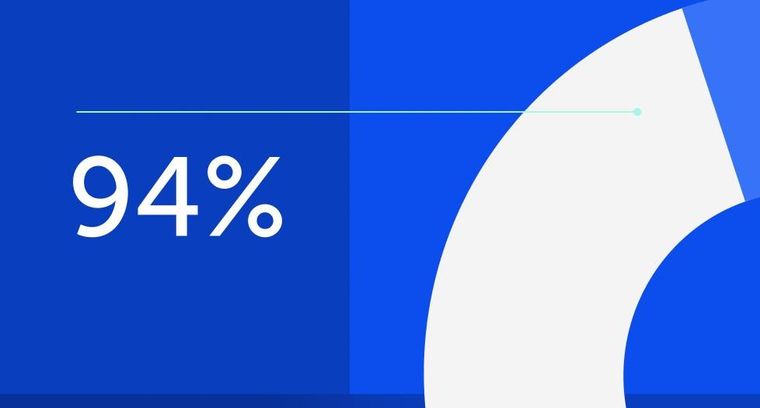
94% of researchers rate our articles as excellent or good
Learn more about the work of our research integrity team to safeguard the quality of each article we publish.
Find out more
ORIGINAL RESEARCH article
Front. Clim., 30 March 2022
Sec. Climate, Ecology and People
Volume 4 - 2022 | https://doi.org/10.3389/fclim.2022.783905
This article is part of the Research TopicUrban ecohydrology & hydrometeorology: Integrating water and greenery management to improve climate resilience in citiesView all 6 articles
Urban water management projects involving stormwater harvesting, detention, and infiltration are being increasingly combined with urban greening to support adaptation and resilience to the changing climate. A novel stormwater harvesting device, the TREENET Inlet, intercepts stormwater runoff from roads and soaks it into the soil through a leaky well to provide passive irrigation directly into street tree root zones. This study investigated the effects of stormwater harvesting through these inlet systems on the growth, water-use, leaf-level gas exchange and productivity of white cedar (Melia azedarach) street trees in a semi-arid climate in South Australia. The results indicated that mature trees with TREENET Inlets and leaky wells transpired 17% more water per unit of canopy area per day, on average for about a year, and 21% more during the dry season. White cedar saplings with stormwater harvesting grew 65% more in height and 60% more in diameter at breast height over a 3-year period than saplings without stormwater harvesting. This is consistent with observed 106% greater stomatal conductance and up to 169% greater photosynthesis rate in the dry season for saplings supported by harvested stormwater. This study shows that stormwater harvesting and infiltration by TREENET Inlets provides significant benefit to white cedar trees growing in a suburban street.
Increased land surface sealing due to urbanization and building of homes and infrastructure to support growing populations has decreased rainfall infiltration to the soil, decreased urban vegetation cover and increased demands on urban water resources (McDonald et al., 2013). Increased stormwater discharge resulting from urbanization presents risks to ecosystems (MacDonald et al., 2015) and downstream communities (Sheng and Wilson, 2009; Pickett et al., 2011). Urban drainage, heat island effects and the warming climate combine to increase temperatures in cities and towns to inhospitable extremes in many temperate, semi-arid and arid regions, resulting in increased heat-related mortality (Santamouris, 2020). Recent predictions have suggested that some Australian cities will experience more extreme climates, with summer maximum temperatures likely reaching 50°C by 2050 (Lewis et al., 2017).
Street trees and other urban vegetation generally have been promoted as making useful contributions to heat island mitigation strategies (Pitman et al., 2015) and to addressing many other pervasive environmental and community issues (Tarran, 2009; Richards et al., 2015). Urban trees can help to reduce air and ground temperatures (Klok et al., 2019; Wang et al., 2019a) through a combination of shading (Aminipouri et al., 2019; Xu et al., 2019) and evapotranspirative cooling (Rahman et al., 2015; Savi et al., 2015). Reduced urban vegetation cover has also been linked to increased physical and mental health issues in communities (Whitmee et al., 2015; Wang et al., 2019b).
While vegetation provides many benefits, a variety of stressors can limit tree health and productivity (Ugolini et al., 2012; Mullaney et al., 2015; Sepúlveda and Johnstone, 2019), and so reduce their services to communities. Sources of stress in urban vegetation include water availability, soil quality, and pollutants (Elloumi et al., 2015; Cannavo et al., 2018; Popek et al., 2018; Sepúlveda and Johnstone, 2019). In climates with hot, dry summers, limited access to water can be the major source of stress, as low rainfall combined with low permeability of built-up areas results in very low soil moisture. With the predicted changes to urban climates, street trees may begin to experience higher water stress and the benefits they provide may diminish (Savi et al., 2015). Urban vegetation requires water to thrive, so improving urban water management has become a priority for communities and their governing bodies.
Solutions have been proposed to the problems of urban heat islands, urban vegetation water stress and health, and increasing demands placed upon existing stormwater management networks. Water sensitive urban design (WSUD) [also called Integrated Urban Water Management Low-impact Developments and Sustainable Urban Drainage Systems (Fletcher et al., 2015)] are able to contribute to addressing these issues simultaneously (Argue, 2004; Palazzo, 2019). WSUD aims to maximize storm water retention while supporting local ecosystems and landscapes (Radcliffe, 2018; Lu et al., 2019; Szota et al., 2019; Thom et al., 2020). WSUD practices typically include the use of materials and devices like permeable paving, constructed wetlands, rain gardens, and enhanced passive stormwater infiltration systems (Szota et al., 2019; Hua et al., 2020) to detain stormwater and soak it into the soil.
Permeable paving allows infiltration of rainwater through roads and paths; it also removes pollutants from water during soil infiltration (Brattebo and Booth, 2003; Beecham et al., 2012; Johnson et al., 2019). Constructed wetlands have been used to reduce stormwater discharge and to provide supplementary water sources during times of drought (Hale et al., 2019). Rain gardens are small stormwater detention areas planted with suitable species, which intercept stormwater during rainfall events and soak it into the soil; the excess filtered water may be discharged slowly into the stormwater system (Lu et al., 2019). Infiltration systems like soakage trenches and leaky wells detain stormwater water below ground in leaky pipes, gravel and other porous media, where water can soak into surrounding soils (Government of South Australia, 2009). Most WSUD devices can be designed to deliver stormwater into the soil in root zones as a form of passive irrigation, although the effectiveness of the different devices in managing storm flows and moderating urban microclimates varies widely and is dependent on many factors (Scharenbroch et al., 2016; Brodnik and Brown, 2018; Szota et al., 2018; Lähde et al., 2019; Li et al., 2019; Zhang et al., 2019; Ebrahimian et al., 2021), with some circumstances resulting in decreased performance or unforeseen negative impacts (Scharenbroch et al., 2016; Brodnik and Brown, 2018; Szota et al., 2018, 2019; Lähde et al., 2019; Li et al., 2019; Zhang et al., 2019; Tu et al., 2020; Ebrahimian et al., 2021).
The TREENET Inlet is a relatively new stormwater harvesting and infiltration device designed to be dispersed throughout urban streets to divert stormwater runoff from the road into the verge subsoil in individual tree root zones (Sapdhare et al., 2019). These kerbside inlets were designed to deliver passive irrigation to street trees to decrease tree water stress during summer, to improve tree health, and to enhance urban cooling. Trees planted within the zone of influence of WSUD devices have been reported to have higher growth rates than trees without harvested water (Grey et al., 2018), however these studies do not relate specifically to kerbside inlets with subterranean leaky wells. This study investigated whether the TREENET Inlets increased water availability for trees, and improve tree leaf gas exchange and growth during the dry season. Three hypotheses were tested: (1) Inlets increase water availability which supports increased tree water use during the dry season; (2) Inlets increase water availability which supports increased photosynthesis during the dry season; and (3) Inlets increase the sapling growth rate.
No apparent damage as a result of the heating of the SFM1 was noticed during this experiment.
This study was conducted between 22nd February 2019 and 18th February 2020 in Hawthorn (Figure 1), a southern suburb of Adelaide, the capital city of South Australia. The local government authority responsible for the site, the City of Mitcham, installed outflow quantity and quality monitoring equipment in December 2015 in a 17.5 ha stormwater sub-catchment. The investment aimed to support empirical research into the effects of stormwater harvesting and infiltration on urban hydrology, vegetation, and climate (Shahzad, 2020). During 2016 and 2017, 183 TREENET Inlets (model R750, Space Down Under, Adelaide, South Australia, Australia) were installed and coupled to leaky wells, each with a nominal capacity of 130 L (Figure 2). Inlets and wells were dispersed at ~10 m spacing throughout the catchment's streets, with spacing in Jeffrey Street (one site in this study) being closer to 7 m. The inlets infiltrated stormwater harvested from the street directly into the soil beneath the road verges. As shown in Figure 2, the tops of the leaky wells are ~0.2 m under the soil surface with the bottoms of the wells being ~0.8 m deep. TREENET Inlets have been found to intercept between 1.8 and 4.5 kilolitres in a year (Shahzad, 2020).
Figure 1. Map of the study area with positions of trees used in this study. Egmont Terrace mature trees (CM_1-3) are indicated by red dots and young trees (CY_1-2) are indicated by orange dots. Jeffrey Street mature trees (EM_1-3) are indicated by dark blue dots and young trees (EY_1-3) are indicated by light blue dots. Young trees in Richmond Road (CY_3-8) are indicated by yellow dots. Positions of streetlights nearby study trees are indicated by open white circles.
White cedar (Melia azedarach) trees of ~60 years of age straddled the boundary of the gauged catchment and so provided ideal subjects for this study. Trees inside the gauged catchment, in Jeffrey Street, Hawthorn (34.974099°S, 138.603885°E), were equipped with TREENET Inlets and leaky wells in their root zones. Melia azedarach upper roots generally can be found from as shallow as ~0.05 m to ~0.75 m deep (Toky and Bisht, 1993; Tourn et al., 1999). Trees just outside the catchment, in Egmont Terrace, Hawthorn (34.973637°S, 138.604174°E), did not have inlets and leaky wells in their root zones. The Jeffrey Street and Egmont Terrace sites are separated by ~60 m (see Figure 1).
Three mature white cedar trees in each of Jeffrey Street and Egmont Terrace were chosen for sap flow monitoring. Tree spacing was similar at the two sites, with 5.8 m in the experimental section of Egmont Terrace, and 5.9 m in Jeffrey Street. White cedar saplings were planted near the mature trees in Jeffrey Street and Egmont Terrace sites by the local authority in winter 2015. Baseline measurement of tree height and diameter at breast height (DBH) of the Jeffrey Street saplings was undertaken by the City of Mitcham in 2016 in anticipation of future research. Baseline measurements did not extend to saplings at the Egmont Terrace site in 2016, so an alternative control was required for comparison with the Jeffrey Street saplings. Approximately 660 m to the north-west of Jeffrey Street, in Richmond Road in the suburb of Westbourne Park (34.970740°S, 138.597873°E), saplings were planted at the same time using stock from the same nursery and batch as the saplings in Jeffrey Street. Richmond Road was not fitted with inlets and leaky wells. The 2016 DBH data did not include measurements of all three saplings in Jeffrey Street and Richmond Road, so the mean DBH of the other two saplings at each site was assumed to be representative.
Impermeable concrete footpaths (1.5 m in width) existed next to the mature trees and the saplings in Jeffrey Street, Egmont Terrace and Richmond Road. Verge surfaces were permeable between the footpath and the kerb; they were either planted with turf grass or were bare soil with small ground-layer plants between the street trees. The width of the permeable verge surfaces varied; Egmont Terrace's was 2.7 m wide, and Jeffrey Street's and Richmond Road's were 1.3 m wide. In addition, trees in Jeffrey Street had been regularly pruned clear of electricity lines present in the street, while no electricity lines were present at the Egmont Terrace site.
For simplicity, all sample trees in Jeffrey Street are denoted as EM for mature trees, and EY for young saplings, where letter E represents enhanced passive infiltration. Those in Egmont Terrace and Richmond Road are denoted as CM and CY, respectively for mature and young trees, where letter C represents a control-sample condition.
This study investigated mature tree water use, sapling leaf gas exchange rates and sapling growth.
Sap flow meters (model SFM1, ICT International, Armidale, NSW Australia) were used to measure sap flow velocity in mature trees between 22 February 2019 and 18 February 2020. The SFM1 uses the heat ratio method described in Burgess et al. (2001). Each sap flow meter uses a set of three probes. One probe is a line heater which produces pulse heat at a prescribed time interval. The other two are sensor probes, each with two thermistors. The two sensor probes are installed either side of the heater probe along the sapflow stream. Heat pulse velocity in sapwood is measured by two pairs of thermistors across two sensor probes at different radial positions (Appendix A in Supplementary Material), based on which sap velocity and sap flow are estimated. Several assumptions, each introducing a source of uncertainty, are involved in this process. To reduce the overall uncertainty, we adopted a different upscaling method which is described in Section Sap Flow Estimation.
Electrical resistivity tomography (ERT) conducted using a PiCUS TreeTronic instrument prior to installing the sap flow meters ensured the meters' thermistors were located in active sapwood (Ganthaler et al., 2019). Sap flow meters were installed ~1.5 m above the ground on the trunks of the trees where active sapwood was identified. Electrical resistivity tomography was also used to determine the heartwood/sapwood boundary location so the cross-sectional area of the active sapwood within each mature tree could be calculated by a method modified from Wang et al. (2016). Appendix A in Supplementary Material.
Six SFM1 meters were installed on the 22nd of February 2019 and remained on trees until 18th of February 2020, one for each of the three mature trees in Jeffrey Street (EM_1, EM_2, EM_3), and in Egmont Terrace (CM_1, CM_2, and CM_3). With few exceptions the sap flow meter probes remained in the position throughout the whole measurement period. The meter was removed and reinstalled back to the same position on 18th November 2019 for CM_1, and the meter at EM_2 was removed on 11th February 2020 due to equipment failure. Data were logged half-hourly and downloaded regularly. No apparent damage as a result of the heating of the SFM1 was noticed during this experiment.
Common practice in estimating tree water use from heat-pulse sap flow meter measurements involves making several assumptions. (1) Wound and misalignment effects are accurately accounted for in estimating the heat pulse velocity. (2) Wood density and thermal property effects are accurately represented in converting the heat pulse velocity to the sap velocity at the points where the pairs of thermistors are located, this sap velocity is referred to as the lumen sap velocity (Edwards et al., 1997). (3) The average of lumen velocities at multiple points of sapwood (e.g., two points in our case) represents the average sap velocity on total sapwood area basis. In some cases, a radial profile of sap velocity is adopted for upscaling lumen sap velocity to sapwood-based sap velocity. (4) Sapwood area is accurately determined so that the sap flow for the whole tree can be calculated. We refer to this procedure as a bottom-up approach, to be distinguished from a top-down approach adopted in this study. Each of the four assumptions in the bottom-up approach can cause errors in the final sap flow estimation. This uncertainty would be considerable for this study in part because only one sap flow meter was available for each sample tree.
Thus, we took a top-down approach in which a calibrated relationship of lumen velocity and whole tree sap flow was applied for estimating sap flow. This was based on two assumptions, one of which is similar to (3) in the bottom-up approach. That is, the ratio of average sapwood area-based sap velocity (or whole tree sap flow or transpiration) to the lumen sap velocity at a fix point is constant. The other is that under wet conditions, trees transpire at the potential transpiration (PT) rate. Once the relationship of a tree is found for the condition under which the whole tree sap flow can be estimated from potential transpiration, it can then be applied to other measurement periods for correcting raw sap flow measurements to estimate sap flow of the tree. A similar approach was used in Liu et al. (2019).
Given that plant water stress can result from both the supply side (root zone moisture) and demand side (potential evapotranspiration) (Yang et al., 2013; Liu et al., 2017), two different functions were used in this study to describe the relationship between tree transpiration (estimated from potential transpiration for selected wet conditions) and uncorrected sap flow, one for lower and one for higher atmospheric demand conditions, as detailed in Appendix A in Supplementary Material.
Two different PT estimates were calculated using a Penman Monteith style approach: reference evapotranspiration (ETo) (Allen et al., 1998) and a hybrid dual-source energy partitioning method (Guan and Wilson, 2009). Both PT estimates were used to provide a range of PT in calculation of the scaling factors, with the reported result being the average of the two.
In the top-down method, the accuracy of lumen sap velocity is not as important as the conventional bottom-up method. Thus, we used the Sap Flow Tool program (provided by ICT International) to obtain the lumen sap velocity time series, with either measured or default values (Appendix A in Supplementary Material). This raw whole-tree sap flow time series was calculated based on the sapwood area estimated from the ERT images. It was then used to find the upscaling relationship, as detailed in Appendix A in Supplementary Material.
Due to the trees being different sizes, direct comparison of volumetric sap flow was inappropriate to investigate the TREENET Inlet's effect on tree water use because a larger tree was more likely to have a large volume of root zone soil. Normalizing the sap flow by canopy area allowed flow rate and volume to be compared, with sap flow volume reported in L/m2 of canopy area (Qc).
Photosynthesis was measured in white cedar saplings on 19 April 2019, which was a warm day with a maximum of 28.7°C, minimum of 16.1°C, and thin cloud cover. Measurements were made twice in each tree, once in the morning and once in the afternoon, using a LI-COR Photosynthesis System (Model LI-6400XT, LI-COR Biosciences, Lincoln, Nebraska, USA). Leaves used for measurements were fully formed healthy leaves selected from the top of the canopy and in direct sunlight (Leuzinger et al., 2010). For each round of measurements two leaves were selected from each tree. During the measurements the CO2 concentration within the chamber was set to 400 μmol·mol−1 and the flow rate to the sample cell was set to 500 μmols−1. Each leaf was exposed to a series of photosynthetically active radiation (PAR) concentrations of 1000, 500, 250, 100, 50, 10, and 0 μmol m−2·s−1. Photosynthesis was measured as it can be a reliable indicator of relative water stress in vegetation (Rahman et al., 2015).
Adaxial (upper side of a leaf) and abaxial (lower side of a leaf) stomatal conductance were measured on young white cedar trees using a leaf porometer (Decagon Model SC-1, Meter Environment, Pullman, Washington, USA). Stomatal conductance is essentially a measure of how open the stomata are and can be used as an indicator of plant water stress, particularly during summer dry seasons (Gimenez et al., 2005; Rahman et al., 2015). Measurements were made on 15th March and 1st, 6th, and 16th April 2019 over the course of each day. Stomatal conductance was measured multiple times between 9 AM and 6 PM for each tree, as conductance is known to fluctuate over the course of any given day (Murray et al., 2019). Three fully formed healthy leaves in direct sunlight were selected from the top of the canopy for each round of measurement. All stomatal conductance measurements were taken on warm days (maximum temperatures for each day were 27.2, 22.5, 22.3, and 33.0°C respectively) with little to no cloud cover.
Tree height and diameter at breast height were measured for young white cedar trees in Jeffrey Street, Egmont Terrace and Richmond Road on 26 September 2019. Tree height, trunk diameter at breast height, leaf area index, canopy area, and sapwood area of the mature street trees were measured or calculated between 22 February 2019 and 26 September 2019. Tree heights were measured using a laser device (Forestry Pro Laser Rangefinder, Nikon, Tokyo, Japan). Tree stem circumference was measured 1.3 m above ground level using a measuring tape, with DBH calculated from this assuming a circular trunk.
Leaf area index (LAI) of the mature tree canopies was measured using a ceptometer (AccuPAR Model LP-80, Meter Environment, Pullman, Washington, USA) by the method described in Rahman et al. (2015). The canopy of one mature subject tree in Egmont Terrace (CM _2) was shaded at the time of measurement by the canopies of neighboring trees so could not be measured; the LAI of CM_2 was assumed to be the mean of the other two specimens (CM_1 and CM_3). Canopy areas were measured by tracing their outlines using the polygon tool in Google Earth Pro which then provided the projected areas.
Rainfall and temperature data were obtained from the Australian Government's Bureau of Meteorology, based at the weather station at Adelaide, South Australia, which is ~5 km north of the study site (Kent Town, Station Number 023090). Wind speed, relative humidity, and solar radiation data were collected at Flinders University, approximately 6 km south of the study site. Sunrise and sunset times were estimated based on solar radiation values. Local climate data and tree canopy data were used to calculate reference evapotranspiration and tree level potential evapotranspiration, which were then used to calibrate sap flow data. For the purpose of this study, the Adelaide dry season is approximated to begin at the start of November and finish at the end of April, based on the long-term rainfall records.
One-way ANOVA was used to compare canopy normalized sap flow data time series (L/m2/day, or mm/day) between EM and CM trees. Two-way ANOVA tests were used to analyse the photosynthesis data with two sets of factors being morning and afternoon, and EY and CY trees. Analysis of covariance (ANCOVA) tests were conducted to analyse temporal variation patterns of the stomatal conductance data between EY and CY trees. These ANOVA and ANCOVA tests were conducted in MATLAB R2020. Tree heights and DBH data, with a low number of samples, were compared using the Mann-Whitney tests with no assumption of normal distribution. The Mann-Whitney tests were conducted using the R statistical software version 3.6.3.
Measurements of tree morphological features are summarized in Table 1. Most variables appear larger for trees in Egmont Terrace (without inlets) than for trees in Jeffrey Street (with inlets), although the Mann-Whitney tests showed the difference was not significant (p > 0.1), except for tree height (p = 0.1). This was likely due to the permeable verge in Egmont Terrace being twice the width of that in Jeffrey Street, providing more water for trees throughout their lifetime, and the trees in Jeffrey Street being regularly pruned to clear electricity lines. The smallest canopy projected area was 29.7 m2 for a tree in Jeffrey Street, the largest was in Egmont Terrace: 70.8 m2.
Average monthly sap flow reduced gradually from the start of monitoring in February 2019 and became negligible in July. No significant sap flow was measured following leaf fall in July through to September (mid-winter and early spring). Sap flow recommenced in spring (October). Water uptake by the trees varied considerably through the 12 months of the study, from 1 L in Jeffrey Street in August and 14,640 L in Egmont Terrace in January 2020 (Table 2). Based on the data shown in Figure 3, the trees on average extracted ~214 L from the soil per day over the duration of the experiment. When the trees were in leaf (i.e., excluding data from July and August), the average water uptake per tree was ~258 L per day.
Figure 3. Daily Qc for each mature tree at two streets [(A,C,E) with TREENET Inlets; (B,D,F) without TREENET Inlets] over entire project duration, adjusted by high (TVET) and low (ETo) potential transpiration rates under selected time windows (13/05/2019 to 19/05/2019, 21/10/2019 to 24/10/2019, 29/10/2019 to 01/11/2019, and 02/02/2020 to 10/02/2020) with least water stress. ANOVA results show a significant difference between the two sites (p = 0.00).
Although Jeffrey Street had TREENET Inlets and Egmont Terrace did not, the trees in Egmont Terrace extracted more water in total from the soil over the duration of the study (Table 2). This extra water uptake is consistent with the fact that the CM trees in Egmont Terrace were larger than the EM trees in Jeffrey Street (Table 1), in both canopy area and stem DBH. A larger tree size tends to be more likely associated with a larger volume of root zone which would have an access to more moisture supply. The following analyses were based on sap flow data normalized by canopy aera (Qc). Comparison of Qc between mature trees in Jeffrey Street (with inlets) and Egmont Terrace (without inlets) revealed 17% greater Qc for the whole measurement period, and 21% greater Qc during the dry seasons for trees with inlets (Figure 4). The difference in Qc between Jeffrey Street and Egmont Terrace was statistically significant (Figure 3). Further analysis of the dry season sap flow showed that the difference between sites was also statistically significant (p = 0.00) (Figure 4).
Figure 4. Average Qc for each site over dry season [(A): February 2019 to April 2019, (B): November 2019 to February 2020]. The presence of the inlets facilitated an increase of ~1.1 L per m2 of canopy per day. ANOVA results show a significant difference between the two sites (p = 0.00).
The time series sap flow data show that there was a large difference between the two dry seasons that were measured, with the 2019 dry season having much lower transpiration than the 2020 dry season. This difference in Qc is likely due to the difference in water availability between the two dry seasons. When compared against the average monthly rainfalls for Adelaide summers (1981–2020) (Bureau of Meteorology, 2020), 2019 had a lower than average precipitation for January to April (summer and autumn) (Figure 5). The 2019 January rainfall was recorded as being 0.0 mm which is significantly less than the long-term average of 20.1 mm. February and March 2019 rainfall was also less than average: 9.0 mm was received compared to the February average of 16.1 mm, and 8.2 mm fell in March compared with the average of 25.9 mm. Although rainfall during spring 2019 (September to November) and the first month of Summer were below average, the total summer rainfall (December 2019 to February 2020) was near average due to comparatively high rainfall in February. Overall, this difference in rainfall would have resulted in an overall higher availability of water for the 2020 dry season compared to the 2019 dry season, which in turn would result in increased tree water use. The sap flow rates showed that the trees with stormwater harvesting inlets had greater sap flow during the day when they were in leaf, particularly during the latter half of 2019 and early in 2020.
Figure 5. Long-term average local temperature (°C), VPD (hPa), RH (%) and rainfall data (mm) (A) and measured rainfall, VPD, RH and temperature data from January 2019 to February 2020 (B), showing comparatively low rainfall for start of 2019 against long term averages and rainfall for 2020 around the long-term average. Temperature data is plotted against the primary Y axis (left), and VPD, RH, and rainfall are plotted against the secondary Y axis (right).
The diurnal pattern of the sap flow indicated that sap flow occurred during the night (Figure 6). Approximately 26% of the total sap flow occurred during night time for CM trees, and 27% for EM trees. Such a large proportion of night-time sap flow suggests daytime water stress. Night time sapflow is not an uncommon phenomenon particularly in a dry condition (Antezana-Vera and Marenco, 2021). The difference between the two sites was not significant (p = 0.41).
Figure 6. Daily Qc for daytime (A) and night-time (B) for Jeffrey Street (blue) and Egmont Terrace (orange) from 22/02/2019 to 18/02/2020. ANOVA results show a significant difference between the two sites for daytime sap flow (p = 0.00) and for night-time sap flow (p = 0.00).
Leaf level gas exchange was greater in saplings with stormwater harvesting (Jeffrey Street) than in those without (Egmont Terrace). This result was consistent at each photosynthetically active radiation (PAR) intensity (Figure 7). In the morning, the rate of photosynthesis at the highest PAR (1000 μmol/m2s) was 44% higher in saplings with inlets; in the afternoon, the rate of photosynthesis was 169% higher. The ANOVA result indicated that TREENET Inlets improved leaf light-response of photosynthesis in the afternoon. However, the TREENET Inlets did not affect the variation of stomatal conductance (leaf temperature and leaf VPD) with different levels of light intensity (Tables 3A–D).
Figure 7. Morning (A) and Afternoon (B) photosynthetic rates for young M. azedarach trees at Jeffrey Street and Egmont Terrace in response to photosynthetically active radiation. Trees at Jeffrey Street showed higher photosynthetic rates than Egmont Terrace for both morning and afternoon of 19/04/2019.
Table 3. P values for 2-way ANOVA tests for photosynthesis rates (A), stomatal conductance (B), leaf temperature (C), and leaf VPD (D), ANCOVA tests of stomatal conductances measured from the porometer (E).
Stomatal conductance fluctuated over the course of the day due to responses to conditions such as solar radiation and temperature (Figure 8). Stomatal conductance was higher for saplings with inlets than for saplings without inlets (Figure 8; Table 3E); abaxial conductance was 106% higher and adaxial conductance was 35% higher. For the saplings (EY) in Jeffrey Street, the adaxial conductance decreased from morning to afternoon, while this trend was not obvious for the trees (CY) in Egmont Street.
Figure 8. Analysis of covariance (ANCOVA) tests for abaxial (A) and adaxial (B) stomatal conductance of young Melia azedarach at Jeffrey Street (with TREENET Inlets) and Egmont Terrace (without inlets).
The Mann-Whitney test result showed that sapling tree heights of Jeffrey Street measured in 2019 were significantly larger than saplings in Richmond Road (p = 0.03). One tree in Richmond Road was treated as outlier, confirmed by new measurements in 2022 (Table 4). The sapling DBH in Jeffrey Street was larger than in Richmond Road with a confidence level of 90% (p = 0.1).
Table 4. 2016, 2019 and 2022 height and DBH measurements for young Melia azedarach in Jeffrey Street and Richmond Road.
Comparing the rates of growth between the Jeffrey Street and Richmond Road suggested that there was a relationship between growth and presence of TREENET inlets. Between 2016 and 2019, DBH increased 60% more for saplings with inlets (Jeffrey Street) than saplings without inlets (Richmond Road) (Table 4). Saplings in streets with inlets grew taller; in Jeffrey Street the saplings grew 65% taller than those in Richmond Road. When considering the measurements in 2022, the DBH and height measurements provide further support for this, with Jeffrey Street's trees being 59% taller and having 77% greater DBH than Richmond Road's trees (Table 4).
The higher rate of Qc in mature trees, and the higher rates of photosynthesis, stomatal conductance, and the greater rate of growth in the saplings, indicated that stormwater harvesting and infiltration through kerbside inlets and leaky wells benefitted the white cedar street trees. These results supported all three hypotheses. The differences resulting from the harvested stormwater were proportionally greater for the saplings than for the mature trees. This might have been due to the smaller water requirements of the saplings being fully met with the additional harvested stormwater, and the young trees with inlets thriving without water stress. Nevertheless, during the dry season, the 21% greater Qc for mature trees suggested that the kerbside inlets and leaky wells in the root zones of mature white cedar trees were also of some benefit.
When considered against recent findings in which inlets harvested between 1.8 and 4.5 kL per inlet per year (Shahzad, 2020), water extraction by the mature trees (Table 2) exceeded the volume harvested by the inlets quite considerably. It is likely, therefore, that the mature trees with inlets would still have experienced water stress at times, although stress would likely have been less and for shorter periods than experienced by trees without inlets. A reduced water deficit rather than an absence of water stress might explain the smaller differences observed between mature trees than between saplings.
Richmond Road and Jeffrey Street have the same verge width. Between Jeffrey Street and Richmond Road, the results show that the DBH and height of EY trees in Jeffrey Street are larger than the CY trees in Richmond Road. The difference in heights between EY in Jeffrey Street and CY in Egmont Terrace appears to be smaller, which is likely related to the width of permeable verge surface in Egmont Terrace being double that of Jeffrey Street. The larger permeable surface area in Egmont Terrace may have resulted in greater infiltration through the surface during rainfall, increasing water availability to both saplings and mature trees. Nevertheless, the DBH of EY saplings in Jeffrey had grown significantly larger than the CY saplings in Egmont Terrace. The fact that the saplings in the narrower verges grew more in DBH suggests the TREENET Inlets and leaky wells more than offset the impact of reduced permeable surface area on sapling growth.
Storm water harvesting has been shown to support urban forests and in some situations to provide greater cooling to urban areas (Coutts et al., 2016). This experiment's data show that mature Melia azedarach street trees can easily transpire 200 L of water per day. Based on water's latent heat of vaporization this means it transfers heat energy of ~500 MJ per tree per day, which is equivalent to running a 12 KW evaporative air conditioner (common in dry summer periods) for 12 h per day. Stormwater harvesting through TREENET Inlets increased evapotranspiration by 1.1 L per square meter of canopy area per day, or 66 L per day for an average mature white cedar canopy area of 60 m2. The increased evapotranspiration due to the harvested stormwater reduced urban heat by an additional 165 MJ per tree per day.
During the 2000–2009 Millennium Drought there was widespread mortality of mature trees in Australia; this was attributed largely to the lack of water and resulting increased susceptibility to disease and pests (van Dijk et al., 2013). By supplying urban trees with more water through WSUD devices it is likely that urban tree mortality might be reduced during drought. This study has shown that TREENET Inlets and leaky wells can effectively increase water availability to support greater transpiration in mature urban street trees and substantially increase the growth rates of saplings in narrow streets where it may not be possible to install larger scale infiltration devices. This result may be significant for highly urbanized and developed cities where competing land use restricts permeable surface area in which trees may be grown.
The combination of the deciduous white cedar and Adelaide's climate, with its high winter rainfall and dry summer, may be well suited to stormwater harvesting for urban climate moderation. The reduced water demands of the deciduous species during winter may have maximized the time available for the harvested water to infiltrate the soil. If evergreen species had been used, their canopies might have intercepted a greater quantity of incident rainfall, and water extraction and transpiration during winter may have reduced the soil moisture level benefit during the dry period. Further studies with evergreen trees are needed to investigate this possibility.
This study showed that water availability for street trees was enhanced through passive infiltration using TREENET Inlets. It would be interesting to see how this improved tree water use may translate into street microclimate amelioration in summer, and how the climate amelioration potential varies with environmental conditions (e.g., heatwaves) and tree species.
TREENET Inlets coupled with leaky well infiltration systems benefitted white cedar (Melia azedarach) street trees growing in Hawthorn in metropolitan Adelaide. Mature trees with inlets and leaky wells showed 17% higher tree crown normalized sap flow than mature trees without inlets and wells and showed 21% higher Qc than mature trees without inlets during dry seasons. Increased sapling height (by 65%) and growth (by 60%) over a 3-year period, greater stomatal conductance (by 106%) and higher rates of photosynthesis (by 169%) showed that stormwater harvesting and infiltration into verge soil appeared to improve the growth and productivity of young street trees. This result supports further installation of inlets within the Adelaide metropolitan area to increase stormwater harvesting to support enhanced urban forest function for environmental purposes such as local climate moderation.
With predictions of rising summer temperatures in Australian cities reaching extremes by 2050, climate adaptation and mitigation actions are becoming more urgent. The potential for stormwater harvesting to support increased urban cooling during the dry summer periods, and to improve tree growth and functioning, suggests that further application and research are warranted. Further study would ideally measure the urban cooling effects of stormwater harvesting and infiltration into tree root zones, and how the effects vary between tree species. While the results of this experiment show TREENET Inlets are an effective WSUD device in terms of supporting urban tree growth and function, further research is required, particularly in relation to tree species, soil type and local climate.
The raw data supporting the conclusions of this article will be made available by the authors, without undue reservation.
XG conducted most of the field work and data analysis and wrote most of the manuscript. TJ contributed to designing the research, obtaining the funding, and edited the manuscript. GL conducted part of statistical analyses and interpreted results. YZ contributed to field work and some data analyses. HG designed the research, supervised the study, wrote part of the manuscript, edited the manuscript, and contributed to obtaining the funding. All authors contributed to the article and approved the submitted version.
This research was funded by the Adelaide and Mount Lofty Ranges Natural Resources Management Board, the City of Mitcham, and Flinders University.
The authors declare that the research was conducted in the absence of any commercial or financial relationships that could be construed as a potential conflict of interest.
All claims expressed in this article are solely those of the authors and do not necessarily represent those of their affiliated organizations, or those of the publisher, the editors and the reviewers. Any product that may be evaluated in this article, or claim that may be made by its manufacturer, is not guaranteed or endorsed by the publisher.
The authors acknowledge the kind assistance of Lawrence Burk, Zidong Lou, Na Liu, Rose Deng, Roger Clay, Kamini Singha, and David Williams during this study.
The Supplementary Material for this article can be found online at: https://www.frontiersin.org/articles/10.3389/fclim.2022.783905/full#supplementary-material
Allen, R. G., Pereira, L. S., Raes, D., and Smith, M. (1998). Crop Evapotranspiration—Guidelines for Computing Crop Water Requirements. Rome: Food and Agriculture Organization of the United Nations (FAO).
Aminipouri, M., Rayner, D., Lindberg, F., Thorsson, S., Knudby, A. J., Zickfeld, K., et al. (2019). Urban tree planting to maintain outdoor thermal comfort under climate change: the case of Vancouver's local climate zones. Build. Environ. 158, 226–236. doi: 10.1016/j.buildenv.2019.05.022
Antezana-Vera, S. A., and Marenco, R. A. (2021). Sap flow rates of Minquartia guianensis in central Amazonia during the prolonged dry season of 2015–2016. J. For. Res. 32, 2067–2076. doi: 10.1007/s11676-020-01193-9
Argue, J. (2004). Water Sensitive Urban Design: Basic Procedures for ‘Source Control’of Stormwater. Adelaide, University of South Australia.
Beecham, S., Pezzaniti, D., and Kandasamy, J. (2012). Stormwater treatment using permeable pavements. Proc. Inst. Civil Eng. 165, 161–170. doi: 10.1680/wama.2012.165.3.161
Brattebo, B. O., and Booth, D. B. (2003). Long-term stormwater quantity and quality performance of permeable pavement systems. Water Res. 37, 4369–4376. doi: 10.1016/S0043-1354(03)00410-X
Brodnik, C., and Brown, R. (2018). Strategies for developing transformative capacity in urban water management sectors: the case of Melbourne, Australia. Technol. Forecast. Social Change 137, 147–159. doi: 10.1016/j.techfore.2018.07.037
Bureau of Meteorology (2020). Climate Statistics for Australian Locations. Adelaide, Kent Town: BOM.
Burgess, S. S. O., Adams, M. A., Turner, N. C., Beverly, C. R., Ong, C. K., Khan, A. A. H., et al. (2001). An improved heat pulse method to measure low and reverse rates of sap flow in woody plants. Tree Physiol. 21, 589–598. doi: 10.1093/treephys/21.9.589
Cannavo, P., Guénon, R., Galopin, G., and Vidal-Beaudet, L. (2018). Technosols made with various urban wastes showed contrasted performance for tree development during a 3-year experiment. Environ. Earth Sci. 77, 650. doi: 10.1007/s12665-018-7848-x
Coutts, A., White, E., Tapper, N., Beringer, J., and Livesley, S. (2016). Temperature and human thermal comfort effects of street trees across three contrasting street canyon environments. Theor. Appl. Climatol. 124, 55–68. doi: 10.1007/s00704-015-1409-y
Ebrahimian, A., Sokolovskaya, N., and Wadzuk, B. (2021). Modeling dynamic performance of urban infiltration trench systems: methodology and a case study in Philadelphia. J. Hydrol. 594, 125938. doi: 10.1016/j.jhydrol.2020.125938
Edwards, W. R. N., Becker, P., and Èermák, J. (1997). A unified nomenclature for sap flow measurements. Tree Physiol. 17, 65–67. doi: 10.1093/treephys/17.1.65
Elloumi, N., Zouari, M., Chaari, L., Rouina, B. B., Abdallah, F. B., and Kallel, M. (2015). Morphological and physiological changes induced in Olea europaea and Prunus dulcis exposed to air fluoride pollution. Braz. J. Bot. 38, 99–106. doi: 10.1007/s40415-014-0112-z
Fletcher, T., Shuster, W., Hunt, W., Ashley, R., Butler, D., Arthur, S., et al. (2015). SUDS, LID, BMPs, WSUD and more—the evolution and application of terminology surrounding urban drainage. Urban Water J. 12, 525–543. doi: 10.1080/1573062X.2014.916314
Ganthaler, A., Sailer, J., Bär, A., Losso, A., and Mayr, S. (2019). Noninvasive analysis of tree stems by electrical resistivity tomography: unraveling the effects of temperature, water status, and electrode installation. Front. Plant Sci. 10, 1455. doi: 10.3389/fpls.2019.01455
Gimenez, C., Gallardo, M., and Thompson, R. B. (2005). “Plant–water relations,” in Encyclopedia of Soils in the Environment, ed. D. Hillel. (Oxford: Elsevier), 231–238.
Government of South Australia (2009). Water Sensitive Urban Design Technical Manual for the Greater Adelaide Region. Adelaide: Department of Planning and Local Government.
Grey, V., Livesley, S. J., Fletcher, T. D., and Szota, C. (2018). Establishing street trees in stormwater control measures can double tree growth when extended waterlogging is avoided. Landsc. Urban Plan. 178, 122–129. doi: 10.1016/j.landurbplan.2018.06.002
Guan, H., and Wilson, J. L. (2009). A hybrid dual-source model for potential evaporation and transpiration partitioning. J. Hydrol. 377, 405–416. doi: 10.1016/j.jhydrol.2009.08.037
Hale, R., Swearer, S. E., Sievers, M., and Coleman, R. (2019). Balancing biodiversity outcomes and pollution management in urban stormwater treatment wetlands. J. Environ. Manage. 233, 302–307. doi: 10.1016/j.jenvman.2018.12.064
Hua, P., Yang, W., Qi, X., Jiang, S., Xie, J., Gu, X., et al. (2020). Evaluating the effect of urban flooding reduction strategies in response to design rainfall and low impact development. J. Clean. Prod. 242, 118515. doi: 10.1016/j.jclepro.2019.118515
Johnson, T., Moore, G., Cameron, D., and Brien, C. (2019). An investigation of tree growth in permeable paving. Urban For. Urban Green. 43, 126374. doi: 10.1016/j.ufug.2019.126374
Klok, L., Rood, N., Kluck, J., and Kleerekoper, L. (2019). Assessment of thermally comfortable urban spaces in Amsterdam during hot summer days. Int. J. Biometeorol. 63, 129–141. doi: 10.1007/s00484-018-1644-x
Lähde, E., Khadka, A., Tahvonen, O., and Kokkonen, T. (2019). Can we really have it all?—designing multifunctionality with sustainable urban drainage system elements. Sustainability 11, 1854. doi: 10.3390/su11071854
Leuzinger, S., Vogt, R., and Körner, C. (2010). Tree surface temperature in an urban environment. Agric. For. Meteorol. 150, 56–62. doi: 10.1016/j.agrformet.2009.08.006
Lewis, S. C., King, A. D., and Mitchell, D. M. (2017). Australia's unprecedented future temperature extremes under paris limits to warming. Geophys. Res. Lett. 44, 9947–9956. doi: 10.1002/2017gl074612
Li, C., Peng, C., Chiang, P.-C., Cai, Y., Wang, X., and Yang, Z. (2019). Mechanisms and applications of green infrastructure practices for stormwater control: a review. J. Hydrol. 568, 626–637. doi: 10.1016/j.jhydrol.2018.10.074
Liu, N., Buckley, T. N., He, X., Zhang, X., Zhang, C., Luo, Z., et al. (2019). Improvement of a simplified process-based model for estimating transpiration under water-limited conditions. Hydrol. Process. 33, 1670–1685. doi: 10.1002/hyp.13430
Liu, N., Guan, H., Luo, Z., Zhang, C., Wang, H., and Zhang, X. (2017). Examination of a coupled supply- and demand-induced stress function for root water uptake modeling. Hydrol. Res. 48, 66–76. doi: 10.2166/nh.2016.173
Lu, W., Qin, X. S., and Jun, C. (2019). A parsimonious framework of evaluating WSUD features in urban flood mitigation. J. Environ. Inform. 33, 17–27. doi: 10.3808/jei.201700373
MacDonald, D. H., Ardeshiri, A., Rose, J. M., Russell, B. D., and Connell, S. D. (2015). Valuing coastal water quality: Adelaide, South Australia metropolitan area. Mar. Policy 52, 116–124. doi: 10.1016/j.marpol.2014.11.003
McDonald, R. I., Marcotullio, P. J., and Güneralp, B. (2013). “Urbanization and global trends in biodiversity and ecosystem services,” in Urbanization, Biodiversity and Ecosystem Services: Challenges and Opportunities: A Global Assessment, eds. T. Elmqvist, M. Fragkias, J. Goodness, B. Güneralp, P. J. Marcotullio, R. I. McDonald, et al. (Dordrecht: Springer Netherlands), 31–52.
Mullaney, J., Lucke, T., and Trueman, S. J. (2015). A review of benefits and challenges in growing street trees in paved urban environments. Landsc. Urban Plan. 134, 157–166. doi: 10.1016/j.landurbplan.2014.10.013
Murray, M., Soh, W. K., Yiotis, C., Batke, S., Parnell, A. C., Spicer, R. A., et al. (2019). Convergence in maximum stomatal conductance of C3 woody angiosperms in natural ecosystems across bioclimatic zones. Front. Plant Sci. 10, 558. doi: 10.3389/fpls.2019.00558
Palazzo, E. (2019). From water sensitive to floodable: defining adaptive urban design for water resilient cities. J. Urban Des. 24, 137–157. doi: 10.1080/13574809.2018.1511972
Pickett, S. T., Cadenasso, M. L., Grove, J. M., Boone, C. G., Groffman, P. M., Irwin, E., et al. (2011). Urban ecological systems: Scientific foundations and a decade of progress. J. Environ. Manage. 92, 331–362. doi: 10.1016/j.jenvman.2010.08.022
Pitman, S. D., Daniels, C. B., and Ely, M. E. (2015). Green infrastructure as life support: urban nature and climate change. Trans. R. Soc. South Australia 139, 97–112. doi: 10.1080/03721426.2015.1035219
Popek, R., Przybysz, A., Gawrońska, H., Klamkowski, K., and Gawroński, S. W. (2018). Impact of particulate matter accumulation on the photosynthetic apparatus of roadside woody plants growing in the urban conditions. Ecotoxicol. Environ. Saf. 163, 56–62. doi: 10.1016/j.ecoenv.2018.07.051
Radcliffe, J. C. (2018). “Australia's Water Sensitive Urban Design,” in 2018 International Sponge City Conference (Xi'an, China).
Rahman, M. A., Armson, D., and Ennos, A. R. (2015). A comparison of the growth and cooling effectiveness of five commonly planted urban tree species. Urban Ecosyst. 18, 371–389. doi: 10.1007/s11252-014-0407-7
Richards, P. J., Farrell, C., Tom, M., Williams, N. S. G., and Fletcher, T. D. (2015). Vegetable raingardens can produce food and reduce stormwater runoff. Urban For. Urban Green. 14, 646–654. doi: 10.1016/j.ufug.2015.06.007
Santamouris, M. (2020). Recent progress on urban overheating and heat island research. Integrated assessment of the energy, environmental, vulnerability and health impact. Synergies with the global climate change. Energy Build. 207, 109482. doi: 10.1016/j.enbuild.2019.109482
Sapdhare, H., Myers, B., Beecham, S., and Brien, C. (2019). Performance of a kerbside inlet to irrigate street trees and to improve road runoff water quality: a comparison of four media types. Environ. Sci. Pollut. Res. 26, 33995–34007. doi: 10.1007/s11356-018-3083-y
Savi, T., Bertuzzi, S., Branca, S., Tretiach, M., and Nardini, A. (2015). Drought-induced xylem cavitation and hydraulic deterioration: risk factors for urban trees under climate change? New Phytol. 205, 1106–1116. doi: 10.1111/nph.13112
Scharenbroch, B. C., Morgenroth, J., and Maule, B. (2016). Tree species suitability to bioswales and impact on the urban water budget. J. Environ. Qual. 45, 199–206. doi: 10.2134/jeq2015.01.0060
Sepúlveda, P., and Johnstone, D. M. (2019). A novel way of assessing plant vitality in urban trees. Forests 10, 2. doi: 10.3390/f10010002
Shahzad, H. (2020). “Catchment hydrology—before and after WSUD treatments,” in 21st National Street Tree Symposium, Urban Forest Festival, ed. G. Williams (Adelaide, South Australia: TREENET Inc.).
Sheng, J., and Wilson, J. (2009). Watershed urbanization and changing flood behavior across the Los Angeles metropolitan region. Natural Hazards 48, 41–57.
Szota, C., Coutts, A. M., Thom, J. K., Virahsawmy, H. K., Fletcher, T. D., and Livesley, S. J. (2019). Street tree stormwater control measures can reduce runoff but may not benefit established trees. Landsc. Urban Plan. 182, 144–155. doi: 10.1016/j.landurbplan.2018.10.021
Szota, C., McCarthy, M. J., Sanders, G. J., Farrell, C., Fletcher, T. D., Arndt, S. K., et al. (2018). Tree water-use strategies to improve stormwater retention performance of biofiltration systems. Water Res. 144, 285–295. doi: 10.1016/j.watres.2018.07.044
Tarran, J. (2009). “People and trees: providing benefits, overcoming impediments,” in TREENET Proceedings of the 10th National Street Tree Symposium, eds. D. Lawry, J. Gardner, and B. Merret, Adelaide, South Australia.
Thom, J. K., Szota, C., Coutts, A. M., Fletcher, T. D., and Livesley, S. J. (2020). Transpiration by established trees could increase the efficiency of stormwater control measures. Water Res. 173, 115597. doi: 10.1016/j.watres.2020.115597
Toky, O. P., and Bisht, R. P. (1993). Above-ground and below-ground biomass allocation in important fuelwood trees from arid north-western India. J. Arid Environ. 25, 315–320. doi: 10.1006/jare.1993.1064
Tourn, G. M., Menvielle, M. F., Scopel, A. L., and Pidal, B. (1999). Clonal strategies of a woody weed: Melia azedarach. Plant Soil 217, 111–117.
Tu, M.-C., Caplan, J. S., Eisenman, S. W., and Wadzuk, B. M. (2020). When green infrastructure turns grey: plant water stress as a consequence of overdesign in a tree trench system. Water 12, 573. doi: 10.3390/w12020573
Ugolini, F., Bussotti, F., Lanini, G. M., Raschi, A., Tani, C., and Tognetti, R. (2012). Leaf gas exchanges and photosystem efficiency of the holm oak in urban green areas of Florence, Italy. Urban For. Urban Green. 11, 313–319. doi: 10.1016/j.ufug.2012.02.006
van Dijk, A. I. J. M., Beck, H. E., Crosbie, R. S., de Jeu, R. A. M., Liu, Y. Y., Podger, G. M., et al. (2013). The Millennium Drought in southeast Australia (2001–2009): natural and human causes and implications for water resources, ecosystems, economy, and society. Water Resour. Res. 49, 1040–1057. doi: 10.1002/wrcr.20123
Wang, C., Wang, Z.-H., Wang, C., and Myint, S. W. (2019a). Environmental cooling provided by urban trees under extreme heat and cold waves in U.S. cities. Remote Sens. Environ. 227, 28–43. doi: 10.1016/j.rse.2019.03.024
Wang, H., Guan, H., Guyot, A., Simmons, C. T., and Lockington, D. A. (2016). Quantifying sapwood width for three Australian native species using electrical resistivity tomography. Ecohydrology 9, 83–92. doi: 10.1002/eco.1612
Wang, R., Zhao, J., Meitner, M. J., Hu, Y., and Xu, X. (2019b). Characteristics of urban green spaces in relation to aesthetic preference and stress recovery. Urban For. Urban Green. 41, 6–13. doi: 10.1016/j.ufug.2019.03.005
Whitmee, S., Haines, A., Beyrer, C., Boltz, F., Capon, A. G., de Souza Dias, B. F., et al. (2015). Safeguarding human health in the Anthropocene epoch: report of The Rockefeller Foundation–Lancet Commission on planetary health. Lancet 386, 1973–2028. doi: 10.1016/S0140-6736(15)60901-1
Xu, M., Hong, B., Jiang, R., An, L., and Zhang, T. (2019). Outdoor thermal comfort of shaded spaces in an urban park in the cold region of China. Build. Environ. 155, 408. doi: 10.1016/j.buildenv.2019.03.049
Yang, Y., Guan, H., Hutson, J., Wang, H., Ewenz, C., Shang, S., et al. (2013). Examination and parameterization of the root water uptake model from stem water potential and sap flow measurements. Hydrol. Process. 27, 2857–2863. doi: 10.1002/hyp.9406
Keywords: stormwater harvesting, TREENET, mediterranean climate, tree water use, sponge city, water sensitive urban design, low-impact development
Citation: Gleeson X, Johnson T, Lee G, Zhou Y and Guan H (2022) Enhanced Passive Stormwater Infiltration Improves Urban Melia Azedarach Functioning in Dry Season. Front. Clim. 4:783905. doi: 10.3389/fclim.2022.783905
Received: 27 September 2021; Accepted: 04 March 2022;
Published: 30 March 2022.
Edited by:
Michael Blum, The University of Tennessee, Knoxville, United StatesReviewed by:
Adam Schreiner-McGraw, United States Department of Agriculture, United StatesCopyright © 2022 Gleeson, Johnson, Lee, Zhou and Guan. This is an open-access article distributed under the terms of the Creative Commons Attribution License (CC BY). The use, distribution or reproduction in other forums is permitted, provided the original author(s) and the copyright owner(s) are credited and that the original publication in this journal is cited, in accordance with accepted academic practice. No use, distribution or reproduction is permitted which does not comply with these terms.
*Correspondence: Huade Guan, aHVhZGUuZ3VhbkBmbGluZGVycy5lZHUuYXU=
Disclaimer: All claims expressed in this article are solely those of the authors and do not necessarily represent those of their affiliated organizations, or those of the publisher, the editors and the reviewers. Any product that may be evaluated in this article or claim that may be made by its manufacturer is not guaranteed or endorsed by the publisher.
Research integrity at Frontiers
Learn more about the work of our research integrity team to safeguard the quality of each article we publish.