- Homeworld Collective, Somerville, MA, United States
Net emissions targets for the coming decades demand that new greenhouse gas removal (GHGR) technologies be developed and scaled to up to 10 GtCO2e/yr. by 2050. Due to the interdisciplinarity and novelty of GHGR technologies, GHGR research faces challenges of adapting technical disciplines to new domains and broadly empowering researchers with the knowledge necessary to identify and solve key problems. This Perspective discusses the significant, but underexplored, role biotechnology could play in several GHGR technologies as well as the common research, community, and knowledge gaps that are limiting progress. The GHGR technologies of focus are (1) the potential for the enzyme carbonic anhydrase to catalyze CO2 exchange in direct air capture; (2) the potential utility of microbes for accelerating soil-based or reactor-based enhanced rock weathering; and (3) the potential for methanotrophic bacteria or methane monooxygenase enzymes to oxidize methane for atmospheric methane removal via enhanced methanotrophy or bioreactors. Research progress on those GHGR methods is strongly limited by lack of interdisciplinary research community development as well as knowledge gaps. There is a need for clear and accessible articulation of actionable problems, ideally paired with risk-tolerant funding opportunities, as a tool for recruiting and empowering relevant researchers to these under-addressed technology areas.
1 Introduction
Achieving climate goals is predicted to require greenhouse gas removal (GHGR) up to 10 Gt-CO2e/yr. by 2050 and 20 Gt-CO2e/yr. by 2,100, using novel technologies that facilitate capture of greenhouse gases from the atmosphere and subsequent storage (National Academies of Sciences, Engineering, and Medicine, Division on Earth and Life Studies, Ocean Studies Board, Board on Chemical Sciences and Technology, Board on Earth Sciences and Resources, Board on Agriculture and Natural Resources, 2019). Today, < 3 × 10−4 GtCO2e/yr. of GHGR is implemented, the overwhelming majority of which is carbon dioxide removal (CDR) that stores carbon in low-durability forms, i.e., as soil, living biomass, or biochar expected to remain stable for <100 years (CDR, 2024). Novel GHGR technology development is therefore needed, particularly for durable methods, i.e., methods that keep the GHG out of the atmosphere for >1,000 years. To meet that need, GHGR has grown over the past decade as a new interdisciplinary field of technology research and development. The 21st century is therefore expected to be a “century of GHGR.”
The 21st century has also been heralded as a “century of biology,” anticipating a continued life sciences revolution. Biological processes mediate many of Earth’s Gt-scale elemental fluxes, suggesting strong potential for biology in GHGR. Indeed, CDR technologies based on ecosystem biomass, soil, and cultivated biomass are well-appreciated, wherein CO2 is captured from the atmosphere through photosynthesis and subsequently stored; storage is primarily in low-durability forms including the biomass of living ecosystems, soil, and biochar, as well as higher-durability forms such as CO2 that is captured from bioprocessing operations such as ethanol manufacturing and then stored geologically (Griscom et al., 2017; Paustian et al., 2019; Dees et al., 2023); however, those established methods are not explored here. Instead, this Perspective, discusses several underappreciated potential applications of biotechnology in durable GHGR and explores the gaps limiting their evaluation and development.
This Perspective begins with an overview of carbonic anhydrase (CA)-enhanced direct air capture (DAC); bio-enhanced rock weathering (bio-ERW) in soils and in reactors; and biological atmospheric methane removal (bio-MR) in reactors and through terrestrial methanotrophy enhancement (ME; Figure 1A). The next section discusses barriers to progress using a framework centered on growing an empowered technology community that conducts mutually-reinforcing research and knowledge work (Figure 1B), focusing on common gaps between the technologies. Engagement of the regulatory field during technology development is also important for ensuring that technologies are safe and can be legally deployed, particularly for engineered organisms that would be used, e.g., in soil; however, regulation is not discussed here, as the focus of this Perspective is on core technology development. This article concludes by advocating for cultivation of interdisciplinary relationships and collaborative problem-identification paired with risk-tolerant funding to enable development of GHGR technologies.
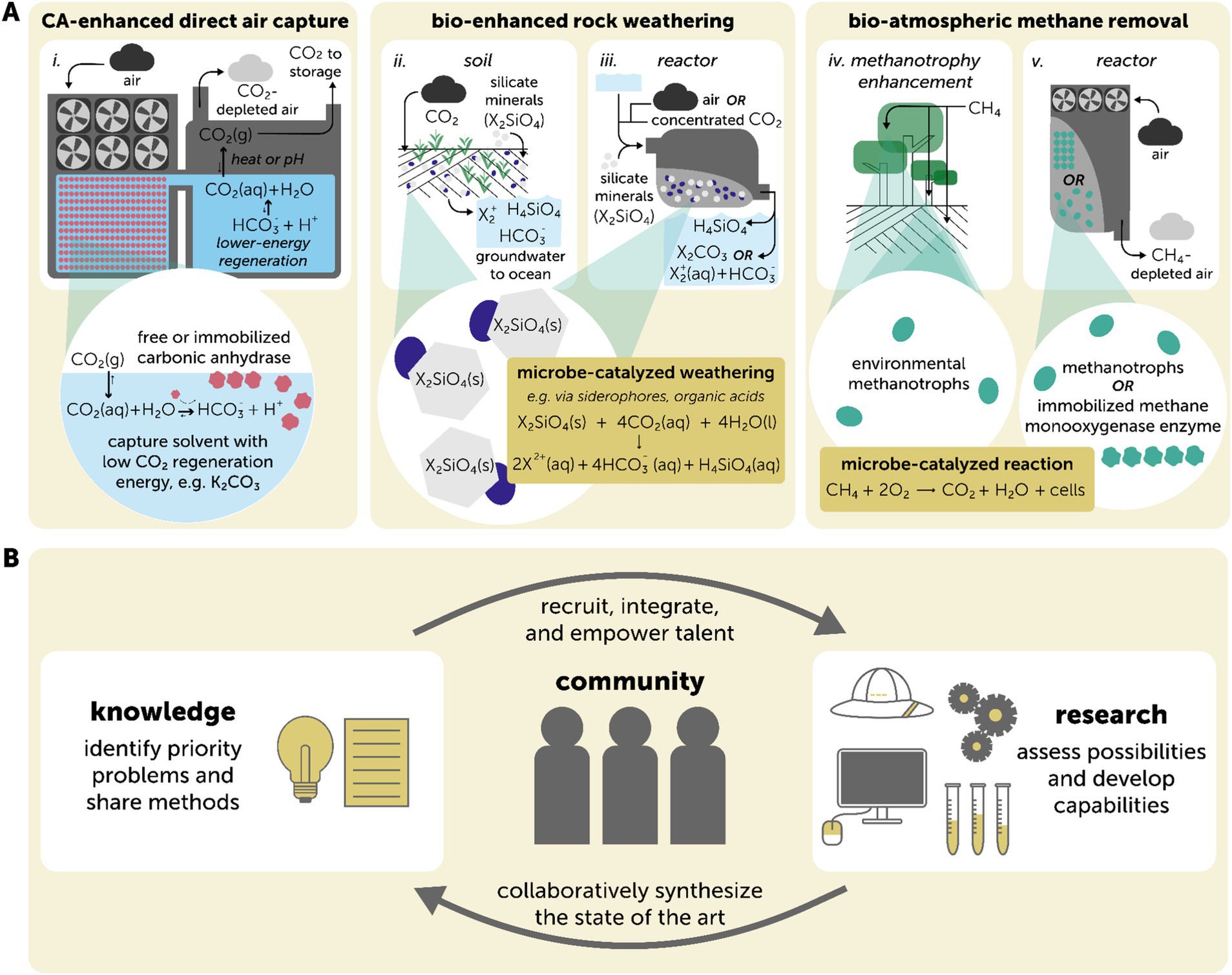
Figure 1. Overview of emerging biological GHGR technologies and productive technology development. (A) Schematics of (i) CA-enhanced DAC; bio-ERW in (ii) soils and (iii) reactors; and bio-MR via (iv) ME and (v) reactors. (B) Growing an empowered technology community that conducts mutually-reinforcing research and knowledge work.
2 Three emerging opportunities for biotech in GHGR
2.1 Carbonic anhydrase-enhanced direct air capture of CO2
DAC is a CDR technology that loads a CO2-capture material, such as an alkaline aqueous solvent, with CO2 from air and then regenerates concentrated CO2 using a heat or pH swing (Erans et al., 2022). The concentrated CO2 output can be stored with high durability, for example via subsurface geologic storage (Massarweh and Abushaikha, 2024). The current cost of DAC at scale is estimated at $100 s to $1,000/tCO2, driven primarily by the cost of a large air contactor and the energy required to regenerate CO2 (Erans et al., 2022; Young et al., 2023). It is difficult to co-optimize air contactor size and CO2 regeneration energy, because CO2 capture materials with a high absorption rate, which allow a smaller air contactor, generally interact with CO2 more strongly and thus have high regeneration energies (McQueen et al., 2021). CO2 absorption into an alkaline aqueous solvent to form (bi) carbonates proceeds by Equations 1–4, with forward and reverse rate constants indicated with positive and negative subscripts and taken from Sharifian et al. (2021):
Biological catalysts may alleviate the trade-off between absorption rate and regeneration energy. The enzyme carbonic anhydrase (CA) catalyzes CO2 (de)hydration (Equations 2, 3; Supuran, 2016), which is rate-limiting in CO2 absorption into aqueous CO2 capture solvents. Through catalysis, CA can enable fast CO2 absorption in CO2 capture solvents with low regeneration energy, like K2CO3 solutions (Salmon and House, 2015). One techno-economic analysis (TEA) indicated reductions1 in cost and regeneration energy of 31.5 and 41% by leveraging CA in point-source capture (Reardon et al., 2015). Indeed, CA has been explored for point-source CO2 capture (Bose and Satyanarayana, 2017; de Oliveira Maciel et al., 2022) and is under commercial development (Saipem and Novozymes to work together to create a more, 2021). Point-source CO2 capture technology absorbs CO2 from industrial waste gas streams, such as those from fossil fuel combustion, and is a far older and more mature technology than DAC, with demonstration at scale occurring as early as 1996 (Ma et al., 2022). This indicates a strong possibility for similar use of CA in DAC (Figure 1Ai; however, this possibility has not yet been explored in peer-reviewed literature, despite being briefly acknowledged in several review articles (Wilcox, 2012; McQueen et al., 2021; Sclarsic, 2021; Sharifian et al., 2021; Erans et al., 2022).
2.2 Bio-enhanced rock weathering
Enhanced rock weathering (ERW) technology seeks to accelerate dissolution of alkalinity-generating minerals, a natural process which already absorbs CO2 at Gt scale (Campbell et al., 2022). Primary limitations on ERW are (1) the rate of mineral dissolution, which limits the overall rate of CDR; and (2) passivation of mineral surfaces by solid metal oxides, which reduces the extent of mineral dissolution and thereby reduces the CDR achieved per unit financial and energy expenditure involved in transporting and deploying minerals (Strefler et al., 2018). In cases where minerals are ground for the purpose of ERW (as opposed to being sourced from already-ground mining and industrial wastes), the energy required to grind minerals is an additional factor contributing to overall efficiency2 (Strefler et al., 2018). Thus, ERW technology is largely concerned with exposing finely-ground minerals to conditions that maximally increase dissolution kinetics and the extent of dissolution, to maximize the rate of CDR and minimize the cost and energy demands per unit CO2 removed.
Organisms including plants, fungi and bacteria shape Earth’s mineralogy (Hazen and Ferry, 2010). Biological mechanisms can increase the rate of mineral weathering: bioweathering mechanisms include cation chelation by organic acids (Drever and Stillings, 1997) and nutrient-scavenging siderophores (Torres et al., 2014, 2019; Van Den Berghe et al., 2021); mechanically-induced cracking (Gazzè et al., 2012; Bonneville et al., 2016; Porder, 2019); oxidoreduction by chemolithotrophs (Napieralski et al., 2019); and export of acids into soil (Porder, 2019). Open questions remain regarding the overall impact and context-dependence of bioweathering mechanisms (Wild et al., 2022), though lab experiments have demonstrated nearly 10-fold weathering rate enhancement (Lunstrum et al., 2023) and little impact in others (Pokrovsky et al., 2021). While it remains unclear whether and to what extent biological activity accelerates natural mineral weathering overall (Finlay et al., 2020), the existence of such mechanisms raises the possibility of leveraging them for biologically-enhanced rock weathering (bio-ERW; Verbruggen et al., 2021; Campbell et al., 2022; Vicca et al., 2022; van den Berghe et al., 2024). Importantly, bio-ERW only affects CDR when the liberated alkalinity neutralizes acidity from CO2: while organic acids and biologically-produced sulfuric acid can accelerate mineral weathering (Drever and Stillings, 1997; Yi et al., 2021), those processes do not necessarily contribute to CDR unless residual alkalinity remains after neutralization of non-CO2 acids.
A leading ERW approach involves spreading crushed alkaline rocks on agricultural soils, where they dissolve and uptake atmospheric CO2 as dissolved (bi)carbonates (Hartmann et al., 2013; Beerling et al., 2020). Stable storage of such (bi)carbonates is hypothesized to occur via transport through groundwater to the ocean (Hartmann et al., 2013), though methods for quantifying the true permanence of carbon removal by ERW are still under development, and are complicated by the risk of CO2 off-gassing if exposed to acidic conditions during transport to the ocean (Clarkson et al., 2024).
It has been proposed that the soil microbiome and rhizosphere could be leveraged to accelerate ERW in soil, for example by managing soils to encourage growth of microbial communities with weathering activity, inoculating soils with microbes, or factoring soil biology into ERW siting (Verbruggen et al., 2021; Vicca et al., 2022; Wild et al., 2022; Figure 1Aii). To date, however, only one pre-print experimentally investigating bio-ERW for CDR in soil is known (Fuenzalida-Meriz et al., 2024); in that study, soils treated with a microbial inoculant encouragingly showed a ~ 10% increase in soil inorganic carbon, soil organic carbon, and crop yield. Further study of bio-ERW in soil is needed (Verbruggen et al., 2021; Vicca et al., 2022; Wild et al., 2022).
Bio-ERW in reactor systems has also been proposed (Corbett et al., 2024; van den Berghe et al., 2024; Figure 1Aiii). In reactors, natural or engineered microbes would accelerate reaction of minerals with atmospheric CO2 or concentrated CO2 streams from other CDR technologies and store them as solid carbonates or dissolved inorganic carbon in the ocean, possibly coupled with metal extraction (van den Berghe et al., 2024). Currently, there are no proposed designs for bio-ERW reactor systems or quantitative analyses of the feasibility of reactor-based ERW.
2.3 Biological atmospheric methane removal
Methane is a potent greenhouse gas that has contributed ~0.5°C of global warming to date (Synthesis Report, n.d.). Even if anthropogenic methane sources are reduced, natural methane sources may increase via feedback to warming (Zhang et al., 2023). Atmospheric methane removal (MR) technologies, all of which are at a conceptual stage, seek to remove methane from the atmosphere (Jackson et al., 2019, 2021; Nisbet-Jones et al., 2022; Lidstrom, 2023; Wang and He, 2023; Gorham et al., 2024). The effect of methane on radiative forcing (i.e., its warming effect) is stronger than that of CO2, though it also labile in the atmosphere (i.e., it oxidizes to CO2 through natural atmospheric processes, and thus has an atmospheric half-life); as a result, the effect of methane effect on radiative forcing is more impactful in the short term than the long term, causing 83-fold and 30-fold greater warming than CO2 over 20 and 100 years, respectively [Intergovernmental Panel on Climate Change (IPCC), 2023]. Methane removal is therefore most impactful in the short term, with the effect on warming of 1 GtCO2 removal being accomplished by 12 MtCH4 and 33 Mt. methane removal over 20 and 100 years.
One broad proposed MR approach involves reactor facilities that process air and oxidize atmospheric methane with oxygen to form CO2 by exposing it to a catalyst, similarly to a DAC air contactor (Jackson et al., 2019, 2021; Lidstrom, 2023; Wang and He, 2023). Such conversion of methane to CO2 can be fairly considered durable removal of methane from the atmosphere, owing to the substantially lower warming potential of CO2 compared to methane. At 2 ppm, atmospheric methane is ~200-fold more dilute than CO2, and its CH bonds are highly stable (438.8 kJ/mol; Sun et al., 2020). Methane oxidation reactors would require very efficient catalysts (Wang and He, 2023; Hickey and Allen, 2024). Existing engineered methane-oxidation catalysts are currently at least an order of magnitude away from efficiency thresholds which would enable net-climate-beneficial oxidation of 2 ppm methane, given the need for significant energy input as heat, light, or electricity, and a recent review stated that such catalysts are not anticipated to undergo the necessary improvements (Abernethy et al., 2023). However, methane monooxygenase enzymes in methanotrophic bacteria (Tucci and Rosenzweig, 2024) catalyze methane oxidation under ambient conditions. Biological MR (bio-MR) reactors bearing methanotrophs (Lidstrom, 2023) or immobilized cell-free have therefore been proposed. Biofilter technologies based on the same principle are in use for point-source emissions, wherein gaseous methane from point sources that are too dilute to oxide by combustion (i.e., they are below 44,000 ppm) are passed over solid supports bearing methanotrophs in the presence of oxygen (La et al., 2018). While orders-of-magnitude efficiency improvements to such reactors would be required to handle 2 ppm methane (Yoon et al., 2009; Lidstrom, 2023), recent discoveries of methanotrophs that oxidize methane below 1,000 ppm (He et al., 2023) or at atmospheric concentrations (Tveit et al., 2019; Schmider et al., 2024) may provide a path forward.
Another bio-MR approach is natural methanotrophy enhancement (ME), wherein methanotrophy in soils or trees is maximized through management practices and amendments. The soil methane sink (i.e., permanent removal of methane from the atmosphere via oxidation to form biomass or CO2 that) is estimated at 30–40 Mt. CH4/yr. (Saunois et al., 2020), and it might be enhanced in natural and agricultural settings by increasing aeration with oxygen-rich air; reducing waterlogging, which serves to increase exposure to oxygen; reducing excess ammonia, which can be an alternative substrate for methanotrophs; or applying minerals bearing micronutrients like copper that are growth-limiting for methanotrophs (Davidson et al., 2024). Very recent work has identified the woody surfaces of trees as another substantial methane sink, preliminarily estimated at 25–50 Mt. CH4/yr. (Gauci et al., 2024). Some research indicates that leaf surfaces may also be a substantial sink (Gorgolewski et al., 2023). ME in trees might be possible through forestry practices (Gauci, 2024). In all cases, care must be taken to ensure that ME does not simultaneously result in increased N2O emissions, since N2O has 10-fold greater warming potential than methane and such feedbacks have been observed (Stein and Lidstrom, 2024).
3 Discussion
CA-enhanced DAC, bio-ERW, and bio-MR are attractive GHGR possibilities, but further work is required to evaluate their potential for impact, and, if warranted, to develop and scale them. This section discusses the gaps across these technologies that must be filled to enable progress, focusing on commonalities. This discussion of gaps is anchored on research gaps, and also accounts for the community and knowledge gaps that must be filled to enable effective research (Table 1).
3.1 Research gaps
The technologies considered here are nascent, with research to date occurring predominantly at the concept phase. Therefore, the first research priority for each is to estimate its viability in theory, and then to identify and pursue promising engineering targets. Common research gaps across the technologies include proposed process designs, TEA, and LCA; use-inspired basic research; engineering research; and heterogeneity in deployment environments and feedstocks.
3.1.1 Process designs, techno-economic analyses, and life-cycle analyses
CA-enhanced DAC, reactor-based bio-ERW, and reactor-based MR, require reactors or facilities for which scalability may be limited by construction cost, materials, or energy. For those technologies, the highest research priority is the proposal of process designs and associated LCA and TEA that assess scalability and guide efforts toward engineering benchmarks to address key constraints. A challenge to producing designs, LCA, and TEA for these technologies is their need for sophisticated facilities, which highlights the importance of integrating communities familiar with related industrial process designs such as DAC, biomining, biomanufacturing, or sewage treatment, as discussed in section 3.2. A key consideration of LCA and TEA for these biological GHGR technologies should be whether the advantages conferred by incorporating biological elements outweigh the accompanying limitations; for example, cells and biocatalysts are able to tolerate a smaller range of physicochemical parameters, such as pH temperature, than abiotic technologies. Earnest comparisons to abiotic variants of each technology should be made to determine whether development of novel biological GHGR technologies is warranted.
For CA-enhanced DAC, design and modeling are needed to identify the CO2 capture and regeneration modalities and process parameter ranges (e.g., heat, pH) where CA might confer a benefit compared to abiotic DAC. Such models should consider a range of potential CA activity, overall CA-catalyzed CO2 mass transfer rates, CA stability, and CA cost, in order to identify targets for CA engineering and manufacturing that would be needed to enable competitive CA-enhanced DAC.
Similarly, design and modeling of reactor-based bio-ERW should assess what ranges of weathering rates, nutrient and metabolic efficiency per unit weathering, and energy feedstock carbon intensity could enable a system that could compete with abiotic ERW reactor approaches (van den Berghe et al., 2024).
For reactor-based bio-MR, design and modeling should assess what ranges of whole-cell specific affinity for methane (for cell-based systems), methane monooxygenase enzyme efficiency (for cell-free systems), and overall methane mass transfer rate could enable a system that is competitive with abiotic MR, given assumptions about nutrient efficiency and costs for growing microbes or manufacturing enzymes (Lidstrom, 2023; He and Lidstrom, 2024).
3.1.2 Use-inspired basic research
Bio-ERW and bio-MR approaches rely on mechanisms that are insufficiently understood for developing scaled GHGR applications, and therefore require basic research to achieve deeper understanding of known mechanisms or survey nature for new, useful mechanisms. For soil-based ERW and ME, the top priority for basic research is to illuminate mechanisms that can help to estimate the GHGR potential of each pathway.
For bio-ERW, the magnitude of bioweathering should be measured under conditions relevant to soil-based or reactor-based bio-ERW in ERW-relevant soil, and the contexts where bioweathering in soil contributes to overall increased weathering (if any) should be identified. The mechanisms, rhizosphere interactions, and microbial community functions underlying such bioweathering, as well as the abiotic factors that impact them, should also be characterized (Verbruggen et al., 2021; Vicca et al., 2022; Wild et al., 2022). In light of such fundamental research, efforts should be made to understand how mechanistic levers for bio-ERW would change at scale, and how measurement, reporting and verification (MRV) could identify the net effects of bio-ERW.
For ME in trees, the magnitudes of methane uptake should be determined in bark and leaves across geographies, and the impact of of tree species, tree age, tissue composition, methanotroph colonization rate, and limiting nutrients on such uptake should be characterized (Gauci, 2024). For ME in soils, the impact of copper and other trace micro-nutrient limitation, aeration, hydrology, and excess ammonia on methane and nitrous oxide flux magnitudes across managed soil types should be characterized (Davidson et al., 2024). For reactor-based bio-MR, efforts should be made to discover and mechanistically characterize a broader range of methanotrophs (Schmider et al., 2024), microbial community functions that support utilization of atmospheric methane, and the methane-uptake efficiency of biofilms for use in reactors (Lidstrom, 2023; He and Lidstrom, 2024).
To maximize impact for GHGR technologies, all of the basic research efforts discussed above should be scoped and pursued in close discourse with the broader effort to develop and assess the relevant GHGR methods.
3.1.3 Engineering research
All of the technologies discussed here require engineering novel industrial systems, land management practices, or research tools. CA-enhanced DAC is the only technology discussed here that is mostly an engineering challenge; the others must proceed in collaboration with use-inspired basic science research. CA-enhanced DAC research may therefore be particularly attractive for funding sources seeking to rapidly accelerate GHGR technology development in the near-term.
For CA-enhanced DAC, the engineering research needed includes protein engineering to produce CA that is stable, active, and amenable to immobilization under DAC-relevant conditions (Mesbahuddin et al., 2021); development of low-volume (<100 μL) assays for CO2 absorption rates to enable high-throughput screening of engineered CA variants; composite materials for CA immobilization at the gas-solvent interface (Molina-Fernández and Luis, 2021; Russo et al., 2022); and design of air contactors containing such materials.
All forms of bio-ERW will require novel lab platforms for rapidly measuring and screening weathering rates to facilitate basic research (Campbell et al., 2022; van den Berghe et al., 2024). Novel methods are needed for rapidly measuring bioweathering rates in soil and distinguishing them from abiotic factors (Campbell et al., 2022; Vicca et al., 2022; Wild et al., 2022). Development of soil-based bio-ERW methods could involve developing ERW siting frameworks that account for biotic factors, land management practices that maximize bioweathering, and/or microbial inoculants that increase weathering. Reactor-based bio-ERW will require development or discovery of stable microbial communities with high weathering rates in reactors, and may also require metabolic engineering in non-model organisms to enhance weathering or metal/biomass recovery.
The basic science underlying ME in trees will require development of standardized methods for gas flux measurements across tree surfaces and foliage to enable comparable measurement across the research community (Gauci, 2024). For implementation, ME methods will require forestry and/or soil management frameworks that balance methane uptake with other economic and environmental factors (Gauci, 2024; Davidson et al., 2024).
For reactor-based bio-MR, novel methods are required to enable the required underlying science and engineering, including methods for cultivating methanotrophic communities that can oxidize ultra-dilute methane; methods for rapidly screening, evolving and/or engineering such methanotrophs (Lidstrom, 2023; He and Lidstrom, 2024); and, for systems that would involve cell-free enzymes, methods for manipulating and engineering cell-free methane monooxygenase enzymes. For bio-MR technology itself, necessary engineering research includes development of air contactors that host methanotrophs and optimize methane mass transfer while minimizing energy and water usage; methods for utilizing methanotrophs under non-growth conditions [e.g., high density immobilization of cells directly onto reactor matrix; and methods for disposing (or valorizing) produced biomass or, if using cell-free methane monooxygenase, methanol].
3.1.4 Heterogeneity
Soil-based bio-ERW and ME approaches are complicated by environmental heterogeneity. For example, variations in geography, crop-type, and soil composition impact soil microbial communities (Wild et al., 2022), while geography, tree species, and tree tissue composition impact the sign and/or magnitude of methane fluxes (Gauci, 2024; Gauci et al., 2024). Understanding the impacts of these heterogeneities on GHGR magnitudes and levers will require basic science and engineering to be performed across diverse environments, demanding standardized sampling methods and tools.
Reactor-based bio-ERW will need to accommodate input materials from different sources, which will vary in mineral composition and trace metal content that could impact microbial metabolism. Engineered bio-ERW reactor systems would need to either be robust to this heterogeneity or be designed specifically for different input materials (van den Berghe et al., 2024).
3.2 Community gaps
Rapidly assessing, and if appropriate, developing, novel biological GHGR technologies will require communities empowered with the skills and collaborative relationships needed to carry out research and synthesize results into new knowledge resources. Several common community gaps must be addressed to enable effective research, including integrating relevant disciplines, orienting basic science researchers toward engineering goals, and recruiting talent from small communities of practice.
3.2.1 Integrating disciplines
The CA-enhanced DAC, bio-ERW, and reactor-based bio-MR require novel cross-disciplinary collaborations.
CA-enhanced DAC requires collaboration between protein engineering, composite biomaterials engineering, and DAC process engineering, but there has been no peer-reviewed research drawing on all three disciplines. Anecdotally, several DAC companies have explored use of CA but abandoned the idea owing to a lack of team capacity to overcome its challenges. The community surrounding point-source capture applications of CA (Bose and Satyanarayana, 2017; de Oliveira Maciel et al., 2022) provides a jumping-off point, but needs input from the most recent advances in protein engineering, which is undergoing a revolution (Wang et al., 2021; Notin et al., 2024). Skills and perspectives from the commodity chemicals biomanufacturing community should also be integrated, given their experience deploying biocatalysts in high-volume industrial processes.
For bio-ERW, the microbial weathering, metagenomics, and ERW communities must collaborate, but only a few articles to date discuss all three topics (Verbruggen et al., 2021; Vicca et al., 2022; Wild et al., 2022; van den Berghe et al., 2024), and no experimental studies addressing all three are known. The value of collaboration is particularly timely for soil-based bio-ERW: to access microbial processes in ERW-relevant conditions across heterogeneous soil environments, it would be helpful for geobiological studies to be integrated into the ERW field trials that are increasingly being performed by academic groups and companies across diverse soils and geographies. However, anecdotally, bio-ERW researchers report that bio-ERW is rarely represented or discussed at their field’s convenings. For reactor-based bio-ERW, the biomining community can provide perspective on incorporating microbes into scaled mineral processing technologies. The sewage and wastewater processing communities should also be engaged, given their experience deploying microbial technologies at scale.
For reactor-based bio-MR, methanotroph biologists and microbial physiologists, or protein engineers in the case of cell-free methane monooxygenase systems, will need to work closely with the process design and ultra-dilute gas separation communities to optimize for atmospheric or near-atmospheric methane concentrations. The community surrounding point-source methane biofilters can be a bridge, but the low methane concentrations targeted in bio-MR will likely require novel reactor formats to optimize mass transfer while minimizing energy for moving air (Lidstrom, 2023; Wang and He, 2023). For ME, microbiologists, soil scientists, botanists, and the gas flux measurement communities will need to collaborate in novel ways.
Bio-ERW and bio-MR technologies may also need input from the genomics technology community, which can help develop tools for more rapid genetic study, manipulation, and screening of non-standard organisms (He and Lidstrom, 2024b; van den Berghe et al., 2024).
3.2.2 Pure-science disciplines
Design, assessment and development of bio-ERW and bio-MR technologies require substantial inputs from the research communities of microbial mineral weathering, methanotrophy, and field-based measurement of gas flux across surfaces, which have historically focused primarily on pure descriptive science rather than technology development.
There may be a cultural inertia to overcome for researchers when shifting from an observation-first ethos to an engineering-first approach focused on assessing potential impact and scaling. It will also require recruitment of those communities to different funding sources, of which they might not currently be aware, or that must be created by funding bodies to attract and accelerate a burst of novel, fast-moving basic and translational research intended to enable GHGR technologies to be developed in order to meet society’s daunting GHGR needs.
3.2.3 Small communities
The microbial weathering and methanotroph research communities who must lead the way on bio-ERW and bio-MR are relatively small and niche, which may complicate the required shift from pure science to engineering research described in the previous section. Given the complex research activities required for GHGR technology development—which span use-inspired basic research, prospective designs, and engineering—active community engagement and support will be needed. Recruitment efforts will be required to empower the pioneering researchers who are already working on these problems; identify the best-equipped researchers, and support them to recruit and train the next generation of GHGR experts; and incentivize prioritization of new research directions. Funding from government and private entities will be required to enable those activities.
3.3 Knowledge gaps
Mature research communities have shared goals and tools, from which actionable subproblems and methods are collaboratively understood and prioritized through literature, discourse, and tacit community knowledge. Given that CA-enhanced DAC, bio-ERW, and bio-MR are nascent technologies that require contributions across novel cross-disciplinary boundaries, intentional work is required to communicate actionable problems to researchers and provide access to specialized research methods and resources to enable them to effectively apply their expertise. Funding must be made available to support creation of such knowledge resources.
3.3.1 Actionable problems
To best enable practitioners to whom GHGR technology may be new, thought leaders should collaboratively synthesize the state of the art and technology goals to identify and itemize actionable problems.
Several excellent resources exist describing goals and challenges in CA-enhanced DAC, bio-ERW (Verbruggen et al., 2021; Campbell et al., 2022; Vicca et al., 2022; van den Berghe et al., 2024), and bio-MR (Lidstrom, 2023; He and Lidstrom, 2024). Accessibility can be maximized through concise itemization of priority problems with specific calls to action, such that individual problems can be easily shared with the most relevant potential contributors. Crucially, these problems should be identified through cross-disciplinary collaborative efforts among the diverse relevant research communities, which may help reveal creative new possibilities. To be most actionable, problems would ideally be supported by a risk-tolerant funding opportunity willing to back early-stage ideas by researchers new to the field.
3.3.2 Specialized research methods
The necessary research for all the technologies discussed here requires specialized methods that cannot be implemented on common platforms, involve non-intuitive best practices, or must be developed anew, which raise barriers to entry. For example, CA-enhanced DAC requires novel methods for screening enzymatic enhancement of gas-to-liquid mass transfer rate; bio-ERW requires measurement of mineral weathering rates, which can be misrepresented by measurement of ions with different rates of liberation (Amann et al., 2022; van den Berghe et al., 2024); reactor-based bio-MR requires cultivating and screening ultra-slow-growing methanotrophs (He and Lidstrom, 2024) that can be supported in a bioreactor platform; and ME requires standardization of methods to measure gas flux across bark and leaf surfaces to enable broad comparability of data (Gauci et al., 2024). These challenges exemplify the need for interdisciplinary collaborations, including teaching and co-creation of methods.
3.3.3 Funding for identification of required research
Given the cross-disciplinary community challenges limiting progress on the biological GHGR technologies discussed in this Perspective, the challenge of identifying specific research problems should be recognized by the funding community as its own worthy research pursuit. In particular, cross-disciplinary collaboration to improve creativity when defining problems should be incentivized. To enable the community to identify and pursue important research problems, funding should be made available for the creation of knowledge resources, for example through collaborative roadmapping efforts or cross-disciplinary workshops.
3.4 Concluding remarks
Given that biology shapes Earth’s CO2 and methane cycles through biocatalyzed CO2 absorption (Badger and Price, 1994), bioweathering (Finlay et al., 2020), and methanotrophy (Saunois et al., 2020), it stands to reason that those mechanisms could be leveraged for Gt-scale GHGR. CA-enhanced DAC, bio-ERW in soils and reactors, and bio-MR through ME or reactors are early-stage GHGR technologies proposing to do so.
For early-stage climate change mitigation technologies like those discussed here, it is crucial that viability assessment and foundational technology development be performed by financially disinterested parties and made publicly-available; that will ensure fair assessment and enablement of the innovation community toward commercialization and scaling of viable approaches (Holmes et al., 2022). Doing so requires growth, empowerment, and resourcing of new research communities.
Each technology requires derisking to estimate its potential for impact. For CA-enhanced DAC, reactor-based bio-ERW, and reactor-based MR, proposing low cost and low energy process designs with associated LCA and TEA are the first step. For soil-based bio-ERW and ME, the first priority is basic research to identify their potential magnitude and the mechanistic levers available for interventions. CA-enhanced DAC is closest to development, as it requires only engineering. The other technologies also require basic research, and several must overcome the complexities of environmental or feedstock heterogeneity.
Each technology also faces community challenges of integrating non-overlapping disciplines, orienting pure-science disciplines toward engineering, and enrolling small expert communities into new, supported, research programs. Articulating actionable problems in a concise, accessible format will empower growth of new research communities, especially if associated with immediate risk-tolerant funding for new ideas. It will be helpful to facilitate high-trust, friendly relationships in the research community through focused in-person workshops that encourage blue-sky thinking, and inclusion of early-stage approaches in broader gatherings on DAC, ERW and MR.
GHGR technologies address a collective-interest problem that must be solved quickly to ensure the well-being of life on Earth. A culture of collaboration and unified effort that embodies optimism about collective agency and capacity for action will most effectively overcome the research, community, and knowledge gaps currently limiting swift progress.
Data availability statement
The original contributions presented in the study are included in the article/supplementary material. Further inquiries can be directed to the corresponding author.
Author contributions
PR: Conceptualization, Writing – original draft.
Funding
The author(s) declare that financial support was received for the research, authorship, and/or publication of this article. This work was supported by funding from Eric and Wendy Schmidt Fund for Strategic Innovation, with grant number G-23-64552, Grantham Foundation for the Protection of the Environment grant number: 2023-331055 (5022) GB-1596685, Chan Zuckerberg Initiative, and the Nathan Cummings Foundation.
Acknowledgments
The author is grateful to Daniel Goodwin, Paul Himmelstein, Ariana Caiati, and Arya Natarajan for helpful conversation and comments.
Conflict of interest
Homeworld Collective, where the author is the Founding Scientist and Cofounder, intends to fund research addressing problems discussed in this manuscript.
Publisher’s note
All claims expressed in this article are solely those of the authors and do not necessarily represent those of their affiliated organizations, or those of the publisher, the editors and the reviewers. Any product that may be evaluated in this article, or claim that may be made by its manufacturer, is not guaranteed or endorsed by the publisher.
Footnotes
1. ^Relative to a 30% monoethanolamine solvent.
2. ^Mg-oxides and hydroxides, such as brucite, have faster dissolution rates and are limited by different factors, such as abundance or synthesis cost (Campbell et al., 2022).
References
Abernethy, S., Kessler, M. I., and Jackson, R. B. (2023). Assessing the potential benefits of methane oxidation technologies using a concentration-based framework. Environ. Res. Lett. 18:094064. doi: 10.1088/1748-9326/acf603
Amann, T., Hartmann, J., Hellmann, R., Pedrosa, E. T., and Malik, A. (2022). Enhanced weathering potentials—the role of in situ CO2 and grain size distribution. Front. Clim. 4, 103–119. doi: 10.3389/fclim.2022.929268
Badger, M. R., and Price, G. D. (1994). The role of carbonic anhydrase in photosynthesis. Annu. Rev. Plant Physiol. Plant Mol. Biol. 45, 369–392. doi: 10.1146/annurev.pp.45.060194.002101
Beerling, D. J., Kantzas, E. P., Lomas, M. R., Wade, P., Eufrasio, R. M., Renforth, P., et al. (2020). Potential for large-scale CO2 removal via enhanced rock weathering with croplands. Nature 583, 242–248. doi: 10.1038/s41586-020-2448-9
Bonneville, S., Bray, A. W., and Benning, L. G. (2016). Structural Fe (II) oxidation in biotite by an ectomycorrhizal Fungi drives mechanical forcing. Environ. Sci. Technol. 50, 5589–5596. doi: 10.1021/acs.est.5b06178
Bose, H., and Satyanarayana, T. (2017). Microbial carbonic anhydrases in biomimetic carbon sequestration for mitigating global warming: prospects and perspectives. Front. Microbiol. 8:1615. doi: 10.3389/fmicb.2017.01615
Campbell, J. S., Foteinis, S., Furey, V., Hawrot, O., Pike, D., Aeschlimann, S., et al. (2022). Geochemical negative emissions technologies: part I. Review. Front. Clim. 4. doi: 10.3389/fclim.2022.879133
CDR. (2024). Fyi. Available at: https://www.cdr.fyi/ (Accessed May 29, 2024).
Clarkson, M. O., Larkin, C. S., Swoboda, P., Reershemius, T., Suhrhoff, T. J., Maesano, C. N., et al. (2024). A review of measurement for quantification of carbon dioxide removal by enhanced weathering in soil. Front. Clim. 6. doi: 10.3389/fclim.2024.1345224
Corbett, T. D. W., Westholm, M., Rosling, A., Calogiuri, T., Poetra, R., Niron, H., et al. (2024). Organic carbon source controlled microbial olivine dissolution in small-scale flow-through bioreactors, for CO2 removal. Npj Materials Degradation 8, 1–13. doi: 10.1038/s41529-024-00454-w
Davidson, E. A., Monteverde, D. R., and Semrau, J. D. (2024). Viability of enhancing methanotrophy in terrestrial ecosystems exposed to low concentrations of methane. Commun. Earth Environ. 5, 1–5. doi: 10.1038/s43247-024-01656-5
de Oliveira Maciel, A., Christakopoulos, P., Rova, U., and Antonopoulou, I. (2022). Carbonic anhydrase to boost CO2 sequestration: improving carbon capture utilization and storage (CCUS). Chemosphere 299:134419. doi: 10.1016/j.chemosphere.2022.134419
Dees, J. P., Sagues, W. J., Woods, E., Goldstein, H. M., Simon, A. J., and Sanchez, D. L. (2023). Leveraging the bioeconomy for carbon drawdown. Green Chem. 25, 2930–2957. doi: 10.1039/D2GC02483G
Drever, J. I., and Stillings, L. L. (1997). The role of organic acids in mineral weathering. Colloids Surf. A Physicochem. Eng. Asp. 120, 167–181. doi: 10.1016/S0927-7757(96)03720-X
Erans, M., Sanz-Pérez, E. S., Hanak, D. P., Clulow, Z., Reiner, D. M., and Mutch, G. A. (2022). Direct air capture: process technology, techno-economic and socio-political challenges. Energy Environ. Sci. 15, 1360–1405. doi: 10.1039/D1EE03523A
Finlay, R. D., Mahmood, S., Rosenstock, N., Bolou-Bi, E. B., Köhler, S. J., Fahad, Z., et al. (2020). Reviews and syntheses: biological weathering and its consequences at different spatial levels – from nanoscale to global scale. Biogeosciences 17, 1507–1533. doi: 10.5194/bg-17-1507-2020
Fuenzalida-Meriz, G., Timmermann-Aranis, T., Wemmer, K., Yip, C., Yang, Y.-Y., Chowdhury, A., et al. (2024). Microbially-enhanced silicate weathering in agricultural soils to remove carbon dioxide. Res. Square. doi: 10.21203/rs.3.rs-4244369/v1
Gauci, V. (2024). Forests and methane: looking beyond carbon for nature-based climate solutions. Environ. Res. Lett. 19:081005. doi: 10.1088/1748-9326/ad5191
Gauci, V., Pangala, S. R., Shenkin, A., Barba, J., Bastviken, D., Figueiredo, V., et al. (2024). Global atmospheric methane uptake by upland tree woody surfaces. Nature 631, 796–800. doi: 10.1038/s41586-024-07592-w
Gazzè, S. A., Saccone, L., Vala Ragnarsdottir, K., Smits, M. M., Duran, A. L., Leake, J. R., et al. (2012). Nanoscale channels on ectomycorrhizal-colonized chlorite: evidence for plant-driven fungal dissolution. J. Geophys. Res. 117:G00N09. doi: 10.1029/2012jg002016
Gorgolewski, A. S., Caspersen, J. P., Vantellingen, J., and Thomas, S. C. (2023). Tree foliage is a methane sink in upland temperate forests. Ecosystems 26, 174–186. doi: 10.1007/s10021-022-00751-y
Gorham, K. A., Abernethy, S., Jones, T. R., Hess, P., Mahowald, N. M., Meidan, D., et al. (2024). Opinion: a research roadmap for exploring atmospheric methane removal via iron salt aerosol. Atmos. Chem. Phys. 24, 5659–5670. doi: 10.5194/acp-24-5659-2024
Griscom, B. W., Adams, J., Ellis, P. W., Houghton, R. A., Lomax, G., Miteva, D. A., et al. (2017). Natural climate solutions. Proc. Natl. Acad. Sci. USA 114, 11645–11650. doi: 10.1073/pnas.1710465114
Hartmann, J., West, A. J., Renforth, P., Köhler, P., De La Rocha, C. L., Wolf-Gladrow, D. A., et al. (2013). Enhanced chemical weathering as a geoengineering strategy to reduce atmospheric carbon dioxide, supply nutrients, and mitigate ocean acidification: Enhanced Weathering. Rev. Geophys. 51, 113–149. doi: 10.1002/rog.20004
Hazen, R. M., and Ferry, J. M. (2010). Mineral evolution: mineralogy in the fourth dimension. Elements 6, 9–12. doi: 10.2113/gselements.6.1.9
He, L., Groom, J. D., Wilson, E. H., Fernandez, J., Konopka, M. C., Beck, D. A. C., et al. (2023). A methanotrophic bacterium to enable methane removal for climate mitigation. Proc. Natl. Acad. Sci. USA 120:e2310046120. doi: 10.1073/pnas.2310046120
He, L., and Lidstrom, M. E. (2024). Utilisation of low methane concentrations by methanotrophs. Adv. Microb. Physiol. 85, 57–96. doi: 10.1016/bs.ampbs.2024.04.005
Hickey, C., and Allen, M. (2024). Economics of enhanced methane oxidation relative to carbon dioxide removal. Environ. Res. Lett. 19:064043. doi: 10.1088/1748-9326/ad4898
Holmes, D., Humbird, D., Dutkiewicz, J., Tejeda-Saldana, Y., Duffy, B., and Datar, I. (2022). Cultured meat needs a race to mission not a race to market. Nat. Food 3, 785–787. doi: 10.1038/s43016-022-00586-9
Intergovernmental Panel on Climate Change (IPCC) (2023). “The Earth’s energy budget, climate feedbacks and climate sensitivity” in Climate change 2021 – The physical science basis: Working group I contribution to the sixth assessment Report of the intergovernmental panel on climate change. eds. V. Masson-Delmotte, P. Zhai, A. Pirani, S. L. Connors, C. Péan, S. Berger, N. et al. (London: Cambridge University Press), 923–1054.
Jackson, R. B., Abernethy, S., Canadell, J. G., Cargnello, M., Davis, S. J., Féron, S., et al. (2021). Atmospheric methane removal: a research agenda. Philos. Trans. A Math. Phys. Eng. Sci. 379:20200454. doi: 10.1098/rsta.2020.0454
Jackson, R. B., Solomon, E. I., Canadell, J. G., Cargnello, M., and Field, C. B. (2019). Methane removal and atmospheric restoration. Nature Sustain. 2, 436–438. doi: 10.1038/s41893-019-0299-x
La, H., Hettiaratchi, J. P. A., Achari, G., and Dunfield, P. F. (2018). Biofiltration of methane. Bioresour. Technol. 268, 759–772. doi: 10.1016/j.biortech.2018.07.043
Lidstrom, M. E. (2023). Direct methane removal from air by aerobic Methanotrophs. Cold Spring Harb. Perspect. Biol. 16, 1–9. doi: 10.1101/cshperspect.a041671
Lunstrum, A., Berghe, M. V. D., Bian, X., John, S., Nealson, K., and West, A. J. (2023). Bacterial use of siderophores increases olivine dissolution rates by nearly an order of magnitude. Geochem Perspect Lett 25, 51–55. doi: 10.7185/geochemlet.2315
Ma, J., Li, L., Wang, H., Du, Y., Ma, J., Zhang, X., et al. (2022). Carbon capture and storage: history and the road ahead. Engineering 14, 33–43. doi: 10.1016/j.eng.2021.11.024
Massarweh, O., and Abushaikha, A. S. (2024). CO2 sequestration in subsurface geological formations: a review of trapping mechanisms and monitoring techniques. Earth Sci. Rev. 253:104793. doi: 10.1016/j.earscirev.2024.104793
McQueen, N., Gomes, K. V., McCormick, C., Blumanthal, K., Pisciotta, M., and Wilcox, J. (2021). A review of direct air capture (DAC): scaling up commercial technologies and innovating for the future. Prog. Energy Combust. Sci. 3:032001. doi: 10.1088/2516-1083/abf1ce
Mesbahuddin, M. S., Ganesan, A., and Kalyaanamoorthy, S. (2021). Engineering stable carbonic anhydrases for CO2 capture: a critical review. Protein Eng. Des. Sel. 34:gzab021. doi: 10.1093/protein/gzab021
Molina-Fernández, C., and Luis, P. (2021). Immobilization of carbonic anhydrase for CO2 capture and its industrial implementation: a review. J. CO2 Util. 47:101475. doi: 10.1016/j.jcou.2021.101475
Napieralski, S. A., Buss, H. L., Brantley, S. L., Lee, S., Xu, H., and Roden, E. E. (2019). Microbial chemolithotrophy mediates oxidative weathering of granitic bedrock. Proc. Natl. Acad. Sci. USA 116, 26394–26401. doi: 10.1073/pnas.1909970117
National Academies of Sciences, Engineering, and Medicine (2019). Negative Emissions Technologies and Reliable Sequestration: A Research Agenda. Washington, DC: The National Academies Press.
Nisbet-Jones, P. B. R., Fernandez, J. M., Fisher, R. E., France, J. L., Lowry, D., Waltham, D. A., et al. (2022). Is the destruction or removal of atmospheric methane a worthwhile option? Philos Trans. A Math. Phys. Eng. Sci. 380:20210108. doi: 10.1098/rsta.2021.0108
Notin, P., Rollins, N., Gal, Y., Sander, C., and Marks, D. (2024). Machine learning for functional protein design. Nat. Biotechnol. 42, 216–228. doi: 10.1038/s41587-024-02127-0
Paustian, K., Larson, E., Kent, J., Marx, E., and Swan, A. (2019). Soil C sequestration as a biological negative emission strategy. Front. Clim. 1:8. doi: 10.3389/fclim.2019.00008
Pokrovsky, O. S., Shirokova, L. S., Zabelina, S. A., Jordan, G., and Bénézeth, P. (2021). Weak impact of microorganisms on ca, Mg-bearing silicate weathering. Npj Materials Degradation 5, 1–10. doi: 10.1038/s41529-021-00199-w
Porder, S. (2019). How plants enhance weathering and how weathering is important to plants. Elements 15, 241–246. doi: 10.2138/gselements.15.4.241
Reardon, J., Shaffer, A., and Vaysman, V. (2015). Novel flow sheet for low energy CO2 capture enabled by biocatalyst delivery system. Akermin, Incorporated. Available at: https://www.osti.gov/biblio/1176875 (Accessed May 29, 2024).
Russo, M. E., Capasso, C., Marzocchella, A., and Salatino, P. (2022). Immobilization of carbonic anhydrase for CO2 capture and utilization. Appl. Microbiol. Biotechnol. 106, 3419–3430. doi: 10.1007/s00253-022-11937-8
Saipem and Novozymes to work together to create a more (2021). Available at: https://www.saipem.com/en/media/press-releases/2021-12-13/saipem-and-novozymes-work-together-create-more-sustainable-co2 (Accessed May 29, 2024).
Salmon, S., and House, A. (2015). “Enzyme-catalyzed solvents for CO2 separation” in Novel materials for carbon dioxide mitigation technology. eds. F. Shi and B. Morreale (Amsterdam: Elsevier), 23–86.
Saunois, M., Stavert, A. R., Poulter, B., Bousquet, P., Canadell, J. G., Jackson, R. B., et al. (2020). The global methane budget 2000–2017. Earth Syst. Sci. Data 12, 1561–1623. doi: 10.5194/essd-12-1561-2020
Schmider, T., Hestnes, A. G., Brzykcy, J., Schmidt, H., Schintlmeister, A., Roller, B. R. K., et al. (2024). Physiological basis for atmospheric methane oxidation and methanotrophic growth on air. Nat. Commun. 15:4151. doi: 10.1038/s41467-024-48197-1
Sclarsic, S. M. H. (2021). A bioengineering roadmap for negative emissions technologies. Massachusetts Institute of Technology. Available at: https://hdl.handle.net/1721.1/130839 (Accessed October 19, 2024).
Sharifian, R., Wagterveld, R. M., Digdaya, I. A., Xiang, C., and Vermaas, D. A. (2021). Electrochemical carbon dioxide capture to close the carbon cycle. Energy Environ. Sci. 14, 781–814. doi: 10.1039/D0EE03382K
Stein, L. Y., and Lidstrom, M. E. (2024). Greenhouse gas mitigation requires caution. Science 384, 1068–1069. doi: 10.1126/science.adi0503
Strefler, J., Amann, T., Bauer, N., Kriegler, E., and Hartmann, J. (2018). Potential and costs of carbon dioxide removal by enhanced weathering of rocks. Environ. Res. Lett. 13:034010. doi: 10.1088/1748-9326/aaa9c4
Sun, L., Wang, Y., Guan, N., and Li, L. (2020). Methane activation and utilization: current status and future challenges. Energ. Technol. 8:1900826. doi: 10.1002/ente.201900826
Supuran, C. T. (2016). Structure and function of carbonic anhydrases. Biochem. J. 473, 2023–2032. doi: 10.1042/BCJ20160115
Synthesis Report. (n.d.). Climate change 2023. Available at: https://www.ipcc.ch/report/sixth-assessment-report-cycle/ (Accessed May 29, 2024).
Torres, M. A., Dong, S., Nealson, K. H., and West, A. J. (2019). The kinetics of siderophore-mediated olivine dissolution. Geobiology 17, 401–416. doi: 10.1111/gbi.12332
Torres, M. A., West, A. J., and Nealson, K. (2014). Microbial acceleration of olivine dissolution via siderophore production. Procedia Earth Planet. Sci. 10, 118–122. doi: 10.1016/j.proeps.2014.08.041
Tucci, F. J., and Rosenzweig, A. C. (2024). Direct methane oxidation by copper- and Iron-dependent methane monooxygenases. Chem. Rev. 124, 1288–1320. doi: 10.1021/acs.chemrev.3c00727
Tveit, A. T., Hestnes, A. G., Robinson, S. L., Schintlmeister, A., Dedysh, S. N., Jehmlich, N., et al. (2019). Widespread soil bacterium that oxidizes atmospheric methane. Proc. Natl. Acad. Sci. USA 116, 8515–8524. doi: 10.1073/pnas.1817812116
Van Den Berghe, M., Merino, N., Nealson, K. H., and West, A. J. (2021). Silicate minerals as a direct source of limiting nutrients: Siderophore synthesis and uptake promote ferric iron bioavailability from olivine and microbial growth. Geobiology 19, 618–630. doi: 10.1111/gbi.12457
van den Berghe, M., Walworth, N. G., Dalvie, N. C., Dupont, C. L., Springer, M., Andrews, M. G., et al. (2024). Microbial catalysis for CO(2) sequestration: a geobiological approach. Cold Spring Harb. Perspect. Biol. 16, 1–16. doi: 10.1101/cshperspect.a041673
Verbruggen, E., Struyf, E., and Vicca, S. (2021). Can arbuscular mycorrhizal fungi speed up carbon sequestration by enhanced weathering? Plants People Planet 3, 445–453. doi: 10.1002/ppp3.10179
Vicca, S., Goll, D. S., Hagens, M., Hartmann, J., Janssens, I. A., Neubeck, A., et al. (2022). Is the climate change mitigation effect of enhanced silicate weathering governed by biological processes? Glob. Chang. Biol. 28, 711–726. doi: 10.1111/gcb.15993
Wang, J., and He, Q. P. (2023). Methane removal from air: challenges and opportunities. Methane 2, 404–414. doi: 10.3390/methane2040027
Wang, Y., Xue, P., Cao, M., Yu, T., Lane, S. T., and Zhao, H. (2021). Directed evolution: methodologies and applications. Chem. Rev. 121, 12384–12444. doi: 10.1021/acs.chemrev.1c00260
Wild, B., Gerrits, R., and Bonneville, S. (2022). The contribution of living organisms to rock weathering in the critical zone. Npj Materials Degradation 6, 1–16. doi: 10.1038/s41529-022-00312-7
Yi, Q., Wu, S., Southam, G., Robertson, L., You, F., Liu, Y., et al. (2021). Acidophilic iron- and sulfur-oxidizing bacteria, Acidithiobacillus ferrooxidans, drives alkaline pH neutralization and mineral weathering in Fe ore tailings. Environ. Sci. Technol. 55, 8020–8034. doi: 10.1021/acs.est.1c00848
Yoon, S., Carey, J. N., and Semrau, J. D. (2009). Feasibility of atmospheric methane removal using methanotrophic biotrickling filters. Appl. Microbiol. Biotechnol. 83, 949–956. doi: 10.1007/s00253-009-1977-9
Young, J., McQueen, N., Charalambous, C., Foteinis, S., Hawrot, O., Ojeda, M., et al. (2023). The cost of direct air capture and storage can be reduced via strategic deployment but is unlikely to fall below stated cost targets. One Earth 6, 899–917. doi: 10.1016/j.oneear.2023.06.004
Keywords: carbon dioxide removal, atmospheric methane removal, direct air capture, enhanced rock weathering, methanotrophy enhancement, carbonic anhydrase, bioweathering, climate biotechnology
Citation: Reginato PL (2025) Biotechnology in direct air capture, enhanced weathering, and methane removal: emerging opportunities and gaps. Front. Clim. 6:1440833. doi: 10.3389/fclim.2024.1440833
Edited by:
Melissa Paola Mezzari, Independent Researcher, Houston, TX, United StatesReviewed by:
Pamela A. Silver, Harvard Medical School, United StatesNeil Dalvie, Research Fellow at the Synthetic Biology Hive, Harvard Medical School, United States, in collaboration with reviewer PS
Copyright © 2025 Reginato. This is an open-access article distributed under the terms of the Creative Commons Attribution License (CC BY). The use, distribution or reproduction in other forums is permitted, provided the original author(s) and the copyright owner(s) are credited and that the original publication in this journal is cited, in accordance with accepted academic practice. No use, distribution or reproduction is permitted which does not comply with these terms.
*Correspondence: Paul L. Reginato, cGF1bEBob21ld29ybGRjb2xsZWN0aXZlLm9yZw==