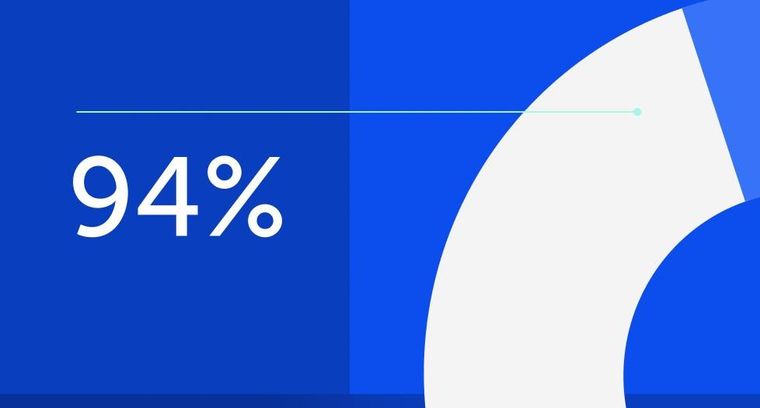
94% of researchers rate our articles as excellent or good
Learn more about the work of our research integrity team to safeguard the quality of each article we publish.
Find out more
ORIGINAL RESEARCH article
Front. Clim., 11 August 2022
Sec. Carbon Dioxide Removal
Volume 4 - 2022 | https://doi.org/10.3389/fclim.2022.928215
This article is part of the Research TopicEnhanced Weathering and Synergistic Combinations with Other CDR MethodsView all 12 articles
Enhanced weathering is a geoengineering strategy aiming to increase continental weathering rates, thereby increasing the delivery of atmospheric carbon (as ) to the oceans. Most enhanced weathering studies focus on the capacity of silicate rocks (e.g., basalt) and minerals (e.g., olivine, Mg2SiO4, or wollastonite CaSiO3) to remove atmospheric CO2. However, carbonate minerals (e.g., calcite, CaCO3) could provide an additional, rapid way to increase export to the oceans. Recent studies suggest that 0.84 Gt C yr−1 could be removed from the atmosphere through the enhanced dissolution of calcite in soils, provided carbonic acid is the main dissolution agent. What is not clear is whether atmospheric CO2 dissolved in soils can be transported by rivers, which typically have lower [pCO2], to the oceans. This difference in calcite solubility between soils (where weathering occurs) and rivers (where is transported) may lead to large amounts of secondary carbonate formation during transport, releasing the CO2 consumed through dissolution. Here, we present a modeling study comparing the estimated soil dissolution capacity (SDC) in 149 of Earth's largest river basins, to the potential transport capacity of carbon (PTCC) in corresponding rivers. We find the SDC can only be exported to the oceans, without secondary carbonate precipitation, if rivers are in disequilibrium with respect to calcite (i.e., SIc = 1). In this instance, 0.92 Gt C yr−1 may be sequestered above background weathering rates, which is ~20% of annual increases in atmospheric carbon. If rivers are at equilibrium with calcite (i.e., SIc = 0), approximately two-thirds of the carbon dissolved in soil waters are lost due to calcite precipitation in rivers, and just 0.26 Gt of additional atmospheric C yr−1 can be transported to the oceans. Overall, the efficacy of enhanced carbonate weathering is a function of the capacity rivers have for transporting the products from carbonate weathering to the oceans, rather than the dissolution capacity of soils. These findings have implications for the efficiency of enhancing silicate weathering for ocean alkalinity enhancement, as secondary carbonate precipitation during transport is not always considered.
The riverine flux of dissolved inorganic carbon (DIC, mostly present as the bicarbonate ion in natural waters), derived from continental weathering, forms a vital link between Earth's atmosphere and oceans, acting to regulate atmospheric [CO2] on million year timescales (Berner and Berner, 2012). A novel approach to combatting the current anthropogenically forced increase in atmospheric CO2, and concomitant global warming, is to artificially increase the flux of solutes from chemical weathering processes, in particular, (Seifritz, 1990; Lackner et al., 1995; Schuiling and Krijgsman, 2006; Köhler et al., 2010; Kelemen et al., 2011; Hartmann et al., 2013). This process of increasing export is known as enhanced weathering (Schuiling and Krijgsman, 2006). The majority of studies concerning enhanced weathering have evaluated the potential of mafic silicate rocks (e.g., basalt) and minerals (e.g., forsterite, Mg2SiO4 and wollastonite, CaSiO3) as reactants (Schuiling and Tickell, 2010; Moosdorf et al., 2014; Renforth et al., 2015; Taylor et al., 2016; Griffioen, 2017; Montserrat et al., 2017; Rigopoulos et al., 2018; Andrews and Taylor, 2019; Haque et al., 2019). The potential of calcium carbonate (CaCO3) and calcium hydroxide (CaOH) dissolution to consume CO2 is poorly constrained (Oh and Raymond, 2006; Zeng et al., 2022b). Carbonate rocks and minerals have faster dissolution kinetics over a range of temperature and pH values in comparison to silicates. For example, experimental studies show calcite dissolution can be >4 orders of magnitude faster than forsterite dissolution at pH 6, c.f. Brantley et al. (2008), Chou et al. (1989), and Plummer et al. (1979). Field observations of silicate mineral dissolution suggest this difference may be even larger (Renforth et al., 2015; White, 2018). Therefore, enhancing carbonate dissolution may be an efficient way to increase the rate of the terrestrial weathering response to increased atmospheric CO2 increase (Rau et al., 2007). Carbonate rocks are also ubiquitous and are often extracted to create aggregates and for other industrial purposes (Hudson et al., 1997; Rau et al., 2007).
Carbonate dissolution with carbonic acid (H2CO3) is often overlooked as a potential method for enhanced weathering since the weathering of carbonates is considered to be a carbon neutral process on geological timescales (Berner and Berner, 2012). However, on the shorter timescales of mineral dissolution reactions and transport to the oceans, carbonate weathering reactions (Equations 1 and 2) dissolve one mole of atmospheric CO2 into solution () per mole of Ca2+ released from a carbonate mineral to aqueous cation. Carbonate rocks are estimated to account for over half of the atmospheric CO2 consumed by rock weathering each year, making carbonate weathering integral to the current understanding of global carbon cycling (Gaillardet et al., 1999).
The influx of anthropogenic CO2 into the global oceans is initially buffered by the dissolution of pre-existing carbonate sediment on the ocean floor (i.e., calcium carbonate neutralization Archer, 1996; Archer et al., 1997), resulting in a shoaling of the carbonate compensation depth (CCD). This initial shoaling is subsequently balanced by a terrestrial input of as weathering rates increase as a consequence of increased atmospheric CO2 (Archer, 1996). The timescales on which these neutralization processes occur are on the order of 1,000–10,000 years (Ridgwell and Hargreaves, 2007). Enhanced weathering increases the rate at which is delivered to the oceans, restoring the equilibrium between seafloor carbonate dissolution and carbonate sedimentation more rapidly than natural weathering rates could achieve. Ultimately, this slows the rate of CCD shoaling, as carbonate weathering products are transferred to the deep ocean to neutralize the influx of atmospheric CO2.
Proposed methods of enhancing carbonate weathering range from point source industrial scale reaction of flue gasses with a limestone seawater mixture (e.g., Rau and Caldeira, 1999; Caldeira and Rau, 2000; Rau et al., 2007), to a large-scale reaction of lime powder on agricultural land with atmospheric CO2 (e.g., Zeng et al., 2022b). In the present study, we focus on liming, a commonplace agricultural method, which involves the spreading of carbonate powder on farm land. Canonically, the carbon in the H2CO3 is derived from the dissolution of atmospheric CO2 into water (Equation 1), but this CO2 can also be derived from the respiration of modern organic matter (e.g., soil carbon <1,000 years old) in the critical zone (Trumbore et al., 1996; Shi et al., 2020). Therefore, the release of cations (chiefly Ca2+) and involved in Equation 2 can help ameliorate the effects of soil acidification, replenish nutrients in the soil profile and increase the ionic strength of soil solutions. Liming has been practiced since antiquity (Connor et al., 2011), and is thought to have played a critical role in the agricultural revolution in Europe, allowing successful intensive crop rotations (Narbarte-Hernandez et al., 2021). Liming is commonplace and considered safe. This is advantageous in comparison to other methods of enhanced weathering, e.g., olivine weathering, which has been shown (in some cases) to release potentially toxic metals in dissolution experiments (Renforth et al., 2015).
Previous studies have demonstrated that liming has the potential to remove appreciable amounts of CO2 from the atmosphere via dissolution. For example, Zeng et al. (2022b) estimate that liming at a global scale could remove ~ 0.84 Gt C yr−1 (Gt = gigaton = 1 ×109 tons), or ~15% of annual increases in atmospheric CO2 (Friedlingstein et al., 2020). For enhanced carbonate weathering via liming to be an effective CO2 consumption mechanism over timescales relevant to the anthropogenic increase in CO2, it is imperative that i) the additional , derived from enhancing carbonate weathering, is not precipitated as secondary carbonate on the continents, and ii) the source of acidity is carbonic acid, with carbon recently derived from the atmosphere. However, many rivers and streams are saturated with respect to calcite, and as these waters outgas and equilibrate with atmospheric CO2 the precipitation of travertines in rivers and calcretes in soils is likely (Dandurand et al., 1982; Drever, 1988; Dreybrodt et al., 1992; Yan et al., 2020; Erlanger et al., 2021). Indeed, the precipitation of carbonates from supersaturated solutions is a well documented phenomenon in a range of climatic settings (Ford and Pedley, 1996; Hudson et al., 1997; Galy et al., 1999; Tipper et al., 2006; Lacelle, 2007; Thomazo et al., 2017; Erlanger et al., 2021). As carbonate precipitation is the reverse of Equation 2, rapid carbonate precipitation in rivers and soils means there is no net consumption of CO2. To date, there has been no systematic study pertaining to whether the carbon (as bicarbonate, ) derived from enhancing carbonate weathering can be successfully transported from the continents to the oceans, without precipitating as secondary carbonate during transit. Therefore, a key issue to quantify is the extent to which the supersaturation of natural waters, induced by liming, limits the transport potential for carbon transfer between the continents and oceans, limiting carbon consumption via enhanced carbonate weathering.
In the present contribution, we define the concept of a potential transport capacity of carbon (PTCC). The PTCC is the difference between the measured concentration in a river basin (), and the theoretical equilibrium concentration before calcite will precipitate (). The equilibrium calcite solubility (and also ) can be determined on the regional or catchment scale as a function of temperature and river pCO2(aq). This solubility represents a chemical equilibrium between a dissolving carbonate mineral (in this case calcite) and river with measured chemistry, constraining the maximum possible concentration river water can have prior to calcite precipitation. As all natural waters already have a concentration resulting from rock and mineral dissolution, the viability of CO2 consumption by enhanced carbonate weathering depends on the difference between the maximum [] (calculated from maximum calcite solubility), and the measured [] within a given river basin. This comparison serves as a PTCC with respect to (or DIC). Our simple calculations highlight that river basins already at or above calcite saturation have very little (or no) capacity to transport additional , which is charge balanced by Ca2+, to the oceans and would be a poor choice for liming. River basins with a low measured [], and are undersaturated with respect to CaCO3, have higher PTCC values and may be appropriate for carbonate weathering by liming. Since many rivers are supersaturated with respect to CaCO3 we evaluate two global simulations of riverine CaCO3 transport capacities, the first at saturation index for calcite (SIc, see methods for definition) = 0 and the second at SIc = 1. These two modeling scenarios provide a baseline PTCC and a maximum PTCC. As a point of reference, the PTCC estimates are compared to a soil dissolution capacity (SDC). This is defined as the total amount of calcite a soil may dissolve, while maintaining a SIc = 0 and a calculated pCO2. Soil pCO2 is often greater than riverine pCO2 (Romero-Mujalli et al., 2019b), and this discrepancy has been observed to result in secondary carbonate precipitation in rivers and other terrestrial environments (Erlanger et al., 2021). Therefore, the SDC serves as an upper bound for estimating CO2 consumption, since degassing and mixing processes will likely lead to soil waters becoming oversaturated and precipitating secondary carbonates.
This approach contributes to the growing body of evidence in favor of enhancing carbonate weathering through liming (Oh and Raymond, 2006; Hamilton et al., 2007; Zeng et al., 2022b), and suggests tenable carbon sequestration potentials.
The modeling approach assumes that it is possible to elevate the concentrations of Ca2+ and in solution through enhanced calcite dissolution until saturation (SIc = 0), or supersaturation (SIc = 1), is reached.
SIc is defined as:
Where αCa2+ and α are the activities of Ca2+ and in solution respectively, and Ksp(calcite) is the equilibrium constant of calcite for a given temperature. Where:
αCa2+ and α are the activities at saturation.
To calculate (i.e., the [] at a given SIc), dissolution, and transport of calcite were assumed to take place under open system conditions, as such it was assumed rivers and soil waters are able to exchange gases with the atmosphere, a constant supply of CO2 from organic matter is available, and rapid dissolution kinetics of calcite. Assuming open system conditions, equilibrium [] in a non-ideal solution can be modeled after after (Drever, 1988; Calmels et al., 2014):
Where K1 and K2 are the first and second dissociation constants of carbonic acid, KCa is the equilibrium constant for calcite dissolution, KH is Henry's law constant for CO2 in water, and are the activity coefficients for calcium and bicarbonate in solution, respectively, and pCO2 is the partial pressure of CO2 in either river or soil waters. A full derivation of Equation (5) is provided in the Supplementary material. The amount of calcite required to reach saturation can then be calculated based on the stoichiometry of Equation 2.
Enhanced rates of organic carbon respiration in soil waters mean pCO2 is generally elevated in soils in comparison to rivers (Romero-Mujalli et al., 2019a; Erlanger et al., 2021). Consequently, according to Equation 5, the at a given temperature and ionic strength in soil waters is typically greater than that of rivers (Erlanger et al., 2021). This is an important distinction. While dissolution rates and [HCO3−] can be elevated in the soil environment, it is not possible to transport these weathering products under equilibrium conditions in rivers to the oceans, where carbonate dissolution products can contribute to long-term carbon storage. This phenomenon has been observed in the poly-lithic Northern-Apenines, where the discrepancy in [HCO3−] between the soil and river environment is caused by a quantitative removal of Ca2+ and HCO3− from river waters, via authigenic carbonate mineral formation (Erlanger et al., 2021). Therefore, it is critical that the discrepancy between the SDC (where most weathering occurs), and the capacity of rivers to transport this carbon (PTCC) is addressed on a global scale.
To compare the open system transport capacity of rivers with the dissolution capacity of high pCO2 soils, soil pCO2 was estimated at a global scale at a grid resolution of 0.5◦ (Romero-Mujalli et al., 2019b):
Where θ is the mean annual volumetric water content of the soil (v/v), T is surface temperature in degrees Celsius, log10(pCO2atm) is logarithm of CO2 partial pressure in the atmosphere (a value of –3.4 is used), b1, b2, b3, and b4 are fitted constants (Romero-Mujalli et al., 2019b). Soil moisture data is from the European Space Agency Climate Change Initiative data portal (ESA, http://www.esa-soilmoisture-cci.org/).
Calculated soil pCO2 values were used in Equation 5 when calculating the carbonate derived SDC. Global gridded riverine pCO2 data were taken from Lauerwald et al. (2015). Riverine pCO2 data were used in Equation 5 when calculating the PTCC for rivers. The solubility of calcite in the river and soil environments was calculated in PHREEQC V3 (Parkhurst and Appelo, 2013) using the Frezchem.dat database (Marion et al., 2010). The Frezchem database was preferred for this analysis as many basins have sub zero annual average temperatures.
This study uses [] for 148 river basins from the GEMS-GLORI database (Figure 1; Meybeck and Ragu, 2012), and Congo data was taken from HYBAM (available at: http://www.ore-hybam.org). This represents ~55% of global runoff (Fekete et al., 2002, 21,000 km3 yr−1). The PTCC, (tons yr−1) is calculated as follows:
Where equib is the modeled equilibrium [] of each river (Mol/L) calculated using Equation 5, measured is the observed [] of each river (Mol/L), Q is the annual discharge of each basin (m3 yr−1), 12 is the atomic mass of carbon, the factor of 0.5 is because half of the C in the is atmospherically derived from the recent atmosphere (Equation 2), and 103 is a conversion factor from kg to tons.
The SDC in each basin was calculated to provide a comparison between the amount of that could be dissolved in soils via the weathering of carbonates, compared to the amount of that can be transported by rivers globally (i.e., PTCC). The SDC (tons yr−1) is calculated as:
Where HCO3−equib is calculated by substituting Equation 6 into Equation 5. The HCO3−equib here is assumed to be entirely derived from the neutralization of CO2 in solution, replicating previous studies (e.g., Zeng et al., 2022b).
K1, K2, KCa, and KCO2 in Equation 5 are all temperature dependent constants, meaning calcite solubility in a given river basin is a function of both temperature and pCO2, and in soils; temperature, soil moisture, and pCO2. Global gridded land temperature data was taken from Fick and Hijmans (2017). Temperature, soil moisture, and pCO2 data were extracted as raster files at 0.5◦ resolution. Data were then clipped to basin polygon shapefiles (GRDC, 2020), and mean and SD values for temperature, pCO2, and soil moisture were extracted. A Monte Carlo error propagation routine was then used to estimate the mean and error for each basin (Supplementary material), based on 1,000 randomly resampled synthetic values derived from the SD of the raw raster data for each catchment (Monte Carlo routine described in Supplementary material). Some larger basins extend over a wide latitude or have significant topography - hence, show more temperature, pCO2, and soil moisture variability. Input data was not available at a high enough resolution to account for seasonal variations. A 40-year average annual temperature was chosen for all model runs.
Three different model scenarios were evaluated:
Model 1: The baseline SDC scenario (assumes SIc = 0). In this scenario, thermodynamic equilibrium with respect to calcite is maintained, meaning calcite dissolution will occur until SIc = 0 for that soil water. This baseline scenario provides a minimum estimate for the dissolution capacity of soil waters and broadly replicates the model conditions estimated by Zeng et al. (2022b).
Model 2: Minimum PTCC scenario, assuming SIc = 0 in the river (transport) environment. In this scenario, river waters can transport carbonate derived until SIc = 0 is reached.
Model 3: Maximum PTCC scenario, assuming SIc = 1 (refer to Section 4.2 for justification) since many rivers draining carbonate lithologies are supersaturated with respect to calcite (Romero-Mujalli et al., 2019b). In this scenario, river waters can transport carbonate derived until SIc = 1 is reached.
The three scenarios outlined are intended to understand i) the difference between the dissolution capacity of soils (SDC) and the transport capacity of rivers (PTCC) with respect to , and ii) the effect of supersaturation vs. saturation on PTCC.
Model results for all catchments are shown in Figure 2, and the 5 catchments with the greatest SDC for Model 1, and PTCC for Models 2 and 3 are shown in Table 1. Full results are shown in the Supplementary material.
Figure 2. Model 1, 2, and 3 results plotted per river basin. Model 1 shows the SDC per basin. Models 2 and 3 display PTCC (potential transport capacity of carbon) values. Marginal bar plots provide a comparison between the SDC of Model 1, and the PTCC of Models 2 and 3 in comparison to annual increases of C in the atmosphere from Friedlingstein et al. (2020).
In the soil environment (when SIc = 0), Model 1 estimates equilibrium dissolution conditions with respect to calcite (Figure 2 and Table 1). Model 1 assumes that the soil HCO3− is entirely derived from calcite dissolution by carbonic acid and the is a function of soil pCO2 (calculated using Equation 6) and temperature. Assuming half of the carbon in the solution is derived from the atmosphere in the reaction between carbonate powder and soil water (Equation 5), Model 1 estimates that the SDC of soils in the river basins in this study is ~0.47 Gt C yr−1. Extrapolated to global discharge this is 0.86 Gt C yr−1, in agreement with Zeng et al. (2022b) (0.84 Gt C yr−1). Current increases in atmospheric C are ~5 Gt C yr−1 (Friedlingstein et al., 2020), therefore, Model 1 represents a 17% removal of C from the atmosphere when extrapolated to global runoff (Figure 2). The amount of CaCO3 required to saturate all soil waters in this study is 4 Gt CaCO3 yr−1, and for all soil waters globally 7.1 Gt CaCO3 yr−1. It is worth noting that these values do not account for the natural weathering of carbonate and silicate already occurring in soils, which can be a substantial source of alkalinity. The SDC serves as a calcite dissolution capacity, rather than an atmospheric carbon sequestration flux. In order for this calcite dissolution to be meaningful as a carbon sequestration strategy, the HCO3− in the soil environment needs to be transported to the oceans without precipitating as a secondary carbonate phase. This transport is principally done via rivers, and the following two models assess the transport capacity of large rivers with respect to calcite.
This model scenario reproduces equilibrium conditions with respect to the aqueous transport of in rivers, i.e., SIc = 0 (Figure 2). The PTCC of Model 2 is 0.15 Gt C yr−1, which extrapolated to global runoff is 0.26 Gt C yr−1 - only 5% of annual increases in atmospheric C (Figure 2). The current rate of C consumption via carbonate weathering is 0.15 Gt C yr−1 (Gaillardet et al., 1997; Zeng et al., 2022b), Model 2 results suggest that the large rivers in this study have the capacity to transport double the amount of HCO3− derived from carbonate weathering each year, and twice that when extrapolated to global discharge. Saturating all rivers in this study via liming would require 2.1 Gt CaCO3 yr−1, and 3.9 Gt CaCO3 yr−1 at a global scale. Model 2 shows that only one-third of the HCO3− that can be dissolved in the soil environment can be transported to the ocean via rivers (without precipitating as secondary carbonates) at SIc = 0 (bar charts, (Figure 2).
This model scenario reproduces supersaturated transport conditions with respect to in rivers, i.e., SIc = 1 (Figure 2). The PTCC of Model 3 is 0.5 Gt C yr−1, which when extrapolated to global runoff is 0.92 Gt C yr−1 - ~20% of annual increases in atmospheric C. These model results suggest that the amount of calcite derived HCO3− that can be transported by rivers globally could be increased by a factor of 6 if supersaturation can be achieved. Saturating all rivers in this study via liming would require 5.1 Gt CaCO3 yr−1, and 9.3 Gt CaCO3 yr−1 on a global scale. Model 3 shows that the transport capacity of rivers at SIc = 1 is similar to the amount of HCO3− that can be dissolved in the soil environment under equilibrium conditions (Figure 2). Therefore, rivers are required to maintain a supersaturated state to transport for all of the , derived from critical zone weathering, to be delivered to the oceans without secondary carbonate precipitation—where it may act to increase the rate of carbonate compensation.
Respiration and decay of organic matter in soil profiles results in soil waters developing a pCO2 content that can be orders of magnitude greater than that of the atmosphere (Figure 3A; Drever, 1988; Friedlingstein et al., 2020). The dominant control on soil respiration, hence soil pCO2, is net primary production, which in itself is largely controlled by temperature (Lloyd and Taylor, 1994), and volumetric soil pore water content (Ilstedt et al., 2000; Blagodatsky and Smith, 2012). Models of respiration rates show that maximum respiration rates occur at soil pore water volumes of ~ 0.3 m3 m−3 (Ilstedt et al., 2000; Romero-Mujalli et al., 2019b), and temperatures >15◦C. In most soils, pCO2 values are ~ 10−2 atm (Drever, 1988), ~ 15 times greater than atmospheric pCO2 10−3.4 atm, (Figure 3A). River waters have intermediate pCO2 values between soils and the atmosphere (Figure 3A) because they are unconfined systems and can degas, equilibrating with the atmosphere. Rivers also have much lower organic carbon concentrations compared to soils, hence high pCO2 concentrations cannot be sustained via organic carbon respiration (Lauerwald et al., 2015).
Figure 3. (A) Difference in pCO2 values between river waters (hexagonal-black points) transporting , and soil waters weathering lime powder (dashed lines). Soil pCO2 values are calculated using Equation 6 (Romero-Mujalli et al., 2019b), labels refer to soil water volume, river pCO2 values are derived from Lauerwald et al. (2015). The difference between soil and river pCO2 values are greatest at higher temperatures, i.e., tropical basins. The mean soil pore water volume for basins in this study is 0.25 m3 m−3, which is derived from ESA soil moisture data. Atmospheric pCO2 (red line) = -3.4. (B) Open system model for different pCO2 solution paths at 10◦C and initial pH = 5 (approximate value for rainwater). Modelled equilibrium soil weathering capacity (Model 1), equilibrium riverine transport (Model 2), and supersaturated riverine transport (Model 3) are shown.
Since calcite solubility is a function of pCO2 (Equation 5), soil waters in warm climates have a much greater calcite solubility (hence equilibrium [HCO3−]) in comparison to their corresponding river waters (Figure 3). For example, the Amazon River (a globally important transport environment) has a calculated HCO3−equib concentration of 2.2 mMol/L at SIc = 0 (Model 2) and 5.2 mMol/L at SIc = 1 (Model 3), whereas soil pore waters in the Amazon basin (i.e., where most weathering takes place) have an equilibrium weathering capacity of 4.7 mMol/L (Table 1). Therefore, the supply of Ca2+ and HCO3− via calcite dissolution in the soil environment can be double that which can be transported to the oceans in the fluvial environment without carbonate precipitation, at SIc = 0 (Figure 3A and Table 1). Comparison of modeled soil [] and spring water [] in karst environments show soils often have greater [] (Zeng et al., 2022a). This demonstrates the of soils multiplied by discharge is not a true measure of carbon capture potential, as the river (assuming calcite saturation), does not have the capacity to transfer this carbon to the oceans at equilibrium conditions (Figure 3B).
However, if rivers are supersaturated with respect to calcite (equivalent to there being a kinetic barrier of nucleation and growth of calcite in the river environment) then in rivers is within the uncertainty of the SDC calculated in this study (Figure 3B and Table 1). Therefore, it is clear that the main control on the carbon capture and sequestration potential of enhanced carbonate weathering is not only the dissolution capacity of soils but the controls on in rivers, where carbon is transferred to the oceans.
Both pCO2 and are greater in the soil environment than in the riverine environment at calcite saturation (e.g., Model 1 vs. Model 2, Figure 3). However, rivers draining carbonate rocks are frequently supersaturated with respect to calcite (Romero-Mujalli et al., 2019a), hence could transport atmospherically derived carbon above their prescribed equilibrium at calcite saturation without it being lost to secondary carbonate precipitation. The global river databases GLORICH (Hartmann et al., 2014) and GEMS-GLORI (Meybeck and Ragu, 2012) were filtered to remove anthropogenic inputs (after Gaillardet et al., 2019). The calcite saturation index was calculated for each river using PHREEQC V3. (frezchem.dat, n = 2,412 Parkhurst and Appelo, 2013). Saturation states >0 are common, 47% of the rivers surveyed were calculated to be oversaturated with respect to calcite (Figure 4). This supports the idea that Model 2 (SIc = 0) serves as a baseline potential transport capacity, and the lack of rivers with SIc >1 suggests Model 3 (SIc = 1) serves as a reasonable upper potential transport limit. A critical question for enhancing carbonate weathering is why rivers become supersaturated with respect to calcite, and how this supersaturation is maintained in a steady-state.
Figure 4. Stacked histogram displaying calculated SIc values for rivers in the GEMS-GLORI (Meybeck and Ragu, 2012) and GLORICH (Hartmann et al., 2014) databases. Mean discharge for GLORICH data is 5 km3 yr−1, the mean for GEMS-GLORI is 170 km3 yr−1.
As the pCO2 of a soil environment is much greater than that of the atmosphere, soil waters will outgas CO2 as they supply the fluvial environment, lowering pCO2(aq) as equilibration with the lower pCO2 atmosphere proceeds (Figure 5; Lauerwald et al., 2015). This outgassing drives calcite supersaturation in river waters (horizontal arrow on Figure 5). Secondly, mixing between atmospherically derived runoff and soil waters can cause a mixture of two fluids which may become supersaturated with respect to calcite (diagonal arrow on Figure 5). Calcite saturation may drive calcite precipitation via the reverse of Equation 2, lowering [] by removal of DIC species to form CaCO3 (vertical arrow on Figure 5). In this instance, the in excess of the calculated (at calcite saturation) will be removed through calcite deposition (Erlanger et al., 2021). CO2 degassing (Lauerwald et al., 2015) and subsequent travertine formation are a well documented phenomena in carbonate terrains (Herman and Lorah, 1987; Dreybrodt et al., 1992; Yan et al., 2020), playing an important role in terrestrial carbon cycling (Raymond et al., 2013). Often, the spontaneous precipitation of carbonate in the fluvial environment is concentrated around specific turbulent environments, e.g., waterfalls and steps (Rogerson et al., 2014). In some cases, calcite precipitation can be microbially mediated (Rogerson et al., 2014). When quantitative precipitation to re-equilibrate waters with respect to calcite does not happen, supersaturation is achieved. Reasons for calcite not precipitating from natural waters with SIc >0 are varied and are explored in the next section. Critically, results from Models 1 and 3 show that for atmospheric carbon dissolved in the soil environment to remain in solution before reaching the oceans, precipitation of calcite (and other Ca or Mg carbonates) must be inhibited in river waters and supersaturation achieved.
Figure 5. Schematic figure presenting two plausible mechanisms for calcite precipitation, or supersaturation, to be achieved. River basins with a mean temperature >15◦C and are undersaturated prior to liming (i.e., positive PTCC) are shown with corresponding modelled soil pore water calcite solubilities from Model 1 and 2 (i.e., SIc = 0). Atmospheric pCO2 is shown as the red line. Precipitation may be triggered either by soil waters degassing when in contact with the atmosphere, or mixing with river waters, creating fluids with even higher SIc values (blue shaded area). Supersaturation via mixing is exacerbated further when river waters are saturated with calcite. All SIc contours are calculated for 25◦C.
For calcite supersaturation to be maintained in rivers the precipitation of carbonate minerals must be inhibited, such that concentrations of can remain above equilibrium. It is important to understand how calcite supersaturation can be maintained in rivers (where is transported) because this will ultimately control the efficiency of CO2 consumption via carbonate weathering. Calcite precipitation can be inhibited in several ways. For example, precipitation can be inhibited by the presence of nucleation inhibitors such as Mg2+, , and organic complexes, which adsorb onto crystal lattices resulting in sluggish calcite precipitation kinetics (Mann, 1988; Astilleros et al., 2010; Dobberschütz et al., 2018). The effect of nucleation inhibitors may then be exacerbated in catchments with short water residence times. If the reaction kinetics of calcite precipitation are slower than the residence time of water in the catchment then disequilibrium in the carbonate system can occur (Herman and Lorah, 1987). The controls on precipitation inhibitors raise interesting points with regards to methods of enhancing carbonate weathering. This study focuses on 149 of the world's largest rivers by discharge, which have long water residence times. Approximately 55% of global discharge is captured in this study, meaning that much smaller streams and rivers draining into the oceans must make up the remaining 45%. Therefore, small rivers could potentially play a crucial role in carbon transfer between the continents and oceans, which is currently unaccounted for. Furthermore, the role of Mg2+ as a calcite precipitation inhibitor suggests that other Mg-bearing carbonates (e.g., dolomite) could make good candidates for enhancing carbonate weathering.
Model 1 calculates an equilibrium dissolution capacity of soils (SDC). In the context of this study, this represents the potential [] in soils that can be achieved by reacting all soil derived pCO2 with calcite. This is the premise of other literature models e.g., Zeng et al. (2022b) and produces similar results. The assumption being that the soil dissolved load shifts from being bedrock dominated to carbonate dominated as a consequence of liming (Zeng et al., 2022b). This has been suggested to represent a weathering flux (Zeng et al., 2022a,b), but this must be an upper limit given 1) the difference in pCO2 between soil waters and rivers (e.g., Figure 2, 3), and 2) natural weathering fluxes will also produce . Natural critical zone fluxes have not been quantified at a global scale and, therefore, cannot be subtracted from the SDC calculated in this study. As such, in this study, it is used as a representative soil dissolution capacity of calcite, to which riverine transport potentials (PTCC) are compared, rather than a potential soil weathering flux for CO2 sequestration as suggested by Zeng et al. (2022b).
Model 2 calculates the transport capacity (PTCC) of in rivers, if the SIc is 0, a conservative estimate. As many river basins are already oversaturated or close to oversaturation with respect to CaCO3 (Figure 2, 4) many basins in Model 2 have negative PTCC values, meaning they already transport in excess of a SIc = 0, i.e., they are oversaturated with respect to calcite. Therefore, they have no capacity to increase carbon consumption through enhanced carbonate weathering. The reasons for this are varied. For example, liming has been prevalent in the Mississippi basin, and much of North America, since the 1950s (Oh and Raymond, 2006)—contributing to the negative transport potentials observed in this basin and much of the U.S. (Figure 2). Negative transport potentials in much of Europe are likely explained by extensive carbonate rock outcrop and karst systems (Goldscheider, 2005), in addition to pollution (Roy et al., 1999, e.g., Seine). Similarly, rivers draining the Himalayan region are known to have dissolved loads dominated by carbonate weathering and precipitate secondary carbonate minerals (Tipper et al., 2006; Bickle et al., 2015). Rivers with their source in the Himalayas with positive transport potentials (e.g., the Mekong) are likely to be supply limited with respect to carbonates—hence may benefit from liming.
Model 3 simulates the transport capacity (PTCC) of if SIc of global rivers is capped at 1 (an upper estimate). Few river basins globally have SIc values >1 (Figure 4), and so PTCC values in Model 3 are largely positive and qualitatively align with SDC results from Model 1 (e.g., comparison of model output in Figure 2). This reiterates the notion that supersaturated transport environments (i.e., Model 3 parameters) are required to transport the products from equilibrium calcite dissolution in the soil environment (i.e., Model 1 parameters, Figure 3).
Basins with the greatest PTCC in Model 2 are those which have both high discharge values and are currently undersaturated with respect to calcite (Figure 6). The largest PTCC values are observed in the tropical and Siberian cratons (e.g., Amazon, Congo, Orinoco, Lena, and Yenisey), which are known to be supply limited (West et al., 2005). Supply limited basins are defined by low erosion rates in comparison to chemical leaching capacities, meaning the capacity for weathering in that particular system outstrips the supply of rock to the critical zone.
Figure 6. Sic vs PTCC >1 ×1014 L yr−1 (top) and rivers with discharge <1 ×1014 L yr−1 (bottom). Error bars are not visible for data where the error is smaller than the data symbol.
At both low (<0◦C) and high (>20◦C) temperatures, at SIc = 0 exceeds for the GEMS river data set (Figure 7). This is indicative of rivers in these temperature ranges being able to enhance their concentrations via enhanced carbonate weathering, and this is reflected in their calculated PTCC predictions (Model 2, Figure 2 and Table 1). Whereas in temperate climates (i.e., 7–20◦C) exceeds or is equivalent to , and fluxes cannot be increased in these rivers.
Figure 7. Measured concentrations in rivers from the GEMS database, , with LOESS fit (star markers and black dashed line with blue CI) and maximum achievable concentration from enhanced carbonate weathering, , at SIc = 0 (Model 2) with LOESS fit (blue dashed line with red CI). Points are coloured by log10(pCO2) and sized based on relative log10(discharge).
Northern high latitude basins have low pCO2 concentrations (Table 1), and as such do not have leaching capacities as great as those in tropical basins. However, these high latitude basins have large PTCC values because of their very low temperatures, which increases calcite solubility—hence, . Therefore, cold river basins with appreciable discharges (e.g., Lena, Yenisey) will have a large potential to transport more in solution via enhanced carbonate weathering, simply because calcite is far more soluble in colder water in comparison to warm (Figure 7).
If calcite solubility is negatively correlated with temperature then it seems counter-intuitive that warm basins (>20◦C) also have an > (Figure 7). Calcite solubility, hence can be high in these warm climates because microbially derived pCO2 production increases with temperature (Equation 6, Lloyd and Taylor, 1994; Romero-Mujalli et al., 2019b), hence the solubility of calcite of is a trade-off between increasing temperature and decreasing calcite solubility, and pCO2 generation increasing calcite solubility as a function of temperature (Figure 7).
Interestingly, the global minimum of the modeled fit representing occurs at ~10◦C (Figure 7), where it is hypothesized carbonate weathering is most intense (Gaillardet et al., 2019; Romero-Mujalli et al., 2019a). This is intriguing as our thermodynamic solubility model suggests this is the least favorable place to transport carbonate derived in rivers to the oceans, whilst maintaining equilibrium (SIc = 0). If temperate climates are relatively poor at transporting the products of carbonate dissolution, as suggested by this model (Figure 7), then we speculate that authigenic carbonate precipitation would be more abundant in temperate climates. Differences in river and bedrock Mg/Ca and stable isotope ratios (e.g., Ca isotope ratios) in temperate climates have been suggested to be a consequence of large amounts of secondary carbonate precipitation (Tipper et al., 2010; Bickle et al., 2015), which provides some credence to this idea. Overall, the intensity of carbonate weathering, and the possibility of secondary carbonate precipitation, make enhancing carbonate weathering in temperate climates unfavorable (Figure 7).
In summary, tropical basins like the Amazon and Congo are ideal for liming as sustained rapid denudation kinetics over periods of millions of years has resulted in shield exposure and thick regolith development (Gaillardet et al., 1997; Braun et al., 2005). High solubility phases, such as CaCO3, have long since been weathered and tropical rivers are often dilute due to high discharges—making them very undersaturated with respect to calcite. A combination of these factors and high organic carbon respiration rates, i.e., very high pCO2 concentrations (Lauerwald et al., 2015), results in tropical basins being ideal for transporting large quantities of carbonate dissolution products (i.e., ) in solution to the oceans (Figure 2, Table 1). Similarly, Siberian rivers could export more than they currently do to the oceans without authigenic carbonate precipitation, on account of their low temperatures, but the effects of tributaries freezing during boreal winter may mean transport capacities are hindered during colder seasons. Freezing rivers at high latitudes may also produce cryogenic carbonate (Lacelle, 2007; Thomazo et al., 2017), but it is difficult to predict the effects of this phenomenon on transport potentials. A potential benefit of liming high latitudes is that carbonate weathering is known to be rapid in sub-arctic climates, in contrast to silicate weathering. For example, carbonate weathering dominates the dissolved loads of poly-lithic basins such as the Mackenzie (Millot et al., 2003). In these cold environments enhancing the weathering of silicate rocks is less feasible as dissolution kinetics are slower (Pogge von Strandmann et al., 2022).
The main purpose of liming in the past has been to amend soil pH, which is often low on account of organic matter respiration in the soil environment generating rich pore waters. It is common for soils to have a pH of ~5.5 in temperate northern hemisphere climates (Tunney et al., 2010), whereas pH values in in tropical soils can be as low as 3.9 (Motavalli et al., 1995) due to higher rates of organic carbon respiration. Ideal soil pH values for agriculture vary with soil type, but generally neutral pH values (7) are required to grow most crops (Oshunsanya, 2019). Reacting the prescribed amount of calcite for each basin with the modeled soil waters in those basins results in modest increases in soil pH, to values sustainable for farming in most conditions (Figure 8). At lower temperatures it is plausible that liming could elevate soil pH to values too alkaline for growing traditional crops in those areas (Figure 8), and this must be recognized as a plausible side effect of using farmland to enhance carbonate weathering. However, it is worth noting that the elevation of soil water pH associated with enhanced carbonate weathering is much lower than that for enhanced silicate weathering, particularly in tropical basins (Köhler et al., 2010).
Figure 8. pH values for soil waters in each basin, after reaction with the prescribed amount of calcite to reach the saturation states required for Model 2 (SIc = 0) and Model 3 (SIc = 1).
Additional benefits of liming include increasing the availability of nutrients, such as nitrate and phosphate, by changing soil water pH (Haynes, 1982). Increasing nutrient availability reduces fertilizer requirements and increases soil health. Liming can also decrease the mobility of heavy metals in soil profiles, reducing the risk of plants incorporating heavy metals into soft tissues (Bolan and Duraisamy, 2003).
Farmland is one potential location to practice liming, though other land types may also be used - e.g., reforestation projects. This study focuses on farmland because i) many parts of North America already practice liming, and this has resulted in an increased export of bicarbonate in waters draining farmland (Oh and Raymond, 2006; Hamilton et al., 2007), and ii) global coverage of farmland is well quantified (e.g., GlobCover database Arino et al., 2008).
CO2 is consumed by enhancing carbonate weathering, provided that the agricultural lime is dissolved by carbonic acid. However, one caveat for enhancing carbonate weathering is a growing body of evidence suggesting that strong acids (e.g., H2SO4) play an important role in rock weathering in large river basins such as the Mekong and Mackenzie (e.g., Calmels et al., 2007; Torres et al., 2016; Relph et al., 2021). Agricultural fertilization can also result in the production of strong acids. Nitrification of nitrate-based fertilizers, used in agriculture, can produce nitric acid (HNO3) (Zbieranowski and Aherne, 2013). The reaction between strong acids (e.g., H2SO4 and HNO3) and CaCO3 results in CO2 release into the atmosphere.
Some modeling studies have assumed that agricultural liming acts as a direct source of CO2 (e.g., Houghton et al., 1997; West and McBride, 2005), and often account for the relatively long timescale process of carbonate precipitation in the ocean. Field trials have demonstrated that liming acts as a sink of CO2 (e.g., Oh and Raymond, 2006; Hamilton et al., 2007), with the reaction of lime and strong acids generally accounting for less than 15% of the charge balance in the soil and river waters (Oh and Raymond, 2006; Hamilton et al., 2007; Biasi et al., 2008). It appears, based on the evidence of field trials, that enhancing carbonate weathering on agricultural land would be a net sink of CO2, but the efficiency can be compromised by the strong acid reaction. The reduction in efficiency of enhancing carbonate weathering can be quantified by understanding how much excess nitrogen, derived from fertilizers, is in each catchment in this study (Supplementary material for calculation, Figure 9). Literature studies (Oh and Raymond, 2006; Hamilton et al., 2007; Biasi et al., 2008) stipulate that ~15% of this excess nitrate (Figure 9) would react with lime products. Assuming the reaction between strong acids and agricultural lime is:
Figure 9. Top: Excess nitrogen based fertiliser per catchment studied (Tonnes yr−1). Nitrogen excess data from Lassaletta et al. (2014), and the mass of nitrogen used is available from: https://www.fao.org/faostat/en/?dat. Black catchments occur for areas where nitrogen mining occurs, which skews estimates of nitrogen use efficiency. Bottom: PTCC values (Tonnes C yr−1) for Model 2, recalculated assuming 15% of excess nitrate in each catchment reacts with agricultural lime applied to aribal land.
Then Equation 9 stoichiometry dictates 1 mol of CO2 is released for every 2 mol of HNO3 reacted with CaCO3. Based on these assumptions the PTCC of Model 2 may decrease from 0.26 to 0.20 Gt C yr−1 (Figure 9) as a consequence of a strong acid reaction. This is assuming that the amount of lime required to reach equilibrium for Model 2 without accounting for a strong acid weathering is applied to the soil, such that strong acid reaction would result in equilibrium saturation with respect to calcite not being achieved. Areas worst affected are unsurprisingly those which have the greatest excess of nitrate (e.g., SE Asia, Figure 9), and qualitatively it is shown that the catchments affected in Figure 9 already have low or negative PTCC values in Figure 2. The PTCC calculated from Model 3 would change from 0.92 Gt C yr−1 to 0.86 Gt C yr−1, adjusting for the reaction of limestone with excess nitrate in soils. Overall, Strong acid weathering of agricultural lime may reduce the efficacy of enhanced carbonate weathering by 5–25% (Figure 9; Oh and Raymond, 2006; Hamilton et al., 2007; Biasi et al., 2008).
The obvious downside to enhanced carbonate weathering is that the dissolution of lime products by strong acids results in CO2 emission, in comparison to silicate weathering, where strong acid weathering only results in a reduction in CO2 draw down efficiency—if ocean alkalinity enhancement is the study aim (Köhler et al., 2010; Taylor et al., 2016). Pedogenic carbonate formation is the aim of other enhanced silicate weathering studies (e.g., Andrews and Taylor, 2019; Haque et al., 2019) because the residence time of pedogenic carbonates is estimated to be long (i.e., 104 years). Strong acid generation from fertilizers may significantly reduce the residence time of pedogenic carbonates, or stop formation altogether (Zamanian et al., 2016). Dissolution of pedogenic carbonates by strong acids would result in a rapid re-release of CO2 to the atmosphere. Indeed, strong acid weathering is an unknown variable in the agricultural enhanced weathering hypothesis and requires dedicated research with meticulous field trials for appropriate quantification of the impacts on carbon cycling.
To assess the practicality of agricultural liming at a global scale, the quantity of farmland in the 5 catchments with the largest SDC (Model 1) and PTCC values (Models 2 and 3) for each scenario were determined using the GlobCover database (Arino et al., 2008). The thickness of limestone powder needing to be spread on farmland annually was then calculated using the molar volume of CaCO3 (36.95 Mol cm3), the mass of limestone required to reach saturation, and the surface area of farmland in each basin. The results for all model scenarios are shown in Table 2.
Table 2. The calculated thickness of lime required to be spread on farmland each year to achieve the estimated SDC (soil dissolution capacity) for Model 1, and estimated PTCC (potential transport capacities of carbon) for Models 2 and 3.
To satisfy Model 1, which represents an equilibrium dissolution capacity of soils, with respect to calcite (SIc = 0), ~1–10 mm yr−1 of limestone powder is required to be spread on all farmland in each basin. This would saturate soil waters with respect to calcite. Typically, <20 mm yr−1 thickness of lime powder is required for rivers to reach SIc = 0 (Model 2) and SIc = 1 (Model 3), though the small amount of farmland in Siberia (i.e., Lena and Yenisey) could require >20 mm yr−1 of lime powder to achieve saturation (Table 2). The amount of lime powder required to achieve saturation or supersaturation with respect to calcite in the 149 rivers modeled is 4.0, 2.1, and 5.1 Gt CaCO3 yr−1 for Model scenarios 1, 2, and 3, respectively. As shown in Figure 9, it is likely the true amount of lime required to reach saturation (Model 2) or supersaturation (Model 3) may be more than this.
Though no database exists for lime powder availability globally, limestone is frequently quarried to create aggregate and cement. Limestone extraction during the 1990s was ~ 1 Gt yr−1 in the U.S. alone, and lime powder is produced as a by-product of this extraction and processing (Hudson et al., 1997; Rau et al., 2007). To satisfy the amount of limestone required for model scenarios in this study, U.S. limestone extraction would require either doubling (Model 2), or increasing five-fold (Models 1 and 3). Investigating sources of alkaline feedstocks for enhancing weathering is not the aim of this study, but it is useful to note that limestone powder is not the only available alkaline material to enhance carbonate weathering. Industrially produced cement, blast furnace slag, kiln dust, and slaked lime could all be used for enhancing weathering (Renforth, 2019). This is with a caveat that of these materials only slaked lime has long term field trials, meaning lime powder and slaked lime are the most attractive materials from an environmental perspective.
Rapid increases in atmospheric CO2 concentrations require that novel methods for carbon sequestration are developed before 2050. Enhancing the dissolution of carbonate rocks on land has been suggested as a potential strategy to remove appreciable amounts of atmospheric CO2 on the timescale of human life. Indeed, carbonates have been shown to react rapidly with atmospheric CO2, but require this dissolved carbon to be delivered to the ocean for sequestration on meaningful timescales (1,000s of years), and that strong acids are of minor importance for dissolution reactions. This study provides estimates for the amount of additional dissolved Earth's rivers can deliver to the oceans under two saturation scenarios—providing both an optimistic and pessimistic scenario for carbon sequestration via enhancing carbonate weathering.
In both transport simulations (Models 2 and 3) continental cratons (tropical South America and Africa and Siberia) were the ideal locations to enhance riverine export via liming, without precipitating authigenic carbonate minerals. This would be expected as these rivers are supply-limited, hence are dilute and often have high discharges (e.g., in tropical areas). A lack of farmland in Siberia may mean that alternative land types for enhancing carbonate weathering need to be used, e.g., shrub land or forests. The efficacy of enhanced weathering in these locations is not known, though the reactivity of carbonate phases could make them plausible land types for liming. High northern latitudes may be ideal places for enhancing carbonate weathering, as olivine dissolution rates in these climates are unfeasible to enhance silicate weathering on meaningful timescales. The amounts of limestone required for rivers to reach saturation in either scenario may be feasible at the large basin scale in comparison to dunite extraction, and alternatives to limestone powder (e.g., blast furnace slag, slaked lime) are available. Liming is also a well understood and relatively widely practiced agricultural technique already, meaning costs and benefits are known. The degree of liming to reach the saturation states proposed in this study results in practical increases in soil water pH in most cases.
Overall, increasing the saturation index of calcite in global rivers to either 0 or 1 via enhancing carbonate weathering will result in a 5–20% sequestration of increases in atmospheric C per year. These values come with the caveat that a weak carbonic acid must be the dissolving agent, as strong acids will release CO2 into the atmosphere. The impact of strong acid dissolution for any agricultural enhanced weathering study requires careful and dedicated field trials. Our model estimates align with literature results for the dissolution capacity of calcite in soils, but these data require that rivers globally maintain an oversaturated state with respect to calcite for successful CO2 sequestration in the global oceans. The plausibility of oversaturation is poorly understood on a global scale, and to develop a deeper understanding of the feasibility of enhanced carbonate weathering more study is needed to understand this phenomenon.
The original contributions presented in the study are included in the article/Supplementary material, further inquiries can be directed to the corresponding author.
WK contributed to the conception, modeling, and writing of this manuscript. ET contributed to the conception and writing of this manuscript. Both authors contributed to the article and approved the final submission.
This study was funded by a UKRI grant [NE/S007164/1]. WK acknowledges funding from NERC studentship NE/S007164/1. ET acknowledges funding from NERC grants NE/T007214/1, NE/P011659/1, and NE/M001865/1.
The authors declare that the research was conducted in the absence of any commercial or financial relationships that could be construed as a potential conflict of interest.
All claims expressed in this article are solely those of the authors and do not necessarily represent those of their affiliated organizations, or those of the publisher, the editors and the reviewers. Any product that may be evaluated in this article, or claim that may be made by its manufacturer, is not guaranteed or endorsed by the publisher.
We thank Emily Stevenson, Luke Bridgestock, Mike Bickle, and Alasdair Knight for helpful discussions and two anonymous reviewers.
The Supplementary Material for this article can be found online at: https://www.frontiersin.org/articles/10.3389/fclim.2022.928215/full#supplementary-material
Andrews, M. G., and Taylor, L. L. (2019). Combating climate change through enhanced weathering of agricultural soils. Elements 15, 253–258. doi: 10.2138/gselements.15.4.253
Archer, D (1996). A data-driven model of the global calcite lysocline. Glob. Biogeochem. Cycles 10, 511–526. doi: 10.1029/96GB01521
Archer, D., Kheshgi, H., and Maier-Reimer, E. (1997). Multiple timescales for neutralization of fossil fuel CO2. Geophys. Res. Lett. 24, 405–408. doi: 10.1029/97GL00168
Arino, O., Bicheron, P., Achard, F., Latham, J., Witt, R., and Weber, J.-L. (2008). The most detailed portrait of earth. Eur. Space Agency 136, 25–31. doi: 10.1109/IGARSS.2007.4423328
Astilleros, J., Fernández-Díaz, L., and Putnis, A. (2010). The role of magnesium in the growth of calcite: an afm study. Chem. Geol. 271, 52–58. doi: 10.1016/j.chemgeo.2009.12.011
Berner, E. K., and Berner, R. A. (2012). Global Environment: Water, Air, and Geochemical Cycles. Princeton, NJ: Princeton University Press.
Biasi, C., Lind, S. E., Pekkarinen, N. M., Huttunen, J. T., Shurpali, N. J., Hyvönen, N. P., et al. (2008). Direct experimental evidence for the contribution of lime to CO2 release from managed peat soil. Soil Biol. Biochem. 40, 2660–2669. doi: 10.1016/j.soilbio.2008.07.011
Bickle, M. J., Tipper, E., Galy, A., Chapman, H., and Harris, N. (2015). On discrimination between carbonate and silicate inputs to himalayan rivers. Am. J. Sci. 315, 120–166. doi: 10.2475/02.2015.02
Blagodatsky, S., and Smith, P. (2012). Soil physics meets soil biology: towards better mechanistic prediction of greenhouse gas emissions from soil. Soil Biol. Biochem. 47, 78–92. doi: 10.1016/j.soilbio.2011.12.015
Bolan, N. S., and Duraisamy, V. (2003). Role of inorganic and organic soil amendments on immobilisation and phytoavailability of heavy metals: a review involving specific case studies. Soil Res. 41, 533–555. doi: 10.1071/SR02122
Brantley, S. L., Kubicki, J. D., and White, A. F. (2008). Kinetics of Water-Rock Interaction. New York, NY: Springer.
Braun, J.-J., Ngoupayou, J. R. N., Viers, J., Dupre, B., Bedimo, J.-P. B., Boeglin, J.-L., et al. (2005). Present weathering rates in a humid tropical watershed: Nsimi, south cameroon. Geochim. Cosmochim. Acta 69, 357–387. doi: 10.1016/j.gca.2004.06.022
Caldeira, K., and Rau, G. H. (2000). Accelerating carbonate dissolution to sequester carbon dioxide in the ocean: geochemical implications. Geophys. Res. Lett. 27, 225–228. doi: 10.1029/1999GL002364
Calmels, D., Gaillardet, J., Brenot, A., and France-Lanord, C. (2007). Sustained sulfide oxidation by physical erosion processes in the mackenzie river basin: climatic perspectives. Geology. 35, 1003–1006. doi: 10.1130/G24132A.1
Calmels, D., Gaillardet, J., and François, L. (2014). Sensitivity of carbonate weathering to soil CO2 production by biological activity along a temperate climate transect. Chem. Geol. 390, 74–86. doi: 10.1016/j.chemgeo.2014.10.010
Chou, L., Garrels, R. M., and Wollast, R. (1989). Comparative study of the kinetics and mechanisms of dissolution of carbonate minerals. Chem. Geol. 78, 269–282. doi: 10.1016/0009-2541(89)90063-6
Connor, D. J., Loomis, R. S., and Cassman, K. G. (2011). Crop Ecology: Productivity and Management in Agricultural Systems. Cambridge: Cambridge University Press.
Dandurand, J., Gout, R., Hoefs, J., Menschel, G., Schott, J., and Usdowski, E. (1982). Kinetically controlled variations of major components and carbon and oxygen isotopes in a calcite-precipitating spring. Chem. Geol. 36, 299–315. doi: 10.1016/0009-2541(82)90053-5
Dobberschütz, S., Nielsen, M., Sand, K., Civioc, R., Bovet, N., Stipp, S., et al. (2018). The mechanisms of crystal growth inhibition by organic and inorganic inhibitors. Nat. Commun. 9, 1–6. doi: 10.1038/s41467-018-04022-0
Drever, J. I (1988). The Geochemistry of Natural Waters, Vol. 437. Englewood Cliffs, NJ: Prentice Hall.
Dreybrodt, W., Buhmann, D., Michaelis, J., and Usdowski, E. (1992). Geochemically controlled calcite precipitation by CO2 outgassing: field measurements of precipitation rates in comparison to theoretical predictions. Chem. Geol. 97, 285–294. doi: 10.1016/0009-2541(92)90082-G
Erlanger, E., Rugenstein, J., Bufe, A., Picotti, V., and Willett, S. (2021). Controls on physical and chemical denudation in a mixed carbonate-siliciclastic orogen. J. Geophys. Res. 126, e2021JF006064. doi: 10.1002/essoar.10505807.1
Fekete, B. M., Vörösmarty, C. J., and Grabs, W. (2002). High-resolution fields of global runoff combining observed river discharge and simulated water balances. Glob. Biogeochem. Cycles 16, 15–11. doi: 10.1029/1999GB001254
Fick, S. E., and Hijmans, R. J. (2017). Worldclim 2: new 1-km spatial resolution climate surfaces for global land areas. Int. J. Climatol. 37, 4302–4315. doi: 10.1002/joc.5086
Ford, T., and Pedley, H. (1996). A review of tufa and travertine deposits of the world. Earth Sci. Rev. 41, 117–175. doi: 10.1016/S0012-8252(96)00030-X
Friedlingstein, P., O'sullivan, M., Jones, M. W., Andrew, R. M., Hauck, J., Olsen, A., et al. (2020). Global carbon budget 2020. Earth Syst. Sci. Data 12, 3269–3340. doi: 10.5194/essd-12-3269-2020
Gaillardet, J., Calmels, D., Romero-Mujalli, G., Zakharova, E., and Hartmann, J. (2019). Global climate control on carbonate weathering intensity. Chem. Geol. 527, 118762. doi: 10.1016/j.chemgeo.2018.05.009
Gaillardet, J., Dupre, B., Allegre, C. J., and Négrel, P. (1997). Chemical and physical denudation in the amazon river basin. Chem. Geol. 142, 141–173. doi: 10.1016/S0009-2541(97)00074-0
Gaillardet, J., Dupré, B., Louvat, P., and Allegre, C. (1999). Global silicate weathering and CO2 consumption rates deduced from the chemistry of large rivers. Chem. Geol. 159, 3–30. doi: 10.1016/S0009-2541(99)00031-5
Galy, A., France-Lanord, C., and Derry, L. A. (1999). The strontium isotopic budget of himalayan rivers in nepal and bangladesh. Geochim. Cosmochim. Acta 63, 1905–1925. doi: 10.1016/S0016-7037(99)00081-2
Goldscheider, N (2005). Karst groundwater vulnerability mapping: application of a new method in the swabian alb, germany. Hydrogeol. J. 13, 555–564. doi: 10.1007/s10040-003-0291-3
GRDC. (2020). Major River Basins of the World / Global Runoff Data Centre, GRDC, 2nd Revised Edn. Koblenz: Federal Institute of Hydrology (BfG).
Griffioen, J (2017). Enhanced weathering of olivine in seawater: the efficiency as revealed by thermodynamic scenario analysis. Sci. Total Environ. 575, 536–544. doi: 10.1016/j.scitotenv.2016.09.008
Hamilton, S. K., Kurzman, A. L., Arango, C., Jin, L., and Robertson, G. P. (2007). Evidence for carbon sequestration by agricultural liming. Glob. Biogeochem. Cycles 21, 2738. doi: 10.1029/2006GB002738
Haque, F., Santos, R. M., Dutta, A., Thimmanagari, M., and Chiang, Y. W. (2019). Co-benefits of wollastonite weathering in agriculture: CO2 sequestration and promoted plant growth. ACS Omega 4, 1425–1433. doi: 10.1021/acsomega.8b02477
Hartmann, J., Lauerwald, R., and Moosdorf, N. (2014). A brief overview of the global river chemistry database, GLORICH. Procedia Earth Planet. Sci. 10, 23–27. doi: 10.1016/j.proeps.2014.08.005
Hartmann, J., West, A. J., Renforth, P., Köhler, P., De La Rocha, C. L., Wolf-Gladrow, D. A., et al. (2013). Enhanced chemical weathering as a geoengineering strategy to reduce atmospheric carbon dioxide, supply nutrients, and mitigate ocean acidification. Rev. Geophys. 51, 113–149. doi: 10.1002/rog.20004
Haynes, R (1982). Effects of liming on phosphate availability in acid soils. Plant Soil. 68, 289–308. doi: 10.1007/BF02197935
Herman, J. S., and Lorah, M. M. (1987). CO2 outgassing and calcite precipitation in falling spring creek, virginia, usa. Chem. Geol. 62, 251–262. doi: 10.1016/0009-2541(87)90090-8
Houghton, J. T., Filho, L. G. M., Lim, B., Treanton, K., and Mamaty, I. (1997). Revised 1996 IPCC Guidelines for National Greenhouse Gas Inventories. v. 1: Greenhouse gas Inventory Reporting Instructions.-v. 2: Greenhouse Gas Inventory Workbook.-v. 3: Greenhouse Gas Inventory Reference Manual. Bracknell.
Hudson, W. R., Little, D. N., Razmi, A. M., Anderson, V., and Weissmann, A. J. (1997). An investigation of the status of by-product fines in the united states. Technical report, University of Texas at Austin. International Center for Aggregates Research.
Ilstedt, U., Nordgren, A., and Malmer, A. (2000). Optimum soil water for soil respiration before and after amendment with glucose in humid tropical acrisols and a boreal mor layer. Soil Biol. Biochem. 32, 1591–1599. doi: 10.1016/S0038-0717(00)00073-0
Kelemen, P. B., Matter, J., Streit, E. E., Rudge, J. F., Curry, W. B., and Blusztajn, J. (2011). Rates and mechanisms of mineral carbonation in peridotite: natural processes and recipes for enhanced, in situ CO2 capture and storage. Annu. Rev. Earth Planet. Sci. 39, 545–576. doi: 10.1146/annurev-earth-092010-152509
Köhler, P., Hartmann, J., and Wolf-Gladrow, D. A. (2010). Geoengineering potential of artificially enhanced silicate weathering of olivine. Proc. Natl. Acad. Sci. U.S.A. 107, 20228–20233. doi: 10.1073/pnas.1000545107
Lacelle, D (2007). Environmental setting, (micro) morphologies and stable c-o isotope composition of cold climate carbonate precipitatesa review and evaluation of their potential as paleoclimatic proxies. Quat. Sci. Rev. 26, 1670–1689. doi: 10.1016/j.quascirev.2007.03.011
Lackner, K. S., Wendt, C. H., Butt, D. P., Joyce Jr, E. L., and Sharp, D. H. (1995). Carbon dioxide disposal in carbonate minerals. Energy 20, 1153–1170. doi: 10.1016/0360-5442(95)00071-N
Lassaletta, L., Billen, G., Grizzetti, B., Anglade, J., and Garnier, J. (2014). 50 year trends in nitrogen use efficiency of world cropping systems: the relationship between yield and nitrogen input to cropland. Environ. Res. Lett. 9, 105011. doi: 10.1088/1748-9326/9/10/105011
Lauerwald, R., Laruelle, G. G., Hartmann, J., Ciais, P., and Regnier, P. A. (2015). Spatial patterns in CO2 evasion from the global river network. Glob. Biogeochem. Cycles 29, 534–554. doi: 10.1002/2014GB004941
Lloyd, J., and Taylor, J. (1994). On the temperature dependence of soil respiration. Funct. Ecol. 8, 315–323. doi: 10.2307/2389824
Mann, S (1988). Molecular recognition in biomineralization. Nature 332, 119–124. doi: 10.1038/332119a0
Marion, G. M., Mironenko, M. V., and Roberts, M. W. (2010). Frezchem: a geochemical model for cold aqueous solutions. Comput. Geosci. 36, 10–15. doi: 10.1016/j.cageo.2009.06.004
Meybeck, M., and Ragu, A. (2012). GEMS-GLORI World River Discharge Database. Paris: Laboratoire de Géologie Appliquée; Université Pierre et Marie Curie. doi: 10.1594/PANGAEA.804574
Millot, R., érôme Gaillardet, J., Dupré, B., and Allègre, C. J. (2003). Northern latitude chemical weathering rates: clues from the mackenzie river basin, canada. Geochim. Cosmochim. Acta 67, 1305–1329. doi: 10.1016/S0016-7037(02)01207-3
Montserrat, F., Renforth, P., Hartmann, J., Leermakers, M., Knops, P., and Meysman, F. J. (2017). Olivine dissolution in seawater: implications for CO2 sequestration through enhanced weathering in coastal environments. Environ. Sci. Technol. 51, 3960–3972. doi: 10.1021/acs.est.6b05942
Moosdorf, N., Renforth, P., and Hartmann, J. (2014). Carbon dioxide efficiency of terrestrial enhanced weathering. Environ. Sci. Technol. 48, 4809–4816. doi: 10.1021/es4052022
Motavalli, P., Palm, C., Parton, W., Elliott, E., and Frey, S. (1995). Soil ph and organic c dynamics in tropical forest soils: evidence from laboratory and simulation studies. Soil Biol. Biochem. 27, 1589–1599. doi: 10.1016/0038-0717(95)00082-P
Narbarte-Hernandez, J., Iriarte, E., Carrancho-Alonso, Á., Olazabal-Uzkudun, A., Rad, C., Arriolabengoa, M., et al. (2021). Geochemical fingerprint of agricultural liming as a regular management practice in modern-period basque farming. Sci. Total Environ. 787, 147525. doi: 10.1016/j.scitotenv.2021.147525
Oh, N.-H., and Raymond, P. A. (2006). Contribution of agricultural liming to riverine bicarbonate export and CO2 sequestration in the ohio river basin. Glob. Biogeochem. Cycles. 20, 2265. doi: 10.1029/2005GB002565
Oshunsanya, S (2019). Soil pH for Nutrient Availability and Crop Performance. London: IntechOpen. doi: 10.5772/68057
Parkhurst, D. L., and Appelo, C. (2013). Description of input and examples for phreeqc version 3 computer program for speciation, batch-reaction, one-dimensional transport, and inverse geochemical calculations. US Geol. Survey Techn. Methods 6, 497. doi: 10.3133/tm6A43
Plummer, L. N., Parkhurst, D. L., and Wigley, T. M. L. (1979). Critical review of the kinetics of calcite dissolution and precipitation. Chem. Modell. Aqueous Syst. 93, 537–573.
Pogge von Strandmann, P. A., Tooley, C., Mulders, J. J., and Renforth, P. (2022). The dissolution of olivine added to soil at 4◦C: Implications for enhanced weathering in cold regions. Front. Climate 8, 827698. doi: 10.3389/fclim.2022.827698
Rau, G. H., and Caldeira, K. (1999). Enhanced carbonate dissolution:: a means of sequestering waste CO2 as ocean bicarbonate. Energy Convers. Manag. 40, 1803–1813. doi: 10.1016/S0196-8904(99)00071-0
Rau, G. H., Knauss, K. G., Langer, W. H., and Caldeira, K. (2007). Reducing energy-related CO2 emissions using accelerated weathering of limestone. Energy 32, 1471–1477. doi: 10.1016/j.energy.2006.10.011
Raymond, P. A., Hartmann, J., Lauerwald, R., Sobek, S., McDonald, C., Hoover, M., et al. (2013). Global carbon dioxide emissions from inland waters. Nature 503, 355–359. doi: 10.1038/nature12760
Relph, K. E., Stevenson, E. I., Turchyn, A. V., Antler, G., Bickle, M. J., Baronas, J. J., et al. (2021). Partitioning riverine sulfate sources using oxygen and sulfur isotopes: implications for carbon budgets of large rivers. Earth Planet Sci. Lett. 567, 116957. doi: 10.1016/j.epsl.2021.116957
Renforth, P (2019). The negative emission potential of alkaline materials. Nat. Commun. 10, 1401. doi: 10.1038/s41467-019-09475-5
Renforth, P., von Strandmann, P. P., and Henderson, G. (2015). The dissolution of olivine added to soil: implications for enhanced weathering. Appl. Geochem. 61, 109–118. doi: 10.1016/j.apgeochem.2015.05.016
Ridgwell, A., and Hargreaves, J. (2007). Regulation of atmospheric CO2 by deep-sea sediments in an earth system model. Glob. Biogeochem. Cycles 21, 2764. doi: 10.1029/2006GB002764
Rigopoulos, I., Harrison, A. L., Delimitis, A., Ioannou, I., Efstathiou, A. M., Kyratsi, T., et al. (2018). Carbon sequestration via enhanced weathering of peridotites and basalts in seawater. Appl. Geochem. 91, 197–207. doi: 10.1016/j.apgeochem.2017.11.001
Rogerson, M., Pedley, H., Kelham, A., and Wadhawan, J. (2014). Linking mineralisation process and sedimentary product in terrestrial carbonates using a solution thermodynamic approach. Earth Surface Dyn. 2, 197–216. doi: 10.5194/esurf-2-197-2014
Romero-Mujalli, G., Hartmann, J., and Börker, J. (2019a). Temperature and CO2 dependency of global carbonate weathering fluxes-implications for future carbonate weathering research. Chem. Geol. 527, 118874. doi: 10.1016/j.chemgeo.2018.08.010
Romero-Mujalli, G., Hartmann, J., Börker, J., Gaillardet, J., and Calmels, D. (2019b). Ecosystem controlled soil-rock CO2 and carbonate weathering-constraints by temperature and soil water content. Chem. Geol. 527, 118634. doi: 10.1016/j.chemgeo.2018.01.030
Roy, S., Gaillardet, J., and Allegre, C. (1999). Geochemistry of dissolved and suspended loads of the seine river, france: anthropogenic impact, carbonate and silicate weathering. Geochim. Cosmochim. Acta 63, 1277–1292. doi: 10.1016/S0016-7037(99)00099-X
Schuiling, R., and Krijgsman, P. (2006). Enhanced weathering: an effective and cheap tool to sequester CO2. Clim. Change 74, 349–354. doi: 10.1007/s10584-005-3485-y
Schuiling, R., and Tickell, O. (2010). Enhanced weathering of olivine to capture CO2. J. Appl. Geochem. 12, 510–519.
Shi, Z., Allison, S. D., He, Y., Levine, P. A., Hoyt, A. M., Beem-Miller, J., et al. (2020). The age distribution of global soil carbon inferred from radiocarbon measurements. Nat. Geosci. 13, 555–559. doi: 10.1038/s41561-020-0596-z
Taylor, L. L., Quirk, J., Thorley, R., Kharecha, P. A., Hansen, J., Ridgwell, A., et al. (2016). Enhanced weathering strategies for stabilizing climate and averting ocean acidification. Nat. Clim. Chang 6, 402–406. doi: 10.1038/nclimate2882
Thomazo, C., Buoncristiani, J.-F., Vennin, E., Pellenard, P., Cocquerez, T., Mugnier, J. L., et al. (2017). Geochemical processes leading to the precipitation of subglacial carbonate crusts at bossons glacier, mont blanc massif (french alps). Front. Earth Sci. 5, 70. doi: 10.3389/feart.2017.00070
Tipper, E. T., Bickle, M. J., Galy, A., West, A. J., Pomiès, C., and Chapman, H. J. (2006). The short term climatic sensitivity of carbonate and silicate weathering fluxes: insight from seasonal variations in river chemistry. Geochim. Cosmochim. Acta 70, 2737–2754. doi: 10.1016/j.gca.2006.03.005
Tipper, E. T., Gaillardet, J., Galy, A., Louvat, P., Bickle, M., and Capmas, F. (2010). Calcium isotope ratios in the world's largest rivers: a constraint on the maximum imbalance of oceanic calcium fluxes. Glob. Biogeochem. Cycles 24, 3574. doi: 10.1029/2009GB003574
Torres, M. A., West, A. J., Clark, K. E., Paris, G., Bouchez, J., Ponton, C., et al. (2016). The acid and alkalinity budgets of weathering in the andes-amazon system: Insights into the erosional control of global biogeochemical cycles. Earth Planet Sci. Lett. 450, 381–391. doi: 10.1016/j.epsl.2016.06.012
Trumbore, S. E., Chadwick, O. A., and Amundson, R. (1996). Rapid exchange between soil carbon and atmospheric carbon dioxide driven by temperature change. Science 272, 393–396. doi: 10.1126/science.272.5260.393
Tunney, H., Sikora, F., Kissel, D., Wolf, A., Sonon, L., and Goulding, K. (2010). A comparison of lime requirements by five methods on grassland mineral soils in ireland. Soil Use Manag. 26, 126–132. doi: 10.1111/j.1475-2743.2010.00263.x
West, A. J., Galy, A., and Bickle, M. (2005). Tectonic and climatic controls on silicate weathering. Earth Planet Sci. Lett. 235, 211–228. doi: 10.1016/j.epsl.2005.03.020
West, T. O., and McBride, A. C. (2005). The contribution of agricultural lime to carbon dioxide emissions in the united states: dissolution, transport, and net emissions. Agric. Ecosyst. Environ. 108, 145–154. doi: 10.1016/j.agee.2005.01.002
White, A. F., and Brantley, S. L. (2018). Chemical Weathering Rates of Silicate Minerals. Berlin; Boston, MA: De Gruyter. doi: 10.1515/9781501509650
Yan, H., Liu, Z., and Sun, H. (2020). Large degrees of carbon isotope disequilibrium during precipitation-associated degassing of CO2 in a mountain stream. Geochim. Cosmochim.Acta 273, 244–256. doi: 10.1016/j.gca.2020.01.012
Zamanian, K., Pustovoytov, K., and Kuzyakov, Y. (2016). Pedogenic carbonates: Forms and formation processes. Earth Sci. Rev. 157, 1–17. doi: 10.1016/j.earscirev.2016.03.003
Zbieranowski, A. L., and Aherne, J. (2013). Ambient concentrations of atmospheric ammonia, nitrogen dioxide and nitric acid in an intensive agricultural region. Atmos Environ. 70, 289–299. doi: 10.1016/j.atmosenv.2013.01.023
Zeng, S., Kaufmann, G., and Liu, Z. (2022a). Natural and anthropogenic driving forces of carbonate weathering and the related carbon sink flux: a model comparison study at global scale. Glob. Biogeochem. Cycles 36, e2021GB007096. doi: 10.1029/2021GB007096
Keywords: carbonate weathering, soil pCO2, carbon sequestration, rivers, enhanced weathering in soils, calcite dissolution capacity
Citation: Knapp WJ and Tipper ET (2022) The efficacy of enhancing carbonate weathering for carbon dioxide sequestration. Front. Clim. 4:928215. doi: 10.3389/fclim.2022.928215
Received: 25 September 2022; Accepted: 23 June 2022;
Published: 11 August 2022.
Edited by:
Jens Hartmann, University of Hamburg, GermanyReviewed by:
Rafael Mattos Dos Santos, University of Guelph, CanadaCopyright © 2022 Knapp and Tipper. This is an open-access article distributed under the terms of the Creative Commons Attribution License (CC BY). The use, distribution or reproduction in other forums is permitted, provided the original author(s) and the copyright owner(s) are credited and that the original publication in this journal is cited, in accordance with accepted academic practice. No use, distribution or reproduction is permitted which does not comply with these terms.
*Correspondence: William J. Knapp, d2prMjdAY2FtLmFjLnVr
Disclaimer: All claims expressed in this article are solely those of the authors and do not necessarily represent those of their affiliated organizations, or those of the publisher, the editors and the reviewers. Any product that may be evaluated in this article or claim that may be made by its manufacturer is not guaranteed or endorsed by the publisher.
Research integrity at Frontiers
Learn more about the work of our research integrity team to safeguard the quality of each article we publish.