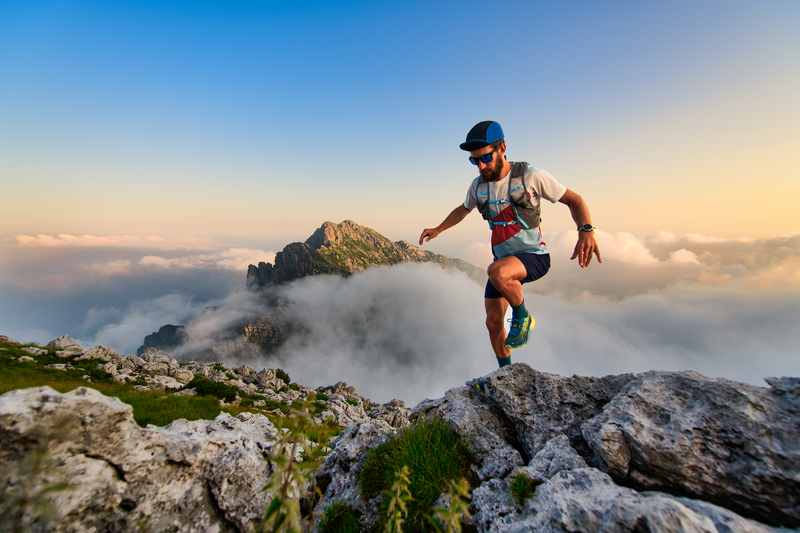
95% of researchers rate our articles as excellent or good
Learn more about the work of our research integrity team to safeguard the quality of each article we publish.
Find out more
REVIEW article
Front. Clim. , 16 August 2022
Sec. Climate, Ecology and People
Volume 4 - 2022 | https://doi.org/10.3389/fclim.2022.941842
This article is part of the Research Topic Climate Change and Health: From Data and Strategies to Real Actions View all 9 articles
Many consequences of climate change undermine the stability of global food systems, decreasing food security and diet quality, and exposing vulnerable populations to multiple forms of malnutrition. The emergence of pandemics such as Covid-19 exacerbate the situation and make interactions even more complex. Climate change impacts food systems at different levels, including changes in soil fertility and crop yield, composition, and bioavailability of nutrients in foods, pest resistance, and risk of malnutrition. Sustainable and resilient food systems, coupled with climate-smart agriculture, are needed to ensure sustainable diets that are adequately diverse, nutritious, and better aligned with contextual ecosystem functions and environmental conservation. Robust tools and indicators are urgently needed to measure the reciprocal food systems-climate change interaction, that is further complicated by pandemics, and how it impacts human health.
Many consequences of climate change threaten food security and diet quality, thereby exposing vulnerable populations across continents to multiple forms of malnutrition. Poor diet is a major cause of mortality and morbidity (Afshin et al., 2019; Micha et al., 2020). Currently, about 690 million people are hungry and the number is expected to surpass 840 million by 2030 (FAO, 2020). As of 2020, 149.2 million children under 5 years of age were stunted and 45.4 million were wasted, partially due to poor diets. Simultaneously, 38.9 million children below 5 years of age were overweight in 2020 (WHO, 2021). These trends are partly driven by inequality and unsustainable foods systems which cannot satisfy food security and nutritional requirements for all. Apart from climate change, other external shocks that adversely impact foods system include pandemics, such as the on-going COVID-19 pandemic, that was projected to add an additional 83–132 million people into the undernourished bracket by 2020 (WHO, 2020).
Climate change worsens unsustainable food systems by directly impacting soil fertility, rain patterns, crop yields and food production, food-nutrient and anti-nutrient composition, and nutrient bioavailability. These changes decrease macro- and micronutrients available in the global food supply. Further problems arise from indirect impacts such as pests that result in increased occurrence of spoilage and food safety hazards at various stages of the food chain from primary production to post-harvest protection through to consumption. Each of these factors may have deleterious impacts on human nutrition (Parfitt et al., 2010; Tirado et al., 2010; Hodges et al., 2011). Measuring this complex and reciprocal food systems-climate change interaction, that is further complicated by pandemics, remains a major challenge especially in how it impacts human health.
This narrative review is based on the outcomes of a Technical Meeting organized by the International Atomic Energy Agency (IAEA) from 19 to 21 October 2020 with the aim of understanding the effectiveness of food-based approaches to improve diet quality under our rapidly changing food systems. This paper covers how food systems and dietary patterns have changed at the community level over time. The vicious and reciprocal cycle between food systems and climate change, in relation to food systems vulnerability and resilience, is discussed. The impact of these interactions on diet quality in terms of food-nutrient, nutrient deficiencies and ultimately the risk of malnutrition is also analyzed. Lastly, this paper highlights the need to develop appropriate measurement tools that can be used to monitor and evaluate the different components and levels (micro- and macro-) of the entire food system.
Food systems comprise all activities from production, post-harvest storage, transportation, processing, distribution, trade and marketing, regulation, consumption of food, and the outcomes of nutrition and health, socio-economy, and the environment. Food systems constitute the first action track of the Decade of Action on Nutrition (WHO, 2017; Demaio and Branca, 2018; Turner et al., 2020). The food environment is an integral part of food systems and consists of an external domain (food availability, product properties, prices, marketing, and regulation) and a personal domain (accessibility, affordability, convenience, and desirability), both of which influence food acquisition, consumption and ultimately nutrition and health outcomes (Turner et al., 2018, 2020; UNSCN, 2019).
The advent of agriculture in the Neolithic revolution marked a shift to predominantly plant-based diets (Leitzmann, 2014). Food systems further evolved with the development of city-states and governance, food storage and means of transportation, trading routes, and consumer demands. Science and technology revolutionized food production, processing, preservation, and transportation (Hueston and McLeod, 2012) with a shift to the consumption of processed energy and macronutrient-dense foods (Vermeulen et al., 2020).
Furthermore, the global meat demand is on the rise, especially within LMICs due to increasing urbanization, education, and affluence (Bruinsma, 2003; Zhang et al., 2017; Headey et al., 2018; Adesogan et al., 2020). For example, per capita meat consumption increased by 20 kg from 1961 to 2014, mainly in Asia and Africa (Vermeulen et al., 2020), while it decreased in many Western countries. High-income countries consume almost six times more milk products and nine times more eggs per capita than low-income countries (Herforth et al., 2019). The global demand for livestock and dairy is projected to increase by 70 and 60%, respectively, between 2010 and 2050.
There is a reciprocal and cyclical interaction between foods systems and climate change. Within the past 40 years, agricultural production has doubled and food supply chains have been globalized (Niles et al., 2017; Von Braun, 2018). Mass food production practices (e.g., fertilizer use, expanded crop and livestock production) and deforestation lead to increased amounts of greenhouse gases and attendant climate change, which in turn result in reduced food production (Niles et al., 2017). Climate change has impacted food systems through weather events such as drought, flooding, and heat waves with attendant loss of life, livelihood, and reduced productivity related to lower soil fertility, disrupted rain patterns, and acid rain from heavy fertilizer use (Niles et al., 2017; Von Braun, 2018). This vicious cycle leads to food insecurity and malnutrition in all its forms, environmental damage, water scarcity, and the emergence of new human, plant, and animal diseases (Tirado et al., 2009; Niles et al., 2017; Von Braun, 2018; Popkin et al., 2020).
The popularization of dietary practices, such as vegetarianism and veganism, in recent decades has resulted in dietary pattern changes that reflect an increased awareness of the environmental footprint associated with the consumption of animal source foods (Leitzmann, 2014). Increased interest in these diets extends beyond a focus on environmental health alone. Unprocessed, vegetarian diets have been associated with several health benefits including longevity and lower rates of diet-related, non-communicable diseases (Burkitt and Trowell, 1977; Keys, 1980; Trowell and Burkitt, 1981; Leitzmann, 2014).
Nevertheless, recent attempts to assess shifts in dietary patterns focus more on the link to socioeconomic status without including how these dietary patterns link to nutrition and health outcomes. Da Costa and colleagues reported that animal source foods were consumed more in western contexts and with increasing income and urbanization (da Costa et al., 2022). At the same time, the definition of a healthy diet has been confusing. O'Hearn et al., assessed dietary patterns in 185 countries and concluded that Western and Latin American regions had healthier dietary patterns compared to Asia and sub-Saharan Africa, but they failed to explain the global rise in the double burden of malnutrition (O'Hearn et al., 2019). This shows that better and more sensitive metrics and tools are needed to better understand the complex dimensions of food systems. Further discussion of the nutritional impacts of dietary patterns occurs in section Climate change, nutrient adequacy, and nutrition and health outcomes.
Sustainable agriculture, nutrition and the livelihoods of millions of people depend on the diversity of crops and livestock species, and intra-species genetic diversity (Sunderland, 2011). The biodiversity of plants and animal species consumed is directly correlated with food security (Sunderland, 2011). Genetic diversity is a critical factor for the continued improvement of crop varieties and livestock breeds, and determines the extent to which genetic resources are passed down to future generations (Rosendal, 2013).
Unfortunately, there has been a dramatic loss of biodiversity, including the diversity of genes, species, and ecosystems due to habitat destruction (i.e., settlement, changing agricultural practices, deforestation, industrialization), global warming, and the uncontrolled spread of invasive species. Pollution, nitrogen deposition, and shifts in precipitation further exacerbate biodiversity loss (Cramer et al., 2017). Over the past 50 years, agriculture has focused too heavily on conventional cereal and horticultural crops leading to the loss of indigenous and traditional food crops (Akinola et al., 2020). While more than 6,000 plant species have been cultivated for food, just 9 account for 66% of total crop production, indicating widespread monoculture agriculture (FAO, 2019). Today, 80–90% of the human diet relies on 12 to 20 species (Chivenge et al., 2015), and only three, rice, maize and wheat contribute nearly 60% of calories and proteins obtained by humans from plants.
Only few terrestrial animal species, namely, cattle, sheep, pig and chicken are domesticated for food production (Robinson and Pozzi, 2011). Almost 26% of livestock breeds are at risk of extinction. About 24% of wild food species are decreasing in abundance, while the status of another 61% is not reported or known (FAO, 2019).
A general reliance on fewer species to feed the world, the resulting loss of biodiversity due to non-utilization and lack of conservation puts food security and human nutrition at great risk (FAO, 2019). Agricultural production therefore must embrace strategies beyond exploiting the same “Green Revolution” technologies from the last half century, which were based on genetic improvement and higher inputs (Kahane et al., 2013). Although such strategies were beneficial in preventing widespread famine, the inappropriate and excessive use of agrochemicals, wasteful water usage via inefficient irrigation systems, loss of beneficial biodiversity (pollinators, soil fauna, etc.) and significantly reduced crop and varietal diversity have had significant deleterious effects on our resulting food systems (Kahane et al., 2013).
Mainstreaming biodiversity conservation is a key strategy to broaden food production to include locally adaptable, often underutilized, nutrient-rich species and ensure diversified, healthy diets and livelihoods among more resilient populations (Bélanger and Pilling, 2019).
Climate change impacts food systems and thereby global food production via changes in yield, biomass food composition and nutritional quality which in turn directly influence human nutrition and health (Glopan, 2020). Climate change can also disrupt food supply chains and transportation, hence food price volatility, and compromised food security, nutrition and human health (FAO, 2020). It exacerbates inequities, and poorer, vulnerable groups tend to suffer more as they are less resilient to shocks (Tirado et al., 2013; Niles et al., 2017; FAO, 2020). Efforts should be put in place to make food systems more climate-smart and nutrition-sensitive, from production to consumption (Bryan et al., 2019; UNSCN, 2020b). Food-based dietary guidelines that include sustainability criteria can help to promote those diets that are good for human and planetary health (UNSCN, 2020a; UN, 2021).
There is a lack of evidence regarding the impact of climate change on human nutrition and health indicators. Climate change may affect human health by altering the food nutrient content via increasing concentrations of CO2 in the atmosphere (Dietterich et al., 2015). Elevated CO2 results in more rapid growth rates but also reduces plant protein content and micronutrients such as calcium, iron, and zinc (Taub et al., 2008; Taub, 2010; Fernando et al., 2012; Loladze, 2014; Myers et al., 2014; Ziska and McConnell, 2016; Medek et al., 2017; Myers, 2017; Smith et al., 2017; Uddling et al., 2018). Most crops grown under elevated CO2–except for legumes and C4 crops—systematically exhibit decreased concentrations of nitrogen and protein in the edible portion (Cotrufo et al., 1998; Pleijel et al., 1999; Idso and Idso, 2001; Jablonski et al., 2002). C3 grains and tubers including rice, wheat, barley, and potatoes experience 7–15% reductions in protein content, whereas C3 legumes and C4 crops show either exceedingly small or insignificant reductions (Myers et al., 2014). Elevated CO2 concentrations of 550 ppm can lead to 3–11% declines in the zinc and iron concentrations of cereal grains and legumes (Myers et al., 2014). Under more extreme conditions, CO2 concentrations of 690 ppm, lead to 5–10% reductions in the concentration of phosphorus, potassium, calcium, sulfur, magnesium, iron, zinc, copper, and manganese across a wide range of crops (Loladze, 2014). The carbon nutrient penalty results in decreases in the global availability of dietary protein of 2.9 to 4.1%, iron 2.8 to 3.6%, and zinc 2.5 to 3.4% (Beach et al., 2019). Overall, the combined effects of projected atmospheric CO2 increases (i.e., carbon nutrient penalty, CO2 fertilization, and climate effects on productivity) will decrease growth in the global availability of nutrients by 19.5% for protein, 13.6% for iron, and 14.6% for zinc relative to expected technology and market gains by 2050.
As discussed in section Evolution of food systems, animal product consumption is on the rise, however an inverse relationship has been observed between animal product consumption and environmental health. This poses a challenge for improving global nutritional status, as the consumption of animal source foods has been linked to improved growth and development in young children, particularly in LMICs. Ecological studies indicate an inverse association between increasing meat consumption per capita and decreasing child stunting rates (UNICEF and The World Bank Group, 2017; Headey et al., 2018). This is attributed to the greater bioavailability of nutrients such as protein and iron from this category of foods. Data from Demographic Health surveys in 49 countries indicates that across sub-Saharan Africa and Asia, dairy, egg, and meat consumption are low while fish consumption is relatively higher (Headey et al., 2018). The urbanization of LMICs seems to be positively associated with egg and fish consumption, and negatively associated with meat consumption (Headey et al., 2018).
Alternative dietary protein sources such as plant proteins, edible insects, seaweed, microalgae and cell-culture based proteins (e.g., cultured milk and eggs; lab-grown meats) (Thavamani et al., 2020; Tso et al., 2020) may offer a suitable alternative to animal source foods with a lower environmental footprint. Edible insects have garnered renewed interest recently as a sustainable protein source. While more than 2,000 species of edible insects collected from wild sources have been identified in traditional diets around the world, a select few have emerged over the past decade as suitable for farming and mass production (Halloran et al., 2018). These insect species convert organic feed substrates very efficiently into animal tissue (protein, fat, and other compounds). Edible insects can be produced on less space, using less water and feed, and they produce less greenhouse gas emission than traditional livestock making them attractive from an environmental sustainability standpoint (Halloran et al., 2016, 2017).
Further research is warranted to evaluate the nutritional quality of these alternative dietary protein sources, with specific focus on the composition and bioavailability of protein, amino acids, essential fatty acids, vitamin B12, zinc and iron and the concentration of anti-nutrient compounds that decrease bioavailability.
Access to safe water remains an extremely important global health issue. More than two billion people live in the dry regions of the world and suffer disproportionately from malnutrition and other health risks related to contaminated or insufficient safe water. The absence of safe water with poor sanitation systems, extreme precipitations with either excessive rainfall, or prolonged drought, all increase exposure to pathogenic microbes resulting in enteric infections and diarrheal diseases. These exacerbate infant and young child malnutrition, leading to retarded growth and development resulting in wasting and stunting (Fink et al., 2011; Guerrant et al., 2013; Ngure et al., 2014). Increased flooding, precipitation, rising temperatures, and other extremes of climate change, are projected to increase the burden of diarrhoeal diseases in low-income regions. Climate change has also been shown to play a role in the spatial and temporal distribution of malaria and is expected to increase the risk of emerging zoonotic diseases. Changes in the survival of pathogens in the environment, changes in migration pathways, carriers and vectors, and changes in the natural ecosystems are all predicted to increase health risks to mankind. Human encroachment into wildlife habitat, including bushmeat hunting and agricultural expansion, has increased the risks of exposure to zoonotic diseases such as HIV, Ebola or SARS-CoV-2 (Goldberg et al., 2012; McGrath, 2020). Furthermore, consumption of poorer quality diets as climate change alters to food system can not only increase the risk of non-communicable diseases as discussed in a prior section, but also increase an individual's susceptibility to infectious diseases (Humphries et al., 2021).
Food systems are faced with various dynamic shocks (Meyer, 2020), which are mostly anthropogenic, including economic, trade, and public health issues such as disease outbreaks (Hamilton et al., 2020). Although climate change is primarily considered an environmental factor, the predicted impacts of climate change, such as increased disease transmission, have wide-sweeping impacts. Food systems depend on the availability of human labor and are easily disrupted by both temporary and chronic shocks. Ebola outbreaks in West Africa (Figuié, 2016) disrupted workers movement, thereby inhibiting food production and food supply chains in the region, while the on-going COVID-19 pandemic has had a similar impact globally (Aday and Aday, 2020; Matthews, 2020; Belton et al., 2021; Weersink et al., 2021).
Many food systems rely on international trade (Gephart et al., 2017; Kummu et al., 2020) and are prone to disruption when countries impose trade restrictions such as export bans on foods to safeguard domestic consumption (Seekell et al., 2017; Aday and Aday, 2020). Food systems that significantly rely on local institutions, knowledge, and farmers are most resilient (Pingali et al., 2005; Hamilton et al., 2020; Kummu et al., 2020; Love et al., 2021). Resilient food systems are based on diversification in production and distribution channels and reduced waste, which cumulatively increase food and nutrition security (Schipanski et al., 2016; Seekell et al., 2017). Similarly, food systems that foster biodiversity have been shown to contribute toward more sustainable food production systems (Snapp et al., 2010). Social factors are another crucial element in food system resiliency. Women are the major food systems stakeholders (Nkengla-Asi et al., 2017), yet there are still large gender-based disparities in access to opportunities to guarantee sustainable and healthy diets for women (Kusakabe, 2004). Food systems fostering gender equity have been shown to be more resilient to shocks, and examples from India and Malawi (Schipanski et al., 2016) have demonstrated that efforts aimed at reducing social injustice, including gender inequity, can foster sustainable and resilient food systems.
As discussed in section Impact of climate change on food nutrient content, climate change is expected to lower the nutrient content of foods. This poses a significant threat because prior attempts to remedy malnutrition via agricultural improvements have focused on achieving caloric sufficiency, leaving hundreds of millions of people to still suffer from micronutrients and protein deficiencies (Nelson et al., 2009; Kahane et al., 2013; Myers et al., 2013, 2017; Springmann et al., 2016; Smith and Myers, 2018; Soares et al., 2019). Interventions such as biofortification (i.e., increase of the micronutrient content of crops by genetic selection), fortification (addition of micronutrients to common edible products that are manufactured in formal and centralized factories), and as a last resource supplementation (provision of pharmaceutical and concentrated forms of micronutrients), have led to significant improvements in population health (Clune et al., 2017; Olson et al., 2021).
Biofortification is a process used to enhance the micronutrient content of staple crops via agronomic practices (i.e., micronutrient fertilizer), conventional plant breeding, and/or genetic modification, making it a food-based strategy with heightened nutritional status at harvest (Khush et al., 2012). Since biofortification is a localized nutrition solution, it is less vulnerable to value-chain disruptions. Furthermore, it allows smallholder farmers across Africa and South Asia to own the solution, as these crops are grown in their fields and the seeds only have to be purchased once as a single investment (Khush et al., 2012). The effects of biofortification on improving nutrition are likewise encouraging, and clinical studies have shown significant improvements in child iron deficiency (Afolami et al., 2021), serum retinol (Afolami et al., 2021), β-carotene concentration (Talsma et al., 2016; Olson et al., 2021). Challenges do remain, however, since sustainability and population coverage are not guaranteed (Bhutta et al., 2013). Biofortification is more sustainable among rural communities as they depend on agriculture for both food and income. Going forward, nutrient bioavailability and anti-nutrient composition of biofortified crops require further attention.
Food fortification, under a public health perspective, is a strategy that takes advantage of using existing food products as micronutrient carriers, manufactured by large, centralized food industries. Maize flour, oil, rice, salt, and wheat flour are the primary food fortification vehicles used to deliver micronutrients. The main advantage of food fortification is that it does not require behavior change modifications since common staples and condiments are used as the fortification vehicles; and social marketing is not needed in fortification programmes.
Iodine deficiency disorders have almost disappeared in most countries with the addition of iodine to salt. Cereal flours containing folic acid have decreased the prevalence of neural tube Defects in both industrialized and LMICs where their consumption is high and the dietary intake of folate is low. Vitamin A deficiency is currently being addressed with fortified sugars and oils in Central America and Eastern and Southern Africa, and iron deficiency can be best prevented via fortified rice, wheat and maize flours at the population level. Additional micronutrient deficiencies (e.g., zinc, vitamins B1, B2, B12, and D) may also be effectively targeted in the future via mainstream fortification vehicles (Dary and Hurrell, 2006; Keats et al., 2019).
Populations most in need often do not receive the fortified commodities targeted for consumption. This is in part due to the fact most food fortification programs are private sector driven, and without adequate private-public sector partnerships, as is the current case (Olson et al., 2021), populations with low purchasing power cannot afford commercialized fortified commodities (Dary, 2007). Supplementation has the restrictions of high cost and exceptionally low coverage. Multiple interventions may be used to effectively target the same population group simultaneously, and this could potentially lead to unintended excess intakes (Olson et al., 2021).
Measuring the impact of changing food systems on diet quality and human health outcomes remains a challenge due to the multi-faceted nature of foods systems and the fact that the existing criterion for a healthy diet is universal instead of being country or context-specific; each country should have domesticated food-based dietary guidelines.
When evaluating diet quality, it is important to consider the data source and how it is processed, interpreted, and used. The source of dietary data can be at the level of the household or the individual. This data can then be processed in the form of metrics or benchmarks, food composition tables, or statistical analysis. Metrics and benchmarks for processing data include nutrient adequacy; consumption of specific health or disease promoting dietary components (e.g., fat, sugar, salt, fruits, and vegetables), and overall quality of diet. No single method is perfect, and all methods have their strengths and weaknesses.
Recent developments such as a resource guide in supporting countries to strengthen nutrition actions based on the policy recommendations of the Second International Conference on Nutrition (ICN2) (WHO, 2018) and the endorsement of the CFS voluntary guidelines for food systems and nutrition are a first step in the right direction toward food systems transformation. However, tools to evaluate the impact of these recommendations and climate-smart solutions are needed. Such tools should be developed with due regard to trade-offs related to crop yield vs. nutrient content and bioavailability, nutritional benefits related to increased animal source food consumption vs. environmental footprint and biodiversity; win-win of moving toward more healthy dietary patterns that are also more sustainable; health consequences of altered food intake behavior; and cross-cutting issues such as gender, urbanization, and food wastage (Figure 1). Future food systems must find ways to provide adequate nourishment without environmental trade-offs.
Figure 1. A food systems continuum and value chain schema to address the link between climate change and diet quality and identify entry opportunities for stable isotope techniques relating to soil, water, and seed biodiversity, food production, nutrient retention and bioavailability, and nutrition, health, and cognitive outcomes.
Some such remaining questions are: (1) What is the impact of climate change on crop nutrient density and bioavailability; are there particularly sensitive nutrients and how does anti-nutrient content vary? (2) What is the role of alternative proteins such as edible insects in the food systems value chain; what is their implication on environmental footprint and food waste? (3) What is the linkage between climate change, sanitary conditions, diet quality and health; what is the role of environmental enteric dysfunction, diarrhea, and mycotoxins? (4) What are the most appropriate tools to holistically assess the foods systems value chain; what role can stable isotope techniques play? (5) What is the minimum set of indicators that can be used to measure the entire food systems continuum (from food production to health including functional outcomes)? Promising innovations in foods systems assessment tools could range from stable isotope techniques (Owino et al., 2017; Owino and Mouratidou, 2019) to assess nutrient bioavailability, functional nutrition outcomes such as body composition, soil fertility, water use efficiency (Wang et al., 2021); metabolomics to reveal the metabolic signature of changes in dietary behaviors and food composition (Jin et al., 2019); to geospatial mapping (Gashu et al., 2021; Giller and Zingore, 2021) to predict the risk of malnutrition based on soil and crop nutrient profiles; (6) What are the implications of climate change on diet quality in the context of population displacement, urbanization, and shifting consumer behavior? (7) How can we make nutrition and health-related research useful to policy makers? (8) What partnerships and collaborations are needed; how can other sectors and disciplines be brought in to comprehensively understand the food systems continuum?
Climate change and unsustainable food systems interact reciprocally with adverse impact on food and nutrition security. Climate change impacts food systems via multiple pathways, including soil fertility, water availability, reduced food yield, reduced food nutrient concentration and bioavailability, increased food anti-nutrient content and increased episodes of infectious diseases. Unsustainable food systems characterized by mass monocultural production with excess fertilizer use, mass livestock production, and deforestation lead to elevated greenhouse gas emissions and loss of biodiversity. Reducing environmental footprints linked to food systems may be achieved by reverting to more sustainable diets that meet nutrition requirements while safeguarding the environment. Dietary diversification, fortification, biofortification, and the inclusion of alternative protein sources (e.g., edible insects) are some of the available alternative options. All of these food systems-climate change-diet and nutrition outcomes are made even more complex by other dynamic factors, including rapid population growth, urbanization, evolving eating habits, and emergent pandemics such as COVID-19. Food systems have witnessed dramatic changes overtime. However, there is a limited ability to measure the multiple points at which these interactions occur. Recent developments at the global level, including ICN2 recommendations and the endorsement of the CFS voluntary guidelines for food systems and nutrition, are a first step in the right direction toward food systems transformation. However, tools to evaluate the impact of these recommendations and climate-smart solutions are needed. The development of these tools should consider crop yield vs. nutrient content and bioavailability, nutritional benefits related to increased animal source food consumption vs. environmental footprint and biodiversity; the win-win of moving toward more healthy, sustainable dietary patterns; unintended health consequences of altered food intake behavior; and cross-cutting issues such as gender, urbanization, and food wastage. Potential health consequences of multiple food-based interventions targeting micronutrient deficiencies must also be assessed. Robust tools and indicators for assessing the impacts and complexity of food systems are needed.
VO, CK, BE, MP, LE, NR, WL, and DT wrote sections of this manuscript. All authors also provided independent review.
All costs related to the virtual Technical Meeting on Leveraging of Stable Isotope Techniques in Evaluating Food-based Approaches to Improve Diet Quality and the Open Access fee for this review were contributed by the IAEA.
We are indebted to all participants of the virtual Technical Meeting on Leveraging of Stable Isotope Techniques in Evaluating Food-based Approaches to Improve Diet Quality (EVT1904254) organized by the IAEA from 19 to 21 October 2020 for their contribution to discussions that inspired the writing of this review. All internal reviewers [Stineke Oenema, Executive Secretary, UN Nutrition; Cornelia Loechl, Head, Nutritional and Health Related Environmental Studies Section, Division of Human Health, International Atomic Energy Agency, Omar Dary, Health Science Specialist, Bureau for Global Health, United States Agency for International Development, Maria Xipsiti, Food and Nutrition Officer, Nutrition and Food Systems Division, FAO] are appreciated for their editorial input.
The authors declare that the research was conducted in the absence of any commercial or financial relationships that could be construed as a potential conflict of interest.
All claims expressed in this article are solely those of the authors and do not necessarily represent those of their affiliated organizations, or those of the publisher, the editors and the reviewers. Any product that may be evaluated in this article, or claim that may be made by its manufacturer, is not guaranteed or endorsed by the publisher.
ASF, Animal Source Foods; CFS, Committee on Food Security; COVID, Coronavirus Disease; DIAAS, Digestible Indispensable Amino Acid Score; FAO, Food and Agriculture Organization of the United Nations; FZA, Fractional Zinc Absorption; HIV, Human Immunodeficiency Virus; IAEA, International Atomic Energy Agency; ICN2, Second International Conference on Nutrition; IRRI, International Rice Research Institute; LMIC, Low-and-middle-income countries; SarCOV2, Severe acute respiratory syndrome Coronavirus 2; UNDP, United Nations Development Programme; UNICEF, United Nations Children's Education Fund; UN SDGs, United Nations Sustainable Development Goals; WHO, World Health Organization.
Aday, S., and Aday, M. S. (2020). Impact of COVID-19 on the food supply chain. Food Qual. Saf. 4, 167–180. doi: 10.1093/fqsafe/fyaa024
Adesogan, A. T., Havelaar, A. H., Mckune, S. L., Eilitt,ä, M., and Dahl, G. E. (2020). Animal source foods: sustainability problem or malnutrition and sustainability solution? Perspective matters. Glob. Food Secur. 25:100325. doi: 10.1016/j.gfs.2019.100325
Afolami, I., Mwangi, M. N., Samuel, F., Boy, E., Ilona, P., Talsma, E. F., et al. (2021). Daily consumption of pro-vitamin A biofortified (yellow) cassava improves serum retinol concentrations in preschool children in Nigeria: a randomized controlled trial. Am. J. Clin. Nutr. 113, 221–231. doi: 10.1093/ajcn/nqaa290
Afshin, A., Sur, P. J., Fay, K. A., Cornaby, L., Ferrara, G., Salama, J. S., et al. (2019). Health effects of dietary risks in 195 countries. 1990–2017: a systematic analysis for the Global Burden of Disease Study 2017. Lancet. 393, 1958–1972. doi: 10.1016/S0140-6736(19)30041-8
Akinola, R., Pereira, L. M., Mabhaudhi, T., De Bruin, F.-M., and Rusch, L. (2020). A review of indigenous food crops in Africa and the implications for more sustainable and healthy food systems. Sustainability 12:3493. doi: 10.3390/su12083493
Beach, R. H., Sulser, T. B., Crimmins, A., Cenacchi, N., Cole, J., Fukagawa, N. K., et al. (2019). Combining the effects of increased atmospheric carbon dioxide on protein, iron, and zinc availability and projected climate change on global diets: a modelling study. Lancet Planet. Health 3, e307–e317. doi: 10.1016/S2542-5196(19)30094-4
Bélanger, J., and Pilling, D. (2019). The State of the World's Biodiversity for Food and Agriculture. Food and Agriculture Organization of the United Nations (FAO).
Belton, B., Rosen, L., Middleton, L., Ghazali, S., Mamun, A.-A., Shieh, J., et al. (2021). COVID-19 impacts and adaptations in Asia and Africa's aquatic food value chains. Mar. Policy. 129:104523. doi: 10.1016/j.marpol.2021.104523
Bhutta, Z. A., Salam, R. A., and Das, J. K. (2013). Meeting the challenges of micronutrient malnutrition in the developing world. Br. Med. Bull. 106, 7–17. doi: 10.1093/bmb/ldt015
Bryan, E., Chase, C., and Schulte, M. (2019). Nutrition-Sensitive Irrigation and Water Management. World Bank.
Chivenge, P., Mabhaudhi, T., Modi, A. T., and Mafongoya, P. (2015). The potential role of neglected and underutilised crop species as future crops under water scarce conditions in Sub-Saharan Africa. Int. J. Environ. Res. Public Health. 12, 5685–5711. doi: 10.3390/ijerph120605685
Clune, S., Crossin, E., and Verghese, K. (2017). Systematic review of greenhouse gas emissions for different fresh food categories. J. Clean. Prod. 140, 766–783. doi: 10.1016/j.jclepro.2016.04.082
Cotrufo, M. F., Ineson, P., and Scott, A. (1998). Elevated CO2 reduces the nitrogen concentration of plant tissues. Glob. Chang. Biol. 4, 43–54. doi: 10.1046/j.1365-2486.1998.00101.x
Cramer, W., Egea, E., Fischer, J., Lux, A., Salles, J.-M., Settele, J., et al. (2017). Biodiversity and Food Security: From Trade-Offs to Synergies. Springer.
da Costa, G. G., da Conceição Nepomuceno, G., and da Silva Pereira, A. (2022). Worldwide dietary patterns and their association with socioeconomic data: an ecological exploratory study. Global Health. 18, 31. doi: 10.1186/s12992-022-00820-w
Dary, O. (2007). “The importance and limitations of food fortification for the management of nutritional anemias,” in Nutritional Anemia, ed J. Badham (Basel: Sight and Life), 315–336.
Dary, O., and Hurrell, R. (2006). Guidelines on Food Fortification With Micronutrients. World Health Organization, Food and Agricultural Organization of the United Nations, Geneva, 1–376.
Demaio, A. R., and Branca, F. (2018). Decade of action on nutrition: our window to act on the double burden of malnutrition. BMJ Glob. Health 3:e000492. doi: 10.1136/bmjgh-2017-000492
Dietterich, L. H., Zanobetti, A., Kloog, I., Huybers, P., Leakey, A. D., Bloom, A. J., et al. (2015). Impacts of elevated atmospheric CO 2 on nutrient content of important food crops. Sci. Data 2, 1–8. doi: 10.1038/sdata.2015.36
FAO (2019). Food and Agriculture Organization of the United Nations Website. FAOSTAT: Food Balance Sheets Page.
FAO, U. (2020). Toolkit for Value Chain Analysis and Market Development Integrating Climate Resilience and Gender Responsiveness - Integrating Agriculture in National Adaptation Plans (NAP-Ag) Programme. Bangkok.
Fernando, N., Panozzo, J., Tausz, M., Norton, R., Fitzgerald, G., and Seneweera, S. (2012). Rising atmospheric CO2 concentration affects mineral nutrient and protein concentration of wheat grain. Food Chem. 133, 1307–1311. doi: 10.1016/j.foodchem.2012.01.105
Figuié, M. (2016). Impact of the Ebola Virus Disease Outbreak on Market Chains and Trade of Agricultural Products in West Africa. Report for FAO REOWA (Resilience, Emergencies and Rehabilitation in West Africa.
Fink, G., Günther, I., and Hill, K. (2011). The effect of water and sanitation on child health: evidence from the demographic and health surveys 1986-2007. Int. J. Epidemiol. 40, 1196–1204. doi: 10.1093/ije/dyr102
Gashu, D., Nalivata, P. C., Amede, T., Ander, E. L., Bailey, E. H., Botoman, L., et al. (2021). The nutritional quality of cereals varies geospatially in Ethiopia and Malawi. Nature 594, 71–76. doi: 10.1038/s41586-021-03559-3
Gephart, J. A., Deutsch, L., Pace, M. L., Troell, M., and Seekell, D. A. (2017). Shocks to fish production: identification, trends, and consequences. Glob. Environ. Change 42, 24–32. doi: 10.1016/j.gloenvcha.2016.11.003
Giller, K. E., and Zingore, S. (2021). Mapping micronutrients in grain and soil unearths hidden hunger in Africa. Nature 594, 31–32. doi: 10.1038/d41586-021-01268-5
Glopan (2020). Global Panel on Agriculture Food Systems for Nutrition: Future Food Systems: for People, Our Planet and Prosperity. Global Panel London.
Goldberg, T. L., Paige, S. B., and Chapman, C. A. (2012). “The Kibale ecohealth project,” in New Directions in Conservation Medicine: Applied Cases of Ecological Health (New York, NY: Oxford University Press), 164–178.
Guerrant, R. L., Deboer, M. D., Moore, S. R., Scharf, R. J., and Lima, A. A. (2013). The impoverished gut—a triple burden of diarrhoea, stunting and chronic disease. Nat. Rev. Gastroenterol. Hepatol. 10, 220–229. doi: 10.1038/nrgastro.2012.239
Halloran, A., Flore, R., Vantomme, P., and Roos, N. (2018). Edible Insects in Sustainable Food Systems. Cham: Springer.
Halloran, A., Hanboonsong, Y., Roos, N., and Bruun, S. (2017). Life cycle assessment of cricket farming in north-eastern Thailand. J. Clean. Prod. 156, 83–94. doi: 10.1016/j.jclepro.2017.04.017
Halloran, A., Roos, N., Eilenberg, J., Cerutti, A., and Bruun, S. (2016). Life cycle assessment of edible insects for food protein: a review. Agron. Sustain. Dev. 36, 1–13. doi: 10.1007/s13593-016-0392-8
Hamilton, H., Henry, R., Rounsevell, M., Moran, D., Cossar, F., Allen, K., et al. (2020). Exploring global food system shocks, scenarios and outcomes. Futures 123:102601. doi: 10.1016/j.futures.2020.102601
Headey, D., Hirvonen, K., and Hoddinott, J. (2018). Animal sourced foods and child stunting. Am. J. Agric. Econ. 100, 1302–1319. doi: 10.1093/ajae/aay053
Herforth, A., Arimond, M., Álvarez-Sánchez, C., Coates, J., Christianson, K., and Muehlhoff, E. (2019). A global review of food-based dietary guidelines. Adv. Nutr. 10, 590–605. doi: 10.1093/advances/nmy130
Hodges, R. J., Buzby, J. C., and Bennett, B. (2011). Postharvest losses and waste in developed and less developed countries: opportunities to improve resource use. J. Agric. Sci. 149:37. doi: 10.1017/S0021859610000936
Hueston, W., and McLeod, A. (2012). “Overview of the global food system: changes over time/space and lessons for future food safety,” in Institute of Medicine (US). Improving Food Safety Through a One Health Approach: Workshop Summary (Washington, DC: National Academies Press (US).
Humphries, D. L., Scott, M. E., and Vermund, S. H., (eds.). (2021). “Pathways linking nutritional status and infectious disease: Causal and conceptual frameworks,” in Nutrition and Infectious Diseases. Nutrition and Health (Cham: Humana), 3–22. doi: 10.1007/978-3-030-56913-6_1
Idso, S. B., and Idso, K. E. (2001). Effects of atmospheric CO2 enrichment on plant constituents related to animal and human health. Environ. Exp. Bot. 45, 179–199. doi: 10.1016/S0098-8472(00)00091-5
Jablonski, L. M., Wang, X., and Curtis, P. S. (2002). Plant reproduction under elevated CO2 conditions: a meta-analysis of reports on 79 crop and wild species. New Phytol. 156, 9–26. doi: 10.1046/j.1469-8137.2002.00494.x
Jin, Q., Black, A., Kales, S. N., Vattem, D., Ruiz-Canela, M., and Sotos-Prieto, M. (2019). Metabolomics and microbiomes as potential tools to evaluate the effects of the Mediterranean diet. Nutrients 11:207. doi: 10.3390/nu11010207
Kahane, R., Hodgkin, T., Jaenicke, H., Hoogendoorn, C., Hermann, M., Hughes, J. D. A., et al. (2013). Agrobiodiversity for food security, health and income. Agron. Sustain. Dev. 33, 671–693. doi: 10.1007/s13593-013-0147-8
Keats, E. C., Neufeld, L. M., Garrett, G. S., Mbuya, M. N., and Bhutta, Z. A. (2019). Improved micronutrient status and health outcomes in low-and middle-income countries following large-scale fortification: evidence from a systematic review and meta-analysis. Am. J. Clin. Nutr. 109, 1696–1708. doi: 10.1093/ajcn/nqz023
Keys, A. (1980). Seven Countries: A Multivariate Analysis of Death and Coronary Heart Disease. Cambridge, MA: Harvard University Press.
Khush, G. S., Lee, S., Cho, J.-I., and Jeon, J.-S. (2012). Biofortification of crops for reducing malnutrition. Plant Biotechnol. Rep. 6, 195–202. doi: 10.1007/s11816-012-0216-5
Kummu, M., Kinnunen, P., Lehikoinen, E., Porkka, M., Queiroz, C., Röös, E., et al. (2020). Interplay of trade and food system resilience: gains on supply diversity over time at the cost of trade independency. Glob. Food Secur. 24:100360. doi: 10.1016/j.gfs.2020.100360
Kusakabe, K. (2004). Women's work and market hierarchies along the border of Lao PDR. Gender Place Cult. 11, 581–594. doi: 10.1080/0966369042000307951
Leitzmann, C. (2014). Vegetarian nutrition: past, present, future. Am. J. Clin. Nutr. 100, 496S−502S. doi: 10.3945/ajcn.113.071365
Loladze, I. (2014). Hidden shift of the ionome of plants exposed to elevated CO2 depletes minerals at the base of human nutrition. Elife 3:e02245. doi: 10.7554/eLife.02245
Love, D. C., Allison, E. H., Asche, F., Belton, B., Cottrell, R. S., Froehlich, H. E., et al. (2021). Emerging COVID-19 impacts, responses, and lessons for building resilience in the seafood system. Glob. Food Secur. 28:100494. doi: 10.1016/j.gfs.2021.100494
Matthews, A. (2020). EU Food system strengths and vulnerabilities during Covid-19. Eurochoices. 19, 4–12. doi: 10.1111/1746-692X.12300
McGrath, M. (2020). Climate Change and Coronavirus: Five Charts About the Biggest Carbon Crash. Science and Environment.
Medek, D., Schwartz, J., and Myers, S. (2017). Estimated effects of future atmospheric CO2 concentrations on protein intake and the risk of protein deficiency by country and region. Environ. Health Perspect. 125, 087002. doi: 10.1289/EHP41
Meyer, M. A. (2020). The role of resilience in food system studies in low-and middle-income countries. Glob. Food Secur. 24:100356. doi: 10.1016/j.gfs.2020.100356
Micha, R., Mannar, V., Afshin, A., Allemandi, L., Baker, P., Battersby, J., et al. (2020). 2020 Global Nutrition Report: Action on Equity to End Malnutrition. Global Nutrition Report.
Myers, S. S. (2017). Planetary health: protecting human health on a rapidly changing planet. Lancet 390, 2860–2868. doi: 10.1016/S0140-6736(17)32846-5
Myers, S. S., Gaffikin, L., Golden, C. D., Ostfeld, R. S., Redford, K. H., Ricketts, T. H., et al. (2013). Human health impacts of ecosystem alteration. Proc. Nat. Acad. Sci. 110, 18753–18760. doi: 10.1073/pnas.1218656110
Myers, S. S., Smith, M. R., Guth, S., Golden, C. D., Vaitla, B., Mueller, N. D., et al. (2017). Climate change and global food systems: potential impacts on food security and undernutrition. Annu. Rev. Public Health 38, 259–277. doi: 10.1146/annurev-publhealth-031816-044356
Myers, S. S., Zanobetti, A., Kloog, I., Huybers, P., Leakey, A. D., Bloom, A. J., et al. (2014). Increasing CO 2 threatens human nutrition. Nature 510, 139–142. doi: 10.1038/nature13179
Nelson, G. C., Rosegrant, M. W., Koo, J., Robertson, R., Sulser, T., Zhu, T., et al. (2009). Climate Change: Impact on Agriculture and Costs of Adaptation. International Food Policy Research Institute.
Ngure, F. M., Reid, B. M., Humphrey, J. H., Mbuya, M. N., Pelto, G., and Stoltzfus, R. J. (2014). Water, sanitation, and hygiene (WASH), environmental enteropathy, nutrition, and early child development: making the links. Ann. N. Y. Acad. Sci. 1308, 118–128. doi: 10.1111/nyas.12330
Niles, M. T., Ahuja, R., Esquivel, J. M., Mango, N., Duncan, M., Heller, M., et al. (2017). Climate change and food systems: Assessing impacts and opportunities. Washington, DC: Meridian Institute.
Nkengla-Asi, L., Babu, S. C., Kirscht, H., Apfelbacher, S., Hanna, R., and Tegbaru, A. (2017). Gender, climate change, and resilient food systems lessons from strategic adaptation by smallholder farmers in Cameroon. IFPRI-Discussion Papers. Washington, DC.
O'Hearn, M., Imamura, F., Cudhea, F., Onopa, J., Reedy, J., Shi, P., et al. (2019). The state of diet quality globally: A Systematic assessment of worldwide dietary patterns using the global dietary database (P10-045-19). Curr. Dev. Nutr. 3, nzz034.P10-045-19. doi: 10.1093/cdn/nzz034.P10-045-19
Olson, R., Gavin-Smith, B., Ferraboschi, C., and Kraemer, K. (2021). Food fortification: the advantages, disadvantages and lessons from sight and life programs. Nutrients 13:1118. doi: 10.3390/nu13041118
Owino, V. O., and Mouratidou, T. (2019). Strengthened data systems to mitigate the double burden of malnutrition: the role of stable isotope technique-derived nutrition indicators. Ann. Nutr. Metab. 75, 119–122. doi: 10.1159/000503669
Owino, V. O., Slater, C., and Loechl, C. U. (2017). Using stable isotope techniques in nutrition assessments and tracking of global targets post-2015. Proc. Nutr. Soc. 76, 495–503. doi: 10.1017/S0029665117000295
Parfitt, J., Barthel, M., and Macnaughton, S. (2010). Food waste within food supply chains: quantification and potential for change to 2050. Philos. Trans. R. Soc. B Biol. Sci. 365, 3065–3081. doi: 10.1098/rstb.2010.0126
Pingali, P., Alinovi, L., and Sutton, J. (2005). Food security in complex emergencies: enhancing food system resilience. Disasters 29, S5–S24. doi: 10.1111/j.0361-3666.2005.00282.x
Pleijel, H., Mortensen, L., Fuhrer, J., Ojanperä, K., and Danielsson, H. (1999). Grain protein accumulation in relation to grain yield of spring wheat (Triticum aestivum L.) grown in open-top chambers with different concentrations of ozone, carbon dioxide and water availability. Agric. Ecosyst. Environ. 72, 265–270. doi: 10.1016/S0167-8809(98)00185-6
Popkin, B. M., Corvalan, C., and Grummer-Strawn, L. M. (2020). Dynamics of the double burden of malnutrition and the changing nutrition reality. Lancet 395, 65–74. doi: 10.1016/S0140-6736(19)32497-3
Robinson, T., and Pozzi, F. (2011). Mapping supply and demand for animal-source foods to 2030. FAO Animal Production and Health Working Paper (Rome), 1–154.
Rosendal, G. K. (2013). The convention on biological diversity and developing countries, Springer Science and Business Media.
Schipanski, M. E., Macdonald, G. K., Rosenzweig, S., Chappell, M. J., Bennett, E. M., Kerr, R. B., et al. (2016). Realizing resilient food systems. Bioscience 66, 600–610. doi: 10.1093/biosci/biw052
Seekell, D., Carr, J., Dell'angelo, J., D'odorico, P., Fader, M., Gephart, J., et al. (2017). Resilience in the global food system. Environ. Res. Lett. 12:025010. doi: 10.1088/1748-9326/aa5730
Smith, M., Golden, C., and Myers, S. (2017). Potential rise in iron deficiency due to future anthropogenic carbon dioxide emissions. GeoHealth 1, 248–257. doi: 10.1002/2016GH000018
Smith, M. R., and Myers, S. S. (2018). Impact of anthropogenic CO2 emissions on global human nutrition. Nat. Clim. Chang. 8, 834–839. doi: 10.1038/s41558-018-0253-3
Snapp, S. S., Blackie, M. J., Gilbert, R. A., Bezner-Kerr, R., and Kanyama-Phiri, G. Y. (2010). Biodiversity can support a greener revolution in Africa. Proc. Nat. Acad. Sci. 107, 20840–20845. doi: 10.1073/pnas.1007199107
Soares, J. C., Santos, C. S., Carvalho, S. M., Pintado, M. M., and Vasconcelos, M. W. (2019). Preserving the nutritional quality of crop plants under a changing climate: importance and strategies. Plant Soil 443, 1–26. doi: 10.1007/s11104-019-04229-0
Springmann, M., Mason-D'croz, D., Robinson, S., Garnett, T., Godfray, H. C. J., Gollin, D., et al. (2016). Global and regional health effects of future food production under climate change: a modelling study. Lancet 387, 1937–1946. doi: 10.1016/S0140-6736(15)01156-3
Sunderland, T. C. (2011). Food security: why is biodiversity important? Int. For. Rev. 13, 265–274. doi: 10.1505/146554811798293908
Talsma, E. F., Brouwer, I. D., Verhoef, H., Mbera, G. N., Mwangi, A. M., Demir, A. Y., et al. (2016). Biofortified yellow cassava and vitamin A status of Kenyan children: a randomized controlled trial. Am. J. Clin. Nutr. 103, 258–267. doi: 10.3945/ajcn.114.100164
Taub, D. (2010). Effects of rising atmospheric concentrations of carbon dioxide on plants. Nat. Educ. Knowl. 3, 21.
Taub, D. R., Miller, B., and Allen, H. (2008). Effects of elevated CO2 on the protein concentration of food crops: a meta-analysis. Glob. Chang. Biol. 14, 565–575. doi: 10.1111/j.1365-2486.2007.01511.x
Thavamani, A., Sferra, T. J., and Sankararaman, S. (2020). Meet the meat alternatives: the value of alternative protein sources. Curr. Nutr. Rep. 9, 346–355. doi: 10.1007/s13668-020-00341-1
Tirado, C., Cohen, M. J., Aberman, N.-L., and Thompson, B. (2009). The impact of climate change on nutrition. Glob. Food Crisis. 129.
Tirado, M. C., Clarke, R., Jaykus, L., Mcquatters-Gollop, A., and Frank, J. (2010). Climate change and food safety: a review. Food Res. Int. 43, 1745–1765. doi: 10.1016/j.foodres.2010.07.003
Tirado, M. C., Crahay, P., Mahy, L., Zanev, C., Neira, M., Msangi, S., et al. (2013). Climate change and nutrition: creating a climate for nutrition security. Food Nutr. Bull. 34, 533–547. doi: 10.1177/156482651303400415
Trowell, H. C., and Burkitt, D. P. (1981). Western Diseases, Their Emergence and Prevention. Cambridge, MA: Harvard University Press.
Tso, R., Lim, A. J., and Forde, C. G. (2020). A critical appraisal of the evidence supporting consumer motivations for alternative proteins. Foods 10:24. doi: 10.3390/foods10010024
Turner, C., Aggarwal, A., Walls, H., Herforth, A., Drewnowski, A., Coates, J., et al. (2018). Concepts and critical perspectives for food environment research: a global framework with implications for action in low-and middle-income countries. Glob. Food Secur. 18, 93–101. doi: 10.1016/j.gfs.2018.08.003
Turner, C., Kalamatianou, S., Drewnowski, A., Kulkarni, B., Kinra, S., and Kadiyala, S. (2020). Food environment research in low-and middle-income countries: a systematic scoping review. Adv. Nutr. 11, 387–397. doi: 10.1093/advances/nmz031
Uddling, J., Broberg, M. C., Feng, Z., and Pleijel, H. (2018). Crop quality under rising atmospheric CO2. Curr. Opin. Plant Biol. 45, 262–267. doi: 10.1016/j.pbi.2018.06.001
UNICEF W. The World Bank Group (2017). Global Database on Child Growth and Malnutrition. Joint Child Malnutrition Estimates- Levels and Trends.
Vermeulen, S. J., Park, T., Khoury, C. K., and Béné, C. (2020). Changing diets and the transformation of the global food system. Ann. N. Y. Acad. Sci. 1478:3. doi: 10.1111/nyas.14446
Von Braun, J. (2018). Economic and for political innovation nutritional improvement. World Rev. Nutr. Diet. 118, 1–9. doi: 10.1159/000484513
Wang, J., Wen, X., Lyu, S., and Guo, Q. (2021). Soil properties mediate ecosystem intrinsic water use efficiency and stomatal conductance via taxonomic diversity and leaf economic spectrum. Sci. Tot. Environ. 783:146968. doi: 10.1016/j.scitotenv.2021.146968
Weersink, A., Von Massow, M., Bannon, N., Ifft, J., Maples, J., Mcewan, K., et al. (2021). COVID-19 and the agri-food system in the United States and Canada. Agric. Syst. 188:103039. doi: 10.1016/j.agsy.2020.103039
WHO (2018). Strengthening Nutrition Action: A Resource Guide for Countries Based on the Policy Recommendations of the Second International Conference on Nutrition. Food and Agriculture Org.
WHO (2020). As More Go Hungry and Malnutrition Persists, Achieving Zero Hunger by 2030 in Doubt, UN Report Warns: Securing Healthy Diets for the Billions WHO Cannot Afford Them Would Save Trillions in Costs. WHO News Release.
WHO (2021). Levels and Trends in Child Malnutrition: UNICEF /WHO / The World Bank Group Joint Child Malnutrition Estimates: Key Findings of the 2021 Edition. Geneva: World Health Organization.
Zhang, J., Wang, D., Eldridge, A. L., Huang, F., Ouyang, Y., Wang, H., et al. (2017). Urban–rural disparities in energy intake and contribution of fat and animal source foods in Chinese children aged 4–17 years. Nutrients 9:526. doi: 10.3390/nu9050526
Keywords: climate change, sustainable food systems, COVID-19 pandemic, nutrient deficiencies, food composition
Citation: Owino V, Kumwenda C, Ekesa B, Parker ME, Ewoldt L, Roos N, Lee WT and Tome D (2022) The impact of climate change on food systems, diet quality, nutrition, and health outcomes: A narrative review. Front. Clim. 4:941842. doi: 10.3389/fclim.2022.941842
Received: 11 May 2022; Accepted: 21 July 2022;
Published: 16 August 2022.
Edited by:
Dingde Xu, Sichuan Agricultural University, ChinaReviewed by:
Huang Kai, University of Chinese Academy of Sciences, ChinaCopyright © 2022 Owino, Kumwenda, Ekesa, Parker, Ewoldt, Roos, Lee and Tome. This is an open-access article distributed under the terms of the Creative Commons Attribution License (CC BY). The use, distribution or reproduction in other forums is permitted, provided the original author(s) and the copyright owner(s) are credited and that the original publication in this journal is cited, in accordance with accepted academic practice. No use, distribution or reproduction is permitted which does not comply with these terms.
*Correspondence: Megan E. Parker, bXBhcmtlckBwYXRoLm9yZw==
Disclaimer: All claims expressed in this article are solely those of the authors and do not necessarily represent those of their affiliated organizations, or those of the publisher, the editors and the reviewers. Any product that may be evaluated in this article or claim that may be made by its manufacturer is not guaranteed or endorsed by the publisher.
Research integrity at Frontiers
Learn more about the work of our research integrity team to safeguard the quality of each article we publish.