2021). The Arctic subpolar gyre state estimate: description and assessment of a data-constrained, dynamically consistent ocean sea ice estimate for 2002–2017. J. Adv. Modeling Earth Syst. 13:e2020MS002398. doi: 10.1029/2020MS002398
Nickford, S., Palter, J. B., Donohue, K., Fassbender, A. J., Gray, A. R., Long, J., et al. (2022). Autonomous wintertime observations of air-sea exchange in the Gulf stream reveal a perfect storm for ocean CO2 uptake. Geophys. Res. Lett. 49:e2021GL096805. doi: 10.1029/2021GL096805
Omand, M. M., D’Asaro, E. A., Lee, C. M., Perry, M. J., Briggs, N., Cetinić, I., et al. (2015). Eddy-driven subduction exports particulate organic carbon from the spring bloom. Science 348, 222–225. doi: 10.1126/science.1260062
Orr, J. C., Najjar, R. G., Aumont, O., Bopp, L., Bullister, J. L., Danabasoglu, G., et al. (2017). Biogeochemical protocols and diagnostics for the CMIP6 ocean model intercomparison project (OMIP). Geosci. Model Dev. 10, 2169–2199. doi: 10.5194/gmd-10-2169-2017
Oschlies, A., Brandt, P., Stramma, L., and Schmidtko, S. (2018). Drivers and mechanisms of ocean deoxygenation. Nat. Geosci. 11, 467–473. doi: 10.1038/s41561-018-0152-2
Ottaviani, D. (2020). economic value of ecosystem services from the deep seas and the areas beyond national juristiction, FAO fisheries and aquaculture circular no. 1210. Rome: FAO.
Proud, R., Handegard, N. O., Kloser, R. J., Cox, M. J., and Brierley, A. S. (2019). From siphonophores to deep scattering layers: uncertainty ranges for the estimation of global mesopelagic fish biomass. ICES J. Mar. Sci. 76, 718–733. doi: 10.1093/icesjms/fsy037
Racape, V., Thierry, V., Mercier, H., and Cabanes, C. (2019). Isow spreading and mixing as revealed by´ deep-Argo floats launched in the charlie-gibbs fracture zone. J. Geophys. Res. Oceans 124, 6787–6808. doi: 10.1029/2019JC015040
Ramirez-Llodra, E., Brandt, A., Danovaro, R., De Mol, B., Escobar, E., German, C. R., et al. (2010). Deep, diverse and definitely different: unique attributes of the world’s largest ecosystem. Biogeosciences 7, 2851–2899. doi: 10.5194/bg-7-2851-2010
Reichl, B. G., and Deike, L. (2020). Contribution of sea-state dependent bubbles to air-sea carbon dioxide fluxes. Geophys. Res. Lett. 47:e2020GL087267. doi: 10.1029/2020GL087267
Roberts, M. J., Jackson, L. C., Roberts, C. D., Meccia, V., Docquier, D., Koenigk, T., et al. (2020). Sensitivity of the Atlantic meridional overturning circulation to model resolution in CMIP6 HighResMIP simulations and implications for future changes. J. Adv. Model. Earth Syst. 12:e2019MS002014. doi: 10.1029/2019MS002014
Roemmich, D., Alford, M. H., Claustre, H., Johnson, K., King, B., Moum, J., et al. (2019). On the future of Argo: a global, full-depth, multi-disciplinary Array. Front. Mar. Sci. 6:439. doi: 10.3389/fmars.2019.00439
Rogers, A. D., Appiah-Madson, H., Ardron, J. A., Bax, N. J., Bhadury, P., Brandt, A., et al. (2023). Accelerating ocean species discovery and laying the foundations for the future of marine biodiversity research and monitoring. Front. Mar. Sci. 10:1224471. doi: 10.3389/fmars.2023.1224471
Rose, K. C., Ferrer, E., Carpenter, S., Crowe, S., Donelan, S., Garcon, V., et al. (2024). Consideration of aquatic deoxygenation as a planetary boundary and key regulator of earth system. Nat. Ecol. Evol. 15, 1–7. doi: 10.1038/s41559-024-02448-y
Ruhl, H., and Smith, K. (2004). Shifts in deep-sea community structure linked to climate and food supply. Science 305, 513–515. doi: 10.1126/science.1099759
Saba, G. K., Burd, A. B., Dunne, J. P., Hernández-León, S., Martin, A. H., Rose, K. A., et al. (2021). Toward a better understanding of fish-based contribution to ocean carbon flux. Limnol. Oceanogr. 66, 1639–1664. doi: 10.1002/lno.11709
Sabine, C. L., Feely, R. A., Gruber, N., Key, R. M., Lee, K., Bullister, J. L., et al. (2004). The oceanic sink for anthropogenic CO2. Science 305, 367–371. doi: 10.1126/science.1097403
Sarmiento, J., Slater, R., Dunne, J., Gnanadesikan, A., and Hiscock, M. (2010). Efficiency of small scale carbon mitigation by patch iron fertilization. Biogeosciences 7, 3593–3624. doi: 10.5194/bg-7-3593-2010
Sayre, R. G., Wright, D. J., Breyer, S. P., Butler, K. A., Van Graafeiland, K., Costello, M. J., et al. (2017). A three-dimensional mapping of the ocean based on environmental data. Oceanography 30, 90–103. doi: 10.5670/oceanog.2017.116
Smith, K. L., Baldwin, R. J., Ruhl, H. A., Kahru, M., Mitchell, B. G., and Kaufmann, R. S. (2006). Climate effect on food supply to depths greater than 4,000 meters in the Northeast Pacific. Limnol. Oceanogr. 51, 166–176. doi: 10.4319/lo.2006.51.1.0166
Smith, L., Cimoli, L., LaScala-Gruenewald, D., Pachiadaki, M., Phillips, B., Pillar, H., et al. (2023). The deep ocean observing strategy: addressing global challenges in the deep sea through collaboration. Mar. Technol. Soc. J. 56, 50–66. doi: 10.4031/MTSJ.56.3.11
Smith, K., Ruhl, H., Kahru, M., Huffard, C., and Sherman, A. (2009). Climate, carbon cycling, and deep-ocean ecosystems. Proc. Natl. Acad. Sci. U. S. A 106, 19211–19218. doi: 10.1073/pnas.0908322106
Smith, K., Ruhl, H., Kahru, M., Huffard, C., and Sherman, A. (2013). Deep ocean communities impacted by changing climate over 24 y in the abyssal Northeast Pacific Ocean. Proc. Natl. Acad. Sci. U. S. A 110, 19838–19841. doi: 10.1073/pnas.1315447110
Snelgrove, P. V. R., and Smith, C. R. (2002). A riot of species in an environmental calm; the paradox of the species-rich deep sea. Oceanogr. Mar. Biol. Ann. Rev. 40, 311–342.
Stramma, L., Schmidt, S., Levin, L., and Johnson, G. (2010). Ocean oxygen minima expansions and their biological impacts. Deep-Sea Res. 57, 587–595. doi: 10.1016/j.dsr.2010.01.005
Subramanian, A., Ummenhofer, C., Giannini, A., Holland, M., Legg, S., Mahadevan, A., et al. (2016). Translating process understanding to improve climate models. Boulder, CO: US CLIVAR.
Sweetman, A. K., Thurber, A., Smith, C., Levin, L., Mora, C., Wei, C., et al. (2018). Major impacts of climate change on deep-sea benthic ecosystems. Elementa Sci. Anthropocene 5, 1–23. doi: 10.1525/elementa.203
Tamsitt, V., Drake, H., Morrison, A., Talley, L., Dufour, C., Gray, A., et al. (2017). Spiraling pathways of global deep waters to the surface of the Southern Ocean. Nat. Commun. 8:172. doi: 10.1038/s41467-017-00197-0
Tandon, A. (2023). Analysis: how the diversity of IPCC authors has changed over three decades. Carbon brief. Available at: https://www.carbonbrief.org/analysis-how-the-diversity-of-ipcc-authors-has-changed-over-three-decades/ (Accessed March 15, 2023).
Thomsen, P. F., and Willerslev, E. (2015). Environmental DNA - an emerging tool in conservation for monitoring past and present biodiversity. Biol. Conserv. 183, 4–18. doi: 10.1016/j.biocon.2014.11.019
Turner, P. J., Thaler, A. D., Freitag, A., and Collins, P. C. (2019). Deep-sea hydrothermal vent ecosystem principles: identification of ecosystem processes, services and communication of value. Mar. Policy 101, 118–124. doi: 10.1016/j.marpol.2019.01.003
Usbeck, R., Schlitzer, R., Fischer, G., and Wefer, G. (2003). Particle fluxes in the ocean: comparison of sediment trap data with results from inverse modeling. J. Mar. Syst. 39, 167–183. doi: 10.1016/S0924-7963(03)00029-0
Von Schuckmann, K., Miniere, A., Gues, F., Cuesta-Valero, F. J., Kirchengast, G., Adusumilli, S., et al. (2023). Heat stored in the earth system 1960–2020: where does the energy go? Earth Syst. Sci. Data 15, 1675–1709. doi: 10.5194/essd-15-1675-2023
Wassénius, E., and Crona, B. I. (2022). Adapting risk assessments for a complex future. One Earth 5, 35–43. doi: 10.1016/j.oneear.2021.12.004
Weijer, W., Cheng, W., Garuba, O. A., Hu, A., and Nadiga, B. T. (2020). CMIP6 models predict significant 21st century decline of the Atlantic meridional overturning circulation. Geophys. Res. Lett. 47:e2019GL086075. doi: 10.1029/2019GL086075
Williams, N. L., Juranek, L. W., Feely, R. A., Johnson, K. S., Sarmiento, J. L., Talley, L. D., et al. (2017). Calculating surface ocean pCO2 from biogeochemical Argo floats equipped with pH: an uncertainty analysis. Glob. Biogeochem. Cycles 31, 591–604. doi: 10.1002/2016GB005541
Wilson, J. D., Barker, S., Edwards, N. R., Holden, P. B., and Ridgwell, A. (2019). Sensitivity of atmospheric CO_2 to regional variability in particulate organic matter remineralization depths. Biogeosciences 16, 2923–2936. doi: 10.5194/bg-16-2923-2019
Woolf, D. K. (2005). Parameterization of gas transfer velocities and sea-state-dependent wave breaking. Tellus 57, 87–94. doi: 10.3402/tellusb.v57i2.16783
Wunsch, C., and Heimbach, P. (2012). Two decades of the Atlantic meridional overturning circulation: anatomy, variations, extremes, prediction, and overcoming its limitations. J. Clim. 26, 7167–7186. doi: 10.1175/JCLI-D-12-00478.1
Yang, N., Jin, D., and Govindarajan, A. F. (2024). Applying environmental DNA approaches to inform marine biodiversity conservation: the case of the ocean twilight zone. Mar. Policy 165:106151. doi: 10.1016/j.marpol.2024.106151
Yool, A., Martin, A. P., Anderson, T. R., Bett, B. J., Jones, D. O., and Ruhl, H. A. (2017). Big in the benthos: future change of seafloor community biomass in a global, body size-resolved model. Glob. Chang. Biol. 23, 3554–3566. doi: 10.1111/gcb.13680
Youngs, M. K., and Flierl, G. R. (2023). Extending residual-mean overturning theory to the topographically localized transport in the Southern Ocean. J. Phys. Oceanogr. 53, 1901–1915. doi: 10.1175/JPO-D-22-0217.1
Yung, C. K., Morrison, A. K., and Hogg, A. M. (2022). Topographic hotspots of Southern Ocean Eddy upwelling. Front. Marine Res. 9:855785. doi: 10.3389/fmars.2022.855785
Zakem, E. J., Lauderdale, J. M., Schlitzer, R., and Follows, M. J. (2021). A flux-based threshold for anaerobic activity in the ocean. Geophys. Res. Lett. 48:e2020GL090423. doi: 10.1029/2020GL090423
Zakem, E. J., Mahadevan, A., Lauderdale, J. M., and Follows, M. J. (2020). Stable aerobic and anaerobic coexistence in anoxic marine zones. ISME J. 14, 288–301. doi: 10.1038/s41396-019-0523-8
Keywords: deep sea, climate science, evidence-based decision making, IPCC, uncertainty, vulnerability and risk
Citation: Pillar HR, Hetherington E, Levin LA, Cimoli L, Lauderdale JM, van der Grient JMA, Johannes K, Heimbach P, Smith L, Addey CI, Annasawmy P, Antonio S, Bax N, Drake HF, Escobar E, Elsler LG, Freilich MA, Gallo ND, Girard F, Harke MJ, Jones DOB, Joshi S, Liang X, Maroni PJ, Sarti O, Stefanoudis PV, Sulpis O and Trossman D (2024) Future directions for deep ocean climate science and evidence-based decision making. Front. Clim. 6:1445694. doi: 10.3389/fclim.2024.1445694
Edited by:
Paul James Durack, Lawrence Livermore National Laboratory (DOE), United StatesReviewed by:
Detelina Ivanova, Climformatics Inc., United StatesAnna Pirani, Ca’ Foscari University of Venice, Italy
Copyright © 2024 Pillar, Hetherington, Levin, Cimoli, Lauderdale, van der Grient, Johannes, Heimbach, Smith, Addey, Annasawmy, Antonio, Bax, Drake, Escobar, Elsler, Freilich, Gallo, Girard, Harke, Jones, Joshi, Liang, Maroni, Sarti, Stefanoudis, Sulpis and Trossman. This is an open-access article distributed under the terms of the Creative Commons Attribution License (CC BY). The use, distribution or reproduction in other forums is permitted, provided the original author(s) and the copyright owner(s) are credited and that the original publication in this journal is cited, in accordance with accepted academic practice. No use, distribution or reproduction is permitted which does not comply with these terms.
*Correspondence: Helen R. Pillar, aGVsZW4ucGlsbGFyQHV0ZXhhcy5lZHU=