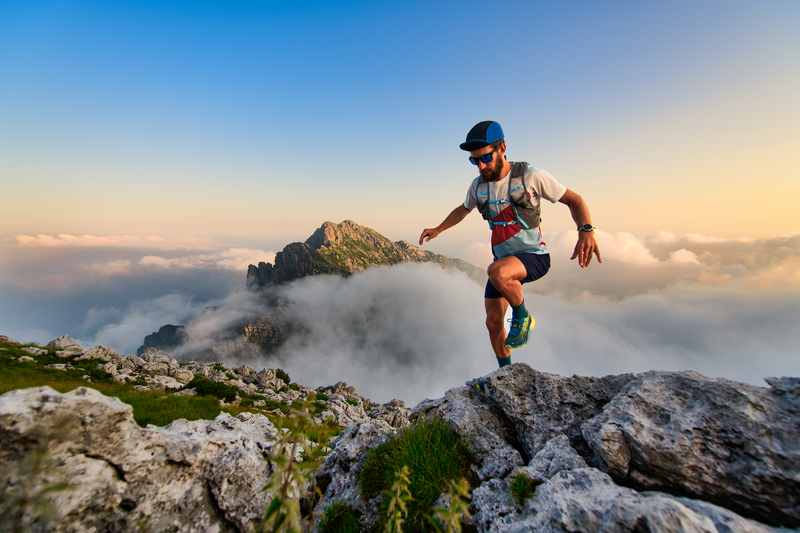
95% of researchers rate our articles as excellent or good
Learn more about the work of our research integrity team to safeguard the quality of each article we publish.
Find out more
PERSPECTIVE article
Front. Clim. , 12 April 2024
Sec. Carbon Dioxide Removal
Volume 6 - 2024 | https://doi.org/10.3389/fclim.2024.1349604
This article is part of the Research Topic Electrochemical Processes for Carbon Dioxide Removal and Long-Term Storage View all articles
Research over the past decade has resulted in various methods for removing CO2 from the atmosphere using seawater and electrochemically generated acids and bases. This Perspective aims to present a unified framework for comparing these approaches. Specifically, these methods can all be seen as falling into one of two categories: those that result in a net increase in ocean alkalinity and use the “ocean as a sponge” for atmospheric CO2 (ocean alkalinity enhancement, or OAE) and those that cycle ocean alkalinity and use the “ocean as a pump” for atmospheric CO2 (ocean alkalinity cycling, or OAC). In this Perspective, approaches for marine carbon dioxide removal (mCDR) using electrochemistry are compared using this framework, and the similarities and differences of these two categories are explored.
There is now scientific consensus that in addition to decarbonization, the active removal of around 5 Gt of atmospheric CO2 per year by 2100 will be necessary to prevent an average global warming of more than 2°C (Calvin et al., 2023). Among the carbon dioxide removal (CDR) technologies being pursued, marine carbon dioxide removal (mCDR) has the potential to reach climate-relevant scales of durable CO2 removal (NASEM, 2022). mCDR leverages the natural role of the oceans in the carbon cycle to remove CO2 from the air. An important group of mCDR techniques are those that remove CO2 using aqueous acid and base that have been generated electrochemically from the seawater itself, with the two most actively pursued approaches being electrochemical ocean alkalinity enhancement (OAE) (Oschlies et al., 2023; Ringham et al., 2024) and what I will refer to here as electrochemical ocean alkalinity cycling (OAC) (de Lannoy et al., 2018). In the case of OAE, CO2 is removed from the atmosphere and durably stored as dissolved inorganic carbon (DIC) in the ocean, whereas OAC captures CO2 from the atmosphere in its gaseous form (Eisaman et al., 2023). In past publications electrochemical OAC been variously referred to as: CO2 extraction from seawater (Eisaman et al., 2012), indirect ocean capture (IOC): acid process (de Lannoy et al., 2018; Eisaman et al., 2018; Eisaman, 2020), direct ocean capture (DOC) (Bui et al., 2023; Kim et al., 2023; Lucas et al., 2023), direct ocean removal (DOR) (NOAA, 2023), CO2 removal from oceanwater (Kim et al., 2023), and electrochemical direct ocean capture (eDOC) (Aleta et al., 2023). This Perspective will use the OAC nomenclature, as it succinctly and clearly emphasizes its relation to electrochemical OAE, the approach to which it is most closely related. The purpose of this perspective is to provide an understanding of the fundamental operating principles and relative merits of electrochemical OAE and OAC.
It should be mentioned that there is what appears to be a third category of approaches, which have variously been termed indirect ocean capture (IOC): base process (Eisaman et al., 2017; de Lannoy et al., 2018; Eisaman et al., 2018) and electrolytic seawater mineralization (La Plante et al., 2023). In these methods, the base is used to increase the alkalinity and pH of seawater to induce the removal of DIC from seawater by precipitating solid carbonates and/or hydroxides. The precipitation step removes some, but not all, of the added alkalinity. Therefore, the alkalinity of the seawater remaining after precipitation is still elevated relative to the starting point. When equilibrated with the air, this alkalinity enhanced seawater will result in the removal of CO2 from the air and storage as DIC in the ocean. These approaches are just a form of OAE where some portion of the added alkalinity goes toward precipitating DIC as solids. While this precipitation may appear inefficient because it removes some of the alkalinity that was just added, these approaches may be motivated by the reuse value or storage stability of solid carbonates or the more gravimetrically and volumetrically dense form of alkalinity in solid hydroxides.
Many methods of alkalinity generation for OAE exist other than the electrochemical generation of aqueous base, such as the addition of terrestrial alkaline minerals to the ocean (Caserini et al., 2022; Eisaman et al., 2023). However, this Perspective will focus specifically on OAE and OAC using electrochemically generated acid and base, and so will generally drop the “electrochemical” qualifier from this point forward.
Figure 1 compares the process flows for OAE, i.e., “ocean as a sponge” (panel A), and OAC, i.e., “ocean as a pump” (panel B). The first step of the OAE process, shown in Figure 1A, is the increase of the total alkalinity (TA) of seawater. For the electrochemical OAE processes we are considering here, this can be accomplished in two ways. One is to generate the acid and base from a separate brine stream (most commonly the acid, base, and salt streams are HCl and NaOH generated from NaCl), and then adding the base mixed with the partially desalted brine to the ocean. Another approach is to generate the acid and base from seawater pumped directly from the ocean, returning the base mixed with the partially desalted seawater back to the ocean. This latter case can be seen as increasing ocean alkalinity through acid removal since the acid that is removed is generated from the seawater itself. As shown on the TA-DIC-pH diagram in Figure 2, the first alkalinity addition step of OAE increases the TA and the pH of the brine or seawater, represented as a vertical arrow on the diagram.
Figure 1. Schematic process flows for the two primary mCDR pathways employing electrochemically generated acid and base: (A) ocean alkalinity enhancement (OAE), or “ocean as a sponge” and (B) ocean alkalinity cycling (OAC), or “ocean as a pump.” The “seawater” label can refer to a separate seawater or brine stream, or to seawater pumped from the ocean itself. The step numbers correspond to those shown in Figure 2. Note that in the OAC case, only a small fraction of the total seawater needed is used to electrochemically generate acid and base – most of the seawater that must be pumped through the system for the OAC process is used in the acidification and CO2 extraction steps, as is indicated qualitatively by the relative seawater arrow widths in panel (B).
Figure 2. The TA-DIC-pH pathway for OAE and OAC. The underlying TA-DIC-pH diagram was created using PyCO2Sys (Humphreys et al., 2022), version 1.8.2 using carbonate equilibrium constants from Lueker et al. (2000), the bisulfate dissociation constant from Dickson (1990), borate: salinity from Lee et al. (2010), and pH on the total scale. The black solid line represents the condition where the surface ocean is in equilibrium with an atmospheric pCO2 value of 420 ppm. The start and end points of both OAC and OAE lie on this line. Because the slope of this line is slightly steeper than the slope of the constant pH lines, the end point of OAE is at a slightly higher pH value than the start. OAC is a rectangle in TA-DIC space, and OAE is an inverted “L” shape. The lengths of the arrows shown above are just one example, and others are possible. For example, the acidification and alkalinity restoration arrows (or the CO2 extraction and CO2 removal arrows) for OAC could be shorter or longer than shown above. Likewise, the alkalinity addition arrow for OAE could be shorter or longer. Finally, for OAE, the length ratio of the CO2 removal arrow to the alkalinity addition arrow is set to be 0.8, but other values are possible (He and Tyka, 2023).
The next, and final, step in the OAE process shown in Figure 1A is the equilibration of the seawater or brine containing elevated TA and pH with the air, resulting in the net removal of CO2 from the air and storage in the ocean as additional DIC (mostly in the form of bicarbonate ions, HCO3−). As indicated by the red box, Monitoring, Reporting, and Verification (MRV) must be performed on this step to quantify the amount of CO2 moved from the air into oceanic DIC as a result of the OAE process (Ho et al., 2023). If the alkalinity is released to the ocean immediately after it is generated, the MRV of CO2 removal must be performed in the open ocean as the plume of enhanced alkalinity spreads and dilutes as it mixes with the ocean. This is referred to as “open system MRV.” In principle, the alkalinity can be contacted with, and remove CO2 from, the air in closed containers before releasing it to the ocean. This is referred to as “closed system MRV.” Relative to open systems, closed systems have the advantage of more straightforward MRV, but the disadvantage of greater cost incurred by the equipment and energy needed for controlled air contacting. For a given implementation, these tradeoffs can be optimized by operating in a hybrid mode that partially equilibrates the alkalinity in a closed system, and then releases to the ocean for the remaining CO2 removal to occur in the open ocean.
The flow diagram and TA-DIC-pH pathway for OAC are shown in Figures 1B, 2. The final two steps of OAC (Steps 3 and 4 – alkalinity restoration and CO2 removal) are the same as the first and only two steps of OAE. In the case of OAC, however, there are two initial steps not required for OAE: acidification and CO2 extraction from seawater. The acidification step shifts the carbonate buffer system of the seawater or brine such that all DIC is now in the form of dissolved CO2 gas. This corresponds to a downward arrow in Figure 2 as TA and pH are decreased. The CO2 extraction step removes this CO2 gas from seawater, resulting in the decrease in DIC shown in Figure 2. Note that because the equilibrium partial pressure of seawater DIC converted to CO2 gas is much less than atmospheric pressure, vacuum stripping must be used to extract relatively pure CO2 gas (de Lannoy et al., 2018; Eisaman, 2018; Eisaman et al., 2018). Step 3 for OAC uses the generated base to restore the seawater TA back to its starting value, as shown by the vertical arrow in Figure 2. Finally, Step 4 of OAC is the equilibration of the seawater with air, resulting in CO2 removal from the air and storage as DIC. As is the case for OAE, the MRV for OAC occurs during this equilibration step, and can be designed as an open, closed, or hybrid system. In the case of OAC, the maximum amount of CO2 pulled from the air into the ocean is equal to the amount of CO2 stripped from seawater in Step 2, resulting a closed loop on the TA-DIC-pH plot that returns the seawater back to its starting conditions.
Empirical data exist for specific approaches to OAE (La Plante et al., 2023; Ringham et al., 2024) and OAC (de Lannoy et al., 2018; Eisaman et al., 2018; Kim et al., 2023) that confirm the general process flows shown in Figures 1, 2. In addition, ocean modeling of OAE has also confirmed this behavior (He and Tyka, 2023; Wang et al., 2023).
From the description above, the “sponge” and “pump” labels for OAE and OAC, respectively, become clear: OAE durably absorbs additional CO2 from the air into the ocean as added DIC, whereas OAC uses the ocean as a pass-through to pull CO2 from air.
One important difference between OAE and OAC is the volume of seawater that must be processed per ton of CO2 removed. For OAC, the amount of CO2 that can be removed from the air using a given volume of seawater is the amount that exactly replaces the DIC that was extracted in the acidification and extraction steps (Steps 1 and 2 of OAC in Figures 1B, 2). A typical value for DIC in seawater is around 0.0025 M (Butler, 2019), meaning that OAC can remove 0.0025 moles of CO2 from the air per liter of seawater, corresponding to approximately 9,089 cubic meters of seawater that must pass through the OAC system per ton of CO2 removed from the air. The amount of seawater that must be processed by OAE for a given amount of CO2 removal is much less than for OAC. Because OAE generates alkalinity from the salt in seawater, the amount of seawater needed for OAE is governed by its NaCl concentration (approximately 0.5 M) rather than the DIC concentration (0.0025 M). Practically, OAE can generate a maximum of approximately 0.25 moles of alkalinity per liter of seawater, with the factor of two less than the salt concentration due to the need for remaining conductivity in the outgoing salt solution. For OAE, the molar ratio of CO2 removed to added alkalinity (ΔCO2/ΔTA) depends on ocean conditions, but a typical range is 0.7–0.8 (He and Tyka, 2023). These values lead to a range of 113 (ΔCO2/ΔTA = 0.8) -129 (ΔCO2/ΔTA = 0.7) cubic meters of seawater that must pass through the OAE system per ton of CO2 removed from the air. Therefore, OAC requires 9,089/129–9,089/113 = 69–80 times more seawater per ton of CO2 removed than OAE. It should be noted that both OAE and OAC require the same amount of seawater to generate the acid and base used in their respective processes, but OAC must pump significant additional volumes of seawater into the system as part of the acidification and CO2 extraction steps of the process. The need for additional seawater pumping will increase both equipment and energy costs (Eisaman et al., 2018; Eisaman, 2020).
Another difference between electrochemical OAE and OAC lies in their byproducts: aqueous acid (HCl) for this specific form of OAE and CO2 gas for OAC. These products need to be used or stored in a manner that prevents a reversal of the CO2 removal process, meaning that the CO2 gas produced by OAC must be durably stored in a way that prevents its leakage to the atmosphere, and the H+ ions in the HCl produced by electrochemical OAE must be prevented from leaking back into the ocean. For HCl, this is most easily accomplished by using the acid in processes that result in its neutralization and the storage, disposal, or beneficial use of the resulting salts. In terms of the rate at which these products are generated, OAC produces one molecule of byproduct CO2 for each molecule removed from the air, so removal of 1GtCO2/y using OAC would require the durable storage of 1Gt/y of byproduct CO2 gas. For the case of OAE, one molecule of HCl is produced for each molecule of NaOH that is generated, meaning that a typical range for (ΔCO2/ΔTA) of 0.7–0.8 (He and Tyka, 2023) corresponds to (1/0.8–1/0.7) = 1.25–1.43 mol(HCl) generated per mol(CO2) removed from the air, or 1.04–1.18 tons(HCl) generated per ton(CO2) on a dry basis. Therefore, the removal of 1GtCO2/y using electrochemical OAE would require the neutralization of 1.04–1.18 Gt/y of byproduct HCl, on a dry basis. Electrochemical OAE generates aqueous acid with typical concentrations of around 0.5 M.
For the CO2 generated by OAC, the sequestration of CO2, either underground or in long-lived materials, will be required (NASEM, 2019). For underground storage, the mineralization of CO2 into solid carbonates via reaction with silicate-containing rocks has been shown to be a promising avenue that reduces concerns about leakage and durability (Matter et al., 2016; Snæbjörnsdóttir et al., 2020; White et al., 2020). To date, the maximum demonstrated rates of mineralization have been on the order of tens of thousands of tons of CO2 per year. In scaling to injection rates of billions of tons of CO2 per year, there is concern that slow reactions will limit the rates of mineralization (Tutolo et al., 2021). In a case where OAC and OAE may complement each other, research is ongoing as to whether this reduced CO2 carbonation efficiency at the gigaton scale can be increased by pretreating, or co-treating (Awolayo et al., 2022), the injection formation with the acid generated by OAE. This approach aims to leverage the orders-of-magnitude increase in mineral dissolution rates and solubilities at low pH values to enhance the concentrations of carbonate forming cations. By enabling CO2 mineralization and acid neutralization at gigaton-per-year scale, this may provide a pathway to enable the deployment of electrochemical OAE, and CDR approaches such as OAC, that require the durable storage of CO2, at climate-relevant scales. That said, pre-existing zones of high permeability may localize injected acid, leading to increased mineral dissolution. This feedback is often termed “fingering” or “wormholing” (Szymczak and Ladd, 2014). Such effects must be minimized for acid pretreatment to realize its potential for CO2 mineralization.
Additionally, through its ability to optimize feedstock properties, the acid generated in electrochemical OAE also potentially enables hybrid approaches with other CDR methods, including enhanced rock weathering (ERW), containerized enhanced weathering, and Biomass Carbon Removal and Storage (BiCRS). Similarly, the CO2 generated in OAC can also be used in hybrid approaches that sequester CO2 in non-geological locations such as concrete.
Despite the fundamental differences between OAE and OAC, some seeming similarities remain. First, with the addition of the equipment and energy needed for controllable contact between alkalinity and air, both OAE and OAC are equally capable of operating as closed or hybrid systems from the perspective of MRV. Second, as seen in Figure 1, the MRV required for OAE and OAC appear to be the same, but there is one important difference: OAE traces the CO2 removal into a plume of TA-enhanced seawater with a typical level of DIC, while OAC traces the CO2 removal into a plume of seawater with drastically reduced DIC and a typical level of TA. Care must be taken in the case of OAC to drive the process along the intended path on the TA-DIC-pH plot, given the almost complete loss of its buffer system after the CO2 extraction step. For OAC, after Step 1 (acidification) and Step 2 (CO2 extraction from seawater), the CO2 has been removed from the seawater and this CO2 must be durably sequestered. It is important to note, however, that this is not the CO2 relevant to MRV. Rather, as shown in Figure 1B, the MRV required for OAC is performed on Step 4 (CO2 extraction from air into the ocean) that occurs after Step 3 (alkalinity restoration). Just like the MRV for OAE, OAC must quantify the amount of CO2 that flows from the air to the ocean to restore equilibrium. As an example, even if one ton of CO2 is removed during Step 2 of OAC, if only half a ton of CO2 is removed from the air in Step 4, perhaps because of alkalinity downwelling from the surface ocean prior to equilibration with the air, then only half a ton of CO2 removal can be claimed. For both OAE and OAC, since the timescale for air-sea gas exchange is longer than the characteristic timescale for dilution, most of the CO2 removal will occur far from the point of dispersion. As a result, MRV will rely on direct measurements of the seawater carbonate chemistry in the vicinity of the outfall (Cyronak et al., 2023; Schulz et al., 2023) combined with ocean modeling to estimate CO2 removal beyond the range of direct detection (Fennel et al., 2023).
In addition to developing MRV for the measurement and verification of CO2 removal, MRV methodologies must also be designed to quantify the environmental and ecological impact of CDR interventions, a subset of MRV sometimes labeled “eMRV.” For example, both OAC and OAE release seawater with elevated pH back to the ocean, resulting in a mixing zone near the point of dispersal where the pH is at its maximum before decreasing as it diffuses away from the mixing zone and mixes with untreated ocean water. Experiments are underway to determine the effect of these changes in carbonate chemistry within the mixing zone on marine ecosystems, with some experiments starting to report results (Gately et al., 2023). The results of these experiments will inform eMRV measurement strategies and establish safe bounds of operation for OAC and OAE.
Scaling CDR to gigatons of CO2 removal per year is a daunting challenge that will almost certainly require the deployment of multiple complimentary approaches. A major thrust of research and development in the next few years should focus on the potential for hybrid approaches, such as electrochemical OAE coupled to CO2 mineralization, ERW, or BiCRS, or the coupling of OAC to CO2 utilization in materials such as concrete. Determining the optimal times and places for deploying various CDR methods will require a clear understanding of their fundamental operating principles and relative merits. Such an understanding and direct comparison for electrochemical OAE and OAC has been lacking to date but is especially important given their superficial similarity in that they both electrochemically generate acid and base from salt. In this Perspective, I have aimed to provide a clear framework for comparing these two promising electrochemical mCDR solutions.
The original contributions presented in the study are included in the article/supplementary material, further inquiries can be directed to the corresponding author.
ME: Conceptualization, Funding acquisition, Writing – original draft, Writing – review & editing.
The author(s) declare that financial support was received for the research, authorship, and/or publication of this article. ME acknowledges funding from the Grantham Foundation for the Protection of the Environment under the SEA MATE (Safe Elevation of Alkalinity for the Mitigation of Acidification Through Electrochemistry) grant and the Yale Center for Natural Carbon Capture.
ME would like to thank Ben Tarbell for discussions on this topic and his “ocean as sponge” vs. “ocean as pump” framing, and Mike Tyka for helpful comments.
ME is Co-Founder and Chief Scientific Advisor at Ebb Carbon, Inc.
All claims expressed in this article are solely those of the authors and do not necessarily represent those of their affiliated organizations, or those of the publisher, the editors and the reviewers. Any product that may be evaluated in this article, or claim that may be made by its manufacturer, is not guaranteed or endorsed by the publisher.
Aleta, P., Refaie, A., Afshari, M., Hassan, A., and Rahimi, M. (2023). Direct ocean capture: the emergence of electrochemical processes for oceanic carbon removal. Energy Environ. Sci. 16, 4944–4967. doi: 10.1039/D3EE01471A
Awolayo, A., Tutolo, B., Lauer, R., de Obeso, J. C., and Goldberg, D. (2022). Water-alternating-gas (WAG) injection as a plausible scheme to optimize carbon dioxide mineralization in the basaltic oceanic crust. In Goldschmidt 2022. Hawaii: Hawaii Convention Center
Bui, J. C., Lucas, É., Lees, E. W., Liu, A. K., Atwater, H. A., Xiang, C., et al. (2023). Exploring bipolar membranes for electrochemical carbon capture. ChemRxiv 4:3bv4p. doi: 10.26434/chemrxiv-2023-3bv4p
Calvin, K., et al. (2023). IPCC, 2023: Climate change 2023: Synthesis report. Contribution of working groups I, II and III to the sixth assessment report of the intergovernmental panel on climate change. Geneva, Switzerland. Intergovernmental Panel on Climate Change.
Caserini, S., Storni, N., and Grosso, M. (2022). The availability of limestone and other raw materials for ocean alkalinity enhancement. Glob. Biogeochem. Cycles 36:e2021GB007246. doi: 10.1029/2021GB007246
Cyronak, T., Albright, R., and Bach, L. T. (2023). “Field experiments in ocean alkalinity enhancement research” in Guide to best practices in ocean alkalinity enhancement research. eds. A. Oschlies, A. Stevenson, L. T. Bach, K. Fennel, R. E. M. Rickaby, and T. Satterfield (Göttingen, Germany: Copernicus Publications)
de Lannoy, C.-F., Eisaman, M. D., Jose, A., Karnitz, S. D., DeVaul, R. W., Hannun, K., et al. (2018). Indirect ocean capture of atmospheric CO2: part I. Prototype of a negative emissions technology. Int. J. Greenhouse Gas Control 70, 243–253. doi: 10.1016/j.ijggc.2017.10.007
Dickson, A. G. (1990). Standard potential of the reaction: AgCl(s) + 12H2(g) = ag(s) + HCl(aq), and the standard acidity constant of the ion HSO4− in synthetic sea water from 273.15 to 318.15 K. J. Chem. Thermodyn. 22, 113–127. doi: 10.1016/0021-9614(90)90074-Z
Eisaman, M. D. (2018). Energy efficient method for stripping CO2 from seawater. United States US9914644B1. Available at: https://patents.google.com/patent/US9914644B1/en.
Eisaman, M. D. (2020). Negative emissions technologies: the tradeoffs of air-capture economics. Joule 4, 516–520. doi: 10.1016/j.joule.2020.02.007
Eisaman, M. D., Geilert, S., Renforth, P., Bastianini, L., Campbell, J., Dale, A. W., et al. (2023). Assessing the technical aspects of ocean-alkalinity-enhancement approaches. State Planet 2-oae2023, 1–29. doi: 10.5194/sp-2-oae2023-3-2023
Eisaman, M. D., Karnitz, S. D., and Rivest, J. L. B. (2017). Chemical extraction from an aqueous solution. United states US20170342328A1. Available at: https://patents.google.com/patent/US20170342328A1/.
Eisaman, M. D., Parajuly, K., Tuganov, A., Eldershaw, C., Chang, N., and Littau, K. A. (2012). CO2 extraction from seawater using bipolar membrane electrodialysis. Energy Environ. Sci. 5:7346. doi: 10.1039/c2ee03393c
Eisaman, M. D., Rivest, J. L. B., Karnitz, S. D., De Lannoy, C.-F., Jose, A., DeVaul, R. W., et al. (2018). Indirect ocean capture of atmospheric CO2: part II. Understanding the cost of negative emissions. Int. J. Greenhouse Gas Control 70, 254–261. doi: 10.1016/j.ijggc.2018.02.020
Fennel, K., Long, M. C., Algar, C., Carter, B., Keller, D., Laurent, A., et al. (2023). “Modelling considerations for research on ocean alkalinity enhancement (OAE)” in Guide to best practices in ocean alkalinity enhancement research. eds. A. Stevenson, L. T. Bach, R. E. M. Rickaby, T. Satterfield, R. Webb, and J. P. Gattuso (Göttingen, Germany: Copernicus Publications)
Gately, J. A., Kim, S. M., Jin, B., Brzezinski, M. A., and Iglesias-Rodriguez, M. D. (2023). Coccolithophores and diatoms resilient to ocean alkalinity enhancement: a glimpse of hope? Sci. Adv. 9:eadg6066. doi: 10.1126/sciadv.adg6066
He, J., and Tyka, M. D. (2023). Limits and CO2 equilibration of near-coast alkalinity enhancement. Biogeosciences 20, 27–43. doi: 10.5194/bg-20-27-2023
Ho, D. T., Bopp, L., Palter, J. B., Long, M. C., Boyd, P. W., Neukermans, G., et al. (2023). Monitoring, reporting, and verification for ocean alkalinity enhancement. State Planet 12:2-oae2023. doi: 10.5194/sp-2-oae2023-12-2023
Humphreys, M. P., Schiller, A. J., Sandborn, D. E., Gregor, L., Pierrot, D., van Heuven, S. M. A. C., et al. (2022). PyCO2SYS: marine carbonate system calculations in Python. Zenodo 3:3744275. doi: 10.5281/zenodo.3744275
Kim, S., Nitzsche, M. P., Rufer, S. B., Lake, J. R., Varanasi, K. K., and Hatton, T. A. (2023). Asymmetric chloride-mediated electrochemical process for CO2 removal from oceanwater. Energy Environ. Sci. 16, 2030–2044. doi: 10.1039/D2EE03804H
La Plante, E. C., Chen, X., Bustillos, S., Bouissonnie, A., Traynor, T., Jassby, D., et al. (2023). Electrolytic seawater mineralization and the mass balances that demonstrate carbon dioxide removal. ACS ES T Eng. 3, 955–968. doi: 10.1021/acsestengg.3c00004
Lee, K., Kim, T.-W., Byrne, R. H., Millero, F. J., Feely, R. A., and Liu, Y.-M. (2010). The universal ratio of boron to chlorinity for the North Pacific and North Atlantic oceans. Geochim. Cosmochim. Acta 74, 1801–1811. doi: 10.1016/j.gca.2009.12.027
Lucas, É., Bui, J., Hwang, M., Wang, K., Bell, A., Weber, A., et al. (2023). Asymmetric bipolar membrane for high current density Electrodialysis operation with exceptional stability. ChemRxiv 6:n4c6x. doi: 10.26434/chemrxiv-2023-n4c6x
Lueker, T. J., Dickson, A. G., and Keeling, C. D. (2000). Ocean pCO2 calculated from dissolved inorganic carbon, alkalinity, and equations for K1 and K2: validation based on laboratory measurements of CO2 in gas and seawater at equilibrium. Mar. Chem. 70, 105–119. doi: 10.1016/S0304-4203(00)00022-0
Matter, J. M., Stute, M., Snaebjornsdottir, S. O., Oelkers, E. H., Gislason, S. R., Aradottir, E. S., et al. (2016). Rapid carbon mineralization for permanent disposal of anthropogenic carbon dioxide emissions. Science 352, 1312–1314. doi: 10.1126/science.aad8132
NASEM (2019). Negative emissions technologies and reliable sequestration: a research agenda. The National Academies Press, Washington, D.C.
NASEM (2022). A research strategy for ocean-based carbon dioxide removal and sequestration. Washington, DC: The National Academies Press.
NOAA (2023). Strategy for NOAA carbon dioxide removal (CDR) research: a White paper documenting a potential NOAA CDR science strategy as an element of NOAA’s climate intervention portfolio. Available at: https://repository.library.noaa.gov/view/noaa/52072.
Oschlies, A., Stevenson, A., Bach, L. T., Fennel, K., Rickaby, R. E. M., and Satterfield, T. (2023). Guide to best practices in ocean alkalinity enhancement research, Göttingen, Germany: Göttingen, Germany.
Ringham, M., Hirtle, N., Shaw, C., Lu, X., Herndon, J., Carter, B., et al. (2024). A comprehensive assessment of electrochemical ocean alkalinity enhancement in seawater: kinetics, efficiency, and precipitation thresholds. EGUsphere 19:108. doi: 10.5194/egusphere-2024-108
Schulz, K. G., Bach, L. T., and Dickson, A. G. (2023). “Seawater carbonate chemistry considerations for ocean alkalinity enhancement research: theory, measurements, and calculations” in Guide to best practices in ocean alkalinity enhancement research. eds. A. Oschlies, A. Stevenson, K. Fennel, R. E. M. Rickaby, T. Satterfield, and J.-P. Gattuso (Göttingen, Germany: Copernicus Publications)
Snæbjörnsdóttir, S. Ó., Sigfússon, B., Marieni, C., Goldberg, D., Gislason, S. R., and Oelkers, E. H. (2020). Carbon dioxide storage through mineral carbonation. Nat. Rev. Earth Environ. 1, 90–102. doi: 10.1038/s43017-019-0011-8
Szymczak, P., and Ladd, A. J. C. (2014). Reactive-infiltration instabilities in rocks. J. Fluid Mech. 738, 591–630. doi: 10.1017/jfm.2013.586
Tutolo, B. M., Awolayo, A., and Brown, C. (2021). Alkalinity generation constraints on basalt carbonation for carbon dioxide removal at the Gigaton-per-year scale. Environ. Sci. Technol. 55, 11906–11915. doi: 10.1021/acs.est.1c02733
Wang, H., Pilcher, D. J., Kearney, K. A., Cross, J. N., Shugart, O. M., Eisaman, M. D., et al. (2023). Simulated impact of ocean alkalinity enhancement on atmospheric CO2 removal in the Bering Sea. Earth Fut. 11:e2022EF002816. doi: 10.1029/2022EF002816
Keywords: carbon dioxide removal, negative emissions technologies, marine carbon dioxide removal, ocean carbon dioxide removal, ocean alkalinity enhancement, indirect ocean capture, direct ocean capture, ocean alkalinity cycling
Citation: Eisaman MD (2024) Pathways for marine carbon dioxide removal using electrochemical acid-base generation. Front. Clim. 6:1349604. doi: 10.3389/fclim.2024.1349604
Received: 04 December 2023; Accepted: 01 April 2024;
Published: 12 April 2024.
Edited by:
Mim Rahimi, University of Houston, United StatesReviewed by:
Charithea Charalambous, Rocky Mountain Institute, United StatesCopyright © 2024 Eisaman. This is an open-access article distributed under the terms of the Creative Commons Attribution License (CC BY). The use, distribution or reproduction in other forums is permitted, provided the original author(s) and the copyright owner(s) are credited and that the original publication in this journal is cited, in accordance with accepted academic practice. No use, distribution or reproduction is permitted which does not comply with these terms.
*Correspondence: Matthew D. Eisaman, bWF0dGhldy5laXNhbWFuQHlhbGUuZWR1
Disclaimer: All claims expressed in this article are solely those of the authors and do not necessarily represent those of their affiliated organizations, or those of the publisher, the editors and the reviewers. Any product that may be evaluated in this article or claim that may be made by its manufacturer is not guaranteed or endorsed by the publisher.
Research integrity at Frontiers
Learn more about the work of our research integrity team to safeguard the quality of each article we publish.