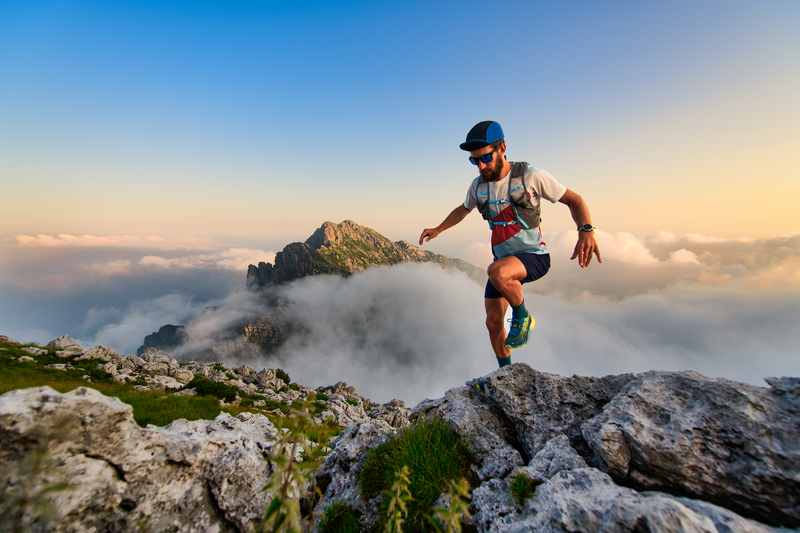
95% of researchers rate our articles as excellent or good
Learn more about the work of our research integrity team to safeguard the quality of each article we publish.
Find out more
ORIGINAL RESEARCH article
Front. Clim. , 23 March 2022
Sec. Climate, Ecology and People
Volume 4 - 2022 | https://doi.org/10.3389/fclim.2022.844831
This article is part of the Research Topic Advances in Marine Heatwave Interactions View all 18 articles
Marine heat waves (MHWs), prolonged discrete anomalously warm water events, have been increasing significantly in duration, intensity and frequency all over the world, and have been associated with a variety of impacts including alteration of ecosystem structure and function. This study assessed the effects of current and future MHWs on the Mediterranean seagrass Posidonia oceanica performance, also testing the importance of the thermal environment where the plant lives. The effects of current MHWs were studied through a mensurative experiment in a cold and in a warm site (West and North-West Sardinia, Italy, respectively). Future MHWs effects were tested through a manipulative experiment using P. oceanica shoots collected from the cold and warm sites and transplanted in a common garden in front of a power plant (North-West Sardinia): here plants were exposed to heat longer in duration and stronger in intensity than the natural MHWs of the last 20 years, resembling the future scenario. Morphological (total # of leaves, maximum leaf length, and percentage of total necrotic leaf length per shoot) and biochemical variables (leaf proteins, carbohydrates, and lipids) were considered. Plants had similar sublethal responses in both the experiments for most of the variables, revealing that current and future MHWs had similar effect types, but different in magnitude depending on the intensity of the waves: in general, the number of leaves, the maximum leaf length and lipid content decreased, while the leaf necrosis and carbohydrates increased. However, also the origin of the plants affected the results, corroborating the hypothesis that the thermal context the plants live affects their tolerance to the heat. Overall, this study provided evidence about the importance of biochemical variations, such as carbohydrate and lipid levels, as potentially good indicators of seagrass heat stress.
As a consequence of the global warming, extreme climatic events (ECEs) have increased in frequency (Coumou and Rahmstorf, 2012). Marine heat waves (MHWs), prolonged discrete anomalously warm water events, occur when sea surface temperature (SST) exceeds for at least 5 days a climatological threshold (Hobday et al., 2016). Analyses of different sources of SST data have revealed a significant increase in MHWs duration, intensity and frequency (Oliver et al., 2018; Darmaraki et al., 2019a). MHWs have had different implications for marine ecosystems, as they have been associated with a variety of impacts, including alteration of ecosystem structure and function (e.g., Wernberg et al., 2016), shifts in species ranges (e.g., Wernberg et al., 2011), mass mortalities (e.g., Garrabou et al., 2009; Fordyce et al., 2019), local extinctions and economic impacts on seafood industries through declines in important fishery species and impacts on aquaculture (Madin et al., 2012; Hughes et al., 2017; Hyndes et al., 2017).
Seagrass meadows are among the planet's coastal ecosystems most effective for providing key ecological services including nursery grounds, nutrient cycling, sediment stabilization, trophic transfer to adjacent habitats and toward higher trophic levels (Hemminga and Duarte, 2000; Larkum et al., 2006) and coastal protection from erosion (Fonseca and Cahalan, 1992; Fonseca and Koehl, 2006). Moreover, seagrasses are highly efficient in sequestering carbon (C): together with saltmarshes and mangroves, they are responsible for capturing up to 70% of the organic C in the marine realm (Nelleman et al., 2009), making them one of the most effective Blue C sinks on the planet (Serrano et al., 2020). Stressors, such as sediments and nutrients inputs from terrestrial runoff, physical disturbance (e.g., trawling, anchoring), invasive species, diseases, aquaculture, overgrazing, algal blooms and global warming, have been shown to cause seagrass declines at scales ranging from square meters to hundreds of square kilometers (e.g., Munkes, 2005; Orth et al., 2006; Williams, 2007; Holmer et al., 2008; Waycott et al., 2009; Bockelmann et al., 2013; Giakoumi et al., 2015).
At the global scale, seagrasses are also influenced by climate change (CC) and understanding their response to the occurrence of ECEs, such as MHWs, represents a timely objective to determine the fate of the related ecosystems functions and services they provide. Indeed, relevant shoot mortalities have been correlated to MHWs occurrence (Marbà and Duarte, 2010; Shields et al., 2019; Smale et al., 2019; Strydom et al., 2020) and loss of the biodiversity linked to the seagrass mortality has been also documented (Nowicki et al., 2019). Responses to heat events of several seagrass species have been investigated at several biological levels (see Nguyen et al., 2021 for a review) and manipulative experiments have provided evidence that MHWs affect growth rate (i.e., Saha et al., 2020), promote leaf necrosis (i.e., Ontoria et al., 2019), reduce the photosynthetic capacity (Marín-Guirao et al., 2016), and alter fatty acid production by decreasing the proportion of polyunsaturated fatty acids (PUFA) and increasing the percentage of saturated fatty acids (SFA) (i.e., Beca-Carretero et al., 2018, 2020).
Posidonia oceanica (L.) Delile is a slow-growing seagrass, endemic to the Mediterranean, that is experiencing a widespread decline throughout the basin (Telesca et al., 2015). The regression of P. oceanica beds and the consequent expansion of alternative habitats (e.g., algal turfs or dead seagrass rhizomes) is particularly common in highly urbanized coastal areas, mostly due to eutrophication and increased sedimentation rates (Montefalcone, 2009; Tamburello et al., 2012). However, how meadows are responding to CC pressures is currently under investigation. Populations living in locally deteriorated conditions, such as high nutrient input, can be more fragile to the effects of MHWs (Pazzaglia et al., 2020). In general, phenological response of seagrasses to environmental changes is intrinsically related to genotype/population plasticity (i.e., the amplitude of the individual reaction norm), an evolutionary component which is affected by many external and internal interacting factors, involving local adaptation/acclimation and genetic/epigenetic diversity (Pazzaglia et al., 2021a). The temperature change projected in the Mediterranean Sea ranges between 0.81 and 3.71°C in the upper layer (0–150 m) by the end of the 21st century, depending on the greenhouse gas emissions scenario (Soto-Navarro et al., 2020). The rising SST has accelerated MHWs occurrence and effects on Mediterranean benthic communities have already been described (Garrabou et al., 2009; Marbà and Duarte, 2010; Rubio-Portillo et al., 2016), even though their effects on subtidal water conditions are anything but obvious: SST is undoubtedly a useful proxy for very shallow water temperature, but using loggers remains recommended to have more accurate and precise temperature estimates as deep water temperature can be scarcely predicted from SST (Ceccherelli et al., 2020). The most severe impacts of MHWs on benthic communities are expected in summer when the intensity of these extreme events can easily exceed the maximum tolerance limit of the species (e.g., those in 2003, 2012, 2015, Darmaraki et al., 2019b). However, contrasting results have been obtained on P. oceanica populations response to MHWs in relation to their distribution. A few mesocosm experiments have fostered the importance of local adaptations, highlighting the different levels of thermotolerance, defined as the ability to survive a normally lethal heat stress (Norris and Hightower, 2000), of shallow vs. deep P. oceanica plants (Marín-Guirao et al., 2016, 2017). The same has been shown for plants living in different thermal environments along latitudinal gradients: shallow and low-latitude plants better tolerate high temperature exposure (4°C above the summer average), in respect to shallow and high-latitude plants (physiological and molecular traits, Marín-Guirao et al., 2016, 2019). Data on fatty acid composition have corroborated these results providing evidence that populations living at warmest temperatures were more thermo-tolerant and exhibited a greater capacity to cope with heat events by adjusting their lipid composition (PUFA/SFA) faster (Beca-Carretero et al., 2018). A recent reciprocal translocation experiment does not support the higher vulnerability of cold-adapted populations to sea-water temperature increase (Bennett et al., 2022), highlighting the importance of further studies in this direction. In fact, despite these results, the resistance of P. oceanica to summer MHWs is still far to be fully understood, and more insights on their biochemical responses to MHWs are needed to address the potential consequences of an increasing occurrence of ECEs on seagrass resilience to climate change.
To provide further insights, we investigated the effects of (i) natural present-day summer MHWs and (ii) simulated longer-lasting and more intense summer MHWs on the morphology and biochemistry of P. oceanica plants with a different thermal history. To this end, we have conducted two parallel field experiments: (i) a mensurative experiment, where the effects of current MHWs were studied on plants at two sites with different thermal regimes, and (ii) a manipulative common garden experiment in the field, where the effects of simulated future MHWs were tested on transplanted plants from the two sites. In the mensurative study, plant responses were evaluated after one and then two MHWs in a row, to evaluate if effects could be cumulative. In the manipulative experiment the effect of acute MHWs, as those expected to occur in the coming decades, was assessed on transplanted plants to explore how the species will respond to future MHWs and whether the responses differ depending on the thermal origin of plants.
The aim of our experiments is to define early warning morphological and biochemical responses of P. oceanica to short-term intense heat events associated with MHWs. The results contribute to assess the morphological and biochemical changes involved in the P. oceanica performance to resist to summer MHWs so to predict the vulnerability and the adaptability of this species to heat waves that will affect the seagrass meadows over the coming decades.
This study was conducted during summer 2020 in North-Western Sardinia (Italy, Western Mediterranean, Figure 1). In this area two sites were selected: Porto Conte (in the Alghero Gulf) and Le Saline (in the Asinara Gulf), West and North-West Sardinia, respectively. Although they are about 30 km apart, they are characterized by putatively different thermal regimes because the west coast (Porto Conte) receives relatively colder Atlantic waters directly through the Western Mid-Mediterranean Current and it is also influenced by upwelling currents (Olita et al., 2013). During the whole study period (July 8th—August 28th 2020), two temperature loggers (HOBO Pendant Temp/Light MX2202) were fixed within the representative seagrass meadow of each site and Le Saline (hereafter warm site) summer mean temperature was 3.48°C warmer than that in Porto Conte (hereafter the cold site).
Figure 1. Study area: North-West Sardinia (Italy). The red spot represents the warm site (Le Saline); the blue spot the cold site (Porto Conte) and the green spot the common garden (Fiume Santo).
To characterize the thermal regime of each site, summer (June 21st - Sept 23rd) SST daily values for the 2000–2019 period were obtained by the Group for High Resolution Sea Surface Temperature (GHRSST), using the 1 km resolution SST (G1SST) dataset produced by the NASA JPL (https://coastwatch.pfeg.noaa.gov/erddap/griddap/jplMURSST41.html), and used as a proxy of the 3 m subtidal temperature. The occurrence of MHWs in the last 20 years at both sites, together with their duration and intensity were calculated based on the 90th percentile of the climatology according to Hobday et al. (2018) metrics, using the rerrdapp (Scott Chamberlain, 2021) and heatwaveR packages (Schlegel and Smit, 2018) in R. Based on these data, MHW intensities and duration to simulate in the common garden were set to resemble possible future scenarios according to Hobday et al. (2016).
At each site five P. oceanica plagiotropic shoots, each bearing an apical shoot and at least five vertical distinguishable shoots, were collected from a meadow at 3 m of depth on July 8th, August 7th and 28th 2020 (hereafter T0, T2, and T3). Only at the end of the summer, the occurred MHWs were identified following the above procedure (using satellite SST) and, a posteriori from plant sampling, temperature anomalies were related to the plant performance.
For this experiment a common garden was created at Fiume Santo site (Asinara Gulf, Figure 1), in a 10,000 m2 area in front of a thermoelectric plant where two coal-fired units operate, with a nominal power of 320 MW, each. To cool up the whole thermoelectric plant system, sea water is continuously taken 1 km faraway offshore and released back close to the shoreline (12–24 m3/sec) about 6–8°C warmer (power plant water, PPW). This creates a marked seawater temperature gradient in the vicinity of the discharge point with a temporal pattern that follows seasonal changes and weather conditions.
Three areas within the thermal gradient generated by the PPW were identified to expose P. oceanica plants to three temperature intensity MHWs: the “Control Temperature” area (CT, unaffected by the PPW), the “Medium Temperature” area (MT, with PPW largely mixed with the natural water) and the “High Temperature” area (HT, with PPW minimally mixed with the natural water). The common garden consisted of three devices (each a 0.8 × 0.8 m metal grid) edged by floating material and fixed at the sea bottom using four concrete blocks to which the corners of each grid were attached by means of 4–5 m long wire cables. As the PPW stratifies on the top of the water column, this system allowed the device deployment at 1 m of depth where the PPW was intercepted at the HT and MT areas (Supplementary Figure 1). P. oceanica shoots were attached to grids by cable ties and they were shaded using neutral shading nets put over the structure to reduce the excess of light (ca. 20%) and reproduce the natural light intensity at the depth of the donor meadows (see below and Supplementary Figure 1). Nets were periodically cleaned to maintain constant shading throughout the experiment which was necessary to reduce potential impacts due to higher irradiance levels (Serrano et al., 2011; Dattolo et al., 2014). Also, fixing the plant cuttings to a suspended grid wouldn't interfere with their performance because P. oceanica shoots take up most of the nutrients from the water rather than through the roots (Ott, 1980).
To assess the potential significance of plant origin to heat tolerance, 15 P. oceanica plagiotropic cuttings, each bearing an apical shoot and at least five distinguishable vertical shoots (same as the mensurative experiment), from both the cold and the warm site were collected by SCUBA divers at 3 m deep on July 8th and immediately transported to the common garden. P. oceanica cuttings were fixed to the three grids, so that each grid had overall 10 cuttings (5 from the cold and 5 from the warm donor site) which were installed in the CT area during 14 days for plant acclimation. Then, the three devices were fixed at the HT, MT and CT area after a gradual 3-day acclimation obtained by manually translocating the grids through the site areas. Therefore, from July 24th (T1) to August 3rd plants were exposed to three different temperature intensities, depending on the area. To make the plants recover from the heat event, in the following 4 days (until August 7th, T2) the HT and the MT grids were gradually moved back to the CT area, where all remained for three more weeks until August 28th (T3). Temperature loggers (HOBO Pendant Temp/Light MX2202) were fixed at each grid for the whole experiment. The threshold (90th percentile) and the MHW categories were defined based on the SST climatology of the common garden site; to define the intensities of the simulated MHWs, data from the temperature loggers were used.
For both experiments on July 8th, August 7th and 28th (corresponding to T0, T2 and T3) five P. oceanica orthotropic shoots per treatment combination (each taken from a plagiotropic shoot) were sampled and the material collected was processed immediately for the morphological analysis and stored frozen (−20°C) for the subsequent biochemical analyses.
In the laboratory, leaves were removed from each shoot and, for both experiments the same variables were considered at all sampling times: the total number of leaves per shoot, the maximum leaf length per shoot (cm), and the total necrotic leaf length per shoot (% over the total leaf length) for the morphological analyses (on the fresh material), and the protein, carbohydrate and lipid contents of leaves for the biochemical analyses. All the morphological variables that could potentially change with varying environmental conditions were considered. Although changes in specific biochemical constituents (i.e., fatty acids) have been described as reliable indicators of seagrass response to temperature changes (Beca-Carretero et al., 2018, 2020), the choice of considering protein, carbohydrate, and lipid total contents as proxies of P. oceanica adaptation to thermal stress (heat waves) was based on recent results obtained in Mediterranean seagrasses (Leiva-Dueñas et al., 2021) and supported by a good cost/benefit ratio.
Before biochemical analyses, leaves of P. oceanica were washed in distilled water to remove sand and salts, and gently scraped with a clean scalpel to remove epiphytes and epibionts. Total protein and lipid contents were determined on aliquots (ca. 50–100 mg wet weight) of unbroken and healthy P. oceanica leaves, whereas carbohydrates were determined on water extracts of leaves. The analysis of carbohydrates was conducted on water extracts rather than intact fragments of leaves to avoid the detection of structural (dominant) carbohydrates composing the leaves.
More specifically, protein content was determined on 50 mg of intact leaves, cut in small pieces with scissors and grounded to a fine powder using a mortar and a pestle, according to Hatree (1972), as modified by Lowry et al. (1951) and Rice (1982) to compensate for phenol interference, and expressed as bovine serum albumin (BSA) equivalents (Pusceddu et al., 2009).
Water soluble carbohydrates were analyzed after extraction from 100 mg of leaves cut in small pieces with scissors added with 5 mL of reagent-grade water. The mixture was grounded and homogenized using a mortar and a pestle until a green surnatant was obtained. The mixture was then centrifuged (Eppendorf 5804, Eppendorf AG, Germany) at 800 g for 15 min and 1 mL of the supernatant was used for the analysis. Carbohydrate concentrations, expressed in glucose equivalents, were determined according to Gerchakov and Hatcher (1972) based on the phenol and concentrated sulfuric acid reaction with saccharides (Pusceddu et al., 2009).
Total lipid contents were determined on 100 mg of leaves cut in small pieces with scissors. Lipid extraction was performed according to Folch et al. (1957) by adding a chloroform-methanol mixture 2:1 v/v. Samples were added with 625 μL of chloroform and 1.25 mL of methanol and vortexed 1 min every 15 min for 1 h at room temperature. Successively, 1.9 mL of chloroform and 450 μL of a 0.2 M KCl solution were added to the mixture. After vortex (1 min) at room temperature samples were centrifuged for 10 min at 4,000 rpm. The supernatant was eliminated and the remaining fraction, after evaporation in a dry hot bath at 80 to 100°C for 20 min, was quantified according to the sulfuric acid carbonization procedure (Marsh and Weinstein, 1966). Total lipids were expressed in tripalmitin equivalents (Pusceddu et al., 2009).
A priori, a non-metric multidimensional scaling (nMDS), based on the Euclidean distance, was used to visualize the overall changes in P. oceanica plants due to the current and future MHWs. Then, to identify the variables with the highest contribution to the dissimilarities, a SIMPER test (90% of cut off) was run.
In the mensurative experiment permutational analyses of variance (PERMANOVAs, Anderson, 2001) were run using a similarity matrix based on the Euclidean distance of untransformed data, where the factor Time (T2 and T3, fixed) was orthogonal to the Site (cold and warm, fixed). In the manipulative experiment, PERMANOVAs were run separately on each time (T2 and T3) considering Origin (cold and warm donor site) and Temperature (HT, MT, CT) fixed and orthogonal factors. For both experiments the response variables were calculated as the change in percentage respect to T0 in morphological (total # of leaves/shoot, maximum leaf length/shoot, and the total necrotic leaf length/shoot) and biochemical (proteins, carbohydrates, and lipids) traits. Pair wise tests were used a posteriori in both the experiments to identify the alternative hypotheses of significant treatments. All statistical analyses were carried out through the software PRIMER 6+, using the included routine package PERMANOVA (Anderson, 2001).
The historical analysis revealed that in the study area twenty and twenty-two summer MHWs occurred since 2000 in the cold and the warm site, respectively (Figure 2), with an evident increase occurrence pattern over time at both sites (Table 1). Because of the different climatology (Figure 3), at the warm site summer MHWs maximum temperature was in mean 0.20°C higher than at the cold site (Table 1), although their intensity over each climatology threshold (Hobday et al., 2016, 2018) never exceeded 3°C at both sites (Figure 2). Since 2000 the duration of the summer MHWs varied from 5 to 59 days at the cold site and from 5 to 36 days at the warm site (Figure 2 and Table 1).
Figure 2. Intensity and duration of the summer MHWs at the warm (left) and cold (right) site: in blue the 2000–2019 MHWs, in black the 2020 (current) MHWs and in red the common garden waves (future MHWs).
Table 1. Frequency, duration and intensity (Hobday et al., 2016) of the past summer MHWs at the two sites in the 2000–2019 period.
Figure 3. Current MHWs. Summer 2020 MHWs at the warm and cold site. Red bars represent sampling times (T0, T2, and T3). Climatology was calculated based on the last 30 years of SST; Threshold represents the 90th percentile of the climatology; 2x, 3x, 4x thresholds to define MHW categories (Hobday et al., 2016).
During the mensurative experiment two MHWs naturally occurred at both sites, each of them with an intensity and duration well within the variability of the waves identified at these same sites over the last 20 years. Particularly, their complete duration was 5 and 9 days (13 days between the two events) and 8 and 41 days (9 days in between), at the cold site and the warm site, respectively (Figure 3). In terms of peak temperature, waves reached 27.34°C and then 27.13°C at the warm site, and 27.19°C and 27.04°C at the cold site (Figure 3). For both sites T0 was before the first summer MHW, T2 was just after the first one and T3 after the second MHW (Figure 3).
Overall, large dissimilarities were found between plants of the two sites, with the differences increasing through time especially in the cold site (nMDS, Figure 4). The traits which contributed the most to the plant changes were in general the total necrotic leaf length and all the biochemical ones (SIMPER test, Table 2).
Figure 4. Current MHWs. nMDS of the interaction between Time and Site: red, warm site; blue, cold site; triangles, T2; squares, T3.
P. oceanica morphological changes were found after summer 2020 MHWs in both sites (i.e., T3; Figure 5 and Table 3). Particularly, opposite effects across time were found at the warm site in the maximum leaf length and the length of necrotic tissue, as they decreased and increased, respectively, although any cumulative effects due to the MHWs over time were evidenced. At the cold site the reverse was found. Furthermore, no significant change was observed in the total number of leaves per shoot in both sites (Figure 5 and Table 3).
Figure 5. Current MHWs. Mean change (%) in the morphological (total of leaves, max leaf length, and total necrotic leaf length) and biochemical variables (proteins, carbohydrates, lipids) at T2 and T3. Bar color corresponds to the site: red, warm site and blue, cold site.
Biochemical variations were also detected in plants at both sites (Figure 5 and Table 3). Carbohydrates changed differently depending on Time and Site interaction: in the warm site there was no difference between T2 and T3, while in the cold one a significant increase was found in T3. Conversely, Site and Time, but not their interaction, significantly affected both protein and lipid contents. After the first MHW (T2) the amount of proteins decreased while the lipids increased in both sites, and after the second wave (T3), proteins increased especially in the cold site plants, and lipids decreased especially at the warm site.
At the common garden, the simulated MHWs were all stronger and longer than the natural occurring at the warm and cold site (Figures 2, 6). Particularly, the MHW duration was overall 52 days (from T0 to T3) for all three temperature treatments (CT, MT and HT), but temperature intensity changed considerably until T2 among treatments: in fact, at the HT area the wave reached 31.37°C, at the MT 29.68°C, while temperature at the CT was not higher than 29.24°C. T0 corresponds to before the different heat intensities, T2 to 15 days of highest temperature treatment, and T3 to other 21 days (from T2) of homogeneous temperature conditions (CT). Temperature in all treatments was always over the temperature threshold with the difference in intensity lasting from T1 to T2 (Figure 6).
Figure 6. Future MHWs. Temporal variation of temperature at the common garden at the CT, MT, and HT area. T0, T2, and T3 refer to the sampling times. T1 refers to the time the shoots were moved to the different temperature areas. Climatology was calculated based on the last 30 years of SST; Threshold represents the 90th percentile of the climatology; 2x, 3x, 4x thresholds to define MHW categories (Hobday et al., 2016).
Changes in the analyzed traits varied through time differently, depending on the temperature treatment and origin of the plants. In general, a larger similarity was found among the replicates from the cold site compared to those from the warm site, both through times (Figure 7A) and temperature treatments especially depending on the origin (Figure 7B). The most important contribution to the dissimilarities was overall due to the total length of necrotic tissue and all the biochemical variables (SIMPER test, Table 4).
Figure 7. Future MHWs. nMDS of the interaction between Time and Origin (A) and Temperature and Origin (B). In the upper ordination (A): red, warm origin; blue, cold one; triangles, T2 and squares, T3. In the lower ordination (B): triangles, cold origin; squares, warm origin; yellow, CT; orange, MT; and red, HT.
The morphology of P. oceanica changed differently between temperature treatments over time, also depending on the origin of the plant (Figure 8 and Table 5). Particularly, variations in the total number of leaves per shoot were only evident in T3 when, regardless the temperature intensity, it decreased importantly in plants from the cold site, while plants from the warm site lost more leaves at the HT and MT than at the CT. At T3 the number of leaves was similar in the plants of the two origins in both HT and MT, while at the CT it increased only in plants from the warm site. The maximum leaf length at T2 decreased depending on the specific combination between origin and temperature treatments: plants from the warm donor site had a higher decrease in the maximum leaf length in MT and HT treatments, than in CT, while treatments had similar effects on plants from the cold donor site. In T3 there was any recovery for this variable in none of the treatment combinations. The necrotic leaf length was highly dependent on the origin of the plant: the highest percentage of necrotic leaf length was recorded in plants from the warm site which showed signs of necrosis regardless the temperature treatments (CT, MT, and HT) already since T2. By contrast, necrotic portions of the leaves were very low and consistent through time in plants from the cold site.
Figure 8. Future MHWs. Mean change (%) in the morphological (total of leaves, max leaf length, and total necrotic leaf length) and biochemical variables (proteins, carbohydrates, lipids) at T2 and T3. Bar color corresponds to the temperature area: yellow = CT, orange = MT and red = HT. Border color corresponds to the plant origin: red = warm site and blue = cold site.
Table 5. Future MHWs: PERMANOVA results on the change at T2 and T3 of the morphological (total # of leaves/shoot, maximum leaf length/shoot and total necrotic leaf length/shoot) and biochemical (proteins, carbohydrates, and lipids) variables due to the effect of the Temperature (HT, MT, and CT) and Origin (cold vs. warm site).
Biochemical variations were also detected in the manipulative experiment (Figure 8 and Table 5). A significant effect of the interaction between temperature and origin was found on the protein content in both times, although the differences were not directly proportional to the temperature treatment. No significant differences in carbohydrates were found in T2 plants, while in T3 differences among temperature treatments were evident and directly proportional to the increase in heat. A significant role was also played by the origin of the plant, the cold plants producing more carbohydrates than the warm plants. At the end, plants from the cold site under HT treatment produced more carbohydrates than any other treatment plants. Finally, different results were found for the lipid content between sampling times: in T2 lipids increased according to the increasing temperature and in plants from the warm origin, more than those from the cold origin. However, in T3 a general decrease in the lipid content was detected especially in the CT and MT plants, while it remained high only in the cold origin plants of the HT treatment.
Heat effects due to current and future summer MHWs were evidenced by both morphological and biochemical P. oceanica variables. Although in both experiments P. oceanica morphology was affected by MHWs, plants were able to overcome both the natural heat event and the simulated MHWs by the end of the study, as all the shoots were still alive. Indeed, all the plants showed only sublethal responses which followed a similar pattern in both experiments for most of the variables, revealing that current and future MHWs had similar effects, but different in magnitude (Supplementary Figure 2): in fact, the plants of the manipulative experiment that experienced higher intensity and longer lasting MHWs, resembling future scenarios (Darmaraki et al., 2019b), showed in general more intense responses, strongly depending on the origin of the plants. This similar pattern, even though different in magnitude, supports the hypothesis that dealing with cuttings for the manipulative experiment, rather than natural shoots (used for the mensurative one), did not introduce other variability: in fact, clonal integration among shoots, known for buffering against environmental changes (Ruocco et al., 2021), should be in P. oceanica about 15 cm (Marbà et al., 2002) and cuttings used in our study were always longer. Therefore, the consistent patterns between the current and future MHWs effects inform on how the seagrass will react to heat events in the future by decreasing the number of leaves, the maximum leaf length and lipid content and by increasing the leaf necrosis and the carbohydrate content. However, they also indicate that the local context will notably influence the performance of the plant.
The effects of current and future summer MHWs were clearly detectable, though results confirm the key role played by the local thermal condition (in terms of climatology) on plant performance. Here the effects of the current MHWs (mensurative experiment) are reported to depend on the site, and the effects of the intensity of MHWs (manipulative experiment) on the plant origin site. More specifically, during the current MHWs the factor site affected maximum leaf length, percentage of the total necrotic leaf length and all the biochemical variables, strongly supporting the hypothesis that P. oceanica has site-specific thermal acclimation responses. The importance of the site was evident also during future MHWs, when changes in the morphological attributes of plants from the warm origin showed, in general, a higher thermo resistance than plants from the cold one. The total number of leaves per shoot decreased homogeneously in the short-term (T2), while at the end of the experiment (T3) only plants of warm origin that experienced the MHW in the CT site (unaffected by the simulated MHW) recovered by producing new leaves. These results suggest that overcoming the effects of MHWs depended on the temperature intensity, but also on the origin of the shoots. Furthermore, maximum leaf length decreased sharply in both the short (T2) and the long-term (T3), but the duration of the heat event had a protrusive effect since it canceled the initial (T2) differences due to the origin and wave intensity. Similar sublethal responses of P. oceanica due to a prolonged induced heat stress were already found in mesocosm experiments (Marín-Guirao et al., 2018), suggesting that the declines reported in natural populations after anomalous warming events could be the consequences of prolonged heat-induced physiological alterations detectable in several morphological traits (Heckathorn et al., 2013).
In both experiments, plant origin also influenced the leaf necrosis, that in shoots from the warm site was much higher than in those from the cold site. This response was proportional in magnitude to the heat stress, as future MHWs triggered a higher leaf necrosis (but only in plants from the warm origin) than the current MHWs (Supplementary Figure 2). Necrosis is known to be a common higher plant response to abiotic stress (Van Doorn et al., 2011; Beca-Carretero et al., 2020), used as an indicator of irreversible heat-induced damage to the whole leaf tissue (since Kappen, 1981), and thus the results are not surprising. However, the fact that this phenomenon only regarded the warm site plants could not be generalized and keeps open the question whether these were the most stressed plants or, rather, those that most likely would have survived to the heat period. Further research is necessary to question to what extent the necrosis should be seen as a sign of leaf senescence and consequent death or activation of a process that could improve the tolerance of the plant by reallocating the resources. The diversion of resources from leaves to rhizomes have been suggested as a likely strategy in the species to conserve resource stocks and withstand biotic (e.g., hervibory; Ruocco et al., 2018) and abiotic (e.g., heat; Marín-Guirao et al., 2018) stress conditions. Unfortunately, as no data after T3 are available, whether the plants would have been destined to a site-dependent mortality remains an unanswered question also because seagrass shoot death is not necessarily anticipated by morphological changes (Ceccherelli et al., 2018) and to date heat resistance thresholds have not been clearly correlated to leaf necrosis. Though it is not possible to robustly infer about which between the two P. oceanica populations is the most vulnerable to the MHWs, the results suggest that they have different optimum temperature thresholds. This hypothesis is corroborated by the different reaction of the two seagrass populations to the same stimuli (common garden experiment), with the potentiality that the same condition can represent either a stimulation or an inhibition until plant death, depending on the local adaptation of individuals (Alexieva et al., 2003). Indeed, different populations of the same species can also be locally adapted (Kawecki and Ebert, 2004) and, since there is still ambiguity about genetic vs. plastic changes and adaptive vs. non-adaptive changes, tailored experiments employing appropriate inferential methods are needed to draw general conclusions on these issues (Merilä and Hendry, 2014; Reusch, 2014). Moreover, further experiments aimed at assessing the development and the survival rate of P. oceanica should be carried out to relate the seagrass performance to the effective resilience to the heat event.
A cumulative effect of two short summer MHWs in a row was not really appreciated neither in terms of shoots morphology nor of leaf biochemistry. Shoot morphology, in fact, was not affected by time in the mensurative experiment, while the biochemical composition varied only inconsistently. This result could be due to either a very rapid recovery of the before-MHW condition or to a complex compensatory metabolism of the plant (Reusch et al., 2005; Traboni et al., 2018). On the other hand, it is not possible to reject the existence of a thermal priming status that hardened the plants during the first MHW making them more resistant to the thermal stress of the second MHW, as recently demonstrated in a few seagrass species (Nguyen et al., 2020), including P. oceanica seedlings (Pazzaglia et al., 2022).
The biochemical composition of the leaves can be good predictor of seagrass stress (Ceccherelli et al., 2018) and the analysis of metabolites in marine plants is used to understand how marine plants respond to dynamic environmental conditions (Kumar et al., 2016). The results of this study also indicate that the content of the largest classes of organic compounds, in particular carbohydrates and lipids, varied significantly depending on the origin of the plant and the temperature. Both in the mensurative and manipulative experiments, carbohydrate content had a late response (T3), with plants having an increase with heat especially for the cold site (current MHW experiment) and cold origin (future MHW experiment), showing again the importance of the origin of the plant. Seagrasses are known to have a high pool of carbohydrates used to buffer the daily and seasonal fluctuations in light availability, so that the variability of carbohydrate content is typically reflected most at a seasonal scale (Alcoverro et al., 2001). The results suggest that the carbohydrate pool could also buffer fluctuations in temperature at least at a short-time scale, so that carbohydrates would deserve to be investigated as early warnings of seagrass resistance ability to summer MHWs. In fact, the warming-induced sugar starvation described in heat-sensitive, but not heat-tolerant P. oceanica plants after a simulated MHWs, further support the importance of carbohydrate metabolism and signaling in the heat-stress response of seagrasses, and hence in their potential as early warning signals (Marín-Guirao et al., 2019). Conversely, recent studies showed how different categories of fatty acids vary when temperature changes: the amount of saturated fatty acid increases and the number of unsaturated fatty acids decreases, maintaining membrane fluidity in response to thermal stress (Beca-Carretero et al., 2018, 2021). Here, a prolonged heat event caused a decrease in the total amount of lipids (consistently in the current and future MHW experiments) that was proportional to the heat intensity (Supplementary Figure 2), thus suggesting that lipids also can be good predictors of heat stress, even as a whole category. Conversely, proteins are likely not a reliable warning of heat stress, because although the interactive effect between temperature and origin was found in both times of the future MHWs experiment (but not in the current ones) a unique direction of the change could not be identified. Similar observations on the protein content were recently found in other seagrasses (Beca-Carretero et al., 2021) and, likely for the intrinsic variability of protein contents, a much higher replication would be needed to define the scale of reliability of such variable. Overall, the change of the biochemical content in primary producers may have an important ecological role (Hernán et al., 2016) and needs to be further investigated also by identifying how specific groups of these biochemical contents may change in relation of heat events. In fact, the change of the components may not only anticipate the changes in the morphological variables after or during a stress, but it can also predict how the trophic interactions will change when nutritional values vary according to the heat, since they can affect the nutritional preferences of seagrass herbivores (Carmen et al., 2012; Hernán et al., 2016) and, consequently, the impact the trophic interactions between producers and consumers.
Overall, morphological changes here induced by MHWs were consistent with expectations formulated on other seagrass species, such as that increased temperature triggers higher necrotic leaf portions (Beca-Carretero et al., 2020) and reduces leaf formation rates (Olsen et al., 2012). The novelty of these findings stands on the high discrepancy between P. oceanica shoots performance of different thermal origin (including the unexpected higher necrosis in warm-origin plants) highlighting the intimate reaction of plants to current and future summer MHWs at least in the short-term after. P. oceanica was suggested to have low thermal optimum and lethal limits compared to other Mediterranean seagrasses (Savva et al., 2018), such as Cymodocea nodosa (Marín-Guirao et al., 2016). In general, seagrass meadow declines are likely linked to the intensity and duration of the MHWs (Marbà and Duarte, 2010; Arias-Ortiz et al., 2018; Smale et al., 2019; Strydom et al., 2020) and these results provided evidence that the resistance to the same event will depend much on the context the seagrass lives or comes from. Even if further studies on the resistance to MHWs are necessary to define the thermal tolerance of P. oceanica and distinguish between acclimation and adaptation process, results are enough to suggest considering the local thermal conditions in restoration efforts (Pazzaglia et al., 2021b), since they can influence the performance of the transplanted plants and thus affect the success of the whole actions.
The original contributions presented in the study are included in the article/Supplementary Material, further inquiries can be directed to the corresponding author.
PS, GC, GP, and LM-G conceived the ideas and designed methodology. PS, APa, and FP collected the data for the morphological analyses. APu and SS collected the data for the biochemical analysis. PS and FP analyzed the data. PS and GC led the writing of the manuscript. All authors have contributed critically to the drafts, gave final approval for publication, and agreed to be accountable for all aspects of the work.
The study has been funded by Italian Ministry of Education and Research PRIN 2017 (MHHWBN) Marine Habitats restoration in a climate change-impaired Mediterranean Sea (MAHRES) and by PON—National Operational Programme—Research and Innovation 2014–2020—PhDs and research contracts on innovation-related topics.
The authors declare that the research was conducted in the absence of any commercial or financial relationships that could be construed as a potential conflict of interest.
All claims expressed in this article are solely those of the authors and do not necessarily represent those of their affiliated organizations, or those of the publisher, the editors and the reviewers. Any product that may be evaluated in this article, or claim that may be made by its manufacturer, is not guaranteed or endorsed by the publisher.
We sincerely thank Luigi Piazzi for helping in transplanting plants on Time 0 and Paola Maglioli and Paolino Schiaffino (EP Produzione Fiume Santo) for supporting us in the logistic activities at the power plant.
The Supplementary Material for this article can be found online at: https://www.frontiersin.org/articles/10.3389/fclim.2022.844831/full#supplementary-material
Alcoverro, T., Manzanera, M., and Romero, J. (2001). Annual metabolic carbon balance of the seagrass Posidonia oceanica: the importance of carbohydrate reserves. Mar. Ecol. Prog. Ser. 211, 105–116. doi: 10.3354/meps211105
Alexieva, V., Ivanov, S., Sergiev, I., and Karanov, E. (2003). Interaction between stresses. Bulg. J. Plant Physiol 29, 1–17.
Anderson, M. J. (2001). A new method for non-parametric multivariate analysis of variance. Austr. Ecol. 26, 32–46. doi: 10.1111/j.1442-9993.2001.01070.pp.x
Arias-Ortiz, A., Serrano, O., Masqué, P., Lavery, P. S., Mueller, U., Kendrick, G. A., et al. (2018). A marine heatwave drives massive losses from the world's largest seagrass carbon stocks. Nat. Clim. Chang. 8, 338–344. doi: 10.1038/s41558-018-0096-y
Beca-Carretero, P., Azcárate-García, T., Julia-Miralles, M., Stanschewski, C. S., Guihéneuf, F., and Stengel, D. B. (2021). Seasonal acclimation modulates the impacts of simulated warming and light reduction on temperate seagrass productivity and biochemical composition. Front. Mar. Sci. 8:e731152. doi: 10.3389/fmars.2021.731152
Beca-Carretero, P., Guihéneuf, F., Krause-Jensen, D., and Stengel, D. B. (2020). Seagrass fatty acid profiles as a sensitive indicator of climate settings across seasons and latitudes. Mar. Environ. Res. 161:105075. doi: 10.1016/j.marenvres.2020.105075
Beca-Carretero, P., Guihéneuf, F., Marín-Guirao, L., Bernardeau-Esteller, J., García-Muñoz, R., Stengel, D. B., et al. (2018). Effects of an experimental heat wave on fatty acid composition in two Mediterranean seagrass species. Mar. Pollut. Bull. 134, 27–37. doi: 10.1016/j.marpolbul.2017.12.057
Bennett, S., Alcoverro, T., Kletou, D., Antoniou, C., Boada, J., Buñuel, X., et al. (2022). Resilience of seagrass populations to thermal stress does not reflect regional differences in ocean climate. New Phytol. 233, 1657–1666. doi: 10.1111/nph.17885
Bockelmann, A. C., Tams, V., Ploog, J., Schubert, P. R., and Reusch, T. B. (2013). Quantitative PCR reveals strong spatial and temporal variation of the wasting disease pathogen, Labyrinthula zosterae in northern European eelgrass (Zostera marina) beds. PLoS ONE 8, e62169. doi: 10.1371/journal.pone.0062169
Carmen, B., Brun, F. G., Onoda, Y., Cambridge, M. L., Bouma, T. J., Vergara, J. J., et al. (2012). Leaf-fracture properties correlated with nutritional traits in nine Australian seagrass species: implications for susceptibility to herbivory. Mar. Ecol. Prog. Ser. 458, 89–102. doi: 10.3354/meps09757
Ceccherelli, G., Oliva, S., Pinna, S., Piazzi, L., Procaccini, G., Marin-Guirao, L., et al. (2018). Seagrass collapse due to synergistic stressors is not anticipated by phenological changes. Oecologia 186, 1137–1152. doi: 10.1007/s00442-018-4075-9
Ceccherelli, G., Pinna, F., Pansini, A., Piazzi, L., and La Manna, G. (2020). The constraint of ignoring the subtidal water climatology in evaluating the changes of coralligenous reefs due to heating events. Sci. Rep. 10, 1–13. doi: 10.1038/s41598-020-74249-9
Coumou, D., and Rahmstorf, S. (2012). A decade of weather extremes. Nat. Clim. Chang. 2, 491–496. doi: 10.1038/nclimate1452
Darmaraki, S., Somot, S., Sevault, F., and Nabat, P. (2019a). Past variability of Mediterranean Sea marine heatwaves. Geophys. Res. Lett. 46, 9813–9823. doi: 10.1029/2019GL082933
Darmaraki, S., Somot, S., Sevault, F., Nabat, P., Narvaez, W. D. C., Cavicchia, L., et al. (2019b). Future evolution of marine heatwaves in the Mediterranean Sea. Climate Dyn. 53, 1371–1392. doi: 10.1007/s00382-019-04661-z
Dattolo, E., Ruocco, M., Brunet, C., Lorenti, M., Lauritano, C., D'esposito, D., et al. (2014). Response of the seagrass Posidonia oceanica to different light environments: Insights from a combined molecular and photo-physiological study. Mar. Environ. Res. 101, 225–236. doi: 10.1016/j.marenvres.2014.07.010
Folch, J., Lees, M., and Stanley, G. S. (1957). A simple method for the isolation and purification of total lipides from animal tissues. J. Biol. Chem. 226, 497–509. doi: 10.1016/S0021-9258(18)64849-5
Fonseca, M. S., and Cahalan, J. A. (1992). A preliminary evaluation of wave attenuation by four species of seagrass. Estuar. Coast. Shelf Sci. 35, 565–576. doi: 10.1016/S0272-7714(05)80039-3
Fonseca, M. S., and Koehl, M. A. R. (2006). Flow in seagrass canopies: the influence of patch width. Estuar. Coast. Shelf Sci. 67, 1–9. doi: 10.1016/j.ecss.2005.09.018
Fordyce, A. J., Ainsworth, T. D., Heron, S. F., and Leggat, W. (2019). Marine heatwave hotspots in coral reef environments: Physical drivers, ecophysiological outcomes, and impact upon structural complexity. Front. Mar. Sci. 6, 498. doi: 10.3389/fmars.2019.00498
Garrabou, J., Coma, R., Bensoussan, N., Bally, M., Chevaldonné, P., Cigliano, M., et al. (2009). Mass mortality in Northwestern Mediterranean rocky benthic communities: effects of the 2003 heat wave. Glob. Chang. Biol. 15, 1090–1103. doi: 10.1111/j.1365-2486.2008.01823.x
Gerchakov, S. M., and Hatcher, P. G. (1972). Improved technique for analysis of carbohydrates in sediments 1. Limnol. Oceanogr. 17, 938–943. doi: 10.4319/lo.1972.17.6.0938
Giakoumi, S., Halpern, B. S., Michel, L. N., Gobert, S., Sini, M., Boudouresque, C. F., et al. (2015). Towards a framework for assessment and management of cumulative human impacts on marine food webs. Conserv. Biol. 29, 1228–1234. doi: 10.1111/cobi.12468
Hatree, E. F. (1972). Determination of protein: a modification of the Lowry method that gives a linear photometric response. Anal. Biochem., 48, 422–427. doi: 10.1016/0003-2697(72)90094-2
Heckathorn, S. A., Giri, A., Mishra, S., and Bista, D. (2013). Heat Stress and Roots. Climate Change and Plant Abiotic Stress Tolerance. Wiley-VCH Verlag GmbH & Co. KGaA. doi: 10.1002/9783527675265.ch05
Hemminga, M. A., and Duarte, C. M. (2000). Seagrass Ecology. Cambridge: Cambridge University Press. doi: 10.1017/CBO9780511525551
Hernán, G., Ramajo, L., Basso, L., Delgado, A., Terrados, J., Duarte, C. M., et al. (2016). Seagrass (Posidonia oceanica) seedlings in a high-CO2 world: from physiology to herbivory. Sci. Rep. 6:38017. doi: 10.1038/srep38017
Hobday, A. J., Alexander, L. V., Perkins, S. E., Smale, D. A., Straub, S. C., Oliver, E. C., et al. (2016). A hierarchical approach to defining marine heatwaves. Prog. Oceanogr. 141, 227–238. doi: 10.1016/j.pocean.2015.12.014
Hobday, A. J., Oliver, E. C., Gupta, A. S., Benthuysen, J. A., Burrows, M. T., Donat, M. G., et al. (2018). Categorizing and naming marine heatwaves. Oceanography 31, 162–173. doi: 10.5670/oceanog.2018.205
Holmer, M., Argyrou, M., Dalsgaard, T., Danovaro, R., Diaz-Almela, E., Duarte, C. M., et al. (2008). Effects of fish farm waste on Posidonia oceanica meadows: synthesis and provision of monitoring and management tools. Mar. Pollut. Bull. 56, 1618–1629. doi: 10.1016/j.marpolbul.2008.05.020
Hughes, T. P., Kerry, J. T., Álvarez-Noriega, M., Álvarez-Romero, J. G., Anderson, K. D., Baird, A. H., et al. (2017). Global warming and recurrent mass bleaching of corals. Nature 543, 373–377. doi: 10.1038/nature21707
Hyndes, G. A., Heck, K. L., Vergés, A., Harvey, E. S., Kendrick, G. A., Lavery, P. S., et al. (2017). Accelerating tropicalization and the transformation of temperate seagrass meadows. Bioscience 66, 938–948. doi: 10.1093/biosci/biw111
Kappen, L. (1981). Ecological Significance of Resistance to High Temperature. In Physiological Plant Ecology I. Berlin: Springer. doi: 10.1007/978-3-642-68090-8_15
Kawecki, T. J., and Ebert, D. (2004). Conceptual issues in local adaptation. Ecol. Lett. 7, 1225–1241. doi: 10.1111/j.1461-0248.2004.00684.x
Kumar, M., Kuzhiumparambil, U., Pernice, M., Jiang, Z., and Ralph, P. J. (2016). Metabolomics: an emerging frontier of systems biology in marine macrophytes. Algal Res. 16, 76–92. doi: 10.1016/j.algal.2016.02.033
Larkum, A. W., Orth, R. J., and Duarte, C. M. (2006). Seagrasses: biology, ecology and conservation. Phycologia 45:5. doi: 10.2216/i0031-8884-45-5-602.1
Leiva-Dueñas, C., Cortizas, A. M., Piñeiro-Juncal, N., Díaz-Almela, E., Garcia-Orellana, J., and Mateo, M. A. (2021). Long-term dynamics of production in western Mediterranean seagrass meadows: Trade-offs and legacies of past disturbances. Sci. Total Environ. 754:142117. doi: 10.1016/j.scitotenv.2020.142117
Lowry, O. H., Rosebrough, N. J., Farr, A. L., and Randall, R. J. (1951). Protein measurement with the Folin phenol reagent. J. Biol. Chem. 193, 265–275. doi: 10.1016/S0021-9258(19)52451-6
Madin, E. M., Ban, N. C., Doubleday, Z. A., Holmes, T. H., Pecl, G. T., and Smith, F. (2012). Socio-economic and management implications of range-shifting species in marine systems. Glob. Environ. Change 22, 137–146. doi: 10.1016/j.gloenvcha.2011.10.008
Marbà, N., and Duarte, C. M. (2010). Mediterranean warming triggers seagrass (Posidonia oceanica) shoot mortality. Glob. Chang. Biol. 16, 2366–2375. doi: 10.1111/j.1365-2486.2009.02130.x
Marbà, N., Hemminga, M. A., Mateo, M. A., Duarte, C. M., Mass, Y. E., Terrados, J., et al. (2002). Carbon and nitrogen translocation between seagrass ramets. Mar. Ecol. Prog. Ser. 226, 287–300. doi: 10.3354/meps226287
Marín-Guirao, L., Bernardeau-Esteller, J., García-Muñoz, R., Ramos, A., Ontoria, Y., Romero, J., et al. (2018). Carbon economy of Mediterranean seagrasses in response to thermal stress. Mar. Pollut. Bull. 135, 617–629. doi: 10.1016/j.marpolbul.2018.07.050
Marín-Guirao, L., Entrambasaguas, L., Dattolo, E., Ruiz, J. M., and Procaccini, G. (2017). Molecular mechanisms behind the physiological resistance to intense transient warming in an iconic marine plant. Front. Plant Sci. 8, 1142. doi: 10.3389/fpls.2017.01142
Marín-Guirao, L., Entrambasaguas, L., Ruiz, J. M., and Procaccini, G. (2019). Heat-stress induced flowering can be a potential adaptive response to ocean warming for the iconic seagrass Posidonia oceanica. Mol. Ecol. 28, 2486–2501. doi: 10.1111/mec.15089
Marín-Guirao, L., Ruiz, J. M., Dattolo, E., Garcia-Munoz, R., and Procaccini, G. (2016). Physiological and molecular evidence of differential short-term heat tolerance in Mediterranean seagrasses. Sci. Rep. 6, 1–13. doi: 10.1038/srep28615
Marsh, J. B., and Weinstein, D. B. (1966). Simple charring method for determination of lipids. J. Lipid Res. 7, 574–576. doi: 10.1016/S0022-2275(20)39274-9
Merilä, J., and Hendry, A. P. (2014). Climate change, adaptation, and phenotypic plasticity: the problem and the evidence. Evol. Appl. 7, 1–14. doi: 10.1111/eva.12137
Montefalcone, M. (2009). Ecosystem health assessment using the Mediterranean seagrass Posidonia oceanica: a review. Ecol. Indic. 9, 595–604. doi: 10.1016/j.ecolind.2008.09.013
Munkes, B. (2005). Seagrass Systems: Stability of Seagrass Systems Against Anthropogenic Impacts (Doctoral Dissertation, Christian-Albrechts Universität Kiel).
Nelleman, C., Corcoran, E., Duarte, C. M., Valdes, L., DeYoung, C., Fonseca, L., et al. (2009). Blue Carbon. A Rapid Response Assessment. United Nations Environment Programme. GRID-Arendal, Norway.
Nguyen, H. M., Kim, M., Ralph, P. J., Marín-Guirao, L., Pernice, M., and Procaccini, G. (2020). Stress memory in seagrasses: first insight into the effects of thermal priming and the role of epigenetic modifications. Front. Plant Sci. 11, 494. doi: 10.3389/fpls.2020.00494
Nguyen, H. M., Ralph, P. J., Marín-Guirao, L., Pernice, M., and Procaccini, G. (2021). Seagrasses in an era of ocean warming: a review. Biol. Rev. 95:12736. doi: 10.1111/brv.12736
Norris, C. E., and Hightower, L. E. (2000). The heat shock response of tropical and desert fish genus. Environ. Stress. Gene Resp. 231:80018. doi: 10.1016/S1568-1254(00)80018-2
Nowicki, R., Heithaus, M., Thomson, J., Burkholder, D., Gastrich, K., and Wirsing, A. (2019). Indirect legacy effects of an extreme climatic event on a marine megafaunal community. Ecol. Monogr. 89:e01365. doi: 10.1002/ecm.1365
Olita, A., Ribotti, A., Fazioli, L., Perilli, A., and Sorgente, R. (2013). Surface circulation and upwelling in the Sardinia Sea: A numerical study. Cont. Shelf Res. 71, 95–108. doi: 10.1016/j.csr.2013.10.011
Oliver, E. C., Donat, M. G., Burrows, M. T., Moore, P. J., Smale, D. A., Alexander, L. V., et al. (2018). Longer and more frequent marine heatwaves over the past century. Nat. Commun. 9, 1–12. doi: 10.1038/s41467-018-03732-9
Olsen, Y. S., Sánchez-Camacho, M., Marbà, N., and Duarte, C. M. (2012). Mediterranean seagrass growth and demography responses to experimental warming. Estuaries Coasts 35, 1205–1213. doi: 10.1007/s12237-012-9521-z
Ontoria, Y., Cuesta-Gracia, A., Ruiz, J. M., Romero, J., and Pérez, M. (2019). The negative effects of short-term extreme thermal events on the seagrass Posidonia oceanica are exacerbated by ammonium additions. PLoS ONE 14, e0222798. doi: 10.1371/journal.pone.0222798
Orth, R. J., Carruthers, T. J., Dennison, W. C., Duarte, C. M., Fourqurean, J. W., Heck, K. L., et al. (2006). A global crisis for seagrass ecosystems. Bioscience 56, 987–996. doi: 10.1641/0006-3568(2006)56987:AGCFSE2.0.CO
Ott, J. A. (1980). Growth and production in Posidonia oceanica (L.) Delile. Marine Ecol. 1, 47–64. doi: 10.1111/j.1439-0485.1980.tb00221.x
Pazzaglia, J., Badalamenti, F., Bernardeau-Esteller, J., Ruiz, J. M., Giacalone, V. M., Procaccini, G., et al. (2022). Thermo-priming increases heat-stress tolerance in seedlings of the Mediterranean seagrass P. oceanica. Marine Pollut. Bull. 174:113164. doi: 10.1016/j.marpolbul.2021.113164
Pazzaglia, J., Nguyen, H. M., Santillán-Sarmiento, A., Ruocco, M., Dattolo, E., Marín-Guirao, L., et al. (2021b). The genetic component of seagrass restoration: what we know and the way forwards. Water 13:829. doi: 10.3390/w13060829
Pazzaglia, J., Reusch, T. B., Terlizzi, A., Marín-Guirao, L., and Procaccini, G. (2021a). Phenotypic plasticity under rapid global changes: The intrinsic force for future seagrasses survival. Evol. Appl. 14, 1181–1201. doi: 10.1111/eva.13212
Pazzaglia, J., Santillán-Sarmiento, A., Helber, S. B., Ruocco, M., Terlizzi, A., Marín-Guirao, L., et al. (2020). Does warming enhance the effects of eutrophication in the seagrass Posidonia oceanica? Front. Mar. Sci. 7, 1067. doi: 10.3389/fmars.2020.564805
Pusceddu, A., Dell'Anno, A., Fabiano, M., and Danovaro, R. (2009). Quantity and bioavailability of sediment organic matter as signatures of benthic trophic status. Mar. Ecol. Prog. Ser. 375, 41–52. doi: 10.3354/meps07735
Reusch, T. B. (2014). Climate change in the oceans: evolutionary versus phenotypically plastic responses of marine animals and plants. Evol. Appl. 7, 104–122. doi: 10.1111/eva.12109
Reusch, T. B., Ehlers, A., Hämmerli, A., and Worm, B. (2005). Ecosystem recovery after climatic extremes enhanced by genotypic diversity. Proc. Nat. Acad. Sci. U.S.A. 102, 2826–2831. doi: 10.1073/pnas.0500008102
Rice, D. L. (1982). The detritus nitrogen problem: new observations and perspectives from organic geochemistry. Mar. Ecol. Prog. Ser. 9:153. doi: 10.3354/meps009153
Rubio-Portillo, E., Izquierdo-Muñoz, A., Gago, J. F., Rosselló-Mora, R., Antón, J., and Ramos-Esplá, A. A. (2016). Effects of the 2015 heat wave on benthic invertebrates in the Tabarca Marine Protected Area (southeast Spain). Mar. Environ. Res. 122, 135–142. doi: 10.1016/j.marenvres.2016.10.004
Ruocco, M., Entrambasaguas, L., Dattolo, E., Milito, A., Marín-Guirao, L., and Procaccini, G. (2021). A king and vassals' tale: Molecular signatures of clonal integration in Posidonia oceanica under chronic light shortage. J. Ecol. 109, 294–312. doi: 10.1111/1365-2745.13479
Ruocco, M., Marín-Guirao, L., Ravaglioli, C., Bulleri, F., and Procaccini, G. (2018). Molecular level responses to chronic versus pulse nutrient loading in the seagrass Posidonia oceanica undergoing herbivore pressure. Oecologia 188, 23–39. doi: 10.1007/s00442-018-4172-9
Saha, M., Barboza, F. R., Somerfield, P. J., Al-Janabi, B., Beck, M., Brakel, J., et al. (2020). Response of foundation macrophytes to near-natural simulated marine heatwaves. Glob. Chang. Biol. 26, 417–430. doi: 10.1111/gcb.14801
Savva, I., Bennett, S., Roca, G., Jordà, G., and Marbà, N. (2018). Thermal tolerance of Mediterranean marine macrophytes: Vulnerability to global warming. Ecol. Evol. 8, 12032–12043. doi: 10.1002/ece3.4663
Schlegel, R. W., and Smit, A. J. (2018). heatwaveR: A central algorithm for the detection of heatwaves and cold-spells. J. Open Source Softw. 3:821. doi: 10.21105/joss.00821
Scott Chamberlain (2021). rerddap: General Purpose Client for 'ERDDAP' Servers. R package version 0.7.6.
Serrano, O., Lavery, P. S., Bongiovanni, J., and Duarte, C. M. (2020). Impact of seagrass establishment, industrialization and coastal infrastructure on seagrass biogeochemical sinks. Mar. Environ. Res. 160:104990. doi: 10.1016/j.marenvres.2020.104990
Serrano, O., Mateo, M. A., and Renom, P. (2011). Seasonal response of Posidonia oceanica to light disturbances. Mar. Ecol. Prog. Ser. 423, 29–38. doi: 10.3354/meps08955
Shields, E. C., Parrish, D., and Moore, K. (2019). Short-term temperature stress results in seagrass community shift in a temperate estuary. Estuaries Coasts 42, 755–764. doi: 10.1007/s12237-019-00517-1
Smale, D. A., Wernberg, T., Oliver, E. C., Thomsen, M., Harvey, B. P., Straub, S. C., et al. (2019). Marine heatwaves threaten global biodiversity and the provision of ecosystem services. Nat. Clim. Chang. 9, 306–312. doi: 10.1038/s41558-019-0412-1
Soto-Navarro, J., Jordá, G., Amores, A., Cabos, W., Somot, S., Sevault, F., et al. (2020). Evolution of Mediterranean Sea water properties under climate change scenarios in the Med-CORDEX ensemble. Clim. Dyn. 54, 2135–2165. doi: 10.1007/s00382-019-05105-4
Strydom, S., Murray, K., Wilson, S., Huntley, B., Rule, M., Heithaus, M., et al. (2020). Too hot to handle: unprecedented seagrass death driven by marine heatwave in a World Heritage Area. Glob. Chang. Biol. 26, 3525–3538. doi: 10.1111/gcb.15065
Tamburello, L., Benedetti-Cecchi, L., Ghedini, G., Alestra, T., and Bulleri, F. (2012). Variation in the structure of subtidal landscapes in the NW Mediterranean Sea. Mar. Ecol. Prog. Ser. 457, 29–41. doi: 10.3354/meps09703
Telesca, L., Belluscio, A., Criscoli, A., Ardizzone, G., Apostolaki, E. T., Fraschetti, S., et al. (2015). Seagrass meadows (Posidonia oceanica) distribution and trajectories of change. Sci. Rep. 5, 1–14. doi: 10.1038/srep12505
Traboni, C., Mammola, S. D., Ruocco, M., Ontoria, Y., Ruiz, J. M., Procaccini, G., et al. (2018). Investigating cellular stress response to heat stress in the seagrass Posidonia oceanica in a global change scenario. Mar. Environ. Res. 141, 12–23. doi: 10.1016/j.marenvres.2018.07.007
Van Doorn, W. G., Beers, E. P., Dangl, J. L., Franklin-Tong, V. E., Gallois, P., Hara-Nishimura, I., et al. (2011). Morphological classification of plant cell deaths. Cell Death Different. 18, 1241–1246. doi: 10.1038/cdd.2011.36
Waycott, M., Duarte, C. M., Carruthers, T. J., Orth, R. J., Dennison, W. C., Olyarnik, S., et al. (2009). Accelerating loss of seagrasses across the globe threatens coastal ecosystems. Proc. Nat. Acad. Sci. U.S.A. 106, 12377–12381. doi: 10.1073/pnas.0905620106
Wernberg, T., Bennett, S., Babcock, R. C., De Bettignies, T., Cure, K., Depczynski, M., et al. (2016). Climate-driven regime shift of a temperate marine ecosystem. Science 353, 169–172. doi: 10.1126/science.aad8745
Wernberg, T., Russell, B. D., Moore, P. J., Ling, S. D., Smale, D. A., Campbell, A., et al. (2011). Impacts of climate change in a global hotspot for temperate marine biodiversity and ocean warming. J. Exp. Mar. Biol. Ecol. 400, 7–16. doi: 10.1016/j.jembe.2011.02.021
Keywords: climate change, leaf biochemistry, leaf necrosis, marine heat waves, ocean warming, Posidonia oceanica, restoration, seagrasses
Citation: Stipcich P, Marín-Guirao L, Pansini A, Pinna F, Procaccini G, Pusceddu A, Soru S and Ceccherelli G (2022) Effects of Current and Future Summer Marine Heat Waves on Posidonia oceanica: Plant Origin Matters? Front. Clim. 4:844831. doi: 10.3389/fclim.2022.844831
Received: 07 January 2022; Accepted: 21 February 2022;
Published: 23 March 2022.
Edited by:
Thomas Wernberg, University of Western Australia, AustraliaReviewed by:
Jorge Terrados, Spanish National Research Council (CSIC), SpainCopyright © 2022 Stipcich, Marín-Guirao, Pansini, Pinna, Procaccini, Pusceddu, Soru and Ceccherelli. This is an open-access article distributed under the terms of the Creative Commons Attribution License (CC BY). The use, distribution or reproduction in other forums is permitted, provided the original author(s) and the copyright owner(s) are credited and that the original publication in this journal is cited, in accordance with accepted academic practice. No use, distribution or reproduction is permitted which does not comply with these terms.
*Correspondence: Patrizia Stipcich, cGF0cml6aWFzdGlwY2ljaEBsaWJlcm8uaXQ=
Disclaimer: All claims expressed in this article are solely those of the authors and do not necessarily represent those of their affiliated organizations, or those of the publisher, the editors and the reviewers. Any product that may be evaluated in this article or claim that may be made by its manufacturer is not guaranteed or endorsed by the publisher.
Research integrity at Frontiers
Learn more about the work of our research integrity team to safeguard the quality of each article we publish.