- 1Research Center for Carbon Solutions, Heriot-Watt University, Edinburgh, United Kingdom
- 2The Climate Map, Brooklyn, NY, United States
- 3Department of Biological Engineering and Department of Brain and Cognitive Sciences, MIT, Cambridge, MA, United States
- 4Department of Media Arts and Sciences, MIT, Cambridge, MA, United States
- 5Department of Neurosciences, University of California, San Diego, San Diego, CA, United States
- 6Howard Hughes Medical Institute, Chevy Chase, MD, United States
- 7McGovern Institute, Department of Brain and Cognitive Sciences, Media Arts and Sciences, Department of Biological Engineering, Center for Neurobiological Engineering, K. Lisa Yang Center for Bionics, Koch Institute, MIT, Cambridge, MA, United States
Over the previous two decades, a diverse array of geochemical negative emissions technologies (NETs) have been proposed, which use alkaline minerals for removing and permanently storing atmospheric carbon dioxide (CO2). Geochemical NETs include CO2 mineralization (methods which react alkaline minerals with CO2, producing solid carbonate minerals), enhanced weathering (dispersing alkaline minerals in the environment for CO2 drawdown) and ocean alkalinity enhancement (manipulation of ocean chemistry to remove CO2 from air as dissolved inorganic carbon). CO2 mineralization approaches include in situ (CO2 reacts with alkaline minerals in the Earth's subsurface), surficial (high surface area alkaline minerals found at the Earth's surface are reacted with air or CO2-bearing fluids), and ex situ (high surface area alkaline minerals are transported to sites of concentrated CO2 production). Geochemical NETS may also include an approach to direct air capture (DAC) that harnesses surficial mineralization reactions to remove CO2 from air, and produce concentrated CO2. Overall, these technologies are at an early stage of development with just a few subjected to field trials. In Part I of this work we have reviewed the current state of geochemical NETs, highlighting key features (mineral resources; processes; kinetics; storage durability; synergies with other NETs such as DAC, risks; limitations; co-benefits, environmental impacts and life-cycle assessment). The role of organisms and biological mechanisms in enhancing geochemical NETs is also explored. In Part II, a roadmap is presented to help catalyze the research, development, and deployment of geochemical NETs at the gigaton scale over the coming decades.
Highlights
- Geochemical NETs (in situ, ex situ and surficial carbon mineralization, enhanced weathering, ocean alkalinity enhancement, etc.) are extensively reviewed.
- The potential role of biotechnology in geochemical NETs is given special focus.
- The Review (Part I) is accompanied by a Roadmap (Part II) to help catalyze development of geochemical NETs at scale in the coming decades.
Introduction
To meet the 2°C climate target set out in the Paris Agreement, the atmospheric concentration of CO2-equivalent (CO2eq) should not exceed 450 ppm (or 430 ppm for the 1.5°C target) (Spier, 2020). To achieve this, a drastic decrease in anthropogenic emissions is required, which can be achieved through the expansion of renewable energy generation and lower emissions from land-use and land-use-change. The International Energy Agency (IEA) estimates that carbon capture and storage (CCS), i.e., capturing and storing CO2 before it is released to the atmosphere, may prevent upwards of 6 Gt CO2 yr.−1 by 2050 (Haszeldine et al., 2018). In addition, negative emissions technologies (NETs) may also need to remove 10 Gt CO2 yr.−1 by 2050, and 20 Gt CO2 yr.−1 by the end of the century (National Academies of Sciences, Engineering, and Medicine, 2019). These targets necessitate technologies capable of capturing, removing, and storing CO2 at a large scale.
Carbon can be stored as organic materials, e.g., terrestrial vegetation, ocean biomass, and biochar, or as pure CO2 deep underground in sedimentary rocks. However, the permanence of these storage media vary greatly, creating uncertainty and legacy issues for industry, policymakers, and regulators (Lackner, 2003). On the other hand, carbon can be permanently stored in the form of the carbonate anion () in solid minerals, e.g., calcium carbonate (CaCO3) and magnesium carbonate (MgCO3), or in the form of dissolved bicarbonate () in ocean water. These forms of storage can be achieved by three main groups of technologies, commonly referred to as CO2 mineralization, enhanced weathering, and ocean alkalinity enhancement, collectively referred to here as “geochemical NETs.”
Most geochemical NETs involve enhancing the reactions of alkaline minerals with CO2 (and H2O), mimicking natural chemical weathering reactions of silicate rocks at the Earth's surface, which removes ~1.1 Gt CO2 yr.−1 from the atmosphere, primarily stored as ocean bicarbonate (Strefler et al., 2018). The goal of geochemical NETs is to add considerably to this natural removal rate as a tool to combat climate change. In the last two decades, and particularly during the past few years, research on geochemical NETs has grown considerably, with many novel approaches being explored. Several companies and projects have been recently established. Though some are already operating at the kiloton (kt) scale, as a group they are, by and large, at an early stage of their development, with just a few at the pilot scale.
In Part I of this work, geochemical NETs are reviewed and their potential impacts and limitations discussed. In Part II, a set of projects and interventions that warrant prioritization are presented in the form of a roadmap with the aim of catalyzing the development and deployment of geochemical NETs at the scale necessary to achieve significant carbon removal.
Overview of Geochemical NETs
A geochemical NET is any technology which involves the use of substantial amounts of alkaline minerals in its flowsheet and involves enhancing the reaction of CO2 and mineral alkalinity for the purpose of safely removing and storing CO2 from the atmosphere as stable carbonate minerals, or dissolved ocean bicarbonate. At a fundamental level, most geochemical NETs are simply an acid-base neutralization of the form given in Equation (1).
Figure 1 conceptualizes how sources of CO2 and mineral alkalinity can be combined, giving rise to various geochemical NETs. In order for such a technology to be carbon negative, it must remove significantly more CO2 than it emits from its life-cycle (Fajardy and Mac Dowell, 2017). Therefore, CO2 must be removed directly or indirectly from the atmosphere, typically using renewable energy or bioenergy, rather than fossil fuel energy.
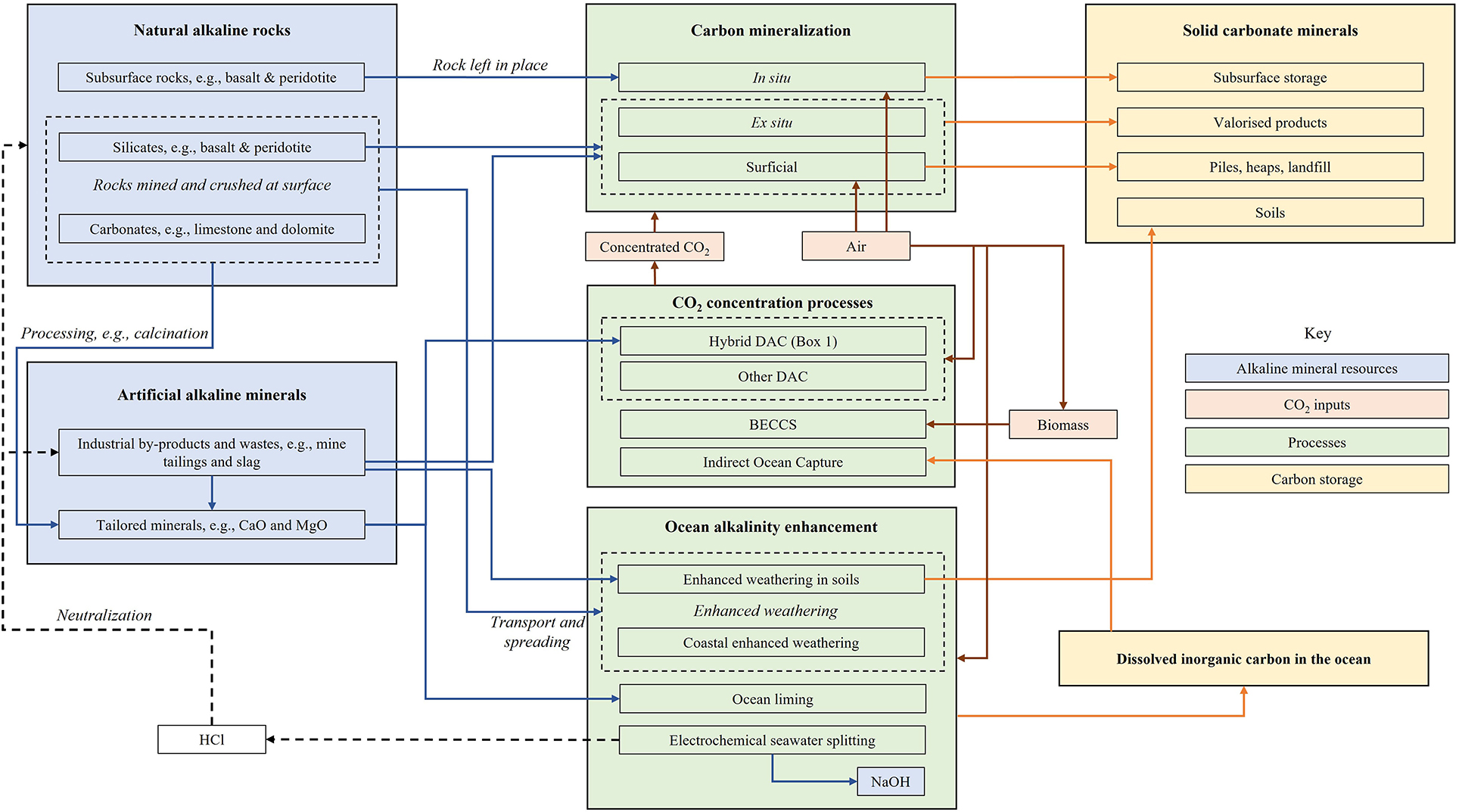
Figure 1. Conceptualizing many of the possibilities for geochemical NETs by combinations of CO2 and alkaline minerals.
In order to create solid carbonate minerals, CO2 (and H2O) must react with alkaline minerals (Table 1). Alkaline minerals are simply any natural or artificial mineral that is rich in alkaline earth metals (second column of the periodic table), particularly magnesium (Mg) or calcium (Ca), since these are far more abundant than strontium or barium, etc. (which also form stable carbonate minerals). Common Ca- and Mg-rich minerals are given in section the Common Alkaline Minerals. These minerals are found in natural igneous, metamorphic, and sedimentary rocks (section Naturally Occurring Alkaline Rocks) as well as in industrial by-products and wastes such as mine tailings, cement kiln dusts, fly ash, slag, desalination brines, etc., or minerals tailored for purpose (section Artificial Alkaline Minerals—Industrial By-Products and Wastes, and Tailored Minerals and Table 2). Abundant silicate minerals rich in alkali metals (first column of the periodic table), in particular sodium and potassium, may be able to contribute in some geochemical NETs, but their carbonates are too soluble for long-term carbon sequestration. While other elements may also form carbonate minerals (e.g., cadmium, cobalt, copper, iron, lead, manganese, nickel, uranium, zinc) their abundance, stability, or toxicity limit their large-scale reaction with CO2.
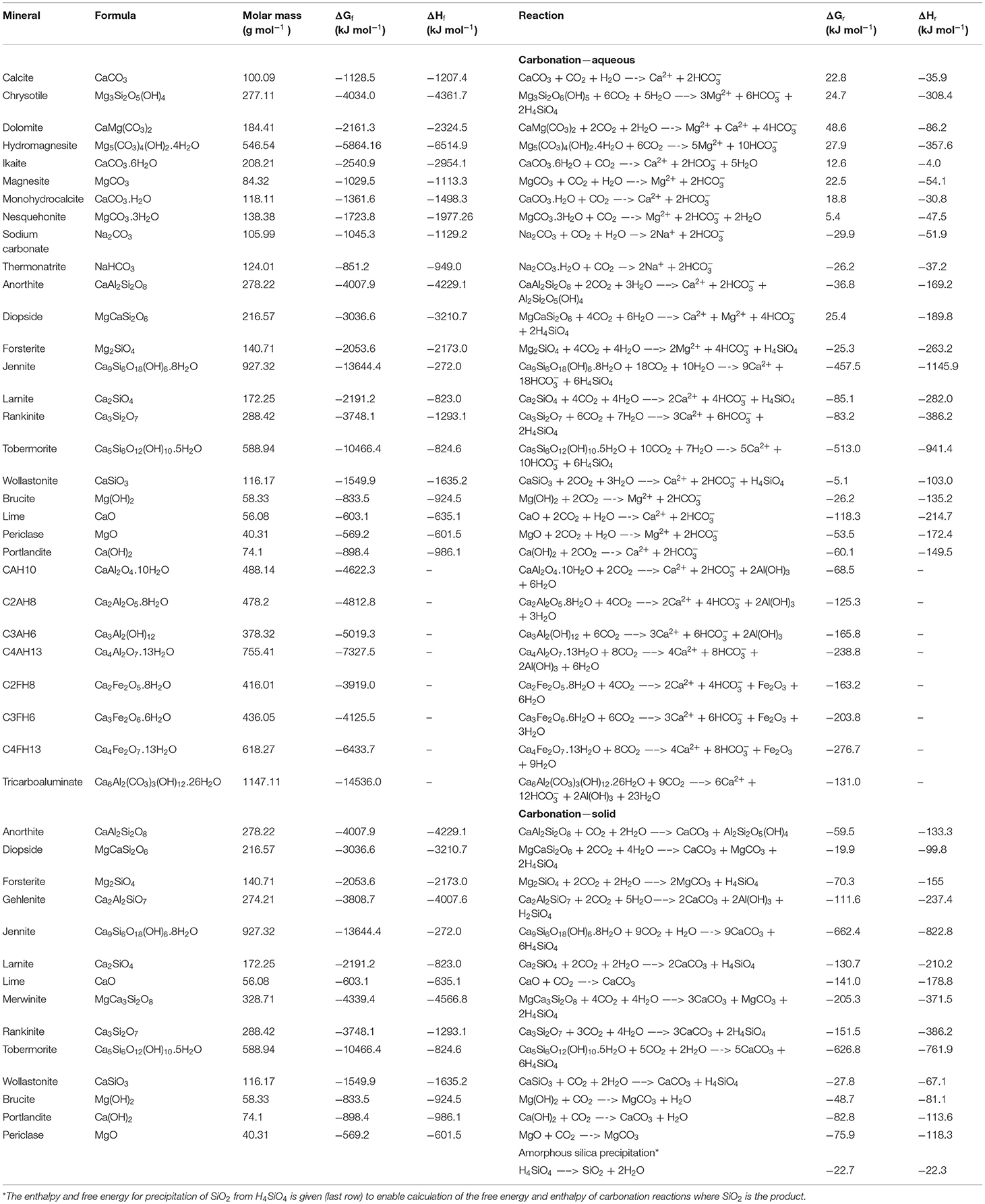
Table 1. Mg-, Ca-, Na- silicate, oxide, hydroxide, aluminate, carbonate, etc., minerals typically encountered in geochemical NETs, with their Gibbs free energies (ΔGf) and enthalpies (ΔHf) of formation (Robie and Hemingway, 1995), and in some cases their Gibbs free energies (ΔGr) of reaction with H2O and CO2, at 1 atm and 25°C [calculated using data from Robie and Hemingway (1995)].
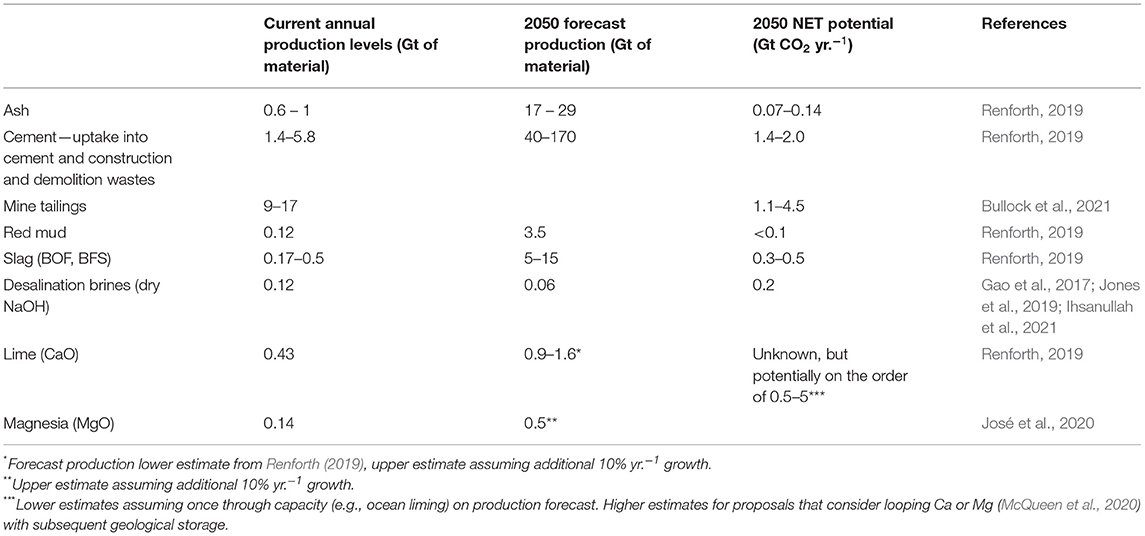
Table 2. Current production values for some common artificial minerals used in geochemical NETs, with estimated production values and NET potentials by 2050.
Most of the reactions between CO2, H2O and mineral alkalinity (section Reaction Chemistry) are thermodynamically favorable, as indicated by their negative Gibbs free energies. The result of these reactions is either a solid carbonate mineral (section Carbonate Products and Other Secondary Minerals), or dissolved ocean bicarbonate. However, owing to kinetic limitations, the reaction between rock outcrops containing natural alkaline minerals and CO2 at ambient conditions occurs on geological timescales. Therefore, the main goal of geochemical NETs is to considerably enhance the rate of these reactions to a timescale relevant to climate change mitigation by manipulating the kinetics (section Kinetics).
Those geochemical NETs which predominantly produce solid carbonate minerals (section CO2 Mineralization), can be divided conceptually into in situ, ex situ and surficial CO2 mineralization. In situ approaches typically involve circulation of CO2-rich fluids through alkaline rocks, e.g., basalt or peridotite, in the Earth's subsurface (Matter and Kelemen, 2009). Ex situ approaches typically involve reacting high concentration CO2 with finely ground natural alkaline minerals or artificial alkaline by-products/wastes in engineered reactors. These reactions typically go to completion within minutes using high temperatures, pressures, concentrated CO2 and/or other reagents such as acids (Sanna and Maroto-valer, 2016). On the other hand, surficial approaches typically involve reaction of air, or CO2-bearing fluids/gases, with ground minerals at the Earth's surface, occurring more slowly than ex situ reactions. Examples include reactions of natural minerals in controlled environments like greenhouses (Myers and Nakagaki, 2020), or in heaps or piles of artificial wastes such as slags (Stolaroff et al., 2005) or mine tailings (Wilson et al., 2006, 2009). In both ex situ and surficial CO2 mineralization, the carbonate products may be valorized (sold or utilized), whereas in in situ approaches the mineralized CO2 is safely and permanently stored underground. Surficial approaches may also be harnessed for the purpose of cost-effective direct air capture (DAC) (Box 1).
Box 1. Harnessing surficial mineralization processes for direct air capture.
Direct Air Capture (DAC) requires the removal of CO2 from the air to produce a concentrated source of CO2. This concentrated CO2 can then either be utilized or permanently stored. Surficial mineralization processes have been proposed that fit this definition. For example calcium oxide (Hanak et al., 2017; Hanak and Manovic, 2018) or magnesium oxide (McQueen et al., 2020) looping systems. Calcium looping is a pre- or post-combustion CO2 capture technology which uses high temperatures. Carbonation is usually performed at around 650°C to form calcium carbonate (CaCO3). The carbonates, while still hot, are then fed into the next part of the system where they are calcined above 900°C. The calcination step regenerates the lime and produces a more concentrated source of CO2 which is suitable for CCS (Martínez et al., 2018). Hybrid surficial DAC works similarly, except that CaO or MgO is carbonated under ambient conditions in air, at potentially unlimited scale. The carbonation step in air has slower reaction kinetics than calcium looping processes and is thought to be dependent on a relative humidity above 55% (Erans et al., 2020; Samari et al., 2020). For further details on these and other DAC systems see (Sanz-Pérez et al., 2016; Okesola et al., 2018; McQueen et al., 2021a).
Other geochemical NETs involve the dispersing of alkaline minerals for the purpose of enhanced weathering in large open spaces, exploiting certain environmental conditions. Where the reactive medium is soil, this is referred to as enhanced weathering in soil, or terrestrial enhanced weathering (section Enhanced Weathering in Soils) (Schuiling and Krijgsman, 2006). Where the weathering takes place at beaches and coastal shelves, the method is referred to as coastal enhanced weathering (Montserrat et al., 2017). Coastal enhanced weathering is one approach for ocean alkalinity enhancement (OAE), which is any process that involves increasing alkalinity in the oceans, resulting in atmospheric CO2 removal. Other methods for OAE include ocean liming (Caserini et al., 2021), and a range of electrochemical processes (House et al., 2007; Davies, 2015; Mustafa et al., 2020). The role of alkaline minerals in ocean-based NETs is discussed in the section Ocean Alkalinity Enhancement. Key features of the different geochemical NETs are summarized in Table 3. Finally, biological mechanisms that influence geochemical reactions or transport ions may potentially be integrated into many of the above-mentioned methods to improve efficiency (section Application of Biotechnology to Geochemical NETs).
Alkaline Mineral Resources
Common Alkaline Minerals
A mineral is an inorganic solid with distinctive chemical and physical properties, composition, and atomic structure, whereas rocks are an assemblage of minerals. In geochemical NETs, the alkalinity for the neutralization reaction (Equation 1) is usually supplied by abundant Ca- and Mg-rich silicate (or aluminosilicate) minerals, and in some cases the oxides, hydroxides or carbonates of calcium and magnesium (see Table 1). Potential material resources for magnesium-bearing minerals are much larger than that of calcium owing to their natural availability, while on the other hand, markets for magnesium-based products are much smaller than that of calcium-based products. Minerals rich in other cations such as Na, K, Fe are also considered in some approaches (Kheshgi, 1995; Palandri and Kharaka, 2005; Campbell, 2019).
Naturally Occurring Alkaline Rocks
Alkaline minerals are found in alkaline rocks, including: (i) igneous rocks, such as basalt and peridotite (McGrail et al., 2006; Kelemen and Matter, 2008; Matter and Kelemen, 2009; Clark, 2019; Kelemen et al., 2020), (ii) metamorphic rocks, such as serpentinites (Okamoto et al., 2006; Power et al., 2013b; Bide et al., 2014; Dichicco et al., 2015), and (iii) sedimentary rocks such as limestone and dolomite (Rau and Caldeira, 1999; Rau et al., 2007; Rau, 2011). There are two main types of igneous and metamorphic alkaline rock considered for geochemical NETs: (i) mafic rocks such as basalt, and (ii) ultramafic rocks such as peridotite and serpentinite. Mafic and ultramafic rocks are chemically and physically distinct. For example, mafic rocks typically contain 15–28% MgO, 1–15% CaO, and 46–54% SiO2 (among other minor components), whereas ultramafic rocks typically contain 35–46% MgO, 5–15% CaO, and 42–48% SiO2 (Sen, 2014). Depending on the particular geochemical NET, some rock types might be more suitable than others, e.g., olivine may be more promising than basalt in enhanced weathering approaches. For in situ mineralization, both mafic (e.g., basalt) and ultramafic (e.g., peridotite and serpentinite) formations with suitable properties, such as high porosities and permeabilities, will allow for cost-effective storage. Together, mafic and ultramafic rocks represent over 90 teratonnes (Tt) of resources, sufficient to store the equivalent of 700-years worth of global CO2 emissions (Bide et al., 2014). For ex situ and surficial mineralization, as well as for enhanced weathering, near-surface deposits of mafic and ultramafic alkaline rocks could be mined, crushed, and ground to create high surface areas to facilitate a reasonable rate of reaction with CO2. In this regard, the available rock resources that could be used for geochemical NETs at the Earth's surface are plentiful, since the estimates for global sand, gravel, and stone reserves amount to more than 190 Tt (Sverdrup et al., 2017). If only a small part of this industry were to be redirected toward production of crushed alkaline rocks for surface geochemical NETs, then many Gt CO2 yr.−1 would be achieved in the near future. The reason is that a robust and expanding industry is already in place, i.e., the construction aggregates industry, which annually extracts and processes 50 billion tons (Gt) of rocks (range 47–59 Gt) (Sverdrup et al., 2017). Finally, it may be possible to use natural carbonate rocks for enhanced weathering and remove CO2 from air in the form of bicarbonate (Kirchner et al., 2020).
Artificial Alkaline Minerals—Industrial By-Products and Wastes, and Tailored Minerals
In addition to naturally occurring mineral resources, some geochemical NETs can also exploit abundant artificial mineral resources (Table 2). These are typically wastes or by-products of industrial processes, landscaping, or quarrying (Dijkstra et al., 2019). On a global scale, it is estimated that 7 Gt of these alkaline mineral by-products/wastes are produced annually, with a combined potential to capture and store CO2 away from the atmosphere at 2.9–8.5 Gt yr.−1 by 2100 (Renforth, 2019). More specifically, these materials include: (i) iron and steelmaking slags (blast furnace, basic oxide, electric arc furnace, ladle furnace, and argon oxygen decarburization slags) (Mayes et al., 2018; Pullin et al., 2019; Reddy et al., 2019; Luo and He, 2021); (ii) cement wastes (cement and concrete wastes, construction and demolition wastes, cement kiln/bypass dust, recycled calcium sulfates, and blended hydraulic slag cement) (Huntzinger et al., 2009a; Medas et al., 2017; Pedraza et al., 2021); (iii) ashes and relevant residues [bottom ash from furnaces and incinerators (municipal solid waste incinerator bottom ash, fly ash, boiler ash, coal slag, oil shale ash), air pollution control residues (cyclone dust, cloth bag dust), and fuel combustion ashes (coal fly ash, lignite fly ash, oil shale, biomass ashes)] (Alba et al., 2001; Baciocchi et al., 2006; Sun et al., 2008; Zhang et al., 2008; Montes-Hernandez et al., 2009; Prigiobbe et al., 2009; Lombardi et al., 2016; Brück et al., 2018; Liu et al., 2018; Ji et al., 2019; Vassilev et al., 2021); (iv) mine and mineral processing wastes (asbestos tailings, nickel tailings, diamond tailings, and red mud) (Wilson et al., 2010, 2014; Power et al., 2014, 2020; Gras et al., 2017; Mervine et al., 2018); (v) alkaline paper mill wastes (lime kiln residues, green liquor dreg, paper sludge) (Pérez-López et al., 2008; Sun et al., 2013; Li and Sun, 2014; Spínola et al., 2021); and (vi) reject brines from desalination (Mustafa et al., 2020). The latter can be employed by electrochemical approaches that aim at removing acidity (HCl) from seawater and return alkalinity (NaOH). Currently, more than 95 million m3 of desalinated water is produced daily on a global scale, which is responsible for generating more than 141 million m3 of brine each day that is typically discharged into the oceans, often negatively affecting the receiving ecosystems (Jones et al., 2019). This number is on the rise, since recent estimates suggest that by 2030 the global desalination capacity will be more than 200 million m3 day−1 (Ihsanullah et al., 2021), while this number could be more than tripled by 2050 since the total global desalination population is projected to increase by 3.2-fold in 2050 compared to the present (Gao et al., 2017). These very large volumes of reject brines (waste) present certain advantages for OAE, since their mean salinity is twice that of seawater (Ihsanullah et al., 2021), suggesting that if they were used for OAE, CO2 removal at the Mt yr.−1 scale at least could be achieved in the nearterm.
Regarding the solid alkaline waste materials, these are generally low-cost (Huijgen et al., 2005) and often deposited in heaps or buried at the shallow subsurface, implying that these are more accessible and more readily available than natural minerals. Furthermore, most legacy deposits may be only partially weathered, suggesting their great potential for CO2 removal. For example, 40–140 years after deposition, a slag deposit in Consett, England, which is estimated to be over 30 Mt, has only reached ~3% of its CO2 sequestration potential (Pullin et al., 2019). Artificial alkaline minerals also tend to have higher reactivities than natural minerals, due to their activation by various industrial pre-treatments (e.g., grinding and heat treatment), which often create high surface areas and higher crystal disorder (La Plante et al., 2021a). However, compared to natural alkaline rocks, they are less abundant and may contain more labile toxic metals, possibly making their use problematic in large-scale geochemical NETs.
Therefore, rather than using wastes and by-products of existing industrial processes, artificial alkaline minerals, tailored for the purpose of negative emissions, could be more promising. For example, the carbonates of calcium and magnesium can be calcined, the CO2 generated by their decomposition could be captured and stored, while the resulting high-reactivity oxides (CaO and MgO) could be used in different NETs such as power generation using an integrated solid-oxide fuel cell (Hanak et al., 2017), metal oxide looping DAC (see Box 1) (McQueen et al., 2020), or ocean liming applications (Renforth and Kruger, 2013; Renforth et al., 2013). Substances other than CaO and MgO have also been investigated for hybrid DAC systems, such as sodium and potassium oxides, and related compounds (Nikulshina et al., 2008; Campbell, 2019).
Reaction Chemistry
Reactions of alkaline minerals with CO2 can occur as gas-solid systems (e.g., Equation 2).
Where X is Mg or Ca. Humidity is usually required to catalyze these reactions. See section Gas-Solid Kinetics for discussion on the kinetics of gas-solid reactions.
Alternatively, and more commonly, reactions of alkaline silicate minerals with CO2 occur in the aqueous phase. First, CO2 dissolves in water forming carbonic acid (H2CO3), which releases acidity, H+, into solution:
Alkaline mineral surfaces then react with H+:
Over time, carbonate minerals may precipitate:
Overall the reaction is:
Theoretically, 1 mol of CO2 is removed for every 1 mol of alkaline metal. Similar reactions can occur for a wide array of alkaline silicate minerals (Table 1). In some geochemical NETs, such as coastal enhanced weathering, the goal is to remove carbon and store it as dissolved ocean bicarbonate, rather than minerals:
In this case, 2 mol of CO2 are theoretically removed for every 1 mol of alkaline metal (example reactions are given in Table 1). The residence time of bicarbonate is tens to hundreds of thousands of years in the ocean and thus it can be considered a stable store of carbon since abiotic mineral carbonate formation is kinetically inhibited by the ocean's chemistry (Renforth and Henderson, 2017). Alkaline carbonates can also be used to remove CO2:
In this instance, 1 mol of CO2 is theoretically removed for every 1 mol of alkaline metal, assuming the carbon is stored as ocean bicarbonate.
Some geochemical NETs do not react alkaline minerals with CO2 or H2CO3 directly, but instead react alkaline minerals with other acids, which are by-products or wastes of other NETs. For example, electrochemical seawater dialysis may produce HCl (House et al., 2007; Davies, 2015):
Where NaOH is used for ocean alkalinity enhancement and CO2 removal:
And where HCl is disposed of by reaction with alkaline minerals.
Furthermore, some geochemical NETs capture CO2 from air and produce concentrated CO2 gas in a looping process (McQueen et al., 2020):
The concentrated CO2 stream can then be used or safely stored geologically. This process may be possible with minerals other than Ca- and Mg- oxides.
Carbonate Products and Other Secondary Minerals
Solid products of geochemical NETs primarily include carbonate minerals such as calcite (CaCO3), magnesite (MgCO3), dolomite (CaMg(CO3)2), and various hydrated magnesium carbonates (Mg5(CO3)4(OH)2·nH2O). These are stable enough to be stored for long time periods. Other carbonate minerals such as siderite (FeCO3), dawsonite (NaAl(CO3)(OH)2), and ankerite (Ca(Fe,Mg,Mn)(CO3)2) can act as stores of carbon, but may only be stable in subsurface environments (Hellevang et al., 2005; Snæbjörnsdóttir et al., 2014; Yu et al., 2020).
Besides carbonates, other products of mineral carbonation and weathering include silica, iron oxides, and clays. These secondary minerals, including the carbonate products, can occlude reactive surfaces, halting further reaction (Béarat et al., 2006; Andreani et al., 2009; Maher et al., 2009; Saldi et al., 2013; Sissmann et al., 2014). The role of clay mineral formation via “reverse weathering” (Equation 16) is a subject of ongoing debate within several geochemical NETs, as these reactions may inhibit their CO2 sequestration efficiencies (Montserrat et al., 2017; Oelkers et al., 2018; Renforth and Campbell, 2021).
Where X is a cation such as Mg2+ or Ca2+. Successful geochemical NETs will likely include approaches for avoiding or minimizing the extent and impact of secondary minerals.
Kinetics
The field of kinetics involves the study of reaction rates, and provides the basis for reactor design and system optimization. Although conversion of alkaline minerals into carbonates is thermodynamically favored in the presence of CO2, the reactions are kinetically inhibited. To become an effective tool for climate change mitigation, conversion rates must be enhanced considerably. Table 3 summarizes some common enhancements. In geochemical NETs, there are many competing effects, and trade-offs will be required. For example, maintaining a low pH can significantly increase the dissolution rate of silicate minerals but will limit the formation of carbonate minerals, while elevated temperatures favor mineral dissolution and carbonate precipitation, they also lead to lower CO2 solubility. These competing effects are particularly relevant to direct carbonation. Separating dissolution and precipitation allows each process to be optimized independently (indirect carbonation). See section Ex situ for more details on direct vs. indirect approaches. Generally speaking, most geochemical NETs are CO2-mineral-water systems that can be divided into gas-solid (section Gas-Solid Kinetics), or aqueous (section Aqueous Phase Kinetics). In the latter, three main processes occur: (i) mineral dissolution (section Mineral Dissolution); (ii) CO2 dissolution and hydration (section CO2 Dissolution and Hydration); and (iii) precipitation of carbonate minerals (section Carbonate Precipitation). Biological influences on kinetics are discussed separately in the section Application of Biotechnology to Geochemical NETs.
Gas-Solid Kinetics
Gas-solid kinetics are relevant to ex situ (Baciocchi et al., 2009) and surficial (Myers and Nakagaki, 2020) CO2 mineralization, as well as DAC (McQueen et al., 2020). These systems operate with gaseous (humidity), rather than liquid water, thus avoiding significant leaching of potentially toxic metals (El-Naas et al., 2015). The reaction of spherical particles of natural and artificial alkaline minerals with CO2 is usually limited by ion diffusion through a growing product layer, a process often described by a shrinking core model such as in Equation (17) (Yagi and Kunii, 1955).
where t is time (s), ρsolid is the molar density of Ca or Mg in the solid phase (mole m−3 of mineral), r is the particle radius (m), d is the thickness of the product layer (m), Cgas is the CO2 concentration in the gas phase (moles m−3 of gas), and D is the carbonate ion diffusivity through the product layer (m2 s−1). The ion diffusivity has an Arrhenius temperature dependence, thus increasing temperature increases carbonation rate (Li, 2020). According to Equation (17), using pure CO2 rather than ambient air increases the reaction rate by 3 orders of magnitude whereas grinding from 10 mm to 10 μm increases mineralization rates by 6 orders of magnitude. Values of D for relevant minerals can vary across 7 orders of magnitude depending on the mineral composition and structure (Myers et al., 2019). Other kinetic enhancements are possible for gas-solid processes. For example, in fluidized bed processes, the use of a nanosilica additive increased the gas-solids contact efficiency and carbonation rates of Ca(OH)2 (Pontiga et al., 2013), while attrition has been shown to prevent the build-up of passivating product layers, improving CO2 uptake by CaO (Chen et al., 2012).
Humidity also plays a crucial role in gas-solid approaches. For example, the rate and extent of reaction between portlandite (Ca(OH)2) and CO2(g) (60–90°C) was found to increase significantly with increasing humidity, proposed to be due to the rate limiting step of dissolution of Ca(OH)2 in adsorbed surface water (Shih et al., 1999). For brucite (Mg(OH)2), dehydroxylation/rehydroxylation processes have been shown to induce morphological changes, including translamellar cracking and delamination, that can serve to enhance carbonation reactivity via disruption of the passivating product layer (McKelvy et al., 2001; Fagerlund et al., 2012). Humidity is found to have similar mechanistic effects on gas-solid carbonation of natural silicate minerals such as wollastonite (CaSiO3) (Longo et al., 2015) and chrysotile (Mg3Si2O5(OH)4) (Larachi et al., 2010, 2012), mine tailings (Veetil and Hitch, 2020), and industrial alkaline by-products/wastes such as air pollution control residue (Baciocchi et al., 2006), fly ash (Liu et al., 2018) and calcium silicate hydrates found in hydrated portland cement (Steiner et al., 2020).
Aqueous Phase Kinetics
Mineral Dissolution
Mineral dissolution is the degradation of a solid mineral in aqueous media, with the subsequent release of soluble species such as Mg2+/Ca2+ and H4SiO4 (e.g., Equation 6). The rate of carbon sequestration in geochemical NETs is often limited by the mineral dissolution rate. Mineral dissolution can be described by the rate law:
where SA is the reactive surface area, k0 is the standard rate constant, EA/RT is apparent activation energy divided by the gas constant, R, and temperature, T, ai is the activity of aqueous species i to the power of n, and f(ΔG) is a function of the Gibbs free energy change (see Lasaga, 1998; Black et al., 2015 for more detail).
As implied in Equation (18), the rate of dissolution is directly proportional to the reactive surface area (Brantley and Mellott, 2000). For earth-surface geochemical NETs that make use of rocks, crushing and milling is needed to increase reactive surface areas (Haug et al., 2010; Moosdorf et al., 2014; Rigopoulos et al., 2016). For subsurface mineralization, high vesicularity basalts provide large reactive surface areas (Galeczka et al., 2014; Xiong et al., 2018). Temperature also plays an important role in mineral dissolution, since the rate constant in Equation (18) greatly depends on temperature and even small increases in the temperature will largely increase mineral dissolution rates. As a result, in ex situ approaches mineral dissolution rates are often enhanced through temperature increase (Gerdemann et al., 2007). For in situ approaches, greater depths are prioritized, since the naturally warmer underground temperatures will greatly enhance carbonation rates, by up to 76 times compared to ambient surface rocks (Paukert et al., 2012). Indeed, fully carbonated peridotites (listevenites) give a good indication of the enormous potential of carbonation of ultramafic rocks at high temperatures (Falk and Kelemen, 2015). Finally, for enhanced weathering at the Earth's surface, warm tropical regions are typically prioritized, since in these areas mineral dissolution rates are greatly accelerated (Kohler et al., 2010).
The composition of the aqueous phase also plays an important role. For example, the dissolution rates of minerals such as forsterite and apatite increase linearly with decreasing pH (Brantley, 2008). However, others may show a non-linear dependence, for instance albite has a parabola-shaped dependence, having a minimum at pH ~5 (Gislason et al., 2014). In some geochemical NETs, carbonic acid provides the acidity needed to enhance dissolution (see Equation 3) (O'Connor et al., 2000; Kanakiya et al., 2017) while in others, organic and inorganic acids will provide acidity (van Hees et al., 2000; Kakizawa et al., 2001; Teir et al., 2007a,b). Organic acids can also catalyze silicate dissolution by acting as chelators, which complex and solubilize cations in the mineral crystal framework (Drever and Stillings, 1997; Lazo et al., 2017; Oelkers et al., 2018). Inorganic ligands, such as sulfate and phosphate (Pokrovsky et al., 2005) may also enhance mineral dissolution.
Dissolution rates are also dependent on the solids' composition. In silicate minerals, the dissolution rate is controlled by breaking of the shortest and strongest (usually the Si–O) bonds. Thus, minerals with a low degree of silica polymerization (e.g., olivine) dissolve at faster rates than minerals with higher degrees (e.g., quartz) (Goldich, 1938). For this reason, artificially tailored minerals such as MgO and CaO exhibit much faster dissolution rates than silicates, making them good candidates for OAE (Kheshgi, 1995; Renforth and Henderson, 2017). Furthermore, calcium-rich minerals dissolve faster than their magnesium-rich counterparts, owing to the comparatively weaker Ca–O bond (Brantley, 2008). The presence of transition metals and their potential for reduction–oxidation (redox) reactions, particularly Fe and Mn, can have a substantial impact on dissolution rates, e.g., Fe(III)–O bond is stronger than Fe(II)–O bond, suggesting that reductive conditions could increase mineral dissolution rates (Brantley, 2008). Silicates tend to dissolve non-stoichiometrically, i.e., the ratio of release rates for the various species is not equal to the stoichiometry of the starting mineral, often because their most soluble elements, e.g., Na, K, Ca, Mg, are released preferentially (Brantley, 2008). Such incongruent dissolution may lead to the formation of a silica-rich outer layer on the particle surfaces, inhibiting further dissolution (Béarat et al., 2006; Andreani et al., 2009; Maher et al., 2009; Saldi et al., 2013; Sissmann et al., 2014). Surfaces can also be passivated by precipitating secondary minerals which limit diffusion of reactants and products (King et al., 2010). Agitation and sonication have been employed to reduce the impact of surface passivation (Santos and Van Gerven, 2011). Organisms can also prevent secondary mineral precipitation by secreting organic chelators (Liermann et al., 2000; Buss et al., 2007; Torres et al., 2019).
Figure 2 shows the CO2 removal potential via enhanced weathering (mineral dissolution) for the most relevant alkaline minerals contained within various types of mine tailings over a 50-year period (Bullock et al., 2022). The removal potential is determined according to weathering (Equation 6) via a shrinking core model under two conditions: “unimproved” (pH of 6–8, and common grain sizes for these materials, e.g., 75 μm for platinum group metal (PGM) tailings) and “improved” (pH of 3–4, and grain sizes of 10 μm for all types). Tailings containing high abundances of olivine, serpentine and clinopyroxene show the highest CO2 removal potential due to their favorable kinetics. The rates of CO2 removal are estimated to become substantially augmented using improved conditions. Specifically, Bullock et al. (2022) estimate that the average annual global CO2 removal potential of tailings weathered over 2030–2100 to be ~93 (unimproved conditions) to 465 (improved conditions) Mt CO2 yr.−1. These data clearly demonstrate the enormous impact that enhancing the reaction kinetics can have on the CO2 removal potential of alkaline materials.
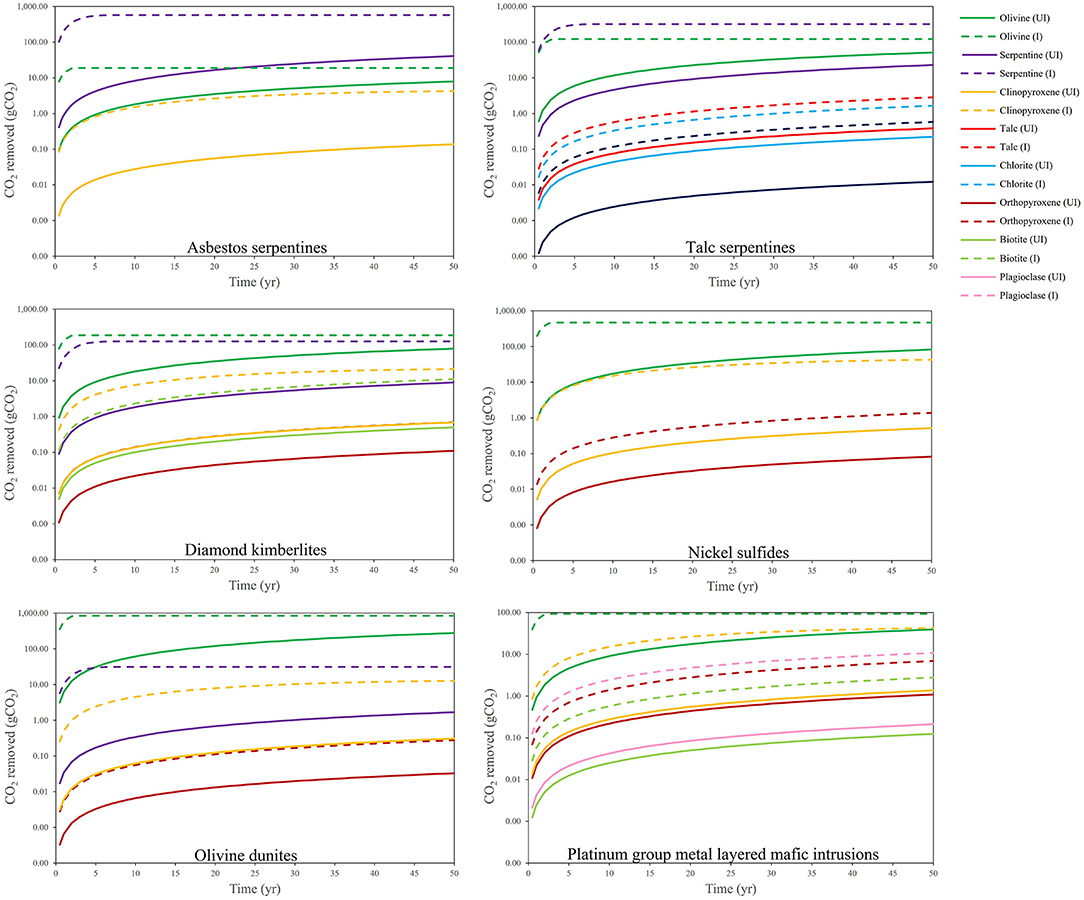
Figure 2. CO2 removal potential by enhanced weathering of a theoretical 1 kg of different mine tailings materials over a 50 yr time period, modeled using a shrinking core model. Minerals typically contained within each tailings material are shown (abundance based on modal mineralogy, with non-reactive or untargeted minerals not included) under unimproved (UI) (pH of 6–8, and common grain sizes for these materials, e.g., 75 μm for PGM tailings) and improved (I) (pH of 3–4, and grain sizes of 10 μm for all types) (Bullock et al., 2022).
CO2 Dissolution and Hydration
In low and neutral pH aqueous solutions CO2 will react with water and form carbonic acid, H2CO3, with deprotonation to form bicarbonate, , and carbonate, (Equations 3–5) (Knoche, 1980). As the pH increases, the equilibrium shifts further to the right, increasing the concentrations of and . At higher pH values (>8) the CO2 reaction mechanism changes, with forming directly via the much faster reaction (Morel and Hering, 1993):
This behavior is the essence behind many geochemical NETs which use dissolved mineral alkalinity as the driving force for CO2 capture and sequestration. However, in some geochemical NETs, mineral dissolution is not the limiting factor, but rather they are constrained by the CO2 availability, particularly where atmospheric air is the CO2-bearing gas (Power et al., 2013b; Gras et al., 2017). According to Henry's Law, doubling the CO2 partial pressure approximately doubles the CO2 solubility (Henry and Banks, 1803). Increasing CO2 partial pressure can be achieved by increasing the total pressure or by increasing the gas phase CO2 concentration (O'Connor et al., 2000, 2001). In some geochemical NETs, CO2 is artificially pre-concentrated by DAC or bioenergy with carbon capture and storage (BECCS) (Kelemen et al., 2020). Others exploit the Earth's natural mechanisms for pre-concentrating CO2. For example, in enhanced weathering in soils, the weathering rate of the applied alkaline minerals is accelerated due to elevated concentrations of CO2 in the soil pores, which traces back to microbial/plant respiration (Robbins, 1986), while some ocean NETs may take advantage of the higher carbon concentration by volume of seawater compared to air (de Lannoy et al., 2018). Furthermore, diffusion of CO2 into the aqueous phase can be artificially accelerated through bubbling (Legendre and Zevenhoven, 2017; Abe et al., 2021), stirring (Gadikota, 2020), spraying solution in scrubbing towers (Gunnarsson et al., 2018), spraying fluids rich in mineral dissolution products (Stolaroff et al., 2005), sparging (Kelemen et al., 2020), or by using thin films of alkaline solution trickled over high surface area packing materials (Keith et al., 2018). The rate of hydration of CO2 into carbonic acid can be catalyzed by the enzyme carbonic anhydrase (CA) (Lindskog, 1997) (section The Influence of Organisms and Biological Mechanisms on the Chemical Reactions Underlying Geochemical NETs). In coastal enhanced weathering, the CO2 concentration from surface to seabed is constantly resupplied since the shallow, high-energy coasts enable rapid sea-air mixing and equilibration. Other factors might also influence CO2 dissolution rates. For example, CO2 solubility in aqueous solutions decreases with increasing temperature, as related via the temperature dependence of Henry's coefficient (Carroll et al., 1991), and also with increasing salinity due to the “salting out effect” (Setschenow, 1889; Yasunishi and Yoshida, 1979).
Carbonate Precipitation
Most geochemical NETs require production of dry solid carbonate minerals, and in some of these approaches carbonate precipitation is the rate-limiting step. For example, in OAE, precipitation of carbonate minerals reduces the efficiency of the overall sequestration by release of CO2:
In general, precipitation occurs when the aqueous medium is oversaturated with respect to the mineral that precipitates, i.e., the ionic activity product is higher than the equilibrium constant; whereas dissolution occurs when the aqueous medium is undersaturated with respect to these minerals (Brantley, 2008). For calcium carbonate precipitation (Equation 7), the stoichiometric solubility product, , is defined by:
where [Ca2+]sat and []sat are the equilibrium concentrations of each species in a solution saturated with CaCO3 (at a specific temperature, pressure, and salinity). The saturation state, Ω, is then defined as:
where [Ca2+] and [] are the concentrations of each species in solution. If Ω = 1, the solution is at equilibrium with the mineral phase. If Ω < 1, the aqueous phase is undersaturated, and calcium carbonate is expected to dissolve, whereas if Ω > 1 then the aqueous phase is oversaturated, and calcium carbonate is expected to precipitate. However, even when saturated, carbonate minerals may not always precipitate. For example, the presence of is known to inhibit calcium carbonate formation (Morse et al., 2007). Similarly, organic and inorganic ligands present in the aqueous phase can inhibit calcium carbonate formation via complexation and adsorption (Morse et al., 2007), although some enhance the precipitation rates by accelerating desolvation kinetics (Schott et al., 2009). The rate of carbonate precipitation increases with increasing pH, owing to the shift of aqueous equilibrium toward (Ruiz-Agudo et al., 2011). Higher temperatures and pressures result in greater precipitation of calcium carbonate and (hydrated) magnesium carbonates (Zeebe and Wolf-Gladrow, 2001; Hänchen et al., 2008). In turbulent conditions, eddy formation increases the diffusion rates of species enhancing carbonate precipitation (Dreybrodt et al., 1997). Precipitation of CaCO3 is ~4 orders of magnitude faster than precipitation of MgCO3, as Ca2+ is much larger than Mg2+ and the water molecules in its coordination sphere are held more loosely, enabling faster exchange with carbonate (Schott et al., 2009). Notably, microorganisms have been observed to catalyze the nucleation of MgCO3 (McCutcheon et al., 2019). Microorganisms can catalyze nucleation of carbonate precipitation by concentrating cations near the surfaces of cell walls or extracellular polymeric substances (Dupraz et al., 2009).
Processes
Processes for geochemical NETs that produce solid carbonate minerals via CO2 mineralization include in situ, ex situ and surficial approaches. Processes which increase the ocean's storage capacity of dissolved inorganic carbon (DIC) are termed OAE and include coastal enhanced weathering, electrochemical seawater splitting, and ocean liming. Enhanced weathering in soils produces both carbonate minerals (carbonation) and also lead to ocean alkalization since cations from rock dissolution will remain dissolved in water and eventually be transferred to the oceans (Renforth, 2012; Lefebvre et al., 2019). Table 3 summarizes some of the defining features of each of these processes. Furthermore, geochemical NETs which use alkaline minerals in their flowsheets but which do not necessarily result in carbon storage are introduced in Box 1. Note that descriptions of geochemical NETs, e.g., CO2 mineralization, enhanced weathering, OAE, etc., in other sources may vary from the ones in this review, and that their defining features may overlap in one or more ways.
CO2 Mineralization
In situ
In CCS, CO2 is typically injected as a pure supercritical fluid into geological formations, such as deep sedimentary formations, salt mines, depleted oil fields, or unmineable coal seams, where it becomes trapped in rock pores and structural spaces, with minimal CO2 mineralization. An impermeable caprock is needed in order to limit leakage and long-term monitoring is required (Zhang and Song, 2014). In in situ mineralization, the focus is on mineral storage, rather than pore and structural storage. This is achieved by injecting supercritical CO2, or CO2-rich fluids, into alkaline geological rock formations. Once injected, the CO2 creates a low pH zone within the rock, enhancing dissolution of the surrounding silicate minerals and causing Mg2+ and Ca2+ to be released (Equation 6). As mineral dissolution increases, the pH begins to increase, which in turn induces the precipitation of stable carbonate minerals (Equation 7). With mineralization there is less need for long-term monitoring of the storage efficacy compared to traditional forms of CCS, particularly when CO2 is pre-dissolved prior to injection. However, pre-dissolution incurs additional cost and complexity compared to injection of pure supercritical CO2 (Blondes et al., 2019).
As mentioned above, there are two main types of alkaline rock formation suitable for in situ mineralization: (i) mafic rocks such as basalt, and (ii) ultramafic rocks such as peridotite and serpentinite. Due to their higher alkalinity, ultramafic rocks have greater potential for CO2 mineralization per cubic volume of rock, while their exothermic reaction with CO2 releases larger amounts of heat which is beneficial for the mineralization reaction kinetics (National Academies of Sciences, Engineering, and Medicine, 2019). However, ultramafic rocks are usually found at greater depths, are less porous and permeable, and have a wider range of crystal size than mafic rocks (Kelemen and Matter, 2008). For both, capacity is large (section Naturally Occurring Alkaline Rocks), and these rocks are widely geographically distributed (Pilorgé et al., 2021). For example, extensive reserves of onshore flood basalts exist in the US, India, and Russia. However, most of the potential lies offshore, as the majority of the seafloor is composed of basalt. Although most peridotite is deeply buried, near-surface deposits can be found in locations such as Oman, United Arab Emirates, the Mediterranean, the Pacific Islands and New Zealand.
There are four main approaches to negative emissions via in situ mineralization which are based on the rock types and engineering methods employed, with two of them being already demonstrated at the kt CO2 yr−1 removal scale (Figure 3). In the first (i) approach, CO2 is injected into porous mafic rock formations, e.g., basalt, as a supercritical fluid. An impermeable caprock is needed to minimize CO2 leakages. If the rocks are not already water-saturated, then some water can be co-injected alongside the supercritical CO2 to facilitate mineralization. This has been successfully demonstrated in 2013 by the Wallula project, whereby 977 tons of water-saturated supercritical CO2 from the industry were injected into a permeable Columbia River basalt at a depth of 900 m over a 3-week period (McGrail et al., 2011, 2014, 2017a,b; Spane et al., 2012; White et al., 2020; Holliman et al., 2021). Although CO2 mineralization was observed in sidewall core samples, evidence suggests that much of the CO2 remains structurally trapped (White et al., 2020; Holliman et al., 2021).
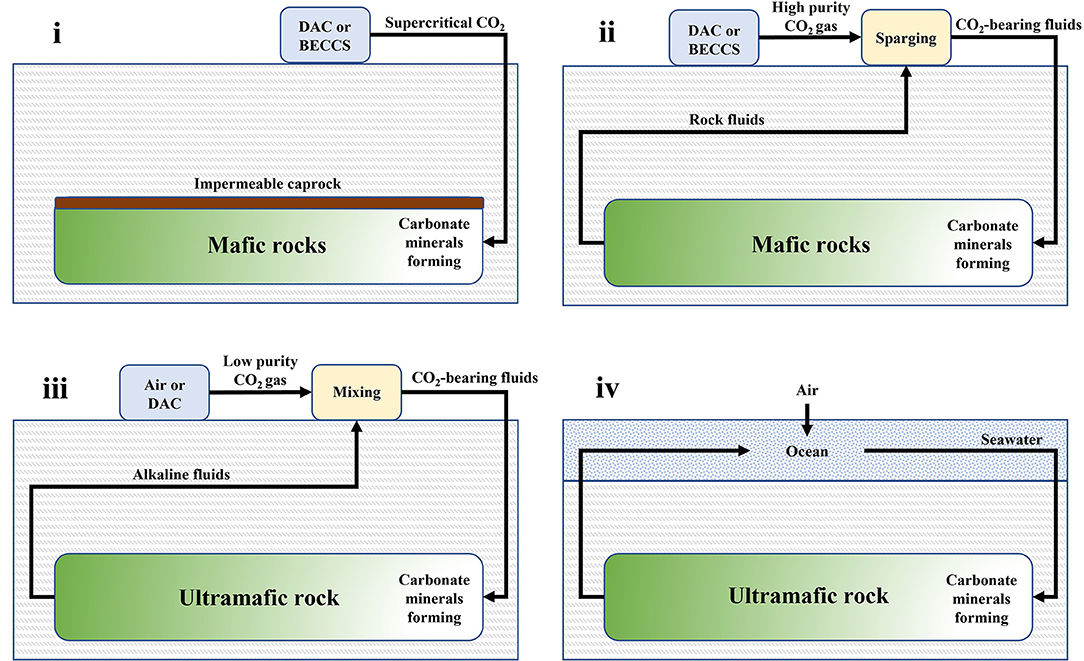
Figure 3. Schematics of four negative emissions approaches for in situ mineralization, i.e., (i) water-charged supercritical CO2 injection into mafic rock; (ii) CO2-bearing fluids circulated through mafic rock; (iii) CO2-bearing fluids circulated through ultramafic rock; and (iv) seawater circulation through ultramafic rock.
In the second (ii) approach, an impermeable caprock is not present and thus pre-dissolution of CO2 in reservoir fluids or seawater is required, prior to injection into porous basalt. Recirculation of the CO2-carrier fluids helps maintain a constant rock formation pressure, reducing the chance of seismic activity, as well as enabling monitoring of the extent of mineralization via tracers such as 14C rich CO2 or Ca isotopes (Matter et al., 2016; Snæbjörnsdóttir et al., 2017; Gíslason et al., 2018; von Strandmann et al., 2019; Clark et al., 2020). This approach was adopted at a geothermal energy plant in Hellisheiði, Iceland (Carbfix project). Specifically, CO2 (and H2S) emitted by the process were pre-dissolved by sparging in water and then co-injected with geothermal brine into highly-fractured basalt at depths of 300–1000 m (Matter et al., 2009; Gislason et al., 2010; Gutknecht et al., 2018). Each ton of CO2 required ~25 tons of water. To date, more than 70,000 tons of CO2 have been injected with an estimated 60% successfully mineralized (Clark et al., 2020). In 2021, the world's first DAC-mineralization plant (Project Orca) went live as a collaboration between CarbFix and the company Climeworks, with the goal to annually remove 4 kt CO2. Modeling studies indicate that basalt carbonation may be limited by alkalinity constraints and lead to the existence of unreacted free-phase CO2 (Tutolo et al., 2021). However, recent field-scale three-dimensional transport models of the CarbFix injection site indicate mineralization rates remain high even after many years of injection and that 300 Mt CO2 can be stored using just 10% of the rock pore space (Ratouis et al., 2022). The role that secondary minerals, e.g., clays and zeolites, play in the reactivity of CO2 with basalt is yet to be fully understood. Furthermore, the evolution of dissolution and precipitation fronts and their effect on rock permeability and fluid flow during the injection period is another phenomenon with great uncertainty (Lisabeth et al., 2017; Peuble et al., 2018).
In the third (iii) approach, alkaline geological fluids are extracted from ultramafic rock formations, such as peridotite, and allowed to absorb CO2 from air in surface ponds creating DIC. The fluids are then recirculated through the rock where DIC reacts and forms carbonate minerals. This speculative approach is based on natural terrestrial alkaline springs and their associated surface travertine deposits (Kelemen and Matter, 2008; Kelemen et al., 2011; Power et al., 2013b). However, the circulation of fluids with such low concentrations of dissolved carbon could be prohibitively expensive. Therefore, it has been suggested that this approach can be scaled cost effectively by combination with DAC that produces low purity (3–5% wt.) CO2 (Kelemen et al., 2020). Although the process seems promising, challenges remain. For example, as the fluid pathways become filled with product carbonate minerals the permeability of the rock formation would reduce, inhibiting CO2 transport to unreacted rock further from the injection well and causing the system to become self-limiting. On the other hand, volume expansion during carbonation can create enough force to fracture the host rock, maintaining permeability. Such “reaction-driven cracking” could increase permeability, thus enhancing the efficiency of in situ mineralization in peridotite (Kelemen and Hirth, 2012; Kelemen et al., 2013; Sohn, 2013; Evans et al., 2020). Overall, the balance between clogging and cracking during in situ mineralization in peridotite remains a key uncertainty.
In the fourth (iv) and final approach, seawater is circulated through ultramafic rocks near the oceans (Kelemen and Matter, 2008). Although largely speculative, some natural analogs exist which indicate potential feasibility (Grozeva et al., 2017; Kelemen, 2017; Picazo et al., 2020). This approach simultaneously increases alkalinity of the ocean, while removing DIC in seawater by reaction with peridotite, thus creating a double driving force for CO2 drawdown from the air into the ocean via manipulation of the Revelle factor (Egleston et al., 2010). Thermal gradients between the rock and seawater could drive natural circulation.
DAC with in situ mineralization is energy intensive and can require significant heat for regenerating capture sorbents, in addition to significant power input for CO2/air sparging and fluid pumping. In both the CarbFix and Orca projects, the heat and power needs are met by geothermal energy (Marieni et al., 2018; Adams et al., 2020). Geothermal fluids typically have temperatures of 70–250°C (Zarrouk and Moon, 2014) enabling integration with DAC systems whose synthetic sorbents (usually amine-based polymers) are regenerated in a similar range. Tectonically active areas such as the Western United States, Alaska, Hawaii, British Columbia, Indonesia, the Philippines, Italy, Turkey, New Zealand, Japan, Iceland, Kenya, Mexico, El Salvador, and Central America (Zarrouk and Moon, 2014) could provide low-cost opportunities for geothermal powered DAC and mineralization. As new geothermal technologies develop, such opportunities could expand elsewhere (Olasolo et al., 2016). Alternatively, the CO2 and power requirement for in situ mineralization could be simultaneously provided by bioenergy (Turner et al., 2018), which has the advantage of lower levelized cost of CO2 capture than DAC. However, pipelines would be needed for transportation of CO2, and issues with biodiversity, land requirements, sustainability, and scalability could arise (Burns and Nicholson, 2017; Smith et al., 2019).
In situ mineralization may have fewer adverse environmental and human health effects than surface-based geochemical NETs, since materials are mostly contained beneath the Earth's surface where they have little direct impact on ecosystems and biodiversity, and typically use less land and fresh water than other NETs (National Academies of Sciences, Engineering, and Medicine, 2019). Wastewater could be co-injected with CO2 for dual benefit (Phan et al., 2018). In situ mineralization could have other potential co-benefits, such as enabling the transition of workers from fossil fuel industries into the clean energy sector where near-identical skills are required. Reaction-driven cracking could be applied to in situ mining of metals and uranium (Kelemen et al., 2020). On the other hand, there are potential risks with the injection of CO2 and fluids for geological storage, including: (i) production and leakage of methane (CH4) and hydrogen sulfide (H2S) to the atmosphere by CO2-reducing bacteria (Guyot et al., 2011); (ii) groundwater acidification (Li et al., 2018); (iii) heavy metal mobilization, which could contaminate local water supply (de Orte et al., 2014); and (iv) increasing seismicity (Blondes et al., 2019). The latter presented certain key challenges, which were identified during the initial stages of the CarbFix project where several (micro)seismic events were initially observed (Hjörleifsdóttir et al., 2021). Improved engineering methods, i.e., constant recirculation of the fluids, reduced seismic occurrences, while engagement with local residents aided public acceptance of the project. Partnership with Climeworks, as well as the addition of a geothermal lagoon for bathing also aided in improving public acceptance (Aradóttir and Hjálmarsson, 2018).
Ex situ
Ex situ routes were the first approaches for CO2 mineralization to be investigated for the purpose of climate change mitigation (Lackner et al., 1995), particularly focusing on reducing point source emissions. Ex situ mineralization involves reacting high surface area alkaline minerals with CO2-rich gases, mainly in engineered reactors (Gerdemann et al., 2007). Ex situ approaches using crushed natural rocks rich in minerals such as olivine (Kwon et al., 2011), serpentine (Park and Fan, 2004; Wang and Maroto-Valer, 2011b; Nduagu et al., 2012), and wollastonite (Huijgen et al., 2006; Daval et al., 2009; Xu et al., 2019) have been investigated, but industrial alkaline wastes and by-products, such as mine tailings (Bodénan et al., 2014) or iron and steel slags (Yadav and Mehra, 2017), are likely better suited to ex situ processes owing to greater reactivity than their natural counterparts, as discussed in the section Artificial Alkaline Minerals—Industrial by-Products and Wastes, and Tailored Minerals. High temperatures and pressures (Domingo et al., 2006), high CO2 partial pressures (Li et al., 2019), additives (Krevor and Lackner, 2009), and mechanical (Fabian et al., 2010; Li and Hitch, 2018), or heat activation (Farhang et al., 2019) could be used to capture and store CO2 within timeframes relevant to industrial processes. Although ex situ processes are likely best integrated with readily available sources of concentrated CO2 from industry, integration with DAC may also be possible. For example, OCO Technology, which makes carbonate construction materials, is now working with London-based Mission Zero Technologies, to use CO2 sourced by air capture (OCO Technology, 2021).
Ex situ processes can be broadly categorized as either “direct” or “indirect.” Direct CO2 mineralization occurs in one step, as a gas-solid (Kwon et al., 2011; Liu et al., 2018) or as a gas-liquid-solid process (Benhelal et al., 2019; Li et al., 2019). Indirect CO2 mineralization methods use multiple steps which overall result in the dissolution of a silicate mineral and the creation of a carbonate mineral. First Mg and/or Ca is extracted from the mineral feedstocks, followed by reaction with CO2. This is usually achieved by a pH swing approach using reagents, e.g., hydrochloric acid (Lackner et al., 1995; Ferrufino et al., 2018), acetic acid (Kakizawa et al., 2001), ammonium salts (Wang and Maroto-Valer, 2011a; Highfield et al., 2012), ammonia and brine (based on solvay process) (Huang et al., 2001) or molten salt (MgCl2·nH2O) (Wendt et al., 1998), where the acidic reagents aid in mineral dissolution and the alkali reagents aid in carbonate precipitation. Reagents should be recycled as part of the process. Direct processes have the advantage of greater simplicity, whereas indirect approaches have the advantage of faster throughput and the production of high purity carbonate minerals (Zevenhoven et al., 2011). Direct mineralization requires pure CO2 for reaction (necessitating integration with either DAC or BECCS), whereas some indirect processes produce reactive alkaline hydroxides that may be suitable for direct reaction with atmospheric CO2.
Ex situ approaches can also produce useful carbonated products (Fernández Bertos et al., 2004; Hills et al., 2020; Qiu, 2020). Other valuable side products such as hydrogen could enable ex situ processes to become more economical (Kularatne et al., 2018). However, life cycle assessments (LCAs) frequently show that not all ex situ approaches result in negative emissions (Ncongwane et al., 2018; Thonemann et al., 2022). Further information on different ex situ processes can be found elsewhere (Sanna et al., 2014; Veetil and Hitch, 2020; Yadav and Mehra, 2021).
Surficial
Surficial CO2 mineralization is any process by which low purity CO2 (either from the air, or low CO2 concentration gases and liquids) is reacted with alkaline materials in piles, fields, pools, or large indoor spaces such as greenhouses. Surficial processes generally require less intensive reaction conditions than ex situ processes, with carbon removal occurring over weeks to months, rather than minutes. Surficial approaches allow minerals to be carbonated near to their site of production, thus reducing mineral transportation costs. Like ex situ approaches, surficial approaches enable the sale of the carbonated minerals, for example, as aggregates for the building and construction sector (Huntzinger et al., 2009a,b; Liu et al., 2021).
Surficial mineralization of crushed alkaline materials was investigated by Myers and Nakagaki (2020) who proposed a gas-solid method whereby finely crushed materials are spread thinly in vertical tiers in a greenhouse. Solar panels drive fans which continuously supply fresh air over the layers of material and trays of water provide the necessary humidity. This approach suggested the use of a variety of alkaline materials from natural mafic and ultramafic rocks to anthropogenic materials such as slag and lime. Other surficial approaches have investigated carbonation of existing mafic and ultramafic mine tailings (Wilson et al., 2006; Power et al., 2010, 2014, 2020; Mervine et al., 2018; Kelemen et al., 2020) and industrial wastes such as slag (Stolaroff et al., 2005). Artificial materials are usually favored due to their greater reactivity than natural minerals. In general, most industrial wastes and by-products (Table 2) present promising opportunities for surficial mineralization due to their wide availability and relatively low cost (Renforth, 2019).
CO2 availability is often the limiting factor in ambient weathering of mine tailings and other industrial alkaline wastes (Wilson et al., 2009; Pullin et al., 2019). Therefore, increasing the CO2 supply in surficial processes using DAC to provide low purity CO2 could lower overall costs compared to air (Kelemen et al., 2020). While higher purity CO2 could theoretically be used, significant losses would occur for systems which are not closed. The availability of humidity in the air could also be a limiting in some cases, with some studies quoting a minimum requirement of 55–60% relative humidity required for the reaction to take place (Erans et al., 2020; Samari et al., 2020).
Surficial mineralization of anthropogenic waste materials may serve a dual purpose of waste management (via a reduction in liability associated with hazardous materials) in addition to CO2 removal. This may be achievable at greater scale and lower cost than ex situ approaches. For example, the building and construction industry is thought to be accountable for 40% of solid waste worldwide (Shan et al., 2017). Stockpiles of steel slag produce highly alkaline leachates (pH > 10) (Yi et al., 2012) that can lead to environmental issues surrounding potential heavy metal mobilization and local pollution (Mayes et al., 2008). Carbonation reduces the pH of these wastes, and reduces the mobility of toxic metals. Similarly, carbonation destroys the hazardous asbestiform aspect of some mine tailings (Bobicki et al., 2012). Likewise, 70 million tons of highly alkaline red mud, a waste product of alumina production, are generated annually. Disposal of this waste is challenging due to aluminum toxicity and leaching of alkalinity into groundwater supplies (Bobicki et al., 2012). Carbonation mitigates these effects (Renforth, 2012) and enables the products to be used as a soil amendment, a reagent for removal of nitrogen and phosphorus from wastewater, a fertilizer additive, brick manufacture, plastic filler, and cement production (Bonenfant et al., 2008).
Most of the work conducted on mineralization processes has focused on ex situ approaches where high conversion can be reached rapidly (Sanna et al., 2014). Alternative surficial approaches are emerging which can potentially combine the scalability of enhanced weathering with the ability to produce useful carbonate products, or to remediate hazardous industrial mineral wastes. However, the kinetics of ambient mineralization are poorly understood, and more work is needed.
Enhanced Weathering in Soils
Enhanced weathering in soils aims to accelerate the natural process of weathering through the spreading of crushed Mg- and Ca-rich silicate rocks in agricultural, urban, and forest soils (Renforth, 2012; Hartmann et al., 2013; Beerling et al., 2020; Haque et al., 2020b). Carbonate rocks, such as limestone or dolomite, could also be used for enhanced weathering in soils, however, (i) they are unlikely to achieve the same spatial flux of alkalinity (Renforth and Campbell, 2021); (ii) they have a lower CO2 sequestration potential; and (iii) they deliver fewer co-benefits than silicate rocks (Beerling et al., 2018). Through enhanced weathering in soils, atmospheric CO2 is drawn down into the soil, dissolved into porewaters and transformed into bicarbonate () and carbonate () anions. The result of this process includes carbon stored as carbonate minerals in soils, or dissolved bicarbonates and carbonates draining into surface waters and eventually transported to the ocean where they contribute to ocean alkalinity (Renforth and Campbell, 2021). Depending on the type of mineral used, enhanced weathering in soils has the potential to remove between 0.3 and 1.25 tons of atmospheric CO2 per ton of mineral dissolved (Renforth, 2012; Moosdorf et al., 2014; Haque et al., 2019), although the maximum scalable potential when using rocks is <1. Cost estimates for enhanced weathering in soils vary by country ranging from US$55–190 per ton of CO2 removed, with estimates for China, India, Indonesia or Brazil at the lower end, and USA, Canada and European countries at the higher end of the cost range (Beerling et al., 2020).
The weathering rate of silicate minerals depends on several abiotic and biotic factors. Specifically, it increases with increasing surface area (Strefler et al., 2018), while higher pH, lower temperature, and precipitation rates, along with varying soil CO2 partial pressure can negatively affect the weathering rates (Verbruggen et al., 2021). Biogeochemical and biomechanical activity can also affect weathering rates in soils (Vicca et al., 2022). Plants may enhance silicate mineral weathering in soils through their roots and associated mycorrhizal fungi, via diverse mechanisms such as the release of organic acids (Taylor et al., 2009; Thorley et al., 2015; Verbruggen et al., 2021) and secretion of acids or stimulation of acid-generating nitrification by nitrogen-fixing plants (Bolan et al., 1991; Epihov et al., 2017; Perakis and Pett-Ridge, 2019). Invertebrates in soil also contribute to weathering, both chemically, through the action of gut microbiota, and mechanically by biopedturbation (Van Groenigen et al., 2019; Vicca et al., 2022).
Besides capturing CO2, enhanced weathering in soils also presents potential associated benefits. For example, soil pH is increased by alkalinity fluxes, eroded soils are replenished in the long-term with macro (e.g., Mg, Ca, K, P, and S) and micronutrients (e.g., B, Mo, Cu, Fe, Mn, Zn, and Ni) (Leonardos et al., 1987; Hartmann et al., 2013; Anda et al., 2015), while plant resilience to biotic and abiotic stress improves (Beerling et al., 2018). Particularly in agricultural land, enhanced weathering in soils could help revert agricultural soil erosion, act as a liming agent, and help reduce the use of fertilizers and pesticides (Kantola et al., 2017; Beerling et al., 2018, 2020; Haque et al., 2020b). As croplands cover 10% of the Earth's land surface (Monfreda et al., 2008), there is potential for large-scale application. Additionally, the equipment currently used in farming (e.g., lime spreaders) can be easily adapted to spread ground rocks on agricultural lands. Forested areas also provide opportunity for enhanced weathering in soils. The associated benefits of applying silicate rock dust on soils could promote plant growth and survival rate, through replenishing soil nutrients (Leonardos et al., 1987; Hartmann et al., 2013; Anda et al., 2015) and through increasing plant resilience to external stresses (Beerling et al., 2018). Moreover, mycorrhizal fungi associated with plant roots are one of the main drivers of silicate rock weathering in soils (Taylor et al., 2009; Thorley et al., 2015; Verbruggen et al., 2021). Urban and brownfield soils also present certain advantages for soil enhanced weathering (Manning and Renforth, 2013). In urban soils, enhanced weathering can be integrated in the landscape design to create carbon sinks, such as “carbon capture gardens,” while brownfields have the potential to develop value by removing CO2 as part of their remediation process (Manning and Renforth, 2013).
Alongside its many potential co-benefits, enhanced weathering in soils also has several drawbacks and risks, associated with comminution (which is the most energy demanding step of the process) (Renforth, 2012), transportation (as the distance from quarry to field increases, the CO2eq emissions increase and by extension the CO2 sequestration potential declines) (Lefebvre et al., 2019), and pollutants embedded into the mineral matrix being released in the environment through weathering (Haque et al., 2020a). For example, depending on the chemical composition of the parent material, the weathering of silicate minerals such as olivine might release heavy metals including chromium (Cr), nickel (Ni), or other elements [e.g., silicon (Si)], affecting the receiving ecosystems. Particularly in croplands, if these elements are present in concentrations higher than recommended by soil quality guidelines, they could be incorporated in the food chain, acting as pollutants and also affecting human and environmental health (ten Berge et al., 2012; Haque et al., 2020a). Plants with metal-accumulating mechanisms have been proposed as a strategy for preventing contamination of soils and water with toxic metals during enhanced weathering (Suhrhoff, 2022). There are also concerns about the effect of alkalinity addition to freshwater ecosystems, which have been shown to be sensitive to pH changes (Morgan, 1987; Wyatt and Stevenson, 2010; Pulido et al., 2012). Furthermore, the mining of natural rocks can have an ecological impact on wildlife, and/or require deforestation (Edwards et al., 2017). To reduce the need for mining and lower the overall cost, silicate-rich non-hazardous by-products or wastes from industrial processes, such as iron and steel slag (Das et al., 2019) or cement kiln dust (Beerling et al., 2020), can be used. Nevertheless, long-term comprehensive field studies across different climates and soil types are required to assess the suitability of spreading industrial alkaline wastes or by-products in soils (Beerling et al., 2020).
Finally, enhanced weathering in soils could be combined with other NETs, such as afforestation or reforestation, or with the feedstock crops used in BECCS and biochar. A combination of different approaches implemented together has a greater potential of achieving the CO2 removal capacity that is needed (Minx et al., 2018) and reducing operational costs (Beerling et al., 2018). Additionally, combining enhanced weathering in soils with afforestation or reforestation and biochar could also lead to synergies, both through negating one another's potential negative impacts, and through increasing the carbon capture uptake (Amann and Hartmann, 2019). Figure 4 shows the synergistic effects of combining enhanced weathering in soils, BECCS, afforestation/reforestation, and biochar. One proposed co-deployment scenario is the combination of enhanced weathering and biochar onto land used to grow crops for BECCS. In this scenario, the crushed rocks would act as a source of micro- and macronutrients (Leonardos et al., 1987; Hartmann et al., 2013; Anda et al., 2015), while biochar would increase nutrient release (Atkinson et al., 2010) and crop productivity (Jeffery et al., 2011; Kantola et al., 2017).
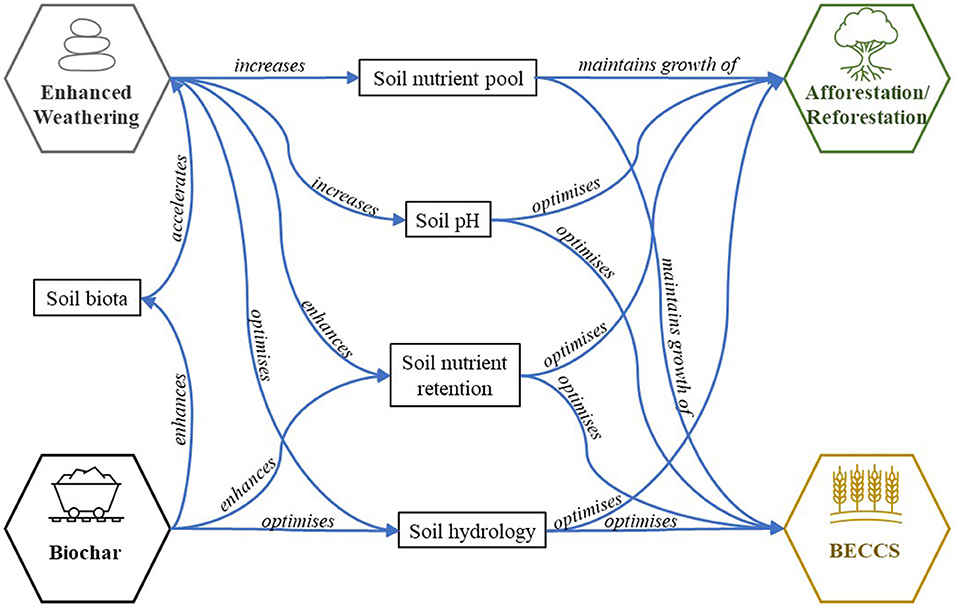
Figure 4. Summary of effects from combining terrestrial NETs: enhanced weathering, biochar, afforestation/reforestation and bioenergy with carbon capture and storage (BECCS). Adapted from Amann and Hartmann (2019).
Ocean Alkalinity Enhancement
Seawater covers the majority of the Earth's surface (Shiklomanov, 1993) and is a large natural reservoir of carbon. Specifically, DIC in ocean water, which is dissolved CO2 gas and bicarbonate and carbonate ions, is 140 times higher, by volume, than gaseous carbon in the atmosphere (de Lannoy et al., 2018). An equilibrium between atmospheric CO2 and surface ocean waters exists, described by the Revelle factor, which accounts for ~25% of anthropogenic (surplus) CO2 emissions already absorbed (Watson et al., 2020). The main mechanism for CO2 mineralization in the oceans is its dissolution in seawater, since when CO2 reacts with water (H2O) it forms carbonic acid (H2CO3), bicarbonate () and acidity (H3O+), the latter mainly neutralized by carbonate ions () forming again bicarbonate, but also contributing to acidification (Egleston et al., 2010). As a result, the oceans are annually sequestering ~0.5 Gt CO2 from the atmosphere (Renforth and Henderson, 2017). However, the capacity of the oceans for CO2 uptake is not infinite and cannot cope with the increasing anthropogenic CO2 emissions. Furthermore, CO2 is acidic and therefore its uptake by surface ocean waters comes at the expense of their natural alkalinity, i.e., their mean pH has dropped by ~0.1 units over the past two centuries from an initial average of ~8.2 (Caldeira et al., 2005), and is projected to further decline by ~0.3 by 2100 (Figuerola et al., 2021). Note that pH scale is logarithmic and therefore the ~0.1 drop corresponds to around a 26% reduction of the surface ocean waters' mean alkalinity.
Research has previously explored injection of CO2 into the deep ocean (Caldeira et al., 2005), however, the possibility of storing neutralized CO2 as increased alkalinity is gaining increasing attention (Renforth and Henderson, 2017), along with the removal of DIC from seawater. Specifically, two main ocean-based NETs include: (i) removing carbon (DIC) from seawater, similar to DAC, using electrochemical processes (de Lannoy et al., 2018; Eisaman et al., 2018; La Plante et al., 2021a); and (ii) artificially increasing ocean alkalinity for enhancing seawater's carbon sequestration capacity (Renforth and Henderson, 2017), a process popularly known as ocean alkalinity enhancement (OAE) (Bach et al., 2019; Gagern et al., 2019). For the first pathway, given that the majority of carbon in seawater is in the form of carbonate and bicarbonate ions, CO2 extraction processes must “swing” alkalinity to extract as much DIC as possible. DIC-depleted seawater will then return to the oceans, where atmospheric CO2 will be taken up to maintain the air-ocean equilibrium. Therefore, ocean water can be used for both carbon capture and/or storage. Other CO2 storage pathways exist, however these are not long-term DIC storage, but rather “ephemeral” storage up to 1,000 years of pure phase CO2, such as dry ice torpedoes where CO2 is released from the sea surface with the goal being to penetrate the sea floor (Herzog and Drake, 1996), and intentional storage of CO2 in deep ocean, as solid CO2 hydrate, possibly in depths higher than 3,000 m, where CO2 is denser than seawater and long-term storage is promoted (Caldeira et al., 2005). However, these storage pathways have been associated with environmental impacts on deep ocean ecosystems (Caldeira et al., 2005), while, even in the deep ocean solid CO2 hydrate will slowly decompose (GHG, 2004).
Electrochemical processes aim at controlling seaweater's pH and trigger the removal of DIC (Sharifian et al., 2021). In the context of geochemical NETs, an acid (HCl) and an alkali (NaOH) are first produced, by seawater/brine electrolysis or electrodialysis, which can be used for two different distinct processes, i.e., the “acid” and the “base” process, respectively. In the acid process, HCl is used to acidify (to pH ~4) degassed (O2 and N2 have been removed) seawater, thus converting DIC to CO2 gas, which is then captured (e.g., using hollow fiber membrane contactors). In the base process NaOH is directly added to seawater to raise the pH (>9) and precipitate carbonates in the form of CaCO3 (de Lannoy et al., 2018). Subsequently, the natural alkalinity is restored in the decarbonized seawater of both processes, which is then returned to the ocean to recapture CO2 and store it as bicarbonate (Eisaman et al., 2018). It could be possible to directly release the elevated-pH seawater from the base process to the ocean, since this will lead to additional atmospheric CO2 uptake due to ocean alkalinity enhancement. Even though only the base process comprises a direct mineralization pathway (CaCO3 is the final product), the gaseous CO2 acid process can also be used to produce carbonate minerals. Single-step carbon sequestration and storage is similar to the base process since carbonate minerals are electrochemically (flow-through membraneless electrolysis) formed from DIC and divalent cations (Ca and Mg) that are naturally contained in seawater, however, in this case, without the need of fine-pore membranes but rather coarse-mesh electrodes (La Plante et al., 2021b). The main challenge in electrochemical processes pertains to high electricity and raw material (mainly electrodes or membranes) inputs (Sharifian et al., 2021), which raise costs and possibly environmental impacts.
In OAE alkaline materials, such as natural or artificial minerals and industrial waste/by-products (Renforth, 2019), are used to increase the oceans' alkalinity, thereby adding to its natural capacity as a carbon sink, i.e., CO2 is permanently (>10,000 years) stored as aqueous bicarbonate ions (Renforth and Henderson, 2017). Three main methods for addition of alkaline materials to the ocean have been proposed: (i) coastal enhanced weathering (Meysman and Montserrat, 2017; Renforth and Campbell, 2021), whereby crushed or pulverized silicate minerals are spread onto beaches and coastal shelves and waves and currents promote their dissolution and the release of alkalinity into the ocean; (ii) ocean liming, whereby lime is spread on the open ocean for artificial alkalinity enhancement (Paquay and Zeebe, 2013; Renforth et al., 2013); and (iii) electrochemical approaches, which do not aim to remove DIC from seawater, but produce alkaline agents that can be used for OAE (House et al., 2009). Wastes from different industrial processes could also be used for OAE at scale (Renforth, 2019). However, alkaline wastes can be enriched with harmful pollutants, such as trace (heavy) metals (Gomes et al., 2016), and therefore, more likely, might find limited application for OAE, at least in the near future.
For silicate minerals to be quickly dissolved in the upper ocean, pulverization (<1 μm) is required (Meysman and Montserrat, 2017), which is energy intensive and not practical (Hangx and Spiers, 2009). On the other hand, it can take up to thousands of years for olivine sand to fully dissolve in coastal environments (Hangx and Spiers, 2009), but high-energy shallow marine environments (coastal shelves and beaches) can greatly accelerate olivine dissolution (Schuiling and de Boer, 2011). Specifically, natural forces acting on the coastal environments, such as waves and currents, can be used to enhance the dissolution rates of silicate minerals (Montserrat et al., 2017). Among the industrially mined silicate minerals, olivine has been found promising for coastal enhanced weathering, since it is abundant and readily available (olivine mines can be found across the world), while its dissolution rate is significantly higher (three orders of magnitude) compared to ordinary quartz (Meysman and Montserrat, 2017). Similar to enhanced weathering in soils, coastal enhanced weathering can capture 0.28 g C per g olivine dissolved in ocean water (Köhler et al., 2013). On the other hand, olivine also contains various nutrients (mainly silicic acid and iron) that improve phytoplankton growth (fertilization), leading to a maximum carbon capture capacity of up to 0.57 g C per g of olivine spread (Hauck et al., 2016). Nonetheless, among others, silicic acid can alter marine biology, shifting phytoplankton species composition toward silicifiers such as diatoms (Köhler et al., 2013), while phytoplankton fertilization cannot be considered as a direct mineralization pathway. Silicifier growth can also turn the color of seawater to greener shades (Bach et al., 2019). Overall, coastal enhanced weathering appears promising, however, many open questions exist, including non-stoichiometric dissolution, pore-water saturation in the seabed, possible secondary reactions, and the exact ecosystem and feedback effects of large-scale olivine dissolution (Montserrat et al., 2017).
The surface ocean waters are supersaturated with respect to calcite (CaCO3), i.e., limestone, and therefore its “activation,” typically through calcination prior to its spreading for OAE, has been proposed (Kheshgi, 1995). As such, ocean liming is typically achieved using artificial alkaline minerals such as calcium oxide (CaO) or more likely calcium hydroxide (Ca(OH)2) (Caserini et al., 2021), which will rapidly dissolve and release alkalinity in seawater (Justnes et al., 2020). Specifically, limestone is mined, crushed, washed, milled, and then calcined, before being transported and dispersed into surface ocean waters for alkalinity enhancement. It has been reported that 1.4–1.7 tons of limestone can uptake 1 ton of CO2 from the atmosphere (Renforth et al., 2013). Since (bi)carbonate ions are added to seawater, ocean liming can benefit calcifiers such as corals (Comeau et al., 2013; Feng et al., 2016), coccolithophores, foraminiferans, and pteropods (Figuerola et al., 2021) which are threatened by ocean acidification (Doney et al., 2020). The rapid growth of calcifiers such as coccolithophores can give a bluer or whiter shade to the ocean color (Bach et al., 2019). Ocean liming faces several engineering challenges (Renforth et al., 2013), particularly in capturing and storing the carbon emissions from limestone decomposition (Paquay and Zeebe, 2013) and ocean spreading (Caserini et al., 2021), along with uncertainties pertaining to the responses of marine organisms to large-scale lime addition (Paquay and Zeebe, 2013; Das and Mangwani, 2015). Furthermore, ocean liming appears to allow calcifiers to outcompete silicifiers, while coastal enhanced weathering allows for the opposite (Moore, 2021). This implies that these NETs can be used in tandem to maximize the uptake of atmospheric CO2 and maintain the balance between different marine (micro)organisms.
Finally, it is possible to use electrochemistry to remove acidity, in the form of HCl, from seawater or from brines [e.g., from the desalination industry (Mustafa et al., 2020)] and store or neutralize this acidity (e.g., using silicate minerals) and return alkalinity (NaOH is produced along with HCl while excess alkalinity can also be produced from the dissolution of silicate minerals) to the oceans (House et al., 2007; Davies, 2015). Electrolysis or electrodialysis can be used to split seawater/brines and generate acidity and alkalinity; however, the proposed processes are typically energy intensive, with values in the range of 3–18 GJ per t of CO2 removed from the atmosphere (Renforth and Henderson, 2017). Brines with high salt concentrations can be used to improve the process efficiency in acid (HCl) and alkali (NaOH) production; however, it is unlikely that the existing brines, primarily from the desalination industry, are sufficient for scaling up this process to the gigaton range. Therefore, it is more likely that electrochemical processes for OAE will be used complementary to coastal enhanced weathering and ocean liming, at least until the technology matures and becomes more energy efficient.
Application of Biotechnology to Geochemical NETs
Organisms are known to control many of the key reactions underlying geochemical NETs (Table 4), and microbial weathering strongly influences geologic cycling (Finlay et al., 2020; Samuels et al., 2020). The organisms, biomolecules and metabolisms underlying biogeochemical activity provide a diversity of mechanisms that can be integrated into geochemical NETs. Further, they can potentially be enhanced through protein engineering, directed evolution, metabolic engineering or other synthetic biology methods. Examples of naturally-existing mechanisms, applications of biotechnology to geochemical NETs to date, and future perspectives are discussed in the following three subsections.
The Influence of Organisms and Biological Mechanisms on the Chemical Reactions Underlying Geochemical NETs
Many bacteria and fungi promote dissolution of alkaline minerals by altering the chemical microenvironment at the mineral surface, in some cases for liberation of essential nutrients (Rogers and Bennett, 2004). Mechanisms include acidification via secretion of protons and weak organic acids, generation of carbonic acid as a byproduct of respiration, production of strong acids by chemolithotrophs, and production of polymeric and small molecule organic acids that act as chelators that catalyze mineral dissolution (Barker et al., 1997; Drever and Stillings, 1997; Nordstrom and Southam, 1997; Bennett et al., 2001; Lazo et al., 2017; Pokharel et al., 2019; Gerrits et al., 2021). Fungi can also increase the surface area of rocks by exerting mechanical forces that induce cracking (Bechinger et al., 1999). Lichen (a mutualism between a fungus and a cyanobacterium) are particularly active in silicate mineral weathering, through penetration of hyphae and thalli, secretion of organic acids, and provision of dissolved carbonate and acidification through respiration (Chen et al., 2000). Rates of secretion of weathering agents by fungi and bacteria are reported to vary by mineral substrate and nutrient availability (Bennett et al., 2001; Schmalenberger et al., 2015), indicating that weathering can occur by mechanisms that are actively regulated by organisms. Organisms can also facilitate alkaline mineral dissolution by inhibiting the formation of passivating iron oxide layers; one such mechanism is secretion of small-molecule chelators known as siderophores (Liermann et al., 2000; Buss et al., 2007; Ahmed and Holmström, 2014; Torres et al., 2019). There has been some disagreement over the extent to which microbes and organic acid chelators catalyze mineral dissolution, and the range of minerals on which they act (Pokrovsky et al., 2009, 2021; Oelkers et al., 2015), indicating the need for further discourse and research to obtain clarity on the contexts in which microbial weathering occurs. Microbial biofilms have also been observed to reduce mineral dissolution rates in some contexts by inhibiting the exchange of ions with the bulk solution (Ullman et al., 1996; Lüttge and Conrad, 2004). Plant roots can also secrete organic acids that act as catalytic chelators (Ryan et al., 2001), and also support fungal and bacterial communities with weathering activity (Kang et al., 2017; Ribeiro et al., 2020; Verbruggen et al., 2021), which together contribute to weathering within the rhizosphere.
Microbially-mediated precipitation of carbonates is also widespread (Zhu and Dittrich, 2016; Görgen et al., 2020), and its mechanisms are more thoroughly characterized than those of silicate dissolution. Many organisms promote carbonate precipitation by generating alkalinity or increasing ionic saturation state through metabolic activity. Photosynthetic organisms, which are thought to have influenced the majority of calcium carbonate formation through Earth's history (Altermann et al., 2006; Riding, 2006), promote carbonate precipitation by increasing pH through conversion of to CO2 and OH−, after which CO2 is assimilated into biomass and OH− is released (Power et al., 2007; Kamennaya et al., 2012). Ureolytic bacteria increase pH by producing NH4+, OH− and through hydrolysis of urea (Zhu and Dittrich, 2016). Microbial degradation of amino acids as an energy source, called ammonification, also produces NH4+, OH− and (González-Muñoz et al., 2010). Anaerobic oxidation of organic matter via reduction of nitrates by denitrifying bacteria, or reduction of sulfates by sulfate-reducing bacteria, produces alkalinity via consumption of protons and thereby also favors carbonate precipitation (Baumgartner et al., 2006; Martin et al., 2013). Oxalotrophic bacteria also promote carbonate precipitation by liberating both and Ca2+, along with CO2, through metabolism of solid calcium oxalate, which is abundant in many soils (Cromack et al., 1977; Braissant et al., 2002). Aerobic oxidation of organic matter by heterotrophs can also promote carbonate precipitation by producing CO2, when CO2 is limiting (Dupraz et al., 2009; Sánchez-Román et al., 2011).
Carbonate precipitation can also be influenced through biophysical mechanisms. Some extracellular materials secreted by microbes, broadly termed extracellular polymeric substances (EPS), contain a diversity of negatively charged or chelating chemical moieties that bind cations, and are observed to either inhibit or promote carbonate precipitation in different contexts. Under conditions of low saturation of bound cations, EPS can inhibit carbonate precipitation by depleting the microenvironment of cations, while under conditions of high saturation, EPS can promote carbonate precipitation by enriching the microenvironment with cations (Dupraz et al., 2009). Degradation of EPS by heterotrophs, which liberates bound cations and (bi)carbonates, can also induce carbonate precipitation (Dupraz et al., 2009). The surfaces of microbial cells themselves, which often bear negatively charged functional groups, can also promote carbonate precipitation by attracting cations (Dupraz et al., 2009). High EPS production in combination with alkalinity generation make cyanobacteria particularly effective at nucleating and precipitating carbonates (De Philippis et al., 2001; Decho et al., 2005; Braissant et al., 2009).
Notably, biological precipitation of magnesite (MgCO3) has been observed at room temperature (McCutcheon et al., 2019; Zhang et al., 2020), despite being inhibited at temperatures below 80°C in abiotic conditions due to the high energy of dehydration of aqueous Mg2+ (Saldi et al., 2009). The proposed mechanism for magnesite precipitation at ambient temperature is dehydration by the negatively charged EPS and cell surfaces (Power et al., 2017). Magnesite precipitation is preferable compared to hydrated magnesium carbonates, since it contains a higher stoichiometric fraction of carbon.
Enzymes and peptides are also known to catalyze reactions relevant to geochemical NETs, including hydration of CO2 and carbonate precipitation. Of these, CO2 hydration is the best studied and presently the most tractable to engineering. Carbonic anhydrases (CAs) are metalloenzymes that catalyze equilibration between dissolved CO2 and carbonic acid (Lindskog, 1997). They are among the most efficient enzymes known in nature, with their rates often being diffusion-limited. They exist as ≥8 evolutionarily distinct families of CA genes across all domains of life (Mesbahuddin et al., 2021), with diverse enzymatic parameters. CAs are involved in diverse processes, including biomineralization of calcium carbonates (Bertucci et al., 2013; Müller et al., 2013b; Karakostis et al., 2016; Sharker et al., 2021). CAs commonly have additional activity, including inhibition or promotion of carbonate precipitation (Miyamoto et al., 2005). A recent study found that bovine CA directly influences carbonate precipitation mechanisms in multiple ways: it interacts with growing calcium carbonate crystals, thereby modifying their growth and morphology; it can also inhibit precipitation under conditions of low CO2, possibly by stabilizing pre-nucleation ion complexes; and it can undergo conformational changes and oligomerization under high pH or high and Ca2+concentrations, abrogating anhydrase activity and instead templating nucleation of calcium carbonate (Rodriguez-Navarro et al., 2019). It has been suggested that the above mechanisms of CA are involved in the controlled growth of calcareous structures like shells (Rodriguez-Navarro et al., 2019). Carbonic anhydrase has also been demonstrated to catalyze the dissolution of calcite, possibly by catalyzing the transfer of protons into the mineral lattice during protonation of (Dong et al., 2020).
Multicellular and unicellular organisms also demonstrate exquisite control over carbonate precipitation using combinations of the aforementioned mechanisms during the formation of shells and intracellular carbonate inclusions. Intracellular formation of carbonates in bacteria has been observed, including in environments that are undersaturated with respect to carbonate mineral phases, suggesting that active mechanisms control the ion transport and mineral precipitation, though the precise mechanisms of control are as-yet unclear (Görgen et al., 2020). Shells in coccolithophores, mollusks, corals and reptilian and avian eggs are typically >95% CaCO3 by mass, with the remaining organic matter consisting of diverse proteins and polysaccharides functioning to scaffold, nucleate, or remodel calcium carbonate, including intracrystalline proteins (Falini et al., 2015; Taylor et al., 2017; Marin, 2020; Gautron et al., 2021). While the specific biomolecular functions of most shell organic components are unclear, many functions have been identified, including proteins that promote or inhibit calcium carbonate nucleation, as well as CAs (Evans, 2019; Marin, 2020). Interestingly, coccolithophores produce individual calcium carbonate particles known as coccoliths intracellularly within specialized vesicles, after which the particles are secreted onto the cell surface to form a shell (Taylor et al., 2017).
Other enzyme families, especially enriched in ocean-dwelling siliceous organisms, facilitate silica polymerization. Silicateins are enzymes found in sponges that catalyze polymerization of amorphous silica (Müller et al., 2013a), and silaffins are highly post-translationally modified peptides found in diatoms that do the same (Sumper and Kröger, 2004; Lechner and Becker, 2015). Silicase is an enzyme reported to catalyze the dissolution of amorphous silica (Schröer et al., 2003), though we are unaware of other published work in which silicase activity is documented reproduction of the original results should be pursued to inspire confidence in their robustness.
Further, active biological transport mechanisms exist for concentrating or depleting Ca2+, Mg2+, H+, , and H4SiO4 within microenvironments (Martin-Jezequel et al., 2000; Dominguez, 2004; Maguire, 2006; Buch-Pedersen et al., 2009; Reinfelder, 2011; Knight et al., 2016). For example, carboxysomes are cyanobacterial organelles within which bicarbonate is actively concentrated and then converted to CO2 by CA, thereby providing high levels of CO2 to the photosynthetic enzyme RuBisCO (Price et al., 2008).
Some research has investigated the influence of supercritical CO2 on microbes, with potential relevance to the use, or passive influence, of microbes on subsurface CO2 mineralization for storage. While supercritical CO2 generally inhibits microbial growth (Yu and Chen, 2019), multiple studies have found organisms with only mildly inhibited growth under supercritical CO2 and have investigated its effects on community structure and geochemistry (Peet et al., 2015; Santillan et al., 2015; Jin and Kirk, 2016; Freedman et al., 2017, 2018; Ham et al., 2017; Li et al., 2017; Boock et al., 2019).
Collectively, the organisms and biological mechanisms discussed in this section constitute a starting point for the integration of biology into alkaline mineral NETs.
Biologically-Enhanced Geochemical NETs
Biotechnology research has explored applied methods for geochemical NETs to a limited degree. Pioneering examples to date have focused primarily on enhanced dissolution and carbonation of mine tailings (Power et al., 2010; McCutcheon et al., 2014) at laboratory scale.
Sulfur-oxidizing bacteria have been proposed for use in dissolution of alkaline minerals by catalyzing the production of sulphuric acid from sulfides, e.g., sulphidic mine tailings (Power et al., 2010). Similar approaches are used for biomining of copper and other metals (Schippers et al., 2014). Laboratory optimization experiments found that such an approach liberated 39% of Mg from chrysotile mine tailings while maintaining leachate pH suitable for carbonate precipitation, and up to 84% of Mg in acidic leachate (McCutcheon et al., 2015). The cost of transporting acid-generating feedstocks, which are consumed stoichiometrically as silicates dissolve, may be strongly influenced by relative geographic location of tailings and feedstocks (Power et al., 2014). Combination of this approach with microbially-mediated carbonate precipitation has been proposed for carbon sequestration in ultramafic mine tailings or other ex situ applications (Power et al., 2014).
Cyanobacteria have been proposed as catalysts for precipitation of carbonates in alkaline mineral NETs (Jansson and Northen, 2010). In laboratory experiments, a cyanobacteria-dominated consortium sourced from an alkaline tailings lake was inoculated into columns of acid-leached chrysotile tailings and caused precipitation of a crust of magnesium carbonates containing ~18-fold more carbon than abiotic controls (McCutcheon et al., 2016). Large-scale bioreactor experiments simulating an artificial alkaline wetland with inoculation of a microbial consortium confirmed cyanobacteria-mediated formation of a magnesium carbonate crust, but found that depletion of essential nutrients, including phosphate, in the reaction medium limited microbial growth and EPS production in the majority of the bioreactor, drastically reducing mineral precipitation (McCutcheon et al., 2014). Increased nutrient delivery in a follow-up experiment resulted in biofilm growth that outpaced carbonate precipitation (McCutcheon et al., 2019); these studies indicate that nutrient distribution is an important consideration in scaled deployment. The latter study also found that diatom growth successfully sequestered ~87% of the silicon in the bioreactor, providing a sink for silicon waste produced during alkaline mineral dissolution. A microbial carbonation experiment on site at a tailings pile failed to produce a cemented carbonate-chrysotile crust; the authors hypothesized that accessibility of water and CO2, as well as effects of weather, limited microbial growth and carbonate precipitation (McCutcheon et al., 2017). Failure of the latter experiment illustrates the need for increased application-focused research and lends support to the notion that microbial carbonation of tailings may benefit from more controlled environments like bioreactors as compared with open tailings piles.
Oxidation of waste organics to CO2 in the presence of alkaline minerals has been proposed as a mechanism for promoting carbonation in contexts where CO2 accessibility is limiting; such an approach sequesters carbon that was fixed into organics, preventing its decay and the subsequent return to the atmosphere (Mitchell et al., 2010; Power et al., 2011, 2014). Heterotrophic bacteria have been shown to promote carbonate precipitation via oxidation of organic matter in laboratory experiments with phototrophic and heterotrophic consortia in mine tailings (Power et al., 2011) and ureolytic microbes in synthetic brine (Mitchell et al., 2010).
These early investigations into microbially-enhanced carbon sequestration in mine tailings illustrate promise for more scaled deployment. Carbon sequestration potential using microbes in mine tailings has been variously estimated at 123 t ha.−1 yr.−1 (McCutcheon et al., 2016) to 222–238 t ha.−1 yr.−1 (McCutcheon et al., 2019) based on rates found in laboratory experiments, or 175 Mt yr.−1 globally if carbonation is taken to completion, which could also be economically viable (Power et al., 2014). Applications using minerals mined for the purpose of weathering would increase this potential drastically. However, field trials and pilot tests, as well as integration and optimization of the combined use of phototrophs, heterotrophs, enzymes and biostimulants will be required to determine the true benefits and costs these approaches can bring to carbon removal and sequestration.
Some authors have proposed the biomass produced during phototroph-mediated carbonate precipitation could be used for production of fuels or other commodity chemicals (Ramanan et al., 2010; Power et al., 2011), providing a second source of economic utility. The biomass could also be fed to heterotrophs, producing carbonic acid and other organic acids that would dissolve alkaline minerals and further precipitate carbonate.
CA has been investigated for applications in carbon capture, utilization and storage (CCUS), primarily in point source CO2 scrubbers (Alvizo et al., 2014; Bose and Satyanarayana, 2017; Giri et al., 2020; Mesbahuddin et al., 2021). Some research has investigated the use of CA in geochemical NETs. Bovine CA has been shown to efficiently promote carbonation of alkaline minerals by alleviating the hydration of CO2 as a kinetic barrier, with 240 and 360% increased sequestration rates of carbon in brucite under non-optimized conditions with sparging of 10% CO2 (Power et al., 2016) or ambient air (Power et al., 2013a), respectively. E. coli expressing recombinant CA were also shown to facilitate carbonate precipitation (Kim et al., 2012; Tan et al., 2018). The native CA activity in unicellular algae was found to facilitate carbonate precipitation during sparging of 10% CO2 in alkaline media while producing algal biomass. These experiments warrant further research into deployment of CA in geochemical NETs.
Ureolytic (Mitchell et al., 2010) and denitrifying (Martin et al., 2013) bacteria have also been explored for their potential to mediate carbonate precipitation for carbon sequestration. However, the utility of these alkalinity-generating heterotrophs is limited by the requirement that the carbon should be delivered as a reduced carbon feedstock (urea in the case of ureolytic), since they only produce enough alkalinity to mineralize the carbonate their metabolism produces (Mitchell et al., 2010). However, they may be useful for sealing pores via carbonate precipitation to form caprock for subsurface storage of supercritical CO2 (Cunningham et al., 2009).
While most engineered biomineralization research has been conducted under ambient pressures, a few studies have been performed at higher pressures more relevant to subsurface mineralization; however these studies were performed using ureolytic and denitrifying bacteria for the purpose of caprock formation (Martin et al., 2013; Mitchell et al., 2013). Organisms have been found growing as deep as 2.8 km in the subsurface, so the challenge of growth under high pressure is not insurmountable (Chivian et al., 2008; Glombitza et al., 2016).
In addition to sequestering carbon, biomineralization of alkaline minerals can provide a means for bioremediation of toxic metals or other pollutants by locking them within solid carbonate (Gadd, 2010; Zhu and Dittrich, 2016; Krajewska, 2018; Jain and Arnepalli, 2019; Ehrlich et al., 2021). Microbially-mediated carbonation of mine tailings may serve the dual purpose of sequestering carbon and remediating mine sites (McCutcheon et al., 2016).
It has also been proposed that alkaline minerals could be integrated into industrial microbial wastewater treatment and biogas production systems, where they would both neutralize acidity and sequester CO2 (Lu et al., 2018), with laboratory work showing promising results. In the methods proposed, microbes are not used for direct interaction with the minerals, but rather the minerals are integrated into existing microbial digestion or microbial electrosynthesis processes.
Toward Further Integration of Biology Into Geochemical NETs
Despite these exciting examples, we posit that the possibility-space of biologically-enhanced geochemical NETs is drastically under-explored given the broad ability of biological mechanisms to influence the key reactions. Organisms or biomolecules could be incorporated into the abiotic geochemical NETs described in the previous sections to improve reaction kinetics or prevent inhibitory passivation.
In environments where the dissolution rate of CO2 is limiting, CAs could be deployed in solution, immobilized on surfaces or within water-permeable beads (Xu et al., 2021), or produced by organisms engineered to secrete or display it (Zhu et al., 2022). CAs could also be used to catalyze the dissolution of calcite, generating alkalinity (Subhas et al., 2017). Silicase enzymes, if developed, could be useful for promoting the dissolution of alkaline minerals that contain polymerized silica, extending fast dissolution beyond the typically-favored orthosilicates (i.e., extend enhanced weathering beyond olivines to include pyroxenes, feldspars, etc.). Silicases could also help to dissolve passivation layers of amorphous silica.
Microbes could also be co-injected alongside aqueous or supercritical carbon dioxide to facilitate in situ mineralization. Microbes and enzymes could be particularly useful in reactor-based mineral weathering environments, where conditions can be more precisely controlled.
Microbes could also be applied alongside minerals that are distributed for terrestrial or coastal enhanced weathering efforts (Ribeiro et al., 2020). Given that environmental microbes generally function in communities, emerging methods for modification of microbial communities (Lawson et al., 2019) could be developed for use in many of the applications proposed above (e.g., in soil). If non-native or engineered microbes were used, the impact of uncontained release on indigenous ecosystems would need to be carefully considered, though challenges to stable establishment of non-native microbial communities is more likely to be encountered than unwanted proliferation (Albright et al., 2022).
Given the ability of organisms to alter chemical microenvironments, they may be useful in contexts where different conditions are desired at the mineral surface compared to the bulk solution; for example, acidic conditions could be generated at a mineral surface to enhance dissolution kinetics while more basic conditions are maintained in the bulk to favor carbonate precipitation, or catalysts could be secreted at the mineral surface to avoid costs of chemically altering the bulk. In general, the microbes or biomolecules used could be native or non-native, and unmodified or engineered. Engineered organisms may provide superior performance, as they could be optimized both for the unnatural chemical and physical environments created by geochemical NETs and for their specific roles in technologies; for example, catalysts discovered using molecular engineering methods could be produced metabolically by engineered organisms.
Biological approaches may also enable tuning of the characteristics of the output materials of geochemical NETs for improved utility; for example, carbonate or silica particles could be produced biologically for use as cement additives that may reduce emissions from cement production, or sequester carbon in concrete, while improving concrete performance (Müller et al., 2013a; Gadikota et al., 2015; Show et al., 2015; Singh et al., 2015; Iravani and Varma, 2019). Diatoms could also be used for low-cost production of silica particles, potentially with surface functionalization or enzymatic display, for use in concrete or other applications (Sardo et al., 2021).
Deployment of microbes or enzymes in the context of geochemical NETs may require tolerance of extreme conditions of pH, ionic strength, temperature, and/or pressure. Enzymes may need to be engineered for stability and/or isolated from extremophilic organisms (Packer and Liu, 2015; Mamo and Mattiasson, 2016; Ma et al., 2019; Ren et al., 2019; Yin et al., 2019); such work is already underway for carbonic anhydrase (Mesbahuddin et al., 2021). Deployed organisms, whether engineered or unmodified, will also need to tolerate those conditions; in the case of engineered organisms, this will require the development of synthetic biology methods for organisms that are presently non-standard (Wannier et al., 2020; Filsinger et al., 2021).
Many of the biogeochemical mechanisms discussed in this section have not received much attention from molecular biotechnology. Development of high-throughput platforms for their study and engineering will benefit both their application to NETs and the study of their underlying biology. For example, platforms for highly multiplexed screening, selection, or directed evolution of variants of enzymes or organisms that dissolve silicates or precipitate carbonates would be useful both for discovering natural mechanisms and for engineering. Such platforms are ubiquitous in well-developed medical domains of molecular biotechnology (Packer and Liu, 2015; Zeymer and Hilvert, 2018; Zeng et al., 2020; Madhavan et al., 2021).
Risks of environmental biocontamination should be considered before deployment of organisms in uncontained environments, such as mine tailings ponds or the subsurface. While engineering organisms for environmental deployment is a new frontier, we note that it is not without precedent, as there are many such research efforts underway (Coleman and Goold, 2019; Jaiswal and Shukla, 2020; Janssen and Stucki, 2020; Rylott and Bruce, 2020; Zhou et al., 2022), including field trials (Carvalho et al., 2015). Advances in biocontainment may provide options for restricting the spread of engineered microbes outside the intended environment (Lee et al., 2018).
Given the apparent potential for biotechnology to contribute to geochemical NETs, we encourage the research and funding communities to more thoroughly investigate possibilities, including designs for possible NETs incorporating biological mechanisms and applied engineering of specific mechanisms, as well as the underlying biogeochemical science. Design and techno-economic considerations should be examined, accounting for the constraints biotechnology could alleviate and introduce, for example, reductions in capital and operating expenditures resulting from improved kinetics and extent of reaction, as well as methods and costs for delivering feedstocks to stimulate organism growth. The diversity of organisms and biomolecules that manipulate key reactions, as well their chemical mechanisms and metabolic and genomic underpinnings, should be more comprehensively examined. New research tools should be developed that will benefit both the fundamental biology and engineering of relevant mechanisms. Although biotechnology applications for geochemical NETs are currently under-developed, they may prove to play an important role with continued dedicated efforts. Possible applications range in complexity, with some accessible today while others will require years or decades of concomitant advances in basic biology, biotechnology, and NETs engineering. The latter group may assist in driving down costs to enable the crucial scaling of NETs by mid-century.
Life Cycle Assessment on Geochemical NETs
Research has mainly focused on techno-economic aspects of different geochemical NETs, and NETs in general. However, this is not the case for other important aspects such as their social perspective, including social acceptance, and their environmental perspective, the latter typically examined using the life cycle assessment (LCA) methodology. Specifically, even though (geochemical) NETs aim at removing CO2, they could also be responsible for emitting GHGs and other pollutants throughout their life cycle (Cooper et al., 2022). Therefore, LCA can play an important role in both identifying such emissions and minimizing them, and also in comparing, from the environmental perspective, different NETs to identify the most promising solution under the local conditions. The reason is that the environmental performance of NETs depends not only on the technology but also on local conditions and spatial restrictions. For example, Lefebvre et al. (2019) found that transportation (distance from quarry to field) was a key limitation to enhanced weathering in soils, whereas Deutz and Bardow (2021) studied the environmental sustainability of the DAC plants in Hinwil and Hellisheiði, operated by Climeworks, and noted that the LCA results are very sensitive to the energy sources. Furthermore, when comparing different NETs, a wide range of environmental impacts should be considered (McQueen et al., 2021b) as well as other aspects of each technology. For example, Cooper et al. (2022) compared the effectiveness in carbon sequestration of afforestation/reforestation, enhanced weathering, DAC, and BECCS and noted that even though the first had the lowest environmental impact it exhibited very low carbon removal rates, whereas BECCS had a lower impact on climate change and toxicity compared to enhanced weathering and DAC, but a much higher impact on land use.
However, more research is required on LCA, since many challenges pertaining to different functional units, system boundaries, the climate change-energy nexus, and the timing of GHG emissions and removals make the direct comparison of different NETs difficult (Goglio et al., 2020). Furthermore, when system expansion has been used in LCA, avoided emissions, due to substitution of certain processes, have been misinterpreted as negative emissions, i.e., as carbon removal from the atmosphere, and therefore there is a need to distinguish between avoided and negative emissions, along with consistency in system boundaries and functional units (Terlouw et al., 2021). For example, when 27 LCA studies on NETs were reviewed by Tanzer and Ramírez (2019), 41% (11 studies) labeled avoided emissions as negative emissions, while it was also noted that system boundary choices also play an important role on the perceived emission balance of a NET. For this reason, guidelines for LCA studies on NETs have been proposed (Müller et al., 2020), while detailed life cycle inventory (LCI) data for different spatial extents, power systems, and chemical processes pertaining to NETs are also required (Cruz et al., 2021). The importance of the system boundary, allocation/system expansion, data availability and accuracy, parameter uncertainty, permanence of CO2 removal, and the need for common guidelines in LCAs for NETs has been also highlighted (McQueen et al., 2021b). In this regard, the integration of LCA with techno-economic analysis (TEA) can decrease NETs uncertainty and improve technology readiness levels (TRL) (Li and Wright, 2020).
Finally, apart from capturing and permanently storing CO2, (geochemical) NETs can also be associated with a wide range of positive but also negative environmental impacts, which have yet to be fully identified and therefore are not typically included in the system boundary of LCA studies. For example, ocean acidification greatly impacts marine ecosystems and reliant human communities (Doney et al., 2020), particularly affecting tropical coral ecosystems (Comeau et al., 2013; Feng et al., 2016). However, these positive effects of alkalinity addition, along with possible negative ones such as changes in the primary productivity, respiration, and photophysiology of living organisms in alkalinized seawater (Gore et al., 2019) or freshwater (Mant et al., 2013) have yet to be fully identified and quantified and therefore cannot be captured in LCA studies that examined ocean alkalinity enhancement NETs. This is also the case for terrestrial NETs. For example, although the potential co-benefits of dispersive enhanced weathering approaches are numerous, these are difficult to quantify and use as inputs in LCA studies, since their potentially irreversible effects may not become apparent until years after application, at which point the socio-technical systems may already be entrenched [see Collingridge dilemma (Collingridge, 1979)]. Therefore, there is a need for more research on the biological responses of different NETs, as to identify and quantify both the negative and positive effects and then use this data as inputs in LCA studies dealing with NETs. By doing so, more robust and reliable LCA results will be obtained which could also play an instrumental role in improving the social acceptability of NETs. Specifically, public acceptability can be a potential constraint on the research and deployment of NETs (Bertram and Merk, 2020) and particularly of the ocean-based NETS, which might face a greater public acceptability challenge than their terrestrial counterparts (Cox et al., 2021). To this end, apart from the environmental perspective, the social and economic perspectives should also be considered, thus examining the overall life cycle sustainability of each NET and effectively communicating the results to improve public perceptions and acceptability.
The Current State of Geochemical NETs
The recent Innovation for Cool Earth Forum (ICEF) Roadmap underlined that geochemical NETs have the lowest ratio of policy coverage to potential impact compared to other NET approaches. Most policy frameworks [e.g., 45Q, CA LCFS, EU CTS (Low Carbon Fuel Standard; EU Emissions Trading System (EU ETS); Internal Revenue Service, 2021)] have criteria that unintentionally exclude geochemical NETs. International agreements prohibit commercial NETs that involve the release of alkaline feedstock into the ocean. However, some government agencies such as the Advanced Research Projects Agency-Energy (ARPA-E) in the US are exploring the potential contribution of alkaline mineral-based technologies to negative emissions, enhanced metal recovery, and the decarbonization of industry and mining (ARPA-E, 2021).
While geochemical NETs remain a niche industry, the emergence of recent projects, such as those that offer carbon removal credits or sequester carbon within concrete, suggest that the industry is expanding. New companies are involved in piloting projects, some partnering with DAC companies that provide input CO2, e.g., the in situ mineralization projects underway in Iceland and Oman. Early but increasing exploration by large organizations in agriculture, mining, and concrete includes diamond miner DeBeers, who is experimenting in-house with mineralizing kimberlite (DeBeers group, 2019), Rio Tinto, who recently invested in a CarbonCapture DAC+mineralization project (Hiar, 2021), and The Global Cement and Concrete Association (80% of global production), whose 2021 roadmap calls for industrial-scale carbon capture at calcination sites (GCCA, 2021).
A list of companies and projects currently known to the authors is shown geographically in the map given in Figure 5. This map illustrates the range of projects underway today, but underlines that we are far from having a large-scale, high-functioning geochemical NETs industry, as many of these projects are in their infancy and have yet to deploy geochemical NETs or remove substantial amounts of CO2.
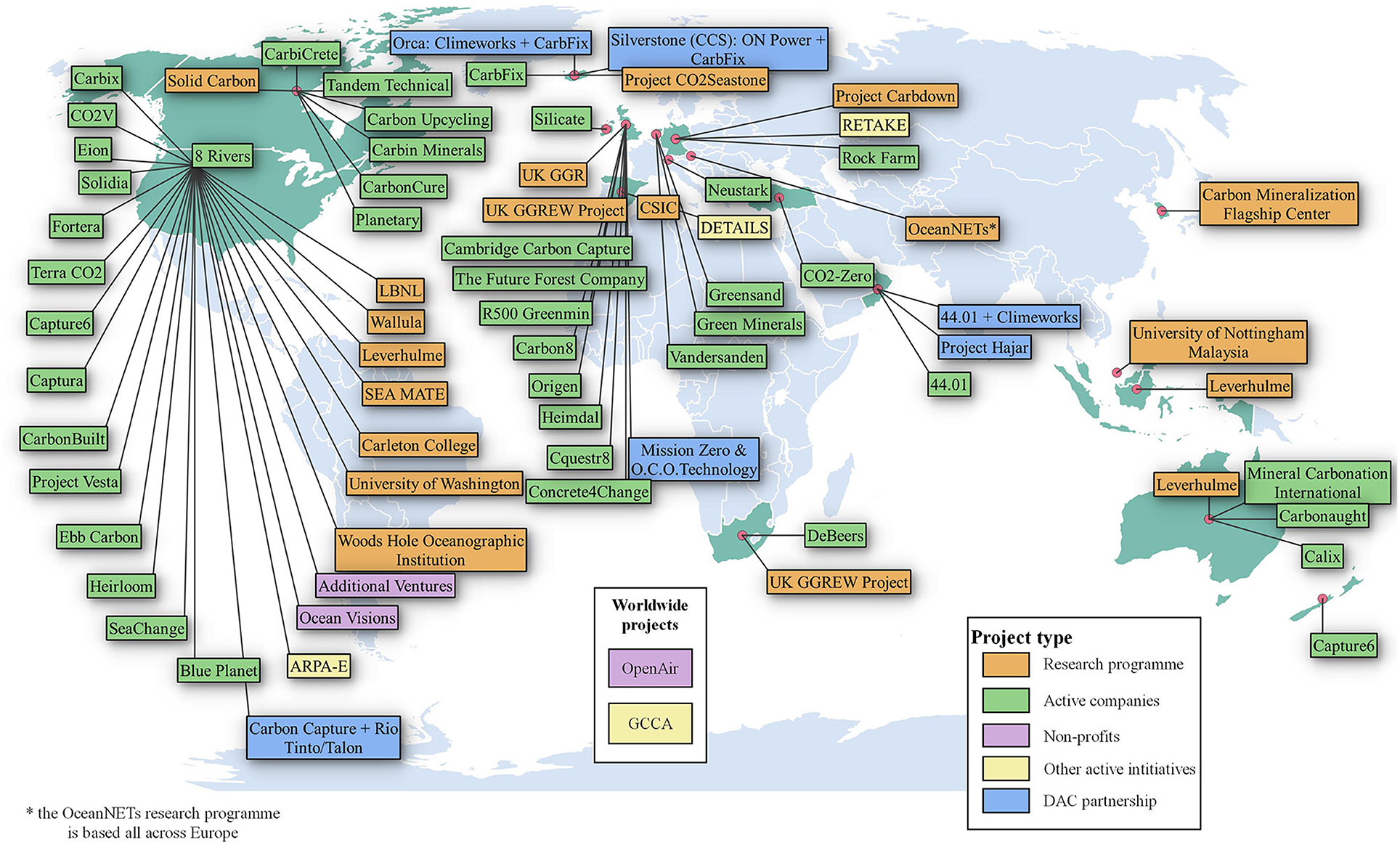
Figure 5. Global map of geochemical NET companies, projects, initiatives and non-profits. The figure includes projects found via basic online searches and may be incomplete. See Supplementary Information for more details and links to the projects.
Final Remarks
In this review, the body of knowledge on geochemical NETs was distilled and comprehensively discussed, focusing on terrestrial, subterranean, and ocean-based geochemical NETs. Over the last two decades, an enormous amount of work has been published relating to geochemical NETs, suggesting the large strides that have been made in this regard. It appears that these technologies have great potential for carbon removal and storage, owing to the vast resource of low cost natural and artificial alkaline minerals, their thermodynamically favorable reactions with CO2, and the long-term stability of the carbon-bearing products they form. However, the field of geochemical NETs remains, by and large, nascent with few fully-fledged technologies being available. To avoid the worst effects of climate change the introduction of geochemical NETs at scale (Gt CO2 yr.−1) is required as soon as possible. To achieve this, proactive, deliberate, and strategic initiatives are necessary for accelerating technology maturity along with substantially improving the public acceptance of these technologies, which is required for their large-scale introduction. For this reason, in Part II of this work, a roadmap, which is accessible and actionable to both specialist and non-specialist actors, is put forth with the goal of catalyzing the implementation of gigaton-scale removal on the timeframes that the Intergovernmental Panel on Climate Change (IPCC) and other key organizations suggest that is needed from as soon as 2025.
Author Contributions
All authors contributed to the drafting and revision of the manuscript, and read and approved the final submitted version.
Funding
The authors at Heriot-Watt University acknowledge UKRI funding under the UK Greenhouse Gas Removal Programme (NE/P019943/1, NE/ P019730/1), EPSRC funding for the Industrial Decarbonisation Research and Innovation Centre (EP/V027050/1), and EU funding under the H2020 Fighting and adapting to climate change programme OceanNETs project 869357. EB acknowledges Lisa Yang and the MIT Climate Grand Challenge initiative.
Conflict of Interest
The authors declare that the research was conducted in the absence of any commercial or financial relationships that could be construed as a potential conflict of interest.
Publisher's Note
All claims expressed in this article are solely those of the authors and do not necessarily represent those of their affiliated organizations, or those of the publisher, the editors and the reviewers. Any product that may be evaluated in this article, or claim that may be made by its manufacturer, is not guaranteed or endorsed by the publisher.
Acknowledgments
This work is a product of collaborative effort between Heriot-Watt University academics and non-governmental organization The Climate Map, whose mission is to uncover frontier opportunities and priority initiatives in carbon removal and storage, and catalyze action on them. We thank the following co-founders of The Climate Map and members of The Climate Map's Steering Committee, who played a key role in conceptualizing The Climate Map and its approach to roadmapping, and whose support made this project possible: Joel Armin-Hoiland, Remi Bouteille, David Mann, and Ryan Wise. We also thank Chris Boulos, Meghan Bush, and Charles Van Tassel for their support of The Climate Map's roadmapping process. We also thank Sarah Sclarsic, Shahar Bracha, Joey Benetatos and Gordon Southam for helpful conversations related to the biotechnology section of this review. Special thanks go to Liam Bullock for granting us permission to republish his kinetic data on the weathering of mine tailings.
Supplementary Material
The Supplementary Material for this article can be found online at: https://www.frontiersin.org/articles/10.3389/fclim.2022.879133/full#supplementary-material
References
Abe, M., Tanaka, S., Noguchi, M., and Yamasaki, A. (2021). Investigation of mineral carbonation with direct bubbling into concrete sludge. ACS Omega 6, 15564–15571. doi: 10.1021/acsomega.0c04758
Adams, B., Sutter, D., Mazzotti, M., and Saar, M. O. (2020). “Combining direct air capture and geothermal heat and electricity generation for net-negative carbon dioxide emissions.” in World Geothermal Congress 2020 (Zurich: ETH Zurich).
Ahmed, E., and Holmström, S. J. M. (2014). Siderophores in environmental research: roles and applications. Microb. Biotechnol. 7, 196–208. doi: 10.1111/1751-7915.12117
Alba, N., Vázquez, E., Gassó, S., and Baldasano, J. M. (2001). Stabilization/solidification of MSW incineration residues from facilities with different air pollution control systems. Durability of matrices versus carbonation. Waste Manag. 21, 313–323. doi: 10.1016/s0956-053x(00)00082-9
Albright, M. B. N., Louca, S., Winkler, D. E., Feeser, K. L., Haig, S.-J., Whiteson, K. L., et al. (2022). Solutions in microbiome engineering: prioritizing barriers to organism establishment. ISME J. 16, 331–338. doi: 10.1038/s41396-021-01088-5
Altermann, W., Kazmierczak, J., Oren, A., and Wright, D. T. (2006). Cyanobacterial calcification and its rock-building potential during 3.5 billion years of Earth history. Geobiology 4, 147–166. doi: 10.1111/j.1472-4669.2006.00076.x
Alvizo, O., Nguyen, L. J., Savile, C. K., Bresson, J. A., Lakhapatri, S. L., Solis, E. O. P., et al. (2014). Directed evolution of an ultrastable carbonic anhydrase for highly efficient carbon capture from flue gas. Proc. Natl. Acad. Sci. U.S.A. 111, 16436–16441. doi: 10.1073/pnas.1411461111
Amann, T., and Hartmann, J. (2019). Ideas and perspectives: synergies from co-deployment of negative emission technologies. Biogeosciences 16, 2949–2960. doi: 10.5194/bg-16-2949-2019
Anda, M., Shamshuddin, J., and Fauziah, C. I. (2015). Improving chemical properties of a highly weathered soil using finely ground basalt rocks. Catena 124, 147–161. doi: 10.1016/J.CATENA.2014.09.012
Andreani, M., Luquot, L., Gouze, P., Godard, M., Hoisé, E., and Gibert, B. (2009). Experimental study of carbon sequestration reactions controlled by the percolation of CO2-rich brine through peridotites. Environ. Sci. Technol. 43, 1226–1231. doi: 10.1021/es8018429
Aradóttir, E. S. P., and Hjálmarsson, E. (2018). CarbFix – public engagement and transparency. Energy Procedia 146, 115–120. doi: 10.1016/j.egypro.2018.07.015
ARPA-E (2021). CO2 mineralization for in situ storage and ex situ enhanced metals recovery workshop. Available online at: https://arpa-e.energy.gov/events/co2-mineralization-for-in-situ-storage-and-ex-situ-enhanced-metals-recovery-workshop (accessed January 27, 2022)
Atkinson, C. J., Fitzgerald, J. D., and Hipps, N. A. (2010). Potential mechanisms for achieving agricultural benefits from biochar application to temperate soils: a review. Plant Soil 337, 1–18. doi: 10.1007/s11104-010-0464-5
Bach, L. T., Gill, S. J., Rickaby, R. E. M., Gore, S., and Renforth, P. (2019). CO2 removal with enhanced weathering and ocean alkalinity enhancement: potential risks and co-benefits for marine pelagic ecosystems. Front. Climate 1, 7. doi: 10.3389/fclim.2019.00007
Baciocchi, R., Costa, G., Di Bartolomeo, E., Polettini, A., and Pomi, R. (2009). The effects of accelerated carbonation on CO2 uptake and metal release from incineration APC residues. Waste Manage. 29, 2994–3003. doi: 10.1016/j.wasman.2009.07.012
Baciocchi, R., Polettini, A., Pomi, R., Prigiobbe, V., Von Zedwitz, V. N., and Steinfeld, A. (2006). CO2 Sequestration by direct gas–solid carbonation of air pollution control (APC) residues. Energy Fuels 20, 1933–1940. doi: 10.1021/ef060135b
Barker, W. W., Welch, S. A., and Banfield, J. F. (1997). “Chapter 12. Biogeochemical weathering of silicate minerals,” in Geomicrobiology (De Gruyter), 391–428. Available online at: https://www.degruyter.com/document/doi/10.1515/9781501509247-014/html (accessed January 11, 2022).
Baumgartner, L. K., Reid, R. P., Dupraz, C., Decho, A. W., Buckley, D. H., Spear, J. R., et al. (2006). Sulfate reducing bacteria in microbial mats: changing paradigms, new discoveries. Sediment. Geol. 185, 131–145. doi: 10.1016/j.sedgeo.2005.12.008
Béarat, H., Mckelvy, M. J., Chizmeshya, A. V. G., Gormley, D., Nunez, R., Carpenter, R. W., et al. (2006). Carbon sequestration via aqueous olivine mineral carbonation: role of passivating layer formation. Environ. Sci. Technol. 40, 4802–4808. doi: 10.1021/es0523340
Bechinger, C., Giebel, K. F., Schnell, M., Leiderer, P., Deising, H. B., and Bastmeyer, M. (1999). Optical measurements of invasive forces exerted by appressoria of a plant pathogenic fungus. Science 285, 1896–1899. doi: 10.1126/science.285.5435.1896
Beerling, D. J., Kantzas, E. P., Lomas, M. R., Wade, P., Eufrasio, R. M., Renforth, P., et al. (2020). Potential for large-scale CO2 removal via enhanced rock weathering with croplands. Nature 583, 242–248. doi: 10.1038/s41586-020-2448-9
Beerling, D. J., Leake, J. R., Long, S. P., Scholes, J. D., Ton, J., Nelson, P. N., et al. (2018). Climate, food and soil security. Nat. Plants 4, 138–147. doi: 10.1038/s41477-018-0108-y
Benhelal, E., Rashid, M. I., Rayson, M. S., Brent, G. F., Oliver, T., Stockenhuber, M., et al. (2019). Direct aqueous carbonation of heat activated serpentine: discovery of undesirable side reactions reducing process efficiency. Appl. Energy 242, 1369–1382. doi: 10.1016/j.apenergy.2019.03.170
Bennett, P. C., Rogers, J. R., Choi, W. J., and Hiebert, F. K. (2001). Silicates, silicate weathering, and microbial ecology. Geomicrobiol. J. 18, 3–19. doi: 10.1080/01490450151079734
Bertram, C., and Merk, C. (2020). Public perceptions of ocean-based carbon dioxide removal: the nature-engineering divide? Front. Clim. 2, 31. doi: 10.3389/fclim.2020.594194
Bertucci, A., Moya, A., Tambutté, S., Allemand, D., Supuran, C. T., and Zoccola, D. (2013). Carbonic anhydrases in anthozoan corals-a review. Bioorg. Med. Chem. 21, 1437–1450. doi: 10.1016/j.bmc.2012.10.024
Bide, T. P., Styles, M. T., and Naden, J. (2014). An assessment of global resources of rocks as suitable raw materials for carbon capture and storage by mineralisation. Appl. Earth Sci. 123, 179–195. doi: 10.1179/1743275814Y.0000000057
Black, J. R., Carroll, S. A., and Haese, R. R. (2015). Rates of mineral dissolution under CO2 storage conditions. Chem. Geol. 399, 134–144. doi: 10.1016/j.chemgeo.2014.09.020
Blondes, M. S., Merrill, M. D., Anderson, S. T., and DeVera, C. A. (2019). Carbon Dioxide Mineralization Feasibility in the United States: U.S. Geological Survey Scientific Investigations Report 2018-5079. p. 29. doi: 10.3133/sir20185079
Bobicki, E. R., Zeng Zhenghe Xu, Q. L., and Hongbo (2012). Carbon capture and storage using alkaline industrial wastes. Prog. Energy Combust. Sci. 38, 302–320. doi: 10.1016/j.pecs.2011.11.002
Bodénan, F., Bourgeois, F., Petiot, C., Augé, T., Bonfils, B., Julcour-Lebigue, C., et al. (2014). Ex situ mineral carbonation for CO2 mitigation: evaluation of mining waste resources, aqueous carbonation processability and life cycle assessment (Carmex project). Miner. Eng. 59, 52–63. doi: 10.1016/j.mineng.2014.01.011
Bolan, N. S., Hedley, M. J., and White, R. E. (1991). Processes of soil acidification during nitrogen cycling with emphasis on legume based pastures. Plant Soil 134, 53–63. doi: 10.1007/BF00010717
Bonenfant, D., Kharoune, L., Sauvé, S., Hausler, R., Niquette, P., and Mimeault Murielle Kharoune, M. (2008). CO2 sequestration by aqueous red mud carbonation at ambient pressure and temperature. Ind. Eng. Chem. Res. 47, 7617–7622. doi: 10.1021/ie7017228
Boock, J. T., Freedman, A. J. E., Tompsett, G. A., Muse, S. K., Allen, A. J., Jackson, L. A., et al. (2019). Engineered microbial biofuel production and recovery under supercritical carbon dioxide. Nat. Commun. 10, 587. doi: 10.1038/s41467-019-08486-6
Bose, H., and Satyanarayana, T. (2017). Microbial carbonic anhydrases in biomimetic carbon sequestration for mitigating global warming: prospects and perspectives. Front. Microbiol. 8, 1615. doi: 10.3389/fmicb.2017.01615
Braissant, O., Decho, A. W., Przekop, K. M., Gallagher, K. L., Glunk, C., Dupraz, C., et al. (2009). Characteristics and turnover of exopolymeric substances in a hypersaline microbial mat. FEMS Microbiol. Ecol. 67, 293–307. doi: 10.1111/j.1574-6941.2008.00614.x
Braissant, O., Verrecchia, E. P., and Aragno, M. (2002). Is the contribution of bacteria to terrestrial carbon budget greatly underestimated? Naturwissenschaften 89, 366–370. doi: 10.1007/s00114-002-0340-0
Brantley, S. L. (2008). “Kinetics of mineral dissolution,” in Kinetics of Water-Rock Interaction, eds S. L. Brantley, J. D. Kubicki, and A. F. White (New York, NY: Springer New York), 151–210.
Brantley, S. L., and Mellott, N. P. (2000). Surface area and porosity of primary silicate minerals. Am. Mineral. 85, 1767–1783. doi: 10.2138/am-2000-11-1220
Brück, F., Schnabel, K., Mansfeldt, T., and Weigand, H. (2018). Accelerated carbonation of waste incinerator bottom ash in a rotating drum batch reactor. J. Environ. Chem. Eng. 6, 5259–5268. doi: 10.1016/j.jece.2018.08.024
Buch-Pedersen, M. J., Pedersen, B. P., Veierskov, B., Nissen, P., and Palmgren, M. G. (2009). Protons and how they are transported by proton pumps. Pflugers Arch. 457, 573–579. doi: 10.1007/s00424-008-0503-8
Bullock, L. A., James, R. H., Matter, J., Renforth, P., and Teagle, D. A. H. (2021). Global carbon dioxide removal potential of waste materials from metal and diamond mining. Front. Clim. 3, 77. doi: 10.3389/fclim.2021.694175
Bullock, L. A., Yang, A., and Darton, R. C. (2022). Kinetics-informed global assessment of mine tailings for CO2 removal. Sci. Total Environ. 808, 152111. doi: 10.1016/j.scitotenv.2021.152111
Burns, W., and Nicholson, S. (2017). Bioenergy and carbon capture with storage (BECCS): the prospects and challenges of an emerging climate policy response. J. Environ. Stud. Sci. 7, 527–534. doi: 10.1007/s13412-017-0445-6
Buss, H. L., Lüttge, A., and Brantley, S. L. (2007). Etch pit formation on iron silicate surfaces during siderophore-promoted dissolution. Chem. Geol. 240, 326–342. doi: 10.1016/j.chemgeo.2007.03.003
Caldeira, K., Akai, M., Brewer, P., Chen, B., Haugan, P., Iwama, T., et al. (2005). “Ocean storage,” in IPCC Special Report on Carbon Dioxide Capture and Storage (Cambridge; New York, NY: Cambridge University Press), 278–318.
Campbell, J. S. (2019). Decomposition of Carbonates in Capture of Carbon Dioxide From Ambient Air. Available online at: https://open.library.ubc.ca/collections/24/24/items/1.0387012
Carroll, J. J., Slupsky, J. D., and Mather, A. E. (1991). The solubility of carbon dioxide in water at low pressure. J. Phys. Chem. Ref. Data 20, 1201–1209. doi: 10.1063/1.555900
Carvalho, D. O., McKemey, A. R., Garziera, L., Lacroix, R., Donnelly, C. A., Alphey, L., et al. (2015). Suppression of a field population of aedes aegypti in brazil by sustained release of transgenic male mosquitoes. PLoS Negl. Trop. Dis. 9, e0003864. doi: 10.1371/journal.pntd.0003864
Caserini, S., Pagano, D., Campo, F., Abbà, A., De Marco, S., Righi, D., et al. (2021). Potential of maritime transport for ocean liming and atmospheric CO2 removal. Front. Clim. 3, 22. doi: 10.3389/fclim.2021.575900
Chen, H., Zhao, C., Yang, Y., and Zhang, P. (2012). CO2 capture and attrition performance of CaO pellets with aluminate cement under pressurized carbonation. Appl. Energy 91, 334–340. doi: 10.1016/j.apenergy.2011.09.032
Chen, J., Blume, H.-P., and Beyer, L. (2000). Weathering of rocks induced by lichen colonization — a review. Catena 39, 121–146. doi: 10.1016/S0341-8162(99)00085-5
Chivian, D., Brodie, E. L., Alm, E. J., Culley, D. E., Dehal, P. S., DeSantis, T. Z., et al. (2008). Environmental genomics reveals a single-species ecosystem deep within Earth. Science 322, 275–278. doi: 10.1126/science.1155495
Clark, D. E. (2019). Mineral Storage of Carbon in Basaltic Rocks at Elevated Temperatures. A Field and Experimental Study. Available online at: https://opinvisindi.is/handle/20.500.11815/1308
Clark, D. E., Oelkers, E. H., Gunnarsson, I., Sigfússon, B., Snæbjörnsdóttir, S. O., Aradóttir, E. S., et al. (2020). CarbFix2: CO2 and H2S mineralization during 3.5 years of continuous injection into basaltic rocks at more than 250 °C. Geochim. Cosmochim. Acta 279, 45–66. doi: 10.1016/j.gca.2020.03.039
Coleman, M. A., and Goold, H. D. (2019). Harnessing synthetic biology for kelp forest conservation1. J. Phycol. 55, 745–751. doi: 10.1111/jpy.12888
Collingridge, D. G. (1979). Entrenchment of technology: the case of lead petrol additives. Sci. Public Policy 6, 332–338.
Comeau, S., Carpenter, R. C., and Edmunds, P. J. (2013). Coral reef calcifiers buffer their response to ocean acidification using both bicarbonate and carbonate. Proc. R. Soc. B. 280, 20122374. doi: 10.1098/rspb.2012.2374
Cooper, J., Dubey, L., and Hawkes, A. (2022). Life cycle assessment of negative emission technologies for effectiveness in carbon sequestration. Procedia CIRP 105, 357–361. doi: 10.1016/j.procir.2022.02.059
Cox, E., Boettcher, M., Spence, E., and Bellamy, R. (2021). Casting a wider net on ocean NETs. Front. Clim. 3, 576294. doi: 10.3389/fclim.2021.576294
Cromack, K., Sollins, P., Todd, R. L., Fogel, R., Todd, A. W., Fender, W. M., et al. (1977). The role of oxalic acid and bicarbonate in calcium cycling by fungi and bacteria: some possible implications for soil animals. Ecol. Bull. 246–252.
Cruz, T. T. da, Perrella Balestieri, J. A., de Toledo Silva, J. M., Vilanova, M. R. N., Oliveira, O. J., and Ávila, I. (2021). Life cycle assessment of carbon capture and storage/utilization: from current state to future research directions and opportunities. Int. J. Greenhouse Gas Control 108, 103309. doi: 10.1016/j.ijggc.2021.103309
Cunningham, A. B., Gerlach, R., Spangler, L., and Mitchell, A. C. (2009). Microbially enhanced geologic containment of sequestered supercritical CO2. Energy Procedia 1, 3245–3252. doi: 10.1016/j.egypro.2009.02.109
Das, S., Kim, G. W., Hwang, H. Y., Verma, P. P., and Kim, P. J. (2019). Cropping with slag to address soil, environment, and food security. Front. Microbiol. 10, 1320. doi: 10.3389/fmicb.2019.01320
Das, S., and Mangwani, N. (2015). Ocean acidification and marine microorganisms: responses and consequences. Oceanologia 57, 349–361. doi: 10.1016/j.oceano.2015.07.003
Daval, D., Martinez, I., Corvisier, J., Findling, N., Goffé, B., and Guyot, F. (2009). Carbonation of Ca-bearing silicates, the case of wollastonite: experimental investigations and kinetic modeling. Chem. Geol. 265, 63–78. doi: 10.1016/j.chemgeo.2009.01.022
Davies, P. A. (2015). Solar thermal decomposition of desalination reject brine for carbon dioxide removal and neutralisation of ocean acidity. Environ. Sci. Water Res. Technol. 1, 131–137. doi: 10.1039/C4EW00058G
de Lannoy, C. F., Eisaman, M. D., Jose, A., Karnitz, S. D., DeVaul, R. W., Hannun, K., et al. (2018). Indirect ocean capture of atmospheric CO2: Part I. Prototype of a negative emissions technology. Int. J. Greenhouse Gas Control 70, 243–253. doi: 10.1016/j.ijggc.2017.10.007
de Orte, M. R., Sarmiento, A. M., DelValls, T. Á., and Riba, I. (2014). Simulation of the potential effects of CO2 leakage from carbon capture and storage activities on the mobilization and speciation of metals. Mar. Pollut. Bull. 86, 59–67. doi: 10.1016/j.marpolbul.2014.07.042
De Philippis, R., Sili, C., Paperi, R., and Vincenzini, M. (2001). Exopolysaccharide-producing cyanobacteria and their possible exploitation: a review. J. Appl. Phycol. 13, 293–299. doi: 10.1023/A:1017590425924
DeBeers group (2019). Available online at: https://www.debeersgroup.com/~/media/Files/D/De-Beers-Group-V2/documents/company-news/2019/MEDIA%20RELEASE%20-%20De%20Beers%20Group
%20carbon%20capture%20project%20receives%20research%20funding.pdf (accessed January 26, 2022).
Decho, A. W., Visscher, P. T., and Reid, R. P. (2005). Production and cycling of natural microbial exopolymers (EPS) within a marine stromatolite. Palaeogeogr. Palaeoclimatol. Palaeoecol. 219, 71–86. doi: 10.1016/j.palaeo.2004.10.015
Deutz, S., and Bardow, A. (2021). Life-cycle assessment of an industrial direct air capture process based on temperature–vacuum swing adsorption. Nature Energy 6, 203–213. doi: 10.1038/s41560-020-00771-9
Dichicco, M. C., Laurita, S., Paternoster, M., Rizzo, G., Sinisi, R., and Mongelli, G. (2015). Serpentinite carbonation for CO2 sequestration in the Southern Apennines: preliminary study. Energy Procedia 76, 477–486. doi: 10.1016/j.egypro.2015.07.888
Dijkstra, J. J., Comans, R. N. J., Schokker, J., and van der Meulen, M. J. (2019). The geological significance of novel anthropogenic materials: deposits of industrial waste and by-products. Anthropocene 28, 100229. doi: 10.1016/j.ancene.2019.100229
Domingo, C., Loste, E., Gómez-Morales, J., García-Carmona, J., and Fraile, J. (2006). Calcite precipitation by a high-pressure CO2 carbonation route. J. Supercrit. Fluids 36, 202–215. doi: 10.1016/j.supflu.2005.06.006
Dominguez, D. C. (2004). Calcium signalling in bacteria. Mol. Microbiol. 54, 291–297. doi: 10.1111/j.1365-2958.2004.04276.x
Doney, S. C., Busch, D. S., Cooley, S. R., and Kroeker, K. J. (2020). The impacts of ocean acidification on marine ecosystems and reliant human communities. Annu. Rev. Environ. Resour. 45, 83–112. doi: 10.1146/annurev-environ-012320-083019
Dong, S., Berelson, W. M., Teng, H. H., Rollins, N. E., Pirbadian, S., El-Naggar, M. Y., et al. (2020). A mechanistic study of carbonic anhydrase-enhanced calcite dissolution. Geophys. Res. Lett. 47, e2020GL089244. doi: 10.1029/2020gl089244
Drever, J. I., and Stillings, L. L. (1997). The role of organic acids in mineral weathering. Colloids Surf. A Physicochem. Eng. Asp. 120, 167–181. doi: 10.1016/S0927-7757(96)03720-X
Dreybrodt, W., Eisenlohr, L., Madry, B., and Ringer, S. (1997). Precipitation kinetics of calcite in the system CaCO3?H2O?C02: the conversion to CO2 by the slow process H++HCO3– → CO2+H2O as a rate limiting step. Geochim. Cosmochim. Acta 61, 3897–3904. doi: 10.1016/S0016-7037(97)00201-9
Dupraz, C., Reid, R. P., Braissant, O., Decho, A. W., Norman, R. S., and Visscher, P. T. (2009). Processes of carbonate precipitation in modern microbial mats. Earth Sci. Rev. 96, 141–162. doi: 10.1016/j.earscirev.2008.10.005
Edwards, D. P., Lim, F., James, R. H., Pearce, C. R., Scholes, J., Freckleton, R. P., et al. (2017). Climate change mitigation: potential benefits and pitfalls of enhanced rock weathering in tropical agriculture. Biol. Lett. 13, 20160715. doi: 10.1098/rsbl.2016.0715
Egleston, E. S., Sabine, C. L., and Morel, F. M. M. (2010). Revelle revisited: buffer factors that quantify the response of ocean chemistry to changes in DIC and alkalinity. Global Biogeochem. Cycles 24. doi: 10.1029/2008GB003407
Ehrlich, H., Bailey, E., Wysokowski, M., and Jesionowski, T. (2021). Forced biomineralization: a review. Biomimetics 6, 46. doi: 10.3390/biomimetics6030046
Eisaman, M. D., Rivest, J. L. B., Karnitz, S. D., de Lannoy, C. F., Jose, A., DeVaul, R. W., et al. (2018). Indirect ocean capture of atmospheric CO2: Part II. Understanding the cost of negative emissions. Int. J. Greenhouse Gas Control 70, 254–261. doi: 10.1016/j.ijggc.2018.02.020
El-Naas, M. H., El Gamal, M., Hameedi, S., and Mohamed, A.-M. O. (2015). CO2 sequestration using accelerated gas-solid carbonation of pre-treated EAF steel-making bag house dust. J. Environ. Manage. 156, 218–224. doi: 10.1016/j.jenvman.2015.03.040
Epihov, D. Z., Batterman, S. A., Hedin, L. O., Leake, J. R., Smith, L. M., and Beerling, D. J. (2017). N2-fixing tropical legume evolution: a contributor to enhanced weathering through the Cenozoic? Proc. Biol. Sci. 284. doi: 10.1098/rspb.2017.0370
Erans, M., Nabavi, S. A., and Manović, V. (2020). Carbonation of lime-based materials under ambient conditions for direct air capture. J. Clean. Prod. 242, 118330. doi: 10.1016/j.jclepro.2019.118330
EU Emissions Trading System (EU ETS). Climate Action (2015). Available online at: https://ec.europa.eu/clima/system/files/2017-03/ets_handbook_en.pdf (accessed February 14, 2022).
Evans, J. S. (2019). The biomineralization proteome: protein complexity for a complex bioceramic assembly process. Proteomics 19, e1900036. doi: 10.1002/pmic.201900036
Evans, O., Spiegelman, M., and Kelemen, P. B. (2020). Phase-field modeling of reaction-driven cracking: determining conditions for extensive olivine serpentinization. J. Geophys. Res. 125, e2019JB018614. doi: 10.1029/2019jb018614
Fabian, M., Shopska, M., Paneva, D., Kadinov, G., Kostova, N., Turianicová, E., et al. (2010). The influence of attrition milling on carbon dioxide sequestration on magnesium–iron silicate. Miner. Eng. 23, 616–620. doi: 10.1016/j.mineng.2010.02.006
Fagerlund, J., Highfield, J., and Zevenhoven, R. (2012). Kinetics studies on wet and dry gas–solid carbonation of MgO and Mg(OH)2 for CO2 sequestration. RSC Adv. 2, 10380–10393. doi: 10.1039/C2RA21428H
Fajardy, M., and Mac Dowell, N. (2017). Can BECCS deliver sustainable and resource efficient negative emissions? Energy Environ. Sci. 10, 1389–1426. doi: 10.1039/c7ee00465f
Falini, G., Fermani, S., and Goffredo, S. (2015). Coral biomineralization: a focus on intra-skeletal organic matrix and calcification. Semin. Cell Dev. Biol. 46, 17–26. doi: 10.1016/j.semcdb.2015.09.005
Falk, E. S., and Kelemen, P. B. (2015). Geochemistry and petrology of listvenite in the Samail ophiolite, Sultanate of Oman: complete carbonation of peridotite during ophiolite emplacement. Geochim. Cosmochim. Acta 160, 70–90. doi: 10.1016/j.gca.2015.03.014
Farhang, F., Oliver, T. K., Rayson, M. S., Brent, G. F., Molloy, T. S., Stockenhuber, M., et al. (2019). Dissolution of heat activated serpentine for CO2 sequestration: the effect of silica precipitation at different temperature and pH values. J. CO2 Utilization 30, 123–129. doi: 10.1016/j.jcou.2019.01.009
Feng, E. Y., Keller, D. P., Koeve, W., and Oschlies, A. (2016). Could artificial ocean alkalinization protect tropical coral ecosystems from ocean acidification? Environ. Res. Lett. 11, 74008. doi: 10.1088/1748-9326/11/7/074008
Fernández Bertos, M., Simons, S. J. R., Hills, C. D., and Carey, P. J. (2004). A review of accelerated carbonation technology in the treatment of cement-based materials and sequestration of CO2. J. Hazard. Mater. 112, 193–205. doi: 10.1016/j.jhazmat.2004.04.019
Ferrufino, G. L. A. A., Ferrufino, G. L. A., Okamoto, S., Dos Santos, J. C., de Carvalho, J. A., Avila, I., et al. (2018). CO2 sequestration by pH-swing mineral carbonation based on HCl/NH4OH system using iron-rich lizardite 1T. J. CO2 Utilization 24, 164–173. doi: 10.1016/j.jcou.2018.01.001
Figuerola, B., Hancock, A. M., Bax, N., Cummings, V. J., Downey, R., Griffiths, H. J., et al. (2021). A review and meta-analysis of potential impacts of ocean acidification on marine calcifiers from the southern ocean. Front. Marine Sci. 8, 584445. doi: 10.3389/fmars.2021.584445
Filsinger, G. T., Wannier, T. M., Pedersen, F. B., Lutz, I. D., Zhang, J., Stork, D. A., et al. (2021). Characterizing the portability of phage-encoded homologous recombination proteins. Nat. Chem. Biol. 17, 394–402. doi: 10.1038/s41589-020-00710-5
Finlay, R. D., Mahmood, S., and Rosenstock, N. (2020). Reviews and Syntheses: Biological Weathering and Its Consequences at Different Spatial Levels–From Nanoscale to Global Scale. Available online at: https://bg.copernicus.org/articles/17/1507/2020/
Freedman, A. J. E., Peet, K. C., Boock, J. T., Penn, K., Prather, K. L. J., and Thompson, J. R. (2018). Isolation, development, and genomic analysis of Bacillus megaterium SR7 for growth and metabolite production under supercritical carbon dioxide. Front. Microbiol. 9, 2152. doi: 10.3389/fmicb.2018.02152
Freedman, A. J. E., Tan, B., and Thompson, J. R. (2017). Microbial potential for carbon and nutrient cycling in a geogenic supercritical carbon dioxide reservoir. Environ. Microbiol. 19, 2228–2245. doi: 10.1111/1462-2920.13706
Gadd, G. M. (2010). Metals, minerals and microbes: geomicrobiology and bioremediation. Microbiology 156, 609–643. doi: 10.1099/mic.0.037143-0
Gadikota, G. (2020). Multiphase carbon mineralization for the reactive separation of CO2 and directed synthesis of H2. Nat. Rev. Chem. 4, 78–89. doi: 10.1038/s41570-019-0158-3
Gadikota, G., Fricker, K., Jang, S. H., and Park, A. H. A. (2015). “Carbonation of silicate minerals and industrial wastes and their potential use as sustainable construction materials,” in Advances in CO2 Capture, Sequestration, and Conversion, eds F. Jin, L.-N. He, and Y. H. Hu (Washington, DC: American Chemical Society), 295–322. Available online at: https://pubs.acs.org/doi/abs/10.1021/bk-2015-1194.ch012
Gagern, A., Rau, G., Rodriguez, D. I., Renforth, P., Burns, W., and Graffy, E. (2019). Ocean Alkalinity Enhancement - Current State of Knowledge and Potential Role of Philanthropy. Available online at: https://www.ceaconsulting.com/wp-content/uploads/Ocean-Alkalinity-Enhancement-CEA-proceedings-doc.pdf (accessed January 7, 2021).
Galeczka, I., Wolff-Boenisch, D., Oelkers, E. H., and Gislason, S. R. (2014). An experimental study of basaltic glass–H2O– CO2 interaction at 22 and 50°C: implications for subsurface storage of CO2. Geochim. Cosmochim. Acta 126, 123–145. doi: 10.1016/j.gca.2013.10.044
Gao, L., Yoshikawa, S., Iseri, Y., Fujimori, S., and Kanae, S. (2017). An economic assessment of the global potential for seawater desalination to 2050. Water 9, 763. doi: 10.3390/w9100763
Gautron, J., Stapane, L., Le Roy, N., Nys, Y., Rodriguez-Navarro, A. B., and Hincke, M. T. (2021). Avian eggshell biomineralization: an update on its structure, mineralogy and protein tool kit. BMC Mol. Cell Biol. 22, 11. doi: 10.1186/s12860-021-00350-0
GCCA (2021). The GCCA 2050 Cement and Concrete Industry Roadmap for Net Zero Concrete. Available online at: https://gccassociation.org/concretefuture/wp-content/uploads/2021/10/GCCA-Concrete-Future-Roadmap-Document-AW.pdf
Gerdemann, S. J., O'Connor, W. K., Dahlin, D. C., Penner, L. R., and Rush, H. (2007). Ex situ aqueous mineral carbonation. Environ. Sci. Technol. 41, 2587–2593. doi: 10.1021/es0619253
Gerrits, R., Wirth, R., Schreiber, A., Feldmann, I., Knabe, N., Schott, J., et al. (2021). High-resolution imaging of fungal biofilm-induced olivine weathering. Chem. Geol. 559, 119902. doi: 10.1016/j.chemgeo.2020.119902
GHG, I. (2004). Gas Hydrates for Deep Ocean Storage of CO2. Fornebu: IEA Greenhouse Gas R&D Programme Report PH4/26. Available online at: https://ieaghg.org/docs/General_Docs/Reports/PH4-26%20CO2%20hydrates.pdf
Giri, A., Sharma, V., Thakur, S., Sharma, T., Kumar, A., and Pant, D. (2020). “Engineering of microbial carbonic anhydrase for enhanced carbon sequestration,” in Chemo-Biological Systems for CO2 Utilization, 1st Edn, eds A. Kumar and S. Sharma (Boca Raton, FL: CRC Press), 91–105. Available online at: https://books.google.co.uk/books?hl=en&lr=&id=MPT0DwAAQBAJ&oi=fnd&pg=PP1&dq=Chemo-Biological+Systems+for+CO2+Utilization&ots=QlKnFsFcE-&sig=LIJiL-FJgoSF1pIesxG6SGvS6wk&redir_esc=y#v=onepage&q=Chemo-Biological%20Systems%20for%20CO2%20Utilization&f=false
Gislason, S. R., Broecker, W. S., Gunnlaugsson, E., Snæbjörnsdóttir, S., Mesfin, K. G., Alfredsson, H. A., et al. (2014). Rapid solubility and mineral storage of CO2 in basalt. Energy Procedia 63, 4561–4574. doi: 10.1016/j.egypro.2014.11.489
Gíslason, S. R., Sigurdardóttir, H., Aradóttir, E. S., and Oelkers, E. H. (2018). A brief history of CarbFix: Challenges and victories of the project's pilot phase. Energy Procedia 146, 103–114. doi: 10.1016/j.egypro.2018.07.014
Gislason, S. R., Wolff-Boenisch, D., Stefansson, A., Oelkers, E. H., Gunnlaugsson, E., Sigurdardottir, H., et al. (2010). Mineral sequestration of carbon dioxide in basalt: a pre-injection overview of the CarbFix project. Int. J. Greenhouse Gas Control 4, 537–545. doi: 10.1016/j.ijggc.2009.11.013
Glombitza, C., Adhikari, R. R., Riedinger, N., Gilhooly, W. P. 3rd, Hinrichs, K.-U., and Inagaki, F. (2016). Microbial sulfate reduction potential in coal-bearing sediments down to ~2.5 km below the seafloor off Shimokita Peninsula, Japan. Front. Microbiol. 7, 1576. doi: 10.3389/fmicb.2016.01576
Goglio, P., Williams, A. G., Balta-Ozkan, N., Harris, N. R. P., Williamson, P., Huisingh, D., et al. (2020). Advances and challenges of life cycle assessment (LCA) of greenhouse gas removal technologies to fight climate changes. J. Clean. Prod. 244, 118896. doi: 10.1016/j.jclepro.2019.118896
Gomes, H. I., Mayes, W. M., Rogerson, M., Stewart, D. I., and Burke, I. T. (2016). Alkaline residues and the environment: a review of impacts, management practices and opportunities. J. Clean. Prod. 112, 3571–3582. doi: 10.1016/j.jclepro.2015.09.111
González-Muñoz, M. T., Rodriguez-Navarro, C., Martínez-Ruiz, F., Arias, J. M., Merroun, M. L., and Rodriguez-Gallego, M. (2010). Bacterial biomineralization: new insights from Myxococcus-induced mineral precipitation. Geol. Soc. London Special Publ. 336, 31–50. doi: 10.1144/SP336.3
Gore, S., Renforth, P., and Perkins, R. (2019). The potential environmental response to increasing ocean alkalinity for negative emissions. Mitig. Adapt. Strateg. Glob. Chang. 24, 1191–1211. doi: 10.1007/s11027-018-9830-z
Görgen, S., Benzerara, K., Skouri-Panet, F., Gugger, M., Chauvat, F., and Cassier-Chauvat, C. (2020). The diversity of molecular mechanisms of carbonate biomineralization by bacteria. Discover Mater. 1, 2. doi: 10.1007/s43939-020-00001-9
Gras, A., Beaudoin, G., Molson, J., Plante, B., Bussière, B., Lemieux, J. M., et al. (2017). Isotopic evidence of passive mineral carbonation in mine wastes from the Dumont Nickel Project (Abitibi, Quebec). Int. J. Greenhouse Gas Control 60, 10–23. doi: 10.1016/j.ijggc.2017.03.002
Grozeva, N. G., Klein, F., Seewald, J. S., and Sylva, S. P. (2017). Experimental study of carbonate formation in oceanic peridotite. Geochim. Cosmochim. Acta 199, 264–286. doi: 10.1016/j.gca.2016.10.052
Gunnarsson, I., Aradóttir, E. S., Oelkers, E. H., Clark, D. E., Arnarson, M. Þ*., Sigfússon, B., et al. (2018). The rapid and cost-effective capture and subsurface mineral storage of carbon and sulfur at the CarbFix2 site. Int. J. Greenhouse Gas Control 79, 117–126. doi: 10.1016/j.ijggc.2018.08.014
Gutknecht, V., Snæbjörnsdóttir, S. Ó., Sigfússon, B., Aradóttir, E. S., and Charles, L. (2018). Creating a carbon dioxide removal solution by combining rapid mineralization of CO2 with direct air capture. Energy Procedia 146, 129–134. doi: 10.1016/j.egypro.2018.07.017
Guyot, F., Daval, D., Dupraz, S., Martinez, I., Ménez, B., and Sissmann, O. (2011). CO2 geological storage: the environmental mineralogy perspective. C. R. Geosci. 343, 246–259. doi: 10.1016/j.crte.2010.12.007
Ham, B., Choi, B.-Y., Chae, G.-T., Kirk, M. F., and Kwon, M. J. (2017). Geochemical influence on microbial communities at CO2-leakage analog sites. Front. Microbiol. 8, 2203. doi: 10.3389/fmicb.2017.02203
Hanak, D. P., Jenkins, B. G., Kruger, T., and Manovic, V. (2017). High-efficiency negative-carbon emission power generation from integrated solid-oxide fuel cell and calciner. Appl. Energy 205, 1189–1201. doi: 10.1016/j.apenergy.2017.08.090
Hanak, D. P., and Manovic, V. (2018). Combined heat and power generation with lime production for direct air capture. Energy Convers. Manage. 160, 455–466. doi: 10.1016/j.enconman.2018.01.037
Hänchen, M., Prigiobbe, V., Baciocchi, R., and Mazzotti, M. (2008). Precipitation in the Mg-carbonate system—effects of temperature and CO2 pressure. Chem. Eng. Sci. 63, 1012–1028. doi: 10.1016/j.ces.2007.09.052
Hangx, S. J. T., and Spiers, C. J. (2009). Coastal spreading of olivine to control atmospheric CO2 concentrations: a critical analysis of viability. Int. J. Greenhouse Gas Control 3, 757–767. doi: 10.1016/j.ijggc.2009.07.001
Haque, F., Chiang, Y. W., and Santos, R. M. (2020a). Risk assessment of Ni, Cr, and Si release from alkaline minerals during enhanced weathering. Open Agri. 5, 166–175. doi: 10.1515/opag-2020-0016
Haque, F., Santos, R. M., and Chiang, Y. W. (2020b). CO2 sequestration by wollastonite-amended agricultural soils – an Ontario field study. Int. J. Greenhouse Gas Control 97, 103017. doi: 10.1016/j.ijggc.2020.103017
Haque, F., Santos, R. M., Dutta, A., Thimmanagari, M., and Chiang, Y. W. (2019). Co-benefits of wollastonite weathering in agriculture: CO2 sequestration and promoted plant growth. ACS Omega 4, 1425–1433. doi: 10.1021/acsomega.8b02477
Hartmann, J., West, A. J., Renforth, P., Köhler, P., De La Rocha, C. L., Wolf-Gladrow, D. A., et al. (2013). Enhanced chemical weathering as a geoengineering strategy to reduce atmospheric carbon dioxide, supply nutrients, and mitigate ocean acidification. Rev. Geophys. 51, 113–149. doi: 10.1002/rog.20004
Haszeldine, R. S., Flude, S., Johnson, G., and Scott, V. (2018). Negative emissions technologies and carbon capture and storage to achieve the Paris Agreement commitments. Philos. Trans. R. Soc. Lond. A 376, 20160447. doi: 10.1098/rsta.2016.0447
Hauck, J., Köhler, P., Wolf-Gladrow, D., and Völker, C. (2016). Iron fertilisation and century-scale effects of open ocean dissolution of olivine in a simulated CO2 removal experiment. Environ. Res. Lett. 11, 024007. doi: 10.1088/1748-9326/11/2/024007
Haug, T. A., Kleiv, R. A., and Munz, I. A. (2010). Investigating dissolution of mechanically activated olivine for carbonation purposes. Appl. Geochem. 25, 1547–1563. doi: 10.1016/j.apgeochem.2010.08.005
Hellevang, H., Aagaard, P., Oelkers, E. H., and Kvamme, B. (2005). Can dawsonite permanently trap CO2? Environ. Sci. Technol. 39, 8281–8287. doi: 10.1021/es0504791
Henry, W., and Banks, J. (1803). III. Experiments on the quantity of gases absorbed by water, at different temperatures, and under different pressures. Philos. Trans. R. Soc. London 93, 29–274. doi: 10.1098/rstl.1803.0004
Herzog, H. J., and Drake, E. M. (1996). Carbon dioxide recovery and disposal from large energy systems. Annu. Rev. Energy Environ. 21, 145–166. doi: 10.1146/annurev.energy.21.1.145
Hiar, C. (2021). RioTinto+CarbonCapture. Available online at: https://www.eenews.net/articles/rio-tinto-invests-in-mining-carbon-dioxide-from-the-air/ (accessed January 26, 2022).
Highfield, J., Lim, H., Fagerlund, J., and Zevenhoven, R. (2012). Mechanochemical processing of serpentine with ammonium salts under ambient conditions for CO 2 mineralization. RSC Adv. 2, 6542–6548. doi: 10.1039/C2RA20575K
Hills, C. D., Tripathi, N., and Carey, P. J. (2020). Mineralization technology for carbon capture, utilization, and storage. Front. Energy Res. 8, 142. doi: 10.3389/fenrg.2020.00142
Hjörleifsdóttir, V., Ingvarsson, G., Ratouis, T., Gunnarsson, G., Snæbjörnsdóttir, S. Ó., and Sigfússon, B. (2021). “Ten years of induced earthquakes in the Húsmúli CO2 injection site, Hellisheiði, Iceland,” in SEG Global Meeting Abstracts. p. 96–100. doi: 10.1190/iceg2021-027.1
Holliman, J. E., Schaef, T., Miller, Q. R., Horner, J., McGrail, B. P., and Polites, E. (2021). “Carbon sequestration in basalts: Sidewall core characterization data from the wallula basalt pilot project,” in AGU Fall Meeting 2021 (AGU).
House, K. Z., House Christopher, H., Schrag, D. P., and Aziz, M. J. (2007). Electrochemical acceleration of chemical weathering as an energetically feasible approach to mitigating anthropogenic climate change. Environ. Sci. Technol. 41, 8464–8470. doi: 10.1021/es0701816
House, K. Z., House, C. H., Schrag, D. P., and Aziz, M. J. (2009). Electrochemical acceleration of chemical weathering for carbon capture and sequestration. Energy Procedia 1, 4953–4960. doi: 10.1016/j.egypro.2009.02.327
Huang, H. P., Shi, Y., Li, W., and Chang, S. G. (2001). Dual alkali approaches for the capture and separation of CO2. Energy Fuels 15, 263–268. doi: 10.1021/ef0002400
Huijgen, W. J. J., Witkamp, G.-J., and Comans, R. N. J. (2005). Mineral CO2 sequestration by steel slag carbonation. Environ. Sci. Technol. 39, 9676–9682. doi: 10.1021/es050795f
Huijgen, W. J. J., Witkamp, G.-J., and Comans, R. N. J. (2006). Mechanisms of aqueous wollastonite carbonation as a possible CO2 sequestration process. Chem. Eng. Sci. 61, 4242–4251. doi: 10.1016/j.ces.2006.01.048
Huntzinger, D. N., Gierke, J. S., Kawatra, S. K., Eisele, T. C., and Sutter, L. L. (2009a). Carbon dioxide sequestration in cement kiln dust through mineral carbonation. Environ. Sci. Technol. 43, 1986–1992. doi: 10.1021/es802910z
Huntzinger, D. N., Gierke, J. S., Sutter, L. L., Kawatra, S. K., and Eisele, T. C. (2009b). Mineral carbonation for carbon sequestration in cement kiln dust from waste piles. J. Hazard. Mater. 168, 31–37. doi: 10.1016/j.jhazmat.2009.01.122
Ihsanullah, I., Atieh, M. A., Sajid, M., and Nazal, M. K. (2021). Desalination and environment: a critical analysis of impacts, mitigation strategies, and greener desalination technologies. Sci. Total Environ. 780, 146585. doi: 10.1016/j.scitotenv.2021.146585
Internal Revenue Service (2021). Credit for carbon oxide sequestration. Federal Reg. 86, 4728–4773. Available online at: https://www.govinfo.gov/content/pkg/FR-2021-01-15/pdf/2021-00302.pdf
Iravani, S., and Varma, R. S. (2019). Biofactories: engineered nanoparticles via genetically engineered organisms. Green Chem. 21, 4583–4603. doi: 10.1039/C9GC01759C
Jain, S., and Arnepalli, D. N. (2019). “Biominerlisation as a remediation technique: A critical review,” in Geotechnical Characterisation and Geoenvironmental Engineering. Lecture Notes in Civil Engineering, Vol 16, eds V. Stalin, and M. Muttharam (Singapore: Springer). doi: 10.1007/978-981-13-0899-4_19
Jaiswal, S., and Shukla, P. (2020). Alternative strategies for microbial remediation of pollutants via synthetic biology. Front. Microbiol. 11, 808. doi: 10.3389/fmicb.2020.00808
Janssen, D. B., and Stucki, G. (2020). Perspectives of genetically engineered microbes for groundwater bioremediation. Environ. Sci. Process. Impacts 22, 487–499. doi: 10.1039/c9em00601j
Jansson, C., and Northen, T. (2010). Calcifying cyanobacteria–the potential of biomineralization for carbon capture and storage. Curr. Opin. Biotechnol. 21, 365–371. doi: 10.1016/j.copbio.2010.03.017
Jeffery, S., Verheijen, F. G. A., van der Velde, M., and Bastos, A. C. (2011). A quantitative review of the effects of biochar application to soils on crop productivity using meta-analysis. Agric. Ecosyst. Environ. 144, 175–187. doi: 10.1016/j.agee.2011.08.015
Ji, L., Yu, H., Zhang, R., French, D., Grigore, M., Yu, B., et al. (2019). Effects of fly ash properties on carbonation efficiency in CO2 mineralisation. Fuel Process. Technol. 188, 79–88. doi: 10.1016/j.fuproc.2019.01.015
Jin, Q., and Kirk, M. F. (2016). Thermodynamic and kinetic response of microbial reactions to high CO2. Front. Microbiol. 7, 1696. doi: 10.3389/fmicb.2016.01696
Jones, E., Qadir, M., van Vliet, M. T. H., Smakhtin, V., and Kang, S.-M. (2019). The state of desalination and brine production: a global outlook. Sci. Total Environ. 657, 1343–1356. doi: 10.1016/j.scitotenv.2018.12.076
José, N., Ahmed, H., Miguel, B., Luís, E., and Jorge, de B. (2020). Magnesia (MgO) production and characterization, and its influence on the performance of cementitious materials: a review. Materials 13, 4752. doi: 10.3390/ma13214752
Justnes, H., Escudero-Oñate, C., Garmo, Ø. A., and Mengede, M. (2020). Transformation kinetics of burnt lime in freshwaterand sea water. Materials 13, 4926. doi: 10.3390/ma13214926
Kakizawa, M., Yamasaki, A., and Yanagisawa, Y. (2001). A new CO2 disposal process via artificial weathering of calcium silicate accelerated by acetic acid. Energy 26, 341–354. doi: 10.1016/S0360-5442(01)00005-6
Kamennaya, N. A., Ajo-Franklin, C. M., Northen, T., and Jansson, C. (2012). Cyanobacteria as biocatalysts for carbonate mineralization. Minerals 2, 338–364. doi: 10.3390/min2040338
Kanakiya, S., Adam, L., Esteban, L., Rowe, M. C., and Shane, P. (2017). Dissolution and secondary mineral precipitation in basalts due to reactions with carbonic acid. J. Geophys. Res. 122, 4312–4327. doi: 10.1002/2017jb014019
Kang, S.-M., Waqas, M., Shahzad, R., You, Y.-H., Asaf, S., Khan, M. A., et al. (2017). Isolation and characterization of a novel silicate-solubilizing bacterial strain Burkholderia eburnea CS4-2 that promotes growth of japonica rice (Oryza sativa L. cv. Dongjin). Soil Sci. Plant Nutr. 63, 233–241. doi: 10.1080/00380768.2017.1314829
Kantola, I. B., Masters, M. D., Beerling, D. J., Long, S. P., and DeLucia, E. H. (2017). Potential of global croplands and bioenergy crops for climate change mitigation through deployment for enhanced weathering. Biol. Lett. 13, 20160714. doi: 10.1098/rsbl.2016.0714
Karakostis, K., Zanella-Cléon, I., Immel, F., Guichard, N., Dru, P., Lepage, T., et al. (2016). A minimal molecular toolkit for mineral deposition? Biochemistry and proteomics of the test matrix of adult specimens of the sea urchin Paracentrotus lividus. J. Proteomics 136, 133–144. doi: 10.1016/j.jprot.2016.01.001
Keith, D. W., Holmes, G., Angelo, D. S., and Heidel, K. (2018). A process for capturing CO2 from the atmosphere. Joule 2, 1573–1594. doi: 10.1016/j.joule.2018.05.006
Kelemen, P. (2017). “Harnessing peridotite alteration for carbon capture and storage,” in CO2 Summit III: Pathways to Carbon Capture, Utilization, and Storage Deployment, eds J. Wilcox, H. Krutka, S. Liguori, and N. M. Dowell (Columbia University). Available online at: https://dc.engconfintl.org/co2_summit3/19/ (accessed January 13, 2022).
Kelemen, P. B., and Hirth, G. (2012). Reaction-driven cracking during retrograde metamorphism: olivine hydration and carbonation. Earth Planet. Sci. Lett. 345–348, 81–89. doi: 10.1016/j.epsl.2012.06.018
Kelemen, P. B., and Matter, J. (2008). In situ carbonation of peridotite for CO2 storage. Proc. Natl. Acad. Sci. U.S.A. 105, 17295–17300. doi: 10.1073/pnas.0805794105
Kelemen, P. B., Matter, J., Streit, E. E., Rudge, J. F., Curry, W. B., and Blusztajn, J. (2011). Rates and mechanisms of mineral carbonation in peridotite: natural processes and recipes for enhanced, in situ CO2 capture and storage. Annu. Rev. Earth Planet. Sci. 39, 545–576. doi: 10.1146/annurev-earth-092010-152509
Kelemen, P. B., McQueen, N., Wilcox, J., Renforth, P., Dipple, G., and Vankeuren, A. P. (2020). Engineered carbon mineralization in ultramafic rocks for CO2 removal from air: review and new insights. Chem. Geol. 550, 119628. doi: 10.1016/j.chemgeo.2020.119628
Kelemen, P. B., Savage, H., and Hirth, G. (2013). “Reaction-driven cracking during mineral hydration, carbonation and oxidation,” in Poromechanics V: Proceedings of the Fifth Biot Conference on Poromechanics, 823–826.
Kheshgi, H. S. (1995). Sequestering atmospheric carbon dioxide by increasing ocean alkalinity. Energy 20, 915–922. doi: 10.1016/0360-5442(95)00035-F
Kim, I. G., Jo, B. H., Kang, D. G., Kim, C. S., Choi, Y. S., and Cha, H. J. (2012). Biomineralization-based conversion of carbon dioxide to calcium carbonate using recombinant carbonic anhydrase. Chemosphere 87, 1091–1096. doi: 10.1016/j.chemosphere.2012.02.003
King, H. E., Plümper, O., and Putnis, A. (2010). Effect of secondary phase formation on the carbonation of olivine. Environ. Sci. Technol. 44, 6503–6509. doi: 10.1021/es9038193
Kirchner, J. S., Lettmann, K. A., Schnetger, B., Wolff, J.-O., and Brumsack, H.-J. (2020). Carbon capture via accelerated weathering of limestone: modeling local impacts on the carbonate chemistry of the southern North Sea. Int. J. Greenhouse Gas Control 92, 102855. doi: 10.1016/j.ijggc.2019.102855
Knight, M. J., Senior, L., Nancolas, B., Ratcliffe, S., and Curnow, P. (2016). Direct evidence of the molecular basis for biological silicon transport. Nat. Commun. 7, 11926. doi: 10.1038/ncomms11926
Knoche, W. (1980). “Chemical reactions of CO2 in water,” in Biophysics and Physiology of Carbon Dioxide (Berlin; Heidelberg: Springer), 3–11.
Köhler, P., Abrams, J. F., Völker, C., Hauck, J., and Wolf-Gladrow, D. A. (2013). Geoengineering impact of open ocean dissolution of olivine on atmospheric CO2, surface ocean pH and marine biology. Environ. Res. Lett. 8, 014009. doi: 10.1088/1748-9326/8/1/014009
Kohler, P., Hartmann, J., and Wolf-Gladrow, D. A. (2010). Geoengineering potential of artificially enhanced silicate weathering of olivine. Proc. Natl. Acad. Sci. U.S.A. 107, 20228–20233. doi: 10.1073/pnas.1000545107
Krajewska, B. (2018). Urease-aided calcium carbonate mineralization for engineering applications: a review. J. Advert. Res. 13, 59–67. doi: 10.1016/j.jare.2017.10.009
Krevor, S. C., and Lackner, K. S. (2009). Enhancing process kinetics for mineral carbon sequestration. Energy Procedia 1, 4867–4871. doi: 10.1016/j.egypro.2009.02.315
Kularatne, K., Sissmann, O., Kohler, E., Chardin, M., Noirez, S., and Martinez, I. (2018). Simultaneous ex-situ CO2 mineral sequestration and hydrogen production from olivine-bearing mine tailings. Appl. Geochem. 95, 195–205. doi: 10.1016/j.apgeochem.2018.05.020
Kwon, S., Fan, M., DaCosta, H. F. M., and Russell, A. G. (2011). Factors affecting the direct mineralization of CO2 with olivine. J. Environ. Sci. 23, 1233–1239. doi: 10.1016/s1001-0742(10)60555-4
La Plante, E. C., Mehdipour, I., Shortt, I., Yang, K., Simonetti, D., Bauchy, M., et al. (2021a). Controls on CO2 mineralization using natural and industrial alkaline solids under ambient conditions. ACS Sustain. Chem. Eng. 9, 10727–10739. doi: 10.1021/acssuschemeng.1c00838
La Plante, E. C., Simonetti, D. A., Wang, J., Al-Turki, A., Chen, X., and Jassby, D. (2021b). Saline water-based mineralization pathway for gigatonne-scale CO2 management. ACS Sustain. Chem. Eng. 9, 1073–1089. doi: 10.1021/acssuschemeng.0c08561
Lackner, K. S. (2003). Climate change. A guide to CO2 sequestration. Science 300, 1677–1678. doi: 10.1126/science.1079033
Lackner, K. S., Wendt, C. H., Butt, D. P., Joyce, E. L., and Sharp, D. H. (1995). Carbon dioxide disposal in carbonate minerals. Energy 20, 1153–1170. doi: 10.1016/0360-5442(95)00071-N
Larachi, F., Daldoul, I., and Beaudoin, G. (2010). Fixation of CO2 by chrysotile in low-pressure dry and moist carbonation: ex-situ and in-situ characterizations. Geochim. Cosmochim. Acta 74, 3051–3075. doi: 10.1016/j.gca.2010.03.007
Larachi, F., Gravel, J.-P., Grandjean, B. P. A., and Beaudoin, G. (2012). Role of steam, hydrogen and pretreatment in chrysotile gas–solid carbonation: opportunities for pre-combustion CO2 capture. Int. J. Greenhouse Gas Control 6, 69–76. doi: 10.1016/j.ijggc.2011.10.010
Lasaga, A. C. (1998). Kinetic Theory in the Earth Sciences. Princeton University Press. Available online at: http://www.jstor.org/stable/j.ctt7zvgxm
Lawson, C. E., Harcombe, W. R., Hatzenpichler, R., Lindemann, S. R., Löffler, F. E., O'Malley, M. A., et al. (2019). Common principles and best practices for engineering microbiomes. Nat. Rev. Microbiol. 17, 725–741. doi: 10.1038/s41579-019-0255-9
Lazo, D. E., Dyer, L. G., and Alorro, R. D. (2017). Silicate, phosphate and carbonate mineral dissolution behaviour in the presence of organic acids: a review. Miner. Eng. 100, 115–123. doi: 10.1016/j.mineng.2016.10.013
Lechner, C. C., and Becker, C. F. W. (2015). Silaffins in silica biomineralization and biomimetic silica precipitation. Mar. Drugs 13, 5297–5333. doi: 10.3390/md13085297
Lee, J. W., Chan, C. T. Y., Slomovic, S., and Collins, J. J. (2018). Next-generation biocontainment systems for engineered organisms. Nat. Chem. Biol. 14, 530–537. doi: 10.1038/s41589-018-0056-x
Lefebvre, D., Goglio, P., Williams, A., Manning, D. A. C., de Azevedo, A. C., Bergmann, M., et al. (2019). Assessing the potential of soil carbonation and enhanced weathering through Life Cycle Assessment: a case study for Sáo Paulo State, Brazil. J. Clean. Prod. 233, 468–481. doi: 10.1016/j.jclepro.2019.06.099
Legendre, D., and Zevenhoven, R. (2017). Detailed experimental study on the dissolution of CO2 and air bubbles rising in water. Chem. Eng. Sci. 158, 552–560. doi: 10.1016/j.ces.2016.11.004
Leonardos, O. H., Fyfe, W. S., and Kronberg, B. I. (1987). The use of ground rocks in laterite systems: an improvement to the use of conventional soluble fertilizers? Chem. Geol. 60, 361–370. doi: 10.1016/0009-2541(87)90143-4
Li, C., Zhong, S., Zhang, F., Wang, Z., Jiang, F., and Wan, Y. (2017). Response of microbial communities to supercritical CO 2 and biogeochemical influences on microbially mediated CO 2 -saline-sandstone interactions. Chem. Geol. 473, 1–9. doi: 10.1016/j.chemgeo.2017.09.026
Li, J., and Hitch, M. (2018). Mechanical activation of magnesium silicates for mineral carbonation, a review. Miner. Eng. 128, 69–83. doi: 10.1016/j.mineng.2018.08.034
Li, J., Jacobs, A. D., and Hitch, M. (2019). Direct aqueous carbonation on olivine at a CO2 partial pressure of 6.5 MPa. Energy 173, 902–910. doi: 10.1016/j.energy.2019.02.125
Li, W., and Wright, M. M. (2020). Negative emission energy production technologies: a techno-economic and life cycle analyses review. Energy Technol. 8, 1900871. doi: 10.1002/ente.201900871
Li, Y., and Sun, R. (2014). Studies on adsorption of carbon dioxide on alkaline paper mill waste using cyclic process. Energy Convers. Manage. 82, 46–53. doi: 10.1016/j.enconman.2014.03.004
Li, Z. (2020). General rate equation theory for gas–solid reaction kinetics and its application to CaO carbonation. Chem. Eng. Sci. 227, 115902. doi: 10.1016/j.ces.2020.115902
Li, Z., Fall, M., and Ghirian, A. (2018). CCS risk assessment: groundwater contamination caused by CO2. Geosci. J. 8, 397. doi: 10.3390/geosciences8110397
Liermann, L. J., Kalinowski, B. E., Brantley, S. L., and Ferry, J. G. (2000). Role of bacterial siderophores in dissolution of hornblende. Geochim. Cosmochim. Acta 64, 587–602. doi: 10.1016/S0016-7037(99)00288-4
Lindskog, S. (1997). Structure and mechanism of carbonic anhydrase. Pharmacol. Ther. 74, 1–20. doi: 10.1016/s0163-7258(96)00198-2
Lisabeth, H., Zhu, W. L., Xing, T. G., and De Andrade, V. (2017). Dissolution-assisted pattern formation during olivine carbonation. Geophys. Res. Lett. 44, 9622–9631. doi: 10.1002/2017GL074393
Liu, G., Schollbach, K., Li, P., and Brouwers, H. J. H. (2021). Valorization of converter steel slag into eco-friendly ultra-high performance concrete by ambient CO2 pre-treatment. Construct. Build. Mater. 280, 122580. doi: 10.1016/j.conbuildmat.2021.122580
Liu, W., Su, S., Xu, K., Chen, Q., Xu, J., Sun, Z., et al. (2018). CO2 sequestration by direct gas–solid carbonation of fly ash with steam addition. J. Clean. Prod. 178, 98–107. doi: 10.1016/j.jclepro.2017.12.281
Lombardi, L., Carnevale, E. A., and Pecorini, I. (2016). Experimental evaluation of two different types of reactors for CO2 removal from gaseous stream by bottom ash accelerated carbonation. Waste Manag. 58, 287–298. doi: 10.1016/j.wasman.2016.09.038
Longo, R. C., Cho, K., Brüner, P., Welle, A., Gerdes, A., and Thissen, P. (2015). Carbonation of wollastonite(001) competing hydration: microscopic insights from ion spectroscopy and density functional theory. ACS Appl. Mater. Interfaces 7, 4706–4712. doi: 10.1021/am508313g
Low Carbon Fuel Standard. Available online at: https://ww2.arb.ca.gov/our-work/programs/low-carbon-fuel-standard (accessed February 14, 2022).
Lu, L., Guest, J. S., Peters, C. A., Zhu, X., Rau, G. H., and Ren, Z. J. (2018). Wastewater treatment for carbon capture and utilization. Nat. Sustain. 1, 750–758. doi: 10.1038/s41893-018-0187-9
Luo, Y., and He, D. (2021). Research status and future challenge for CO2 sequestration by mineral carbonation strategy using iron and steel slag. Environ. Sci. Pollut. Res. Int. 28, 49383–49409. doi: 10.1007/s11356-021-15254-x
Lüttge, A., and Conrad, P. G. (2004). Direct observation of microbial inhibition of calcite dissolution. Appl. Environ. Microbiol. 70, 1627–1632. doi: 10.1128/AEM.70.3.1627-1632.2004
Ma, C., Wu, R., Huang, R., Jiang, W., You, C., Zhu, L., et al. (2019). Directed evolution of a 6-phosphogluconate dehydrogenase for operating an enzymatic fuel cell at lowered anodic pHs. J. Electroanal. Chem. 851, 113444. doi: 10.1016/j.jelechem.2019.113444
Madhavan, A., Arun, K. B., Binod, P., Sirohi, R., Tarafdar, A., Reshmy, R., et al. (2021). Design of novel enzyme biocatalysts for industrial bioprocess: harnessing the power of protein engineering, high throughput screening and synthetic biology. Bioresour. Technol. 325, 124617. doi: 10.1016/j.biortech.2020.124617
Maguire, M. E. (2006). Magnesium transporters: properties, regulation and structure. Front. Biosci. 11, 3149–3163. doi: 10.2741/2039
Maher, K., Steefel, C. I., White, A. F., and Stonestrom, D. A. (2009). The role of reaction affinity and secondary minerals in regulating chemical weathering rates at the Santa Cruz Soil Chronosequence, California. Geochim. Cosmochim. Acta 73, 2804–2831. doi: 10.1016/j.gca.2009.01.030
Mamo, G., and Mattiasson, B. (2016). “Alkaliphilic microorganisms in biotechnology,” in Biotechnology of Extremophiles: Advances and Challenges, ed P. H. Rampelotto (Cham: Springer International Publishing), 243–272.
Manning, D. A. C., and Renforth, P. (2013). Passive sequestration of atmospheric CO2 through coupled plant-mineral reactions in urban soils. Environ. Sci. Technol. 47, 135–141. doi: 10.1021/es301250j
Mant, R. C., Jones, D. L., Reynolds, B., Ormerod, S. J., and Pullin, A. S. (2013). A systematic review of the effectiveness of liming to mitigate impacts of river acidification on fish and macro-invertebrates. Environ. Pollut. 179, 285–293. doi: 10.1016/j.envpol.2013.04.019
Marieni, C., Prikryl, J., Aradóttir, E. S., Gunnarsson, I., and Stefánsson, A. (2018). Towards “green” geothermal energy: co-mineralization of carbon and sulfur in geothermal reservoirs. Int. J. Greenhouse Gas Control 77, 96–105. doi: 10.1016/j.ijggc.2018.07.011
Marin, F. (2020). Mollusc shellomes: past, present and future. J. Struct. Biol. 212, 107583. doi: 10.1016/j.jsb.2020.107583
Martin, D., Dodds, K., Butler, I. B., and Ngwenya, B. T. (2013). Carbonate precipitation under pressure for bioengineering in the anaerobic subsurface via denitrification. Environ. Sci. Technol. 47, 8692–8699. doi: 10.1021/es401270q
Martínez, I., Arias, B., Grasa, G. S., and Abanades, J. C. (2018). CO2 capture in existing power plants using second generation Ca-looping systems firing biomass in the calciner. J. Clean. Prod. 187, 638–649. doi: 10.1016/j.jclepro.2018.03.189
Martin-Jezequel, V., Hildebrand, M., and Brzezinski, M. A. (2000). Silicon metabolism in diatoms: implications for growth. J. Phycol. 36, 821–840. doi: 10.1046/j.1529-8817.2000.00019.x
Matter, J. M., Broecker, W. S., Stute, M., Gislason, S. R., Oelkers, E. H., Stefánsson, A., et al. (2009). Permanent carbon dioxide storage into basalt: the CarbFix Pilot Project, Iceland. Energy Procedia 1, 3641–3646. doi: 10.1016/j.egypro.2009.02.160
Matter, J. M., and Kelemen, P. B. (2009). Permanent storage of carbon dioxide in geological reservoirs by mineral carbonation. Nat. Geosci. 2, 837–841. doi: 10.1038/ngeo683
Matter, J. M., Stute, M., Snæbjörnsdottir, S., Oelkers, E. H., Gislason, S. R., Aradottir, E. S., et al. (2016). Rapid carbon mineralization for permanent disposal of anthropogenic carbon dioxide emissions. Science 352, 1312–1314. doi: 10.1126/science.aad8132
Mayes, W. M., Riley, A. L., Gomes, H. I., Brabham, P., Hamlyn, J., Pullin, H., et al. (2018). Atmospheric CO2 sequestration in iron and steel slag: Consett, County Durham, United Kingdom. Environ. Sci. Technol. 52, 7892–7900. doi: 10.1021/acs.est.8b01883
Mayes, W. M., Younger, P. L., and Aumônier, J. (2008). Hydrogeochemistry of alkaline steel slag leachates in the UK. Water Air Soil Pollut. 195, 35–50. doi: 10.1007/s11270-008-9725-9
McCutcheon, J., Dipple, G. M., Wilson, S. A., and Southam, G. (2015). Production of magnesium-rich solutions by acid leaching of chrysotile: a precursor to field-scale deployment of microbially enabled carbonate mineral precipitation. Chem. Geol. 413, 119–131. doi: 10.1016/j.chemgeo.2015.08.023
McCutcheon, J., Power, I. M., Harrison, A. L., Dipple, G. M., and Southam, G. (2014). A greenhouse-scale photosynthetic microbial bioreactor for carbon sequestration in magnesium carbonate minerals. Environ. Sci. Technol. 48, 9142–9151. doi: 10.1021/es500344s
McCutcheon, J., Power, I. M., Shuster, J., Harrison, A. L., Dipple, G. M., and Southam, G. (2019). Carbon sequestration in biogenic magnesite and other magnesium carbonate minerals. Environ. Sci. Technol. 53, 3225–3237. doi: 10.1021/acs.est.8b07055
McCutcheon, J., Turvey, C. C., Wilson, S. A., Hamilton, J. L., and Southam, G. (2017). Experimental deployment of microbial mineral carbonation at an asbestos mine: potential applications to carbon storage and tailings stabilization. Minerals 7, 191. doi: 10.3390/min7100191
McCutcheon, J., Wilson, S. A., and Southam, G. (2016). Microbially accelerated carbonate mineral precipitation as a strategy for in situ carbon sequestration and rehabilitation of asbestos mine sites. Environ. Sci. Technol. 50, 1419–1427. doi: 10.1021/acs.est.5b04293
McGrail, B. P., Schaef, H. T., Ho, A. M., Chien, Y.-J., Dooley, J. J., and Davidson, C. L. (2006). Potential for carbon dioxide sequestration in flood basalts. J. Geophys. Res. 111, 5653–5660. doi: 10.1029/2005jb004169
McGrail, B. P., Schaef, H. T., Spane, F. A., Cliff, J. B., Qafoku, O., Horner, J. A., et al. (2017a). Field validation of supercritical CO2 reactivity with basalts. Environ. Sci. Technol. Lett. 4, 6–10. doi: 10.1021/acs.estlett.6b00387
McGrail, B. P., Schaef, H. T., Spane, F. A., Horner, J. A., Owen, A. T., Cliff, J. B., et al. (2017b). Wallula Basalt pilot demonstration project: post-injection results and conclusions. Energy Procedia 114, 5783–5790. doi: 10.1016/j.egypro.2017.03.1716
McGrail, B. P., Spane, F. A., Amonette, J. E., Thompson, C. R., and Brown, C. F. (2014). Injection and monitoring at the Wallula Basalt pilot project. Energy Procedia 63, 2939–2948. doi: 10.1016/j.egypro.2014.11.316
McGrail, B. P., Spane, F. A., Sullivan, E. C., Bacon, D. H., and Hund, G. (2011). The Wallula basalt sequestration pilot project. Energy Procedia 4, 5653–5660. doi: 10.1016/j.egypro.2011.02.557
McKelvy, M. J., Chizmeshya, A. V. G., and Bearat, H. (2001). “Developing mechanistic understanding of CO2 mineral sequestration reaction processes,” in Tech. Conference. Available online at: https://www.researchgate.net/profile/Hamdallah-Bearat/publication/282019071_Developing_a_Mechanistic_Understanding_of_CO2_Mineral_Sequestration_Reaction_Processes/links/563b36af08aeed0531dd6e33/Developing-a-Mechanistic-Understanding-of-CO2-Mineral-Sequestration-Reaction-Processes.pdf
McQueen, N., Gomes, K. V., McCormick, C., Blumanthal, K., Pisciotta, M., and Wilcox, J. (2021a). A review of direct air capture (DAC): scaling up commercial technologies and innovating for the future. Prog. Energy Combust. Sci. 3, 032001. doi: 10.1088/2516-1083/abf1ce
McQueen, N., Kelemen, P., Dipple, G., Renforth, P., and Wilcox, J. (2020). Ambient weathering of magnesium oxide for CO2 removal from air. Nat. Commun. 11, 1–10. doi: 10.1038/s41467-020-16510-3
McQueen, N., Kolosz, B., Psarras, P., and McCormick, C. (2021b). Analysis and quantification of negative emissions. Life 4, 1. Available online at: https://cdrprimer.org/about
Medas, D., Cappai, G., De Giudici, G., Piredda, M., and Podda, S. (2017). Accelerated carbonation by cement kiln dust in aqueous slurries: chemical and mineralogical investigation. Greenh. Gases Sci. Technol. 7, 692–705. doi: 10.1002/ghg.1681
Mervine, E. M., Wilson, S. A., Power, I. M., Dipple, G. M., Turvey, C. C., Hamilton, J. L., et al. (2018). Potential for offsetting diamond mine carbon emissions through mineral carbonation of processed kimberlite: an assessment of De Beers mine sites in South Africa and Canada. Mineral. Petrol. 112, 755–765. doi: 10.1007/s00710-018-0589-4
Mesbahuddin, M. S., Ganesan, A., and Kalyaanamoorthy, S. (2021). Engineering stable carbonic anhydrases for CO2 capture: a critical review. Protein Eng. Des. Sel. 34, gzab021. doi: 10.1093/protein/gzab021
Meysman, F. J. R., and Montserrat, F. (2017). Negative CO2 emissions via enhanced silicate weathering in coastal environments. Biol. Lett. 13, 20160905. doi: 10.1098/rsbl.2016.0905
Minx, J. C., Lamb, W. F., Callaghan, M. W., Fuss, S., Hilaire, J., Creutzig, F., et al. (2018). Negative emissions — part 1: research landscape and synthesis. Environ. Res. Lett. 13, 063001. doi: 10.1088/1748-9326/aabf9b
Mitchell, A. C., Dideriksen, K., Spangler, L. H., Cunningham, A. B., and Gerlach, R. (2010). Microbially enhanced carbon capture and storage by mineral-trapping and solubility-trapping. Environ. Sci. Technol. 44, 5270–5276. doi: 10.1021/es903270w
Mitchell, A. C., Phillips, A., Schultz, L., Parks, S., Spangler, L., Cunningham, A. B., et al. (2013). Microbial CaCO3 mineral formation and stability in an experimentally simulated high pressure saline aquifer with supercritical CO2. Int. J. Greenhouse Gas Control 15, 86–96. doi: 10.1016/j.ijggc.2013.02.001
Miyamoto, H., Miyoshi, F., and Kohno, J. (2005). The carbonic anhydrase domain protein nacrein is expressed in the epithelial cells of the mantle and acts as a negative regulator in calcification in the mollusc Pinctada fucata. Zool. Sci. 22, 311–315. doi: 10.2108/zsj.22.311
Monfreda, C., Ramankutty, N., and Foley, J. A. (2008). Farming the planet: 2. Geographic distribution of crop areas, yields, physiological types, and net primary production in the year 2000. Global Biogeochem. Cycles 22. doi: 10.1029/2007GB002947
Montes-Hernandez, G., Pérez-López, R., Renard, F., Nieto, J. M., and Charlet, L. (2009). Mineral sequestration of CO2 by aqueous carbonation of coal combustion fly-ash. J. Hazard. Mater. 161, 1347–1354. doi: 10.1016/j.jhazmat.2008.04.104
Montserrat, F., Renforth, P., Hartmann, J., Leermakers, M., Knops, P., and Meysman, F. J. R. (2017). Olivine Dissolution in Seawater: implications for CO2 Sequestration through Enhanced Weathering in Coastal Environments. Environ. Sci. Technol. 51, 3960–3972. doi: 10.1021/acs.est.6b05942
Moore, D. (2021). Saving the planet with appropriate biotechnology: 4. Coccolithophore cultivation and deployment. Mexican J. Biotechnol. 6, 129–155. doi: 10.29267/mxjb.2021.6.1.129
Moosdorf, N., Renforth, P., and Hartmann, J. (2014). Carbon dioxide efficiency of terrestrial enhanced weathering. Environ. Sci. Technol. 48, 4809–4816. doi: 10.1021/es4052022
Morel, F. M. M., and Hering, J. G. (1993). Principles and Applications of Aquatic Chemistry. Available online at: https://books.google.com/books?hl=en&lr=&id=lgXpDwAAQBAJ&oi=fnd&pg=PR11&ots=Xa_R5R22vq&sig=KWOq_M1dWuT7vPjBW08ifKQzKok
Morgan, M. D. (1987). Impact of nutrient enrichment and alkalinization on periphyton communities in the New Jersey Pine Barrens. Hydrobiologia 144, 233–241. doi: 10.1007/BF00005557
Morse, J. W., Arvidson, R. S., and Lüttge, A. (2007). Calcium carbonate formation and dissolution. epsc511.wustl.edu 107, 342–381. doi: 10.1021/cr050358j
Müller, L. J., Kätelhön, A., Bachmann, M., Zimmermann, A., Sternberg, A., and Bardow, A. (2020). A guideline for life cycle assessment of carbon capture and utilization. Front. Energy Res. 8, 15. doi: 10.3389/fenrg.2020.00015
Müller, W. E. G., Schröder, H. C., Burghard, Z., Pisignano, D., and Wang, X. (2013a). Silicateins–a novel paradigm in bioinorganic chemistry: enzymatic synthesis of inorganic polymeric silica. Chemistry 19, 5790–5804. doi: 10.1002/chem.201204412
Müller, W. E. G., Schröder, H. C., Schlossmacher, U., Neufurth, M., Geurtsen, W., Korzhev, M., et al. (2013b). The enzyme carbonic anhydrase as an integral component of biogenic Ca-carbonate formation in sponge spicules. FEBS Open Bio 3, 357–362. doi: 10.1016/j.fob.2013.08.004
Mustafa, J., Mourad, A. A. H. I., Al-Marzouqi, A. H., and El-Naas, M. H. (2020). Simultaneous treatment of reject brine and capture of carbon dioxide: a comprehensive review. Desalination 483, 114386. doi: 10.1016/j.desal.2020.114386
Myers, C., and Nakagaki, T. (2020). Direct mineralization of atmospheric CO 2 using natural rocks in Japan Environmental Research Letters OPEN ACCESS RECEIVED Direct mineralization of atmospheric CO 2 using natural rocks in Japan. Environ. Res. Lett. 15, 124018. doi: 10.1088/1748-9326/abc217
Myers, C. A., Nakagaki, T., and Akutsu, K. (2019). Quantification of the CO2 mineralization potential of ironmaking and steelmaking slags under direct gas-solid reactions in flue gas. Int. J. Greenhouse Gas Control 87, 100–111. doi: 10.1016/j.ijggc.2019.05.021
National Academies of Sciences, Engineering, and Medicine (2019). Negative Emissions Technologies and Reliable Sequestration: A Research Agenda. Washington, DC: The National Academies Press. doi: 10.17226/25259
Ncongwane, M. S., Broadhurst, J. L., and Petersen, J. (2018). Assessment of the potential carbon footprint of engineered processes for the mineral carbonation of PGM tailings. Int. J. Greenhouse Gas Control 77, 70–81. doi: 10.1016/j.ijggc.2018.07.019
Nduagu, E., Björklöf, T., Fagerlund, J., Wärnå, J., Geerlings, H., and Zevenhoven, R. (2012). Production of magnesium hydroxide from magnesium silicate for the purpose of CO2 mineralisation – part 1: application to Finnish serpentinite. Miner. Eng. 30, 75–86. doi: 10.1016/j.mineng.2011.12.004
Nikulshina, V., Ayesa, N., Galvez, M. E., and Steinfeld, A. (2008). Feasibility of Na-based thermochemical cycles for the capture of CO2 from air—thermodynamic and thermogravimetric analyses. Chem. Eng. J. 140, 62–70. doi: 10.1016/j.cej.2007.09.007
Nordstrom, D., and Southam, G. (1997). “Chapter 11. Geomicrobiology of sulfide mineral oxidation,” in Geomicrobiology (De Gruyter), 361–390. Available online at: https://www.degruyter.com/document/doi/10.1515/9781501509247-013/html (accessed January 11, 2022).
OCO Technology (2021). OCO Technology Chosen as a Key Partner for Breakthrough DAC Technology Programme. Available online at: https://oco.co.uk/o-c-o-technology-chosen-as-key-partner-for-breakthrough-dac-technology-programme/#:~:text=O.C.O%20Technology%20chosen%20as
%20key%20partner%20for%20breakthrough%20DAC%20technology%20programme,-25th%20May%202021&text=Carbon%20capture%20specialist%20O.C.O
%20Technology,Air%20Capture%20(DAC)%20technology
O'Connor, W. K., Dahlin, D. C., Nilsen, D. N., and Walters, R. P. (2000). Carbon Dioxide Sequestration by Direct Aqueous Mineral Carbonation. Available online at: https://www.osti.gov/biblio/897123
O'Connor, W. K., Nielsen, D. N., Gerdemann, S. J., Rush, G. E., Waltera, R. P., and Turner, P. C. (2001). 18th Annual International Pittsburgh Coal Conference. Newcastle, NSW.
Oelkers, E. H., Benning, L. G., Lutz, S., Mavromatis, V., Pearce, C. R., and Plümper, O. (2015). The efficient long-term inhibition of forsterite dissolution by common soil bacteria and fungi at Earth surface conditions. Geochim. Cosmochim. Acta 168, 222–235. doi: 10.1016/j.gca.2015.06.004
Oelkers, E. H., Declercq, J., Saldi, G. D., Gislason, S. R., and Schott, J. (2018). Olivine dissolution rates: a critical review. Chem. Geol. 500, 1–19. doi: 10.1016/j.chemgeo.2018.10.008
Okamoto, I., Mizuochi, Y., Ninomiya, A., Kato, T., Yajima, T., and Ohsumi, T. (2006). “In-situ test on CO2 fixation by serpentinite rock mass in Japan,” in 8th International Conference on Greenhouse Gas Control Technologies (GHGT-8), 19–22 June 2006, Trondheim, Norway (Amsterdam: Elsevier), 475–480. Available online at: https://www.researchgate.net/profile/Koichi-Kato-4/publication/290396270_In-situ_Test_on_CO2_Fixation_by_Serpentinite_Rock_Mass_in_Japan/links/5696f94108ae34f3cf1deb5f/In-situ-Test-on-CO2-Fixation-by-Serpentinite-Rock-Mass-in-Japan.pdf
Okesola, A. A., Oyedeji, A. A., Abdulhamid, A. F., Olowo, J., Ayodele, B. E., and Alabi, T. W. (2018). “Direct air capture: A review of carbon dioxide capture from the air,” in IOP Conference Series: Materials Science and Engineering, Vol. 413 (Ota: IOP Publishing). doi: 10.1088/1757-899X/413/1/012077
Olasolo, P., Juárez, M. C., Morales, M. P., D'Amico, S., and Liarte, I. A. (2016). Enhanced geothermal systems (EGS): a review. Renew. Sustain. Energy Rev. 56, 133–144. doi: 10.1016/j.rser.2015.11.031
Packer, M. S., and Liu, D. R. (2015). Methods for the directed evolution of proteins. Nat. Rev. Genet. 16, 379–394. doi: 10.1038/nrg3927
Palandri, J. L., and Kharaka, Y. K. (2005). Ferric iron-bearing sediments as a mineral trap for CO 2 sequestration: iron reduction using sulfur-bearing waste gas. Chem. Geol. 217, 351–364. doi: 10.1016/j.chemgeo.2004.12.018
Paquay, F. S., and Zeebe, R. E. (2013). Assessing possible consequences of ocean liming on ocean pH, atmospheric CO2 concentration and associated costs. Int. J. Greenhouse Gas Control 17, 183–188. doi: 10.1016/j.ijggc.2013.05.005
Park, A.-H. A., and Fan, L.-S. (2004). CO2 mineral sequestration: physically activated dissolution of serpentine and pH swing process. Chem. Eng. Sci. 59, 5241–5247. doi: 10.1016/j.ces.2004.09.008
Paukert, A. N., Matter, J. M., Kelemen, P. B., Shock, E. L., and Havig, J. R. (2012). Reaction path modeling of enhanced in situ CO2 mineralization for carbon sequestration in the peridotite of the Samail Ophiolite, Sultanate of Oman. Chem. Geol. 330–331, 86–100. doi: 10.1016/j.chemgeo.2012.08.013
Pedraza, J., Zimmermann, A., Tobon, J., Schomäcker, R., and Rojas, N. (2021). On the road to net zero-emission cement: integrated assessment of mineral carbonation of cement kiln dust. Chem. Eng. J. 408, 127346. doi: 10.1016/j.cej.2020.127346
Peet, K. C., Freedman, A. J. E., Hernandez, H. H., Britto, V., Boreham, C., Ajo-Franklin, J. B., et al. (2015). Microbial growth under supercritical CO2. Appl. Environ. Microbiol. 81, 2881–2892. doi: 10.1128/AEM.03162-14
Perakis, S. S., and Pett-Ridge, J. C. (2019). Nitrogen-fixing red alder trees tap rock-derived nutrients. Proc. Natl. Acad. Sci. U.S.A. 116, 5009–5014. doi: 10.1073/pnas.1814782116
Pérez-López, R., Montes-Hernandez, G., Nieto, J. M., Renard, F., and Charlet, L. (2008). Carbonation of alkaline paper mill waste to reduce CO2 greenhouse gas emissions into the atmosphere. Appl. Geochem. 23, 2292–2300. doi: 10.1016/j.apgeochem.2008.04.016
Peuble, S., Andreani, M., Gouze, P., Pollet-Villard, M., Reynard, B., and Van de Moortele, B. (2018). Multi-scale characterization of the incipient carbonation of peridotite. Chem. Geol. 476, 150–160. doi: 10.1016/j.chemgeo.2017.11.013
Phan, T. N., Gogri, M. P., Kabir, C. S., and Reza, Z. A. (2018). Exploring safe disposal of CO2 and wastewater in saline aquifers. J. Pet. Sci. Eng. 170, 197–205. doi: 10.1016/j.petrol.2018.06.065
Picazo, S., Malvoisin, B., Baumgartner, L., and Bouvier, A. S. (2020). Low temperature serpentinite replacement by carbonates during seawater influx in the Newfoundland Margin. Minerals. Available online at: https://www.mdpi.com/643828
Pilorgé, H., Kolosz, B., Wu, G. C., and Freeman, J. (2021). “Global mapping of CDR opportunities,” in Carbon Dioxide Removal Primer (cdrprimer.org). Available online at: https://cdrprimer.org/read/chapter-3
Pokharel, R., Gerrits, R., Schuessler, J. A., and von Blanckenburg, F. (2019). Mechanisms of olivine dissolution by rock-inhabiting fungi explored using magnesium stable isotopes. Chem. Geol. 525, 18–27. doi: 10.1016/j.chemgeo.2019.07.001
Pokrovsky, O. S., Schott, J., and Castillo, A. (2005). Kinetics of brucite dissolution at 25°C in the presence of organic and inorganic ligands and divalent metals. Geochim. Cosmochim. Acta 69, 905–918. doi: 10.1016/j.gca.2004.08.011
Pokrovsky, O. S., Shirokova, L. S., Bénézeth, P., Schott, J., and Golubev, S. V. (2009). Effect of organic ligands and heterotrophic bacteria on wollastonite dissolution kinetics. Am. J. Sci. 309, 731–772. doi: 10.2475/08.2009.05
Pokrovsky, O. S., Shirokova, L. S., Zabelina, S. A., Jordan, G., and Bénézeth, P. (2021). Weak impact of microorganisms on Ca, Mg-bearing silicate weathering. NPJ Mater. Degradation 5, 1–10. doi: 10.1038/s41529-021-00199-w
Pontiga, F., Valverde, J. M., Moreno, H., and Duran-Olivencia, F. J. (2013). Dry gas–solid carbonation in fluidized beds of Ca(OH)2 and nanosilica/Ca(OH)2 at ambient temperature and low CO2 pressure. Chem. Eng. J. 222, 546–552. doi: 10.1016/j.cej.2013.02.067
Power, I. M., Dipple, G. M., Bradshaw, P., and Harrison, A. L. (2020). Prospects for CO2 mineralization and enhanced weathering of ultramafic mine tailings from the Baptiste nickel deposit in British Columbia, Canada. Int. J. Greenhouse Gas Control 94, 102895. doi: 10.1016/j.ijggc.2019.102895
Power, I. M., Dipple, G. M., and Southam, G. (2010). Bioleaching of ultramafic tailings by Acidithiobacillus spp. for CO2 sequestration. Environ. Sci. Technol. 44, 456–462. doi: 10.1021/es900986n
Power, I. M., Harrison, A. L., and Dipple, G. M. (2016). Accelerating mineral carbonation using carbonic anhydrase. Environ. Sci. Technol. 50, 2610–2618. doi: 10.1021/acs.est.5b04779
Power, I. M., Harrison, A. L., Dipple, G. M., and Southam, G. (2013a). Carbon sequestration via carbonic anhydrase facilitated magnesium carbonate precipitation. Int. J. Greenhouse Gas Control 16, 145–155. doi: 10.1016/j.ijggc.2013.03.011
Power, I. M., Harrison, A. L., Dipple, G. M., Wilson, S. A., Kelemen, P. B., Hitch, M., et al. (2013b). Carbon mineralization: from natural analogues to engineered systems. Rev. Mineral. Geochem. 77, 305–306. doi: 10.2138/rmg.2013.77.9
Power, I. M., Kenward, P. A., Dipple, G. M., and Raudsepp, M. (2017). Room temperature magnesite precipitation. Cryst. Growth Des. 17, 5652–5659. doi: 10.1021/acs.cgd.7b00311
Power, I. M., McCutcheon, J., Harrison, A. L., Wilson, S. A., Dipple, G. M., Kelly, S., et al. (2014). Strategizing carbon-neutral mines: a case for pilot projects. Minerals 4, 399–436. doi: 10.3390/min4020399
Power, I. M., Wilson, S. A., Small, D. P., Dipple, G. M., Wan, W., and Southam, G. (2011). Microbially mediated mineral carbonation: roles of phototrophy and heterotrophy. Environ. Sci. Technol. 45, 9061–9068. doi: 10.1021/es201648g
Power, I. M., Wilson, S. A., Thom, J. M., Dipple, G. M., and Southam, G. (2007). Biologically induced mineralization of dypingite by cyanobacteria from an alkaline wetland near Atlin, British Columbia, Canada. Geochem. Trans. 8, 13. doi: 10.1186/1467-4866-8-13
Price, G. D., Badger, M. R., Woodger, F. J., and Long, B. M. (2008). Advances in understanding the cyanobacterial CO2-concentrating-mechanism (CCM): functional components, Ci transporters, diversity, genetic regulation and prospects for engineering into plants. J. Exp. Bot. 59, 1441–1461. doi: 10.1093/jxb/erm112
Prigiobbe, V., Polettini, A., and Baciocchi, R. (2009). Gas–solid carbonation kinetics of air pollution control residues for CO2 storage. Chem. Eng. J. 148, 270–278. doi: 10.1016/j.cej.2008.08.031
Pulido, C., Keijsers, D. J. H., Lucassen, E. C. H. E. T., Pedersen, O., and Roelofs, J. G. M. (2012). Elevated alkalinity and sulfate adversely affect the aquatic macrophyte Lobelia dortmanna. Aquatic Ecol. 46, 283–295. doi: 10.1007/s10452-012-9399-7
Pullin, H., Bray, A. W., Burke, I. T., Muir, D. D., Sapsford, D. J., Mayes, W. M., et al. (2019). Atmospheric carbon capture performance of legacy iron and steel waste. Environ. Sci. Technol. 53, 9502–9511. doi: 10.1021/acs.est.9b01265
Qiu, Q. (2020). A state-of-the-art review on the carbonation process in cementitious materials: Fundamentals and characterization techniques. Construct. Build. Mater. 247, 118503. doi: 10.1016/j.conbuildmat.2020.118503
Ramanan, R., Kannan, K., Deshkar, A., Yadav, R., and Chakrabarti, T. (2010). Enhanced algal CO(2) sequestration through calcite deposition by Chlorella sp. and Spirulina platensis in a mini-raceway pond. Bioresour. Technol. 101, 2616–2622. doi: 10.1016/j.biortech.2009.10.061
Ratouis, T. M. P., Snæbjörnsdóttir, S. Ó., Voigt, M. J., Sigfússon, B., Gunnarsson, G., Aradóttir, E. S., et al. (2022). Carbfix 2: a transport model of long-term CO2 and H2S injection into basaltic rocks at Hellisheidi, SW-Iceland. Int. J. Greenhouse Gas Control 114, 103586. doi: 10.1016/j.ijggc.2022.103586
Rau, G. H. (2011). CO2 mitigation via capture and chemical conversion in seawater. Environ. Sci. Technol. 45, 1088–1092. doi: 10.1021/es102671x
Rau, G. H., and Caldeira, K. (1999). Enhanced carbonate dissolution:: a means of sequestering waste CO2 as ocean bicarbonate. Energy Convers. Manage. 40, 1803–1813. doi: 10.1016/S0196-8904(99)00071-0
Rau, G. H., Knauss, K. G., Langer, W. H., and Caldeira, K. (2007). Reducing energy-related CO2 emissions using accelerated weathering of limestone. Energy 32, 1471–1477. doi: 10.1016/j.energy.2006.10.011
Reddy, K. R., Gopakumar, A., and Chetri, J. K. (2019). Critical review of applications of iron and steel slags for carbon sequestration and environmental remediation. Rev. Environ. Sci. Technol. 18, 127–152. doi: 10.1007/s11157-018-09490-w
Reinfelder, J. R. (2011). Carbon concentrating mechanisms in eukaryotic marine phytoplankton. Ann. Rev. Mar. Sci. 3, 291–315. doi: 10.1146/annurev-marine-120709-142720
Ren, C., Wen, X., Mencius, J., and Quan, S. (2019). Selection and screening strategies in directed evolution to improve protein stability. Bioresour. Bioprocess. 6, 1–14. doi: 10.1186/s40643-019-0288-y
Renforth, P. (2012). The potential of enhanced weathering in the UK. Int. J. Greenhouse Gas Control 10, 229–243. doi: 10.1016/j.ijggc.2012.06.011
Renforth, P. (2019). The negative emission potential of alkaline materials. Nat. Commun. 10, 1–8. doi: 10.1038/s41467-019-09475-5
Renforth, P., and Campbell, J. S. (2021). The role of soils in the regulation of ocean acidification. Philos. Trans. R. Soc. Lond. B Biol. Sci. 376. doi: 10.1098/rstb.2020.0174
Renforth, P., and Henderson, G. (2017). Assessing ocean alkalinity for carbon sequestration. Rev. Geophys. 55, 636–674. doi: 10.1002/2016RG000533
Renforth, P., Jenkins, B. G., and Kruger, T. (2013). Engineering challenges of ocean liming. Energy 60, 442–452. doi: 10.1016/j.energy.2013.08.006
Renforth, P., and Kruger, T. (2013). Coupling mineral carbonation and ocean liming. Energy Fuels 27, 4199–4207. doi: 10.1021/ef302030w
Ribeiro, I. D. A., Volpiano, C. G., Vargas, L. K., Granada, C. E., Lisboa, B. B., and Passaglia, L. M. P. (2020). Use of mineral weathering bacteria to enhance nutrient availability in crops: a review. Front. Plant Sci. 11, 590774. doi: 10.3389/fpls.2020.590774
Riding, R. (2006). Cyanobacterial calcification, carbon dioxide concentrating mechanisms, and Proterozoic–Cambrian changes in atmospheric composition. Geobiology 4, 299–316. doi: 10.1111/j.1472-4669.2006.00087.x
Rigopoulos, I., Vasiliades, M. A., Ioannou, I., Efstathiou, A. M., Godelitsas, A., and Kyratsi, T. (2016). Enhancing the rate of ex situ mineral carbonation in dunites via ball milling. Adv. Powder Technol. 27, 360–371. doi: 10.1016/j.apt.2016.01.007
Robbins, C. W. (1986). Carbon dioxide partial pressure in lysimeter soils 1. Agron. J. 78, 151–158. doi: 10.2134/agronj1986.00021962007800010031x
Robie, R. A., and Hemingway, B. S. (1995). Thermodynamic Properties of Minerals and Related Substances at 298.15 K and 1 Bar (105 Pascals) Pressure and at Higher Temperatures. U.S. Government Printing Office. Available online at: https://play.google.com/store/books/details?id=FOx0hxWSWUYC
Rodriguez-Navarro, C., Cizer, Ö., Kudłacz, K., Ibañez-Velasco, A., Ruiz-Agudo, C., Elert, K., et al. (2019). The multiple roles of carbonic anhydrase in calcium carbonate mineralization. CrystEngComm 21, 7407–7423. doi: 10.1039/C9CE01544B
Rogers, J. R., and Bennett, P. C. (2004). Mineral stimulation of subsurface microorganisms: release of limiting nutrients from silicates. Chem. Geol. 203, 91–108. doi: 10.1016/j.chemgeo.2003.09.001
Ruiz-Agudo, E., Putnis, C. V., Rodriguez-Navarro, C., and Putnis, A. (2011). Effect of pH on calcite growth at constant aCa2+/aCO32- ratio and supersaturation. Geochim. Cosmochim. Acta 75, 284–296. doi: 10.1016/j.gca.2010.09.034
Ryan, P. R., Delhaize, E., and Jones, D. L. (2001). Function and mechanism of organic anion exudation from plant roots. Annu. Rev. Plant Physiol. Plant Mol. Biol. 52, 527–560. doi: 10.1146/annurev.arplant.52.1.527
Rylott, E. L., and Bruce, N. C. (2020). How synthetic biology can help bioremediation. Curr. Opin. Chem. Biol. 58, 86–95. doi: 10.1016/j.cbpa.2020.07.004
Saldi, G. D., Daval, D., Morvan, G., and Knauss, K. G. (2013). The role of Fe and redox conditions in olivine carbonation rates: an experimental study of the rate limiting reactions at 90 and 150°C in open and closed systems. Geochim. Cosmochim. Acta 118, 157–183. doi: 10.1016/j.gca.2013.04.029
Saldi, G. D., Jordan, G., Schott, J., and Oelkers, E. H. (2009). Magnesite growth rates as a function of temperature and saturation state. Geochim. Cosmochim. Acta 73, 5646–5657. doi: 10.1016/j.gca.2009.06.035
Samari, M., Ridha, F., Manovic, V., Macchi, A., and Anthony, E. J. (2020). Direct capture of carbon dioxide from air via lime-based sorbents. Mitigation Adaptat. Strat. Global Change 25, 25–41. https://link.springer.com/article/10.1007/s11027-019-9845-0
Samuels, T., Bryce, C., Landenmark, H., Marie-Loudon, C., Nicholson, N., Stevens, A. H., et al. (2020). “Microbial weathering of minerals and rocks in natural environments,” in Biogeochemical Cycles: Ecological Drivers and Environmental Impact, 59–79. Available online at: https://agupubs.onlinelibrary.wiley.com/doi/abs/10.1002/9781119413332.ch3
Sánchez-Román, M., Romanek, C. S., Fernández-Remolar, D. C., Sánchez-Navas, A., McKenzie, J. A., Pibernat, R. A., et al. (2011). Aerobic biomineralization of Mg-rich carbonates: implications for natural environments. Chem. Geol. 281, 143–150. doi: 10.1016/j.chemgeo.2010.11.020
Sanna, A., and Maroto-valer, M. (2016). CO2 sequestration by ex-situ mineral carbonation. World Scientific. Available online at: https://play.google.com/store/books/details?id=hxWyDgAAQBAJ
Sanna, A., Uibu, M., Caramanna, G., Kuusikb, R., and Maroto-Valer, M. M. (2014). A review of mineral carbonation technologies to sequester CO2. Chem. Soc. Rev. 43, 8049–8080. doi: 10.1039/C4CS00035H
Santillan, E.-F. U., Shanahan, T. M., Omelon, C. R., Major, J. R., and Bennett, P. C. (2015). Isolation and characterization of a CO2-tolerant Lactobacillus strain from Crystal Geyser, Utah, U.S.A. Front. Earth Sci. 3, 41. doi: 10.3389/feart.2015.00041
Santos, R. M., and Van Gerven, T. (2011). Process intensification routes for mineral carbonation. Greenhouse Gases Sci. Technol. 1, 287–293. doi: 10.1002/ghg.36
Sanz-Pérez, E. S., Murdock, C. R., Didas, S. A., and Jones, C. W. (2016). Direct capture of CO2 from ambient air. Chem. Rev. 116, 11840–11876. doi: 10.1021/acs.chemrev.6b00173
Sardo, A., Orefice, I., Balzano, S., Barra, L., and Romano, G. (2021). Mini-review: potential of diatom-derived silica for biomedical applications. NATO Adv. Sci. Inst. Ser. E Appl. Sci. 11, 4533. doi: 10.3390/app11104533
Schippers, A., Hedrich, S., Vasters, J., Drobe, M., Sand, W., and Willscher, S. (2014). “Biomining: metal recovery from ores with microorganisms,” in Geobiotechnology I: Metal-related Issues, eds A. Schippers, F. Glombitza, and W. Sand (Berlin: Springer Berlin Heidelberg), 1–47.
Schmalenberger, A., Duran, A. L., Bray, A. W., Bridge, J., Bonneville, S., Benning, L. G., et al. (2015). Oxalate secretion by ectomycorrhizal Paxillus involutus is mineral-specific and controls calcium weathering from minerals. Sci. Rep. 5, 12187. doi: 10.1038/srep12187
Schott, J., Pokrovsky, O. S., and Oelkers, E. H. (2009). The link between mineral dissolution/precipitation kinetics and solution chemistry. Rev. Mineral. Geochem. 70, 207–258. doi: 10.2138/rmg.2009.70.6
Schröer, H. C., Krasko, A., Pennec, G. L., Adell, T., Wiens, M., Hassanein, H., et al. (2003). “Silicase, an enzyme which degrades biogenous amorphous silica: contribution to the metabolism of silica deposition in the demosponge Suberites domuncula,” in Silicon Biomineralization: Biology — Biochemistry — Molecular Biology — Biotechnology, ed W. E. G. Müller (Berlin: Springer Berlin Heidelberg), 249–268.
Schuiling, R. D., and de Boer, P. L. (2011). Rolling stones; fast weathering of olivine in shallow seas for cost-effective CO2 capture and mitigation of global warming and ocean acidification. Earth Syst. Dyn. Discuss. 2, 551–568. doi: 10.5194/esdd-2-551-2011
Schuiling, R. D., and Krijgsman, P. (2006). Enhanced weathering: an effective and cheap tool to sequester CO2. Clim. Change 74, 349–354. doi: 10.1007/s10584-005-3485-y
Sen, G. (ed). (2014). “Alkaline and ultra-alkaline rocks, carbonatites, and kimberlites,” in Petrology (Berlin; Heidelberg: Springer), 243–260. Available online at: https://link.springer.com/book/10.1007/978-3-642-38800-2
Setschenow, J. (1889). Über die konstitution der salzlösungen auf grund ihres verhaltens zu kohlensäure. Zeitschrift für Physikalische Chemie 4U, 117–125. doi: 10.1515/zpch-1889-0409
Shan, M., Hwang, B.-G., and Zhu, L. (2017). A global review of sustainable construction project financing: policies, practices, and research efforts. Sustain. Sci. Pract. Policy 9, 2347. doi: 10.3390/su9122347
Sharifian, R., Wagterveld, R. M., Digdaya, I. A., Xiang, C., and Vermaas, D. A. (2021). Electrochemical carbon dioxide capture to close the carbon cycle. Energy Environ. Sci. 14, 781–814. doi: 10.1039/D0EE03382K
Sharker, M. R., Kim, S. C., Hossen, S., Sumi, K. R., Choi, S. K., Choi, K. S., et al. (2021). Carbonic anhydrase in pacific abalone haliotis discus hannai: characterization, expression, and role in biomineralization. Front. Mol. Biosci. 8, 655115. doi: 10.3389/fmolb.2021.655115
Shih, S. M., Ho, C. S., Song, Y. S., and Lin, J. P. (1999). Kinetics of the reaction of Ca(OH)2 with CO2 at low temperature. Ind. Eng. Chem. Res. 38, 1316–1322. doi: 10.1021/ie980508z
Shiklomanov, I. A. (1993). “World fresh water resources,” in Water in Crisis, ed P. H. Gleick (Oxford: Oxford University Press), 13–24.
Show, S., Tamang, A., Chowdhury, T., Mandal, D., and Chattopadhyay, B. (2015). Bacterial (BKH1) assisted silica nanoparticles from silica rich substrates: a facile and green approach for biotechnological applications. Colloids Surf. B Biointerfaces 126, 245–250. doi: 10.1016/j.colsurfb.2014.12.039
Singh, L. P., Goel, A., Bhattachharyya, S. K., Ahalawat, S., Sharma, U., and Mishra, G. (2015). Effect of morphology and dispersibility of silica nanoparticles on the mechanical behaviour of cement mortar. Int. J. Concrete Struct. Mater. 9, 207–217. doi: 10.1007/s40069-015-0099-2
Sissmann, O., Brunet, F., Martinez, I., Guyot, F., Verlaguet, A., Pinquier, Y., et al. (2014). Enhanced olivine carbonation within a basalt as compared to single-phase experiments: reevaluating the potential of CO2 mineral sequestration. Environ. Sci. Technol. 48, 5512–5519. doi: 10.1021/es405508a
Smith, P., Adams, J., Beerling, D. J., Beringer, T., Calvin, K. V., Fuss, S., et al. (2019). Land-management options for greenhouse gas removal and their impacts on ecosystem services and the sustainable development goals. Annu. Rev. Environ. Resour. 44, 255–286. doi: 10.1146/annurev-environ-101718-033129
Snæbjörnsdóttir, S., Wiese, F., Fridriksson, T., Ármansson, H., Einarsson, G. M., and Gislason, S. R. (2014). CO2 storage potential of basaltic rocks in Iceland and the oceanic Ridges. Energy Procedia 63, 4585–4600. doi: 10.1016/j.egypro.2014.11.491
Snæbjörnsdóttir, S. Ó., Oelkers, E. H., Mesfin, K., Aradóttir, E. S., Dideriksen, K., Gunnarsson, I., et al. (2017). The chemistry and saturation states of subsurface fluids during the in situ mineralisation of CO2 and H2S at the CarbFix site in SW-Iceland. Int. J. Greenhouse Gas Control 58, 87–102. doi: 10.1016/j.ijggc.2017.01.007
Sohn, R. A. (2013). Reaction-driven cracking in the TAG deep-sea hydrothermal field: Implications for serpentinization and carbonation of peridotite. ui.adsabs.harvard.edu, MR22A−07. Available online at: https://ui.adsabs.harvard.edu/abs/2013AGUFMMR22A.07S
Spane, F. A., Bonneville, A., McGrail, B. P., and Thome, P. D. (2012). Hydrologic Characterization Results and Recommendations for the Wallula basalt Pilot Well. PNWD-4368, Battelle-Pacific Northwest Division, Richland, Washington. Available online at: http://www.bigskyco2.org/sites/default/files/documents/Spane_PNWD_HydrolicReport_2012.pdf
Spier, J. (2020). “the ‘strongest’ climate ruling yet”: The dutch supreme court's urgenda judgment. Neth. Int. Law Rev. 67, 319–391. doi: 10.1007/s40802-020-00172-5
Spínola, A. C., Pinheiro, C. T., Ferreira, A. G. M., and Gando-Ferreira, L. M. (2021). Mineral carbonation of a pulp and paper industry waste for CO2 sequestration. Process Saf. Environ. Prot. 148, 968–979. doi: 10.1016/j.psep.2021.02.019
Steiner, S., Lothenbach, B., Proske, T., Borgschulte, A., and Winnefeld, F. (2020). Effect of relative humidity on the carbonation rate of portlandite, calcium silicate hydrates and ettringite. Cem. Concr. Res. 135, 106116. doi: 10.1016/j.cemconres.2020.106116
Stolaroff, J. K., Lowry, G. V., and Keith, D. W. (2005). Using CaO-and MgO-rich industrial waste streams for carbon sequestration. Energy Convers. Manage. 46, 687–699. doi: 10.1016/j.enconman.2004.05.009
Strefler, J., Amann, T., Bauer, N., Kriegler, E., and Hartmann, J. (2018). Potential and costs of carbon dioxide removal by enhanced weathering of rocks. Environ. Res. Lett. 13, 034010. doi: 10.1088/1748-9326/aaa9c4
Subhas, A. V., Adkins, J. F., Rollins, N. E., Naviaux, J., Erez, J., and Berelson, W. M. (2017). Catalysis and chemical mechanisms of calcite dissolution in seawater. Proc. Natl. Acad. Sci. U.S.A. 114, 8175–8180. doi: 10.1073/pnas.1703604114
Suhrhoff, T. J. (2022). Phytoprevention of heavy metal contamination from terrestrial enhanced weathering: can plants save the day? Front. Clim. 3, 820204. doi: 10.3389/fclim.2021.820204
Sumper, M., and Kröger, N. (2004). Silica formation in diatoms: the function of long-chain polyamines and silaffins. J. Mater. Chem. 14, 2059–2065. doi: 10.1039/B401028K
Sun, J., Bertos, M. F., and Simons, S. J. R. (2008). Kinetic study of accelerated carbonation of municipal solid waste incinerator air pollution control residues for sequestration of flue gas CO 2. Energy Environ. Sci. 1, 370–377. doi: 10.1039/B804165M
Sun, R., Li, Y., Liu, C., Xie, X., and Lu, C. (2013). Utilization of lime mud from paper mill as CO2 sorbent in calcium looping process. Chem. Eng. J. 221, 124–132. doi: 10.1016/j.cej.2013.01.068
Sverdrup, H. U., Koca, D., and Schlyter, P. (2017). A simple system dynamics model for the global production rate of sand, gravel, crushed rock and stone, market prices and long-term supply embedded into the WORLD6 model. BioPhys. Econ. Resource Qual. 2, 8. doi: 10.1007/s41247-017-0023-2
Tan, S.-I., Han, Y.-L., Yu, Y.-J., Chiu, C.-Y., Chang, Y.-K., Ouyang, S., et al. (2018). Efficient carbon dioxide sequestration by using recombinant carbonic anhydrase. Process Biochem. 73, 38–46. doi: 10.1016/j.procbio.2018.08.017
Tanzer, S. E., and Ramírez, A. (2019). When are negative emissions negative emissions? Energy Environ. Sci. 12, 1210–1218. doi: 10.1039/C8EE03338B
Taylor, A. R., Brownlee, C., and Wheeler, G. (2017). Coccolithophore cell biology: chalking up progress. Ann. Rev. Mar. Sci. 9, 283–310. doi: 10.1146/annurev-marine-122414-034032
Taylor, L. L., Leake, J. R., Quirk, J., Hardy, K., Banwart, S. A., and Beerling, D. J. (2009). Biological weathering and the long-term carbon cycle: Integrating mycorrhizal evolution and function into the current paradigm. Geobiology 7, 171–191. doi: 10.1111/j.1472-4669.2009.00194.x
Teir, S., Eloneva, S., Fogelholm, C.-J., and Zevenhoven, R. (2007a). Dissolution of steelmaking slags in acetic acid for precipitated calcium carbonate production. Energy 32, 528–539. doi: 10.1016/j.energy.2006.06.023
Teir, S., Revitzer, H., Eloneva, S., Fogelholm, C.-J., and Zevenhoven, R. (2007b). Dissolution of natural serpentinite in mineral and organic acids. Int. J. Miner. Process. 83, 36–46. doi: 10.1016/j.minpro.2007.04.001
ten Berge, H. F. M., van der Meer, H. G., Steenhuizen, J. W., Goedhart, P. W., Knops, P., and Verhagen, J. (2012). Olivine weathering in soil, and its effects on growth and nutrient uptake in Ryegrass (Lolium perenne L.): a pot experiment. PLoS ONE 7, e42098. doi: 10.1371/journal.pone.0042098
Terlouw, T., Bauer, C., Rosa, L., and Mazzotti, M. (2021). Life cycle assessment of carbon dioxide removal technologies: a critical review. Energy Environ. Sci. 14, 1701–1721. doi: 10.1039/D0EE03757E
Thonemann, N., Zacharopoulos, L., Fromme, F., and Nühlen, J. (2022). Environmental impacts of carbon capture and utilization by mineral carbonation: a systematic literature review and meta life cycle assessment. J. Clean. Prod. 332, 130067. doi: 10.1016/j.jclepro.2021.130067
Thorley, R. M. S., Taylor, L. L., Banwart, S. A., Leake, J. R., and Beerling, D. J. (2015). The role of forest trees and their mycorrhizal fungi in carbonate rock weathering and its significance for global carbon cycling. Plant Cell Environ. 38, 1947–1961. doi: 10.1111/pce.12444
Torres, M. A., Dong, S., Nealson, K. H., and West, A. J. (2019). The kinetics of siderophore-mediated olivine dissolution. Geobiology 17, 401–416. doi: 10.1111/gbi.12332
Turner, P. A., Mach, K. J., Lobell, D. B., Benson, S. M., Baik, E., Sanchez, D. L., et al. (2018). The global overlap of bioenergy and carbon sequestration potential. Clim. Change 148, 1–10. doi: 10.1007/s10584-018-2189-z
Tutolo, B. M., Awolayo, A., and Brown, C. (2021). Alkalinity generation constraints on basalt carbonation for carbon dioxide removal at the gigaton-per-year scale. Environ. Sci. Technol. 55, 11906–11915. doi: 10.1021/acs.est.1c02733
Ullman, W. J., Kirchman, D. L., Welch, S. A., and Vandevivere, P. (1996). Laboratory evidence for microbially mediated silicate mineral dissolution in nature. Chem. Geol. 132, 11–17. doi: 10.1016/S0009-2541(96)00036-8
Van Groenigen, J. W., Van Groenigen, K. J., Koopmans, G. F., Stokkermans, L., Vos, H. M. J., and Lubbers, I. M. (2019). How fertile are earthworm casts? A meta-analysis. Geoderma 338, 525–535. doi: 10.1016/j.geoderma.2018.11.001
van Hees, P. A. W., Lundström, U. S., and Giesler, R. (2000). Low molecular weight organic acids and their Al-complexes in soil solution—composition, distribution and seasonal variation in three podzolized soils. Geoderma 94, 173–200. doi: 10.1016/S0016-7061(98)00140-2
Vassilev, S. V., Vassileva, C. G., and Petrova, N. L. (2021). Mineral carbonation of biomass ashes in relation to their CO2 capture and storage potential. ACS Omega 6, 14598–14611. doi: 10.1021/acsomega.1c01730
Veetil, S. P., and Hitch, M. (2020). Recent developments and challenges of aqueous mineral carbonation: a review. Int. J. Environ. Sci. Technol. 17, 4359–4380. doi: 10.1007/s13762-020-02776-z
Verbruggen, E., Struyf, E., and Vicca, S. (2021). Can arbuscular mycorrhizal fungi speed up carbon sequestration by enhanced weathering? Plants People Planet. 3, 445–453. doi: 10.1002/ppp3.10179
Vicca, S., Goll, D. S., Hagens, M., Hartmann, J., Janssens, I. A., Neubeck, A., et al. (2022). Is the climate change mitigation effect of enhanced silicate weathering governed by biological processes? Glob. Chang. Biol. 28, 711–726. doi: 10.1111/gcb.15993
von Strandmann, P. A. E. P., Burton, K. W., Snæbjörnsdóttir, S. O., Sigfússon, B., Aradóttir, E. S., Gunnarsson, I., et al. (2019). Rapid CO 2 mineralisation into calcite at the CarbFix storage site quantified using calcium isotopes. Nat. Commun. 10, 1–7. doi: 10.1038/s41467-019-10003-8
Wang, X., and Maroto-Valer, M. (2011a). Integration of CO2 capture and storage based on pH-swing mineral carbonation using recyclable ammonium salts. Energy Procedia 4, 4930–4936. doi: 10.1016/j.egypro.2011.02.462
Wang, X., and Maroto-Valer, M. M. (2011b). Dissolution of serpentine using recyclable ammonium salts for CO2 mineral carbonation. Fuel 90, 1229–1237. doi: 10.1016/j.fuel.2010.10.040
Wannier, T. M., Nyerges, A., Kuchwara, H. M., Czikkely, M., Balogh, D., Filsinger, G. T., et al. (2020). Improved bacterial recombineering by parallelized protein discovery. Proc. Natl. Acad. Sci. U.S.A. 117, 13689–13698. doi: 10.1073/pnas.2001588117
Watson, A. J., Schuster, U., Shutler, J. D., Holding, T., Ashton, I. G. C., Landschützer, P., et al. (2020). Revised estimates of ocean-atmosphere CO2 flux are consistent with ocean carbon inventory. Nat. Commun. 11, 1–6. doi: 10.1038/s41467-020-18203-3
Welch, S. A., Barker, W. W., and Banfield, J. F. (1999). Microbial extracellular polysaccharides and plagioclase dissolution. Geochim. Cosmochim. Acta 63, 1405–1419. doi: 10.1016/S0016-7037(99)00031-9
Wendt, C. H., Lackner, K. S., Butt, D. P., and Ziock, H. J. (1998). Thermodynamic Calculations for Acid Decomposition of Serpentine and Olivine in MgCl2 Melts, II. Reaction Equilibria in MgCl2 Melts. Los Alamos Nat. Lab., Report. LA-984529.
White, S. K., Spane, F. A., Schaef, H. T., Miller, Q. R. S., White, M. D., Horner, J. A., et al. (2020). Quantification of CO2 Mineralization at the Wallula Basalt pilot project. Environ. Sci. Technol. 54, 14609–14616. doi: 10.1021/acs.est.0c05142
Wilson, S. A., Barker, S. L. L., Dipple, G. M., and Atudorei, V. (2010). Isotopic disequilibrium during uptake of atmospheric CO2 into mine process waters: implications for CO2 sequestration. Environ. Sci. Technol. 44, 9522–9529. doi: 10.1021/es1021125
Wilson, S. A., Dipple, G. M., Power, I. M., Thom, J. M., Anderson, R. G., Raudsepp, M., et al. (2009). Carbon dioxide fixation within mine wastes of ultramafic-hosted ore deposits: examples from the clinton creek and cassiar chrysotile deposits, Canada. Econ. Geol. 104, 95–112. doi: 10.2113/gsecongeo.104.1.95
Wilson, S. A., Harrison, A. L., Dipple, G. M., Power, I. M., Barker, S. L. L., Ulrich Mayer, K., et al. (2014). Offsetting of CO2 emissions by air capture in mine tailings at the Mount Keith Nickel Mine, Western Australia: rates, controls and prospects for carbon neutral mining. Int. J. Greenhouse Gas Control 25, 121–140. doi: 10.1016/j.ijggc.2014.04.002
Wilson, S. A., Raudsepp, M., and Dipple, G. M. (2006). Verifying and quantifying carbon fixation in minerals from serpentine-rich mine tailings using the Rietveld method with X-ray powder diffraction data. Am. Mineral. 91, 1331–1341. doi: 10.2138/am.2006.2058
Wyatt, K. H., and Stevenson, R. J. (2010). Effects of acidification and alkalinization on a periphytic algal community in an Alaskan Wetland. Wetlands 30, 1193–1202. doi: 10.1007/s13157-010-0101-3
Xiong, W., Wells, R. K., Horner, J. A., Schaef, H. T., Skemer, P. A., and Giammar, D. E. (2018). CO2 mineral sequestration in naturally Porous Basalt. Environ. Sci. Technol. Lett. 5, 142–147. doi: 10.1021/acs.estlett.8b00047
Xu, X., Kentish, S. E., and Martin, G. J. O. (2021). Direct air capture of CO2 by microalgae with buoyant beads encapsulating carbonic anhydrase. ACS Sustainable Chem. Eng. 9, 9698–9706. doi: 10.1021/acssuschemeng.1c01618
Xu, X., Liu, W., Chu, G., Zhang, G., Luo, D., Yue, H., et al. (2019). Energy-efficient mineral carbonation of CaSO4 derived from wollastonite via a roasting-leaching route. Hydrometallurgy 184, 151–161. doi: 10.1016/j.hydromet.2019.01.004
Yadav, S., and Mehra, A. (2017). Experimental study of dissolution of minerals and CO2 sequestration in steel slag. Waste Manag. 64, 348–357. doi: 10.1016/j.wasman.2017.03.032
Yadav, S., and Mehra, A. (2021). A review on ex situ mineral carbonation. Environ. Sci. Pollut. Res. 28, 12202–12231. doi: 10.1007/s11356-020-12049-4
Yagi, S., and Kunii, D. (1955). Studies on combustion of carbon particles in flames and fluidized beds. Symp. Combust. 5, 231–244. doi: 10.1016/S0082-0784(55)80033-1
Yasunishi, A., and Yoshida, F. (1979). Solubility of carbon dioxide in aqueous electrolyte solutions. J. Chem. Eng. Data 24, 11–14. doi: 10.1021/je60080a007
Yi, H., Xu, G., Cheng, H., Wang, J., Wan, Y., and Chen, H. (2012). An overview of utilization of steel slag. Procedia Environ. Sci. 16, 791–801. doi: 10.1016/j.proenv.2012.10.108
Yin, Q., Zhou, G., Peng, C., Zhang, Y., Kües, U., Liu, J., et al. (2019). The first fungal laccase with an alkaline pH optimum obtained by directed evolution and its application in indigo dye decolorization. AMB Express 9, 151. doi: 10.1186/s13568-019-0878-2
Yu, L., Wu, K., Liu, L., Liu, N., Ming, X., and Oelkers, E. H. (2020). Dawsonite and ankerite formation in the LDX-1 structure, Yinggehai basin, South China sea: An analogy for carbon mineralization in subsurface sandstone aquifers. Appl. Geochem. 120, 104663. doi: 10.1016/j.apgeochem.2020.104663
Yu, T., and Chen, Y. (2019). Effects of elevated carbon dioxide on environmental microbes and its mechanisms: a review. Sci. Total Environ. 655, 865–879. doi: 10.1016/j.scitotenv.2018.11.301
Zarrouk, S. J., and Moon, H. (2014). Efficiency of geothermal power plants: a worldwide review. Geothermics 51, 142–153. doi: 10.1016/j.geothermics.2013.11.001
Zeebe, R. E., and Wolf-Gladrow, D. (2001). CO2 in Seawater: Equilibrium, Kinetics, Isotopes. Available online at: https://books.google.com/books?hl=en&lr=&id=g3j3Zn4kEscC&oi=fnd&pg=PA1&ots=lcQrM3smtH&sig=qaeWok2WH6_hUH4V9vBiB6KOnoM
Zeng, W., Guo, L., Xu, S., Chen, J., and Zhou, J. (2020). High-throughput screening technology in industrial biotechnology. Trends Biotechnol. 38, 888–906. doi: 10.1016/j.tibtech.2020.01.001
Zevenhoven, R., Fagerlund, J., and Songok, J. K. (2011). CO2 mineral sequestration: developments toward large-scale application. Greenh. Gases Sci. Technol. 1, 48–57. doi: 10.1002/ghg3.7
Zeymer, C., and Hilvert, D. (2018). Directed evolution of protein catalysts. Annu. Rev. Biochem. 87, 131–157. doi: 10.1146/annurev-biochem-062917-012034
Zhang, C., Li, X., Lyu, J., and Li, F. (2020). Comparison of carbonate precipitation induced by Curvibacter sp. HJ-1 and Arthrobacter sp. MF-2: further insight into the biomineralization process. J. Struct. Biol. 212, 107609. doi: 10.1016/j.jsb.2020.107609
Zhang, D., and Song, J. (2014). Mechanisms for geological carbon sequestration. Procedia IUTAM 10, 319–327. doi: 10.1016/j.piutam.2014.01.027
Zhang, H., He, P.-J., Shao, L.-M., and Lee, D.-J. (2008). Temporary stabilization of air pollution control residues using carbonation. Waste Manag. 28, 509–517. doi: 10.1016/j.wasman.2007.02.005
Zhou, Y., Kumar, M., Sarsaiya, S., Sirohi, R., Awasthi, S. K., Sindhu, R., et al. (2022). Challenges and opportunities in bioremediation of micro-nano plastics: a review. Sci. Total Environ. 802, 149823. doi: 10.1016/j.scitotenv.2021.149823
Zhu, T., and Dittrich, M. (2016). Carbonate precipitation through microbial activities in natural environment, and their potential in biotechnology: a review. Front Bioeng Biotechnol 4, 4. doi: 10.3389/fbioe.2016.00004
Zhu, Y., Liu, Y., Ai, M., and Jia, X. (2022). Surface display of carbonic anhydrase on Escherichia coli for CO2 capture and mineralization. Synth. Syst. Biotechnol. 7, 460–473. doi: 10.1016/j.synbio.2021.11.008
Nomenclature
Carbonic anhydrase (CA): Enzyme that catalyzes equilibration between dissolved CO2 and carbonic acid.
CO2 mineralization: also referred to as mineral carbonation, a process by which CO2 becomes a solid mineral, namely carbonate. The term carbon mineralization is also widely used in the field and should not be confused with conversion of organic carbon into CO2.
Direct air capture (DAC): an engineered process of capturing carbon dioxide directly from ambient air and generating a concentrated stream of CO2 for sequestration or utilization.
Dissolved inorganic carbon (DIC): HCO3–(aq) + CO32–(aq) + CO2(aq)
Enhanced weathering: a method whereby crushed alkaline minerals, typically Mg- and Ca-rich silicates, are spread in the environment where they undergo physical, chemical and biological weathering, thus removing CO2 from the atmosphere and storing it as carbonate minerals and ocean bicarbonate. Two main types of enhanced weathering exist—coastal enhanced weathering and enhanced weathering in soils. Over longer time frames, these are also methods of ocean alkalinity enhancement.
Extracellular polymeric substances (EPS): A broad term for biological polymers secreted by microbes. Depending on specific properties, EPS can influence ion concentrations in microenvironments, nucleate carbonate precipitation, and catalyze mineral dissolution.
Ex situ mineralization: High surface area alkaline minerals are reacted with CO2-rich gases in engineered reactors, enhanced by elevated temperature and pressure, or using reagents. Mineral reactants are usually transported to a site of CO2 production. Carbonate mineral products may be utilized.
Geochemical NETs: Any negative emissions technology which involves substantial amounts of alkali or alkaline minerals in its flowsheet.
Indirect ocean capture (IOC): Technologies which remove dissolved inorganic carbon from the ocean, and thus CO2 from the atmosphere, via air-ocean gas exchange due to the pH sensitivity of the ocean's carbonate buffer system.
In situ mineralization: CO2-bearing fluids or wet supercritical CO2 are injected into suitable rock formations beneath the Earth's surface where the CO2 is mineralized.
Negative emissions technologies (NETs): Any technology which removes CO2 from the air, directly or indirectly, for the purpose of climate change mitigation. Also known as carbon dioxide removal (CDR) technologies.
Ocean alkalinity enhancement (OAE): Technologies that increase the alkalinity of seawater to enhance the ocean's natural carbon sink.
Ocean liming: Spreading soluble alkaline minerals in the ocean to increase ocean alkalinity.
Surficial mineralization: Air, or low purity CO2-bearing gases or fluids, are reacted with high surface area natural alkaline rocks, mine tailings or other alkaline industrial wastes, in large piles, heaps, or in controlled spaces such as greenhouses, forming carbonate minerals. Surficial processes, like enhanced weathering, occur more slowly than ex situ processes but unlike enhanced weathering, minerals are not transported or spread in the environment.
Keywords: carbon dioxide removal (CDR), mineral carbonation, enhanced weathering in soils, coastal enhanced weathering, biomineralization, ocean liming, climate change
Citation: Campbell JS, Foteinis S, Furey V, Hawrot O, Pike D, Aeschlimann S, Maesano CN, Reginato PL, Goodwin DR, Looger LL, Boyden ES and Renforth P (2022) Geochemical Negative Emissions Technologies: Part I. Review. Front. Clim. 4:879133. doi: 10.3389/fclim.2022.879133
Received: 18 February 2022; Accepted: 12 May 2022;
Published: 22 June 2022.
Edited by:
Ben W. Kolosz, University of Pennsylvania, United StatesReviewed by:
Rafael Mattos Dos Santos, University of Guelph, CanadaRon Zevenhoven, Åbo Akademi University, Finland
Copyright © 2022 Campbell, Foteinis, Furey, Hawrot, Pike, Aeschlimann, Maesano, Reginato, Goodwin, Looger, Boyden and Renforth. This is an open-access article distributed under the terms of the Creative Commons Attribution License (CC BY). The use, distribution or reproduction in other forums is permitted, provided the original author(s) and the copyright owner(s) are credited and that the original publication in this journal is cited, in accordance with accepted academic practice. No use, distribution or reproduction is permitted which does not comply with these terms.
*Correspondence: James S. Campbell, amFtZXMuY2FtcGJlbGxAaHcuYWMudWs=