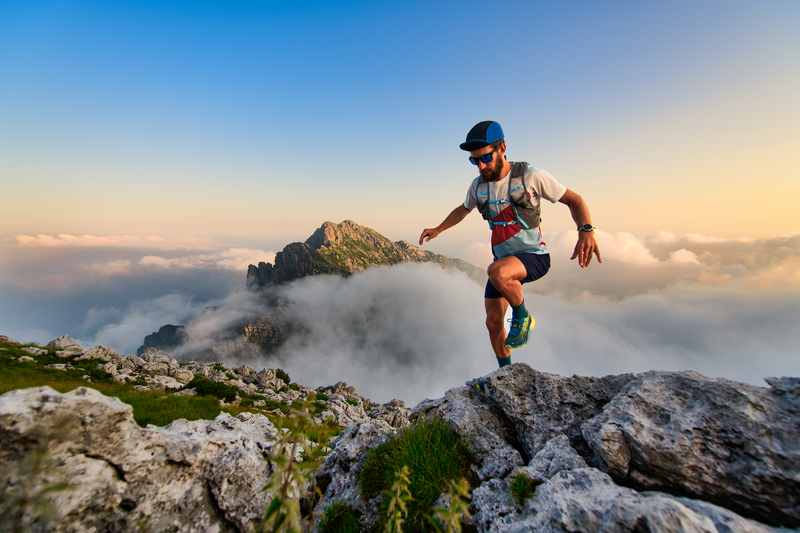
95% of researchers rate our articles as excellent or good
Learn more about the work of our research integrity team to safeguard the quality of each article we publish.
Find out more
ORIGINAL RESEARCH article
Front. Clim. , 08 August 2022
Sec. Climate Risk Management
Volume 4 - 2022 | https://doi.org/10.3389/fclim.2022.760894
This article is part of the Research Topic Climatic Hazards and Disaster Risk Reduction in South-Central America and the Caribbean View all 6 articles
The increase in urbanization processes has considerably changed urban flows, as basin impermeabilization generates increased surface runoff volumes. This induces storm floods that simultaneously generate mobility problems, infrastructure damage, and in extreme conditions, pedestrian deaths. This problem has been widely studied. For small basins with steep watersheds, however, there is little literature to be found. In these basins, the problem is even more complex, as flows are faster and more energized, given the surface slopes, which generates further problems, including drainage infrastructure damage, landslides, and fallen trees. Many solutions have been proposed, which include Sustainable Urban Drainage Systems (SUDS), which work together with the drainage network. These were studied in the present investigation, via the modeling of a basin with those characteristics typical of mountains, using SWMM's Low Impact Development LID model. It was found that rain barrels and green roofs were viable solutions, independently, although the application of additional techniques, such as cisterns and bioretention cells, is also indispensable. The present study also concludes that, although the techniques studied are implemented on a small scale, these should be both planned and implemented by municipal authorities.
As urbanization processes proceed, urban flows increase, as they modify hydrological regulation in urban basins. This is a result of basin impermeabilization, which causes lower infiltration, interception, and evapotranspiration levels, and consequently, increased volume of surface runoff (Jha et al., 2012; Hirabayashi et al., 2013; Woods Ballard et al., 2015; Huang et al., 2017; Feng et al., 2021). This generates storm floods, which occur when runoff flows overcome the flow designed for the drainage network, and subsequently creates mobility problems (Gómez Valentín, 2006; Jha et al., 2012; Ertan and Çelik, 2021), infrastructure damage, and in extreme conditions, pedestrian death. This is a broadly studied problem in basins with medium to low slopes. However, for small basins with steep slopes, there is very little to be found in the literature. In said basins, the problem is even more complex, as the flows are substantially faster, owing to surface slopes which impede the ponding process in the soil. Such is the case of Manizales, a city located in the Colombian Andes, with a medium slope of 21.9°, and altitudes between 1,563 and 2,326 m.a.s.l., where there is evidence of increased frequency of storm flooding in recent years (Rey Valencia and Marcela, 2019). There are certain zones of Manizales with very high, steep slopes and dense settlements, as in the case of the area of study: the Palogrande-San Luis subbasin. This is a tributary of the Chinchiná river, with a total area of ~2.5 km2 (IDEA, 2005). It boasts abrupt topography, with and altitude range of 1,974–2,166 m.a.s.l.
In countries worldwide, this problem has been mitigated with the implementation of Sustainable Urban Drainage Systems (SUDS), which work together with existing drainage networks (Fletcher et al., 2015; Griffiths, 2017). SUDS are a group of practices and techniques which are applied improve urban drainage integral management, and fundamentally replicate, as far as is possible, those hydrological conditions which existed prior to the urbanization process (Fletcher et al., 2015). SUDS techniques include a number of structural techniques, such as wetlands, swales, infiltration trenches, rain deposits, bioretention techniques, and vegetative swales, among many others. They also incorporate non-structural techniques such as new road and building designs that minimize impermeable areas and maximize the use of permeable soil, vegetation, and contaminant reduction, not to mention educational programs that seek to modify behavior. In terms of structural measures, small-scale measures that control runoff generation at the source are emphasized (Woods Ballard et al., 2015).
Among the techniques studied for flow retention is rain water harvesting, specifically, tanks or domestic storage, as on-site means to reduce flow generation during precipitation events (Sample and Liu, 2014; Burns et al., 2015). Of these, those which are most often-suggested are commercial models (rain barrels, rain cisterns, etc.) although they have minimal storage capacity. However, theory indicates that the efficiency of these is greatly influenced by local precipitation patterns and the characteristics of the area in which they are installed (Ward et al., 2012; Steffen et al., 2013; Campisano and Modica, 2015), such that their efficiency in mountainous cities with space limitations should be examined.
Green roofs have also been widely used, and various studies have demonstrated their efficiency in mitigating peak flows, reducing pollution, improving ecosystemic services, and generally improving those climatic conditions associated with comfort (Cohen et al., 2012; Baptiste et al., 2015). Green roofs have been studied in detail both on the individual roof (Villarreal, 2007; Hilten et al., 2008; Kasmin et al., 2010; Metselaar, 2012; Stovin et al., 2012; Locatelli et al., 2014; Carson et al., 2015; Krebs et al., 2016; Johannessen et al., 2019), and basin levels (Carter and Jackson, 2007; Ashbolt et al., 2013; Krebs et al., 2013; Palla and Gnecco, 2015; Rosa et al., 2015; Warsta et al., 2017).
Another small-scale technique is that of bioretention cells, which are shallow, surface garden depressions designed to reduce and manage runoff, through landscape improvements in the areas in which they are implemented. These consist of shallow depressions for the temporary accumulation of water, a layer of fertilized soil, in which vegetation coverage is planted, a filtering medium and linings to prevent infiltration, where inappropriate (Woods Ballard et al., 2015). Additionally, these function as contaminant removal systems, and may easily be incorporated into road, residential, commercial, or industrial areas (Toronto Region Conservation Authority, 2010).
Despite that expressed above, each technique relies upon application site conditions, as well as slopes and the way in which the precipitation falls, to be efficient. The matter of slopes is of special interest, given that, in mountainous areas, these may be the main causes of flow increases. The objective of the present investigation was to evaluate the behavior of the selected SUDS, which in this case, encompassed: detention deposits, green roofs, and bioretention cells (with linings to avoid undesirable infiltration). For this analysis, the Palogrande San Luis pilot basin was selected, as it presents the above-mentioned characteristics. In said basin, the SWMM hydrodynamic model was implemented to simulate the behavior of the current network, and it was found that basin response is quite rapid. The SUDS proposed were evaluated by means of the model validated in SWMM to determine hydraulic efficiency.
The pluvial floods analysis was carried out in the city of Manizales, the capital of the Caldas department, located in the central-western region of Colombia (Figure 1). Its urban area totals 439.36 km2, and its population was 396,075, in 2015 (DANE, 2019). It presents mountainous characteristics, with V-shaped geoforms, steep slopes, at 25%, and an average altitude of 2,153 m.a.s.l. Owing to its geographic location, it has a bimodal climate with two seasons with high precipitation levels, and two with low precipitation levels, both for intra-annual and diurnal cycles (Delgado et al., 2020). It boasts high levels of climatic variability, with half yearly and multiannual precipitations of 2,178 mm, a bimodal precipitation regime, and significant spatial and temporal variability (Rincón et al., 2015; Delgado et al., 2020). In Manizales, eight microclimates have been identified, with precipitation values of between 100 and 500 mm, during the rainy months (Upegui et al., 2015).
Manizales is home to an extensive meteorological network, with 46 monitoring stations in 2021: 22 meteorological stations, 24 hydrometeorological stations that measure precipitation, temperature, and river levels. The remainder are support stations as alarm stations, and plant headquarters. These stations send data to the collection center at the Institute for Environmental Studies (IDEA), at 5-min intervals (UN and CORPOCALDAS, 2014).
Manizales's lowest levels are at its principal tributaries, the Chinchiná River and Olivares Creek, such that runoff flows toward those natural drainage points. Said tributaries also have their own tributaries, although in the Olivares Creek basin, many of these were covered completely, in order to capitalize on constructible land. Parallel to the main tributaries are the city's main roads: next to the Olivares Creek is the Avenida Kevin Ángel, and next to the Chinchiná River is the Avenida Panamericana, a principal access road to the city. The Avenida Santander is the third main road, and occupies the middle-ground between the basins of the main drainage watersheds.
In order to determine which mechanisms collide to generate storm floods in the city, modeling was applied to the urban Palogrande-San Luis Basin (Figure 1), which has steep slopes, where hydraulic modeling occurred by way of the SWMM model, a hydrodynamic runoff water model (Rossman, 2015; Rossman and Huber, 2016). This model revealed the ways in which surface runoff was produced in Critical Zones, and how drainage networks were shared, such that recommendations could be generated for storm flood mitigation.
Within the basin, there are four main soil uses: forests, scrub, and low and medium-intensity urban areas, in accordance with the map created by Cabezas Suárez and Zambrano Nájera (2016). The aerial photographs of the area led to the identification of impervious surfaces, soil roughness coefficients, and subbasin parameters (Figures 2A,B). The upper part of the basin is mainly urban, and houses educational and athletic complexes (slopes between 1.2 and 34.5%). The lower part contains forests with slopes between 22.6 and 56.4 % (Figure 2D). Area soils (Figure 2C) include clay, silt and silty sand areas, with permeabilities between 0.025 and 5 mm/h. The most permeable soils are in the upper part, and the least permeable area is in the lower and forested parts (Giraldo, 2017). Based on the drainage network (Hoyos and Felipe, 2003; Aguas de Manizales, 2014), manhole information was obtained for pipes and outfalls (Figure 2B). This revealed that almost the entire basin deposited its rain and waste water into the Palogrande Creek, which presents a concentration of area networks that represents ~69 % of the basin's area (Figure 2D).
Figure 2. (A) Base coverage and soil use maps, (B) Discretization, (C) Soil map with hydraulic conductivity, and (D) Sewer network.
Hydrodynamic modeling was performed on the area of study, by way of SWMM, Storm Water Management Model version 5.1.012, a dynamic runoff water model that is used for unique events or the continuous simulation of urban drainage systems. It is widely used, at present (Rossman, 2015, 2016). In order to model the hydrology in the area of study, interception, surface storage, infiltration, and rainfall-runoff process were considered. Interception and surface storage were determined using land cover map and parameters recommendation by Hromadka and Whitley (1989), Tsihrintzis and Hamid (2001), and Rossman (2015). For each type of coverage Manning roughnesses were identified, in accordance with the recommendations of the United States Department of Agriculture (USDA-ARS, 2008). Infiltration was calculated using Green-Ampt model, for which soil parameters were taken from data obtained by Giraldo (n.d.) who carry out different soil surveys in the basin, to obtain: saturated hydraulic conductivity (Ks) and suction potential (ψ). The initial moisture deficit (Δθ) was assumed to be 0.05 for all sub-basins (Figure 2C). Rainfall-runoff was modeled via the dynamic wave method.
For hydraulic modeling a Digital Model Elevation DEM with a resolution of 10 × 10 m was selected. This was identified as optimal because it adequately represented the topographic characteristics of the area of study. With this DEM, the average slope was calculated for each sub-basin, and the surface flow direction was defined for the discretization. The discretization was completed by checking the surface flow direction obtained from the DEM and the flow direction of the sewer system. For the wooded area, only the direction surface flow was considered. Thus, the basin was divided into 92 subbasins (Figure 2B), with areas of between 0.30 and 4.08 ha. Information regarding manholes, conduits, and overflow discharges was obtained from the sewerage network map. The missing invert levels were completed by field data, information reported in Hoyos and Felipe (2003) and interpolation with nearby manholes. The entire sewerage network flows to the San Luis river, and so the river was included within the model as a rectangular channel, its height was taken from the maximum values recorded by the flow gauge station located at the outlet of the basin, called Ruta 30 gauge, and the parameters of width and coefficient of roughness were taken from field visits. Roughnesses were calibrated with hyetographs-hydrograms observed at the Ruta 30 station.
The calibration and validation process was made using the fitness indicators volumetric error percentage (M), percent bias (PBIAS), and the Nash-Sutcliffe (NSE) indicator.
Where Qsim, i is the flow simulated for period i (l/s), Qobs, i is that observed (l/s). For the calibration, five (5) events were selected, four of which presented a single peak flow. The eleven (11) events chosen for the validation presented one or various peak flows, with durations of between 60 and 110 min (Table 1). In the basin, as in the city, the majority of events are short-lived (30 and 90 min), and very intense (Rincón et al., 2015).
Satisfactory results were obtained for both calibration and validation, with NSE values between 0.76 and 0.94, PBIAS values between −30.09 and 8.33 and volumetric errors between ± 7 and 18% for calibration (Figure 3). NSE values between 0.5 and 0.91, and volumetric errors between ± 0.4 – 15% were observed, with the exception of one event that presented a 31% error for validation.
With the model calibrated and validated, different LID techniques were simulated. For this, the LID (Low Impact Development) SWMM module was applied (green roofs, rain barrels, and bioretention cells), in order to evaluate possible solutions to the problem. The hydraulic analysis was performed with rain obtained with the IDF from the Agronomy meteorological station (Pareja and Osorio, 1997), with a 22 min concentration time (Vélez Upegui et al., 2011) and a return period of 2 years, as recommended in Colombian regulations (Ministerio de Vivienda Ciudad y Territorio, 2017). Additionally, a temporal distribution corresponding to the 95th percentile was taken (Rincón et al., 2015), as this is a critical temporal distribution. The hydrological behavior analysis occurred by way of the percentage difference in flood flow, flood volume, and total flood time for each manhole evaluated in the critical area, before and after each SUDS implementation.
To select the SUDS alternatives to be implemented, a literature research of the different existing typologies was carried out, which were analyzed with respect to the limiting factors of mountain cities: (1) lack of space, (2) in Manizales the accumulation of water in the ground triggers landslides, therefore the SUDS that favor infiltration cannot be used (3) high surface slopes.
From the available SUDS, green roofs and rainwater harvesting are two strategies that are applicable in the dense urban center, particularly for multifamily complexes. Permeable pavement and bioretention are also highly adaptable, and may provide benefits in a variety of land use settings. Permeable pavement can be very effective around trees and associated subsurface planting soil structures, but are also very costly. For the present study, green roofs, rain barrels, and bioretention cells were selected. The location of each SUDS was determined in accordance with the space available in each case: roofs with appropriate slopes for green roofs, habitational units for rain barrels, open space for cisterns, and green space for bioretention cells. Topography was not a limitation, as those SUDS selected were small-scale.
Green roofs capture runoff via a layer of vegetation and soil, installed on top of sloped roof, although these can be installed on flat roofs, as well. The rooftop vegetation allows for evapotranspiration processes to reduce volume and discharge peak runoff from entering the conveyance system. They can be extensive, with thin soil layers, and are lighter, less expensive, and generally require low maintenance. Another type is intensive green roofs, which are characterized by a deeper soil layer, for which reason they have higher weights, capital costs, and have more maintenance requirements (Government of the Hong Kong Especial Administrative Region, 2013; Woods Ballard et al., 2015; Rossman and Huber, 2016). In general, green roofs have the following components: supporting structures capable of supporting the weight, a waterproofing barrier layer, designed to protect the building, a drainage layer, which consists of porous media, that captures and stores water for plan uptake and storm buffering, a geosynthetic layer, to prevent clogging of the porous media, and plants, which may be adapted to regional climate variation, with shallow rooting depths (Government of the Hong Kong Especial Administrative Region, 2013; Woods Ballard et al., 2015; Rossman and Huber, 2016). Green roofs were selected, as these can be adapted to all types of roofs, and are considered most effective at reducing runoff volume and rates for land uses with different percentages of rooftop coverage, including commercial, industrial, or multifamily housing (Stephens et al., 2002). It has been proved that these can retain as much as 80% of total annual runoff, in areas with ~70% of impervious area (Stephens et al., 2002). As an initial scenario (Scenario 1), extensive green roofs were installed, as the roofs in the area lacked transport capacity, and areas apt for their implementation were selected. Areas were identified by means of aerial photography and the Open Layer tool (Kalberer and Walker, 2018) from Qgis. This required total roof areas of 25,910.3 m2. Roof design occurred in accordance with the recommendations of Carson et al. (2015) (Table 2).
Second, rainwater harvesting, with rain barrels, was explored, as this technology has gained significant public attention in Colombia. Rainwater harvesting is also a means by which to reuse water for a number of domestic purposes, such as car washing, filling swimming pools, and lawn and landscaping irrigation, among others. Rain barrels and cisterns may be constructed of any material, whether on-site or pre-manufactured, sizes vary by hundreds to gallons for residential uses, and storage systems may be located either above or below ground (Government of the Hong Kong Especial Administrative Region, 2013; Department of Environmental Conservation, 2015; Woods Ballard et al., 2015). The basic components of rain barrels and cisterns include: watertight containers, secure covers, coarse inlet filters, overflow pipes, manholes or access hatches, drains for cleaning, and extraction systems. Advantages of rain barrels and cisterns include that they may be used in most areas (residential, commercial, or industrial), have minimal site constraints, could be applied to manage almost every land use type, including those of dense urban areas. Benefits include the reduction of storm water runoff that enters the drainage system, both in terms of volume and peak discharge, the reduction of transport of pollutants associated with atmospheric deposition on rooftops into receiving waters, especially heavy metals and other airborne pollutants (United States Environmental Protection Agency, 2005), and the reduction of water consumption for non-potable uses, which in turn reduces the demand on municipal water systems (Department of Environmental Conservation, 2015).
In this research, two types of rain deposits were implemented: 10,000 l cisterns (Scenario 2), and commercial 500, 600, 1,000, 1,100, and 200 l barrels (Scenarios 3–7, the parameters applied may be observed in Table 3). The C coefficient of barrels was determined by means of the equation proposed by Villalonga (2016). The n exponent was set at 0.5, and a retention time of 1 h was determined for all barrels. Additionally, one two-level option was considered for those barrels with best performance, thus maximizing the number of barrels to be installed. Four 1 m3 barrels were installed (Scenario 8). To determine the number of barrel units, the roofs for each of the sub-basins were identified and classified according to their area, from <100 m2 to >300 m2, in ranges of 50 m2. On the other hand, for the 1,000 l option it is increased to a height of 1,030 to 2,060 meters. In the case of the 2,000 l barrel option, the height of each unit is preserved.
Bioretention cells are a filtering technique. They collect rainwater and return it to the conveyance system, or allow it to partially infiltrate into the soil. These facilities normally consist of filtration beds, ponding areas, organic or mulch layers, and plants. Cells are implemented on the landside to treat runoff from roadways, parking lots, and commercial buildings (here runoff from roadways was the goal). Bioretention systems perform well for pollutant removal, as sediment is removed through filtration. Additionally, they perform well for runoff volume reduction. One of the major constraints thereof, however, includes clogging, due to accumulated sediment, waste, and other debris form roadways, thus annual maintenance is required, such as replacing mulch and dead or diseased plants (Government of the Hong Kong Especial Administrative Region, 2013; Woods Ballard et al., 2015). According to that, a ninth scenario (Scenario 9) was configured, in which, together with the barrels with the best performance (Scenario 7), bioretention cells were implemented. The bioretention cells were configured in 3.0 × 0.8-m modules, in accordance with the longitude considered for each of the subbasins in which the alternative was implemented, capturing the flow from half of the roadways in the area of influence (Table 4).
The results from the different scenarios will be reviewed in one of the most critical areas of the zone, as observed below (Figure 4). This zone has four outputs, which correspond to manhole 20,084, 20,082, 20,101, and 20,073. The responses in manholes 20,084 and 20,073 were analyzed, as these are the only two that present flooding.
The analysis of storm flooding revealed the existence of indications that the frequency of said floods had been on the rise (Corporación OSSO and Universidad EAFIT, 2011; UNISDR et al., 2016; Rey Valencia and Marcela, 2019). The communes (city-wide administrative divisions) in which flooding occurred were reviewed in detail, and it was concluded that there were points, which hereinafter will be called Critical Zones, or CZ, which were more likely to flood. These correspond to (1) road and steep slope convergence areas, (2) access stairways to neighborhoods with steep slopes to roadways, and (3) natural slopes with steep slopes to roadways. Just two periods were identified, owing to the scarcity of continuous registries of flooding. Said periods were between 1953–1986 and 2009–2016. During said periods, a significant increase in storm flooding was observed in the Palogrande, Ecoturístico Cerro de oro, La Fuente, La Estación, Ciudadela del Norte, and La Macarena communes. All of these are zones with large amounts of CZ, owing to steep slopes (Figure 5). On the other hand, the center of the city (the San José and Cumanday communes) is relatively flat, but storm flooding registers are also notable. This zone is home to the city's oldest drainage network, and has a high population density, for which reason it is inferred that flooding may owe to a hydraulic capacity problem, which would render the topography irrelevant, in this case.
In those communes concentrated in the northern region of the city, those in the Olivares Creek basin, there is additional flooding in both of the periods analyzed, which is attributed to the way in which urban expansion has occurred in the city. This has involved high levels of impermeabilization and the destruction of natural drainage sites (Aguilar Gómez, 2010; Correa and Duque, 2015). Similarly, in accordance with that documented by Aguilar, the creation of those communes in the southern area of the city, which empty into the Chinchiná River, was initiated following 1980, thus altering hydrological behavior from that point on (Aguilar Gómez, 2010). This is reflected in more commonplace flooding during the 2009–2016 period. On performance of a correlation between flooding and precipitation events registered on the same dates, it was observed that said events had magnitudes of between 15 and 70 mm, with durations of between 58 and 135 min, and average intensities between 10 and 41 mm/h (Rey Valencia and Marcela, 2019). In other words, it was confirmed that said events were both short, but of significant magnitude.
Flooding in CZ is more severe, as the surface and network velocities are quite high, which accelerates the flow all the more, and generates additional peak flows. Those zones where flooding is located, owing to their topographies, are densely-packed, and have little green space that might offer a place in which to implement solutions. The analysis of this type of flooding, performed with the SWMM model, regarding the Palogrande-Ruta 30 Creek shows that storm flooding is rapid, and begins almost together with the precipitation event (Figure 5). For the precipitation event analyzed, it was observed that the modeled system rapidly surcharged (nearing min 15), with significant increases in the energy present in pipes and flow velocities. At min 15 (see Figure 6), certain pipes may be observed to be working under critical conditions. By min 20, the first manholes are flooded. The most critical model condition occurred at min 30, where there were a total of 127 manholes flooded, of which 43 presented values above 100 l/s. On the other hand, at the same minute, there were 201 pipes (51.1%) in excess of the 93 % capacity limit.
The majority of basin soils are minimally permeable, they have steep slopes, and as such, the volumes of surface runoff generated correspond to nearly the totality of the precipitation, thus achieving runoff coefficients between 58 and 98%. In the upper part of the basin, surface runoff corresponds to ~90% of precipitation in all subbasins. In the lower part, the runoff generated varies in accordance with the level of impermeabilization. Flow velocities in the middle of the basin may exceed 10 m/s in pipes with slopes between 24.1 and 68.9%, while in the upper part, velocities between 5 and 10 m/s also occur.
The urban area of the basin presents considerable levels of impermeabilization, with high home densities, caused by a lack of land apt for construction in the area. This hampers the drainage network response. Recall that it has been documented by other authors that, in Manizales, many natural drainage areas have been waterproofed, which aggravates the situation even more (Aguilar Aguilar Gómez, 2010; Correa and Duque, 2015). The red line in Figure 5 shows, approximately, the limit of those CZ described previously (in this case, they are all Type 1—roadway convergences with high, steep slopes). It is there where almost all flow accumulation processes advanced upstream, and generated flooding. This provoked energy head of up to 18 meters, in the worst of cases (in 17 manholes, energy heads between 10 and 18 m were generated), many of which remained flooded for up to 2 h. The model permitted the evaluation of the typical way in which flooding occurs in this type of basin: flows are initiated rapidly, with the precipitation event, in part owing to the intense nature of precipitation in this type of basin, which was not evaluated in the present study, but which has been documented by other authors (Rincón et al., 2015; Delgado et al., n.d.). They also end abruptly, owing to the fact that subbasins are small, and so transit times are short (approximately by the 1 h mark, runoff flows have been evacuated). The velocities reached, especially in the so-called CZ, thanks to steep slopes, are quite high: in this case up to 10 m/s. This generates larger peak flows than those initially considered in the designs, and so, they cause the system to be overloaded quickly.
Consequently, the energy generated in the system is also quite high, which creates serious infrastructure problems as mentioned in the introduction. As such, it is especially important to control flow speeds by way of detention/retention techniques. That reinforced by the theory noted in the introduction, indicates that the network problem is not necessarily a capacity problem, but rather a velocity problem. As such, drainage network expansions are not considered absolute solutions. Instead, integral techniques, such as SUDS, should be considered. With the model, that expressed is confirmed, and it is observed that it is even more serious, owing to the topographical characteristic of this type of basin. Thus, the study of SUDS technique implementation is important. However, in this case, space problems are present which impede the implementation of the most widely-used techniques, such as infiltration. Additionally, the frequent occurrence of landslides in the area would not permit the implementation of any such technique. For this reason, in the present study, certain SUDS techniques that could be considered small-scale (green roofs, rain barrels, and bioretention cells) were examined.
Green roofs, acting independently from other techniques, achieved significant runoff flow attenuation (57% of the total volume of all manholes). The number of manholes flooded remained almost the same as the initial condition (Table 3), but the flooding volume in the manholes was reduced significantly. Minute 35 remained critical, as the condition without SUDS, but flooding at this time was reduced by half. Specifically, at outlet 20,084 (Table 5), the flood volume was completely retained, while in manhole 20,073, where the majority of the flow from the zone analyzed was deposited, 40% of the flood volume was reduced, as was 50% of the peak flow. For the rest of the zone, roofs generated reductions in flood peak flows of between 10 and 35%. They, without fail, surpassed the limits imposed by Colombian regulations, at 25%. Further, the flood volume was diminished for all manholes, by over 57%, which achieved total attenuation in the aforementioned manhole. However, it should be noted that the destination of areas is considerable (50.31%), which would impose significant maintenance costs on a large part of the population in the area examined. Thus, green roofs are an ideal practice for the retention and mitigation of the effects of intense precipitation, and should be seriously considered for implementation in new urban areas, where the development trend is similar to the selected area of study. If these are included from the beginning, they will be considerably more economical. However, this technique is more costly than others, and as such, must be contemplated in general planning programs, and should not depend on owner permission.
Scenario 2, which consisted of the implementation of two cisterns, showed a total flood volume reduction for outlet manhole 20,084, owing to cistern location, which remained in subbasins 4.1 and 4.5, respectively (Table 6). For oulet manhole 20,073, a 13% reduction in flood volume and 36% reduction in peak flow was achieved, which makes this a successful measure (Table 6). Manholes flooded with this SUDS are almost the same as for green roofs that in turn is similar to initial condition. Reduction in total volume for all the zone is just of 2% (Table 5). It should be noted that the cisterns occupied quite a low percentage of the subbasin area. However, they achieved a significant reduction in flood volume and peak flows.
Unlike the cisterns, scenarios with small barrels (3–6) did not achieve the expected results, as they did not reduce flood flows in manholes, and the channels continued to work under pressure. On evaluation of the impact on flood flows, it was observed that, in manhole 20,084, the first scenarios, with tanks, Sc. 3–6, were only able to decrease flood volume by 10% at the outlets (Table 6) and other manholes in the zone (Table 5), and there was a slight increase in peak flow. In manhole 20073, which receives the majority of the flow, scenarios 3–6 produced results different from those expected, as they generated increases in runoff volumes and peak flows (Table 5). This behavior has been documented by other authors, who indicate that this type of technology is less effective for precipitation of greater magnitudes (Holman-Dodds et al., 2003; Chaosakul et al., 2013). The area occupied, compared to the total area observed, was quite similar for scenarios 3–6, in terms of cistern area, but the results were considerably different in hydraulic terms. Additionally, the maintenance effort required for inhabitants in scenarios 3–6 would be quite high, as, although barrels are technically relatively economical, they require constant maintenance. Cisterns, on the other hand, were implemented in public areas, for which the city drainage system would be responsible.
In order to achieve greater decreases in flood volumes and peak flows, it would be necessary to considerably expand the area for tanks, by way of scenarios seven and eight. In said scenarios, for manhole 20,084, a total decrease in flood volume, and as such, peak flow, was achieved (Table 5). For manhole 20,073, which receives the majority of the flow from those tanks in scenarios 7–8, 81 and 89% of flooding was avoided, and similar relief was observed in peak flows. For other manholes in the zone, performance was very similar, with 62 and 63% reductions in total volume (Table 3), although as in the other SUDS, the number of manholes flooded remained almost the same as the initial condition. Reductions at critical times were significant. It is notable that the behavior both for the two-level 1,000 l option and the one-level 2,000 l option were very similar, which demonstrates that both alternatives constitute valid peak flow mitigation solutions. However, in the case of Scenario 8, less space is required. Regarding the previously-mentioned barrel techniques, twice the space is required, which implies much greater effort on the part of the population, in terms of maintenance. They might also have the advantage of being able to incorporate the reuse of stored rain water (which was not contemplated in the present study), and with this, the home's initial investment would be compensated by the reduction in the consumption of potable water. These techniques present multiple advantages: reductions in the consumption of potable water for uses which do not require this, such as toilets, cleaning of outdoor areas, irrigation, etc. Additionally, it is quite economical and easy to implement. However, in order for it to be functional, there must be a large number of units, and so it cannot be left to the whim of homeowners, but must rather be contemplated seriously in planning and drainage structure programs.
On the addition of bioretention cells to rain deposits, the best performance was achieved (the two-level 1,000 l barrels), the flood volume was completely contained, and as such, so too was the peak flow, in manhole 20,084, total attenuation of the flood volume occurred, which had not been achieved with the previous techniques. The reduction in the total flooding volume was greater than all other techniques applied, at 64% (Table 3). This technique presented an additional advantage: that it was implemented in the green space on sidewalks, which have the potential to improve the aesthetics of said areas.
When SUDS performance was compared in the outfall of the analyzed area (Figure 7), it was observed that the rain barrels implemented in scenarios four and five did not achieve significant mitigation, while the barrels in Scenario 6 made a significant difference in peak flows. However, there was a marked difference with green roofs (the purple line) insofar as peak flows. With that in mind, scenarios seven and eight considerably reduced peak flows, and with scenario nine, total mitigation thereof was achieved.
Figure 7. Comparison of the hydrograph, in manhole 20,073, in its initial condition vs. to the different techniques applied.
SUDS have been recognized in Colombia as a solution for problems of rainfall surplus management. In this regard, several cities have started to implement them as individual initiatives. However, these research show that these initiatives are insufficient if not applied in a massive way. This, coupled with the fact that the problems are more serious in densely populated urban areas with little space to implement more efficient SUDS, makes implementing solutions more complex. For dense areas, several authors recommend few SUDS, including water storage, pervious pavement, soakaways, infiltration trenches, and green roofs. As modeled by Saldarriaga et al. it was demonstrated that storage units or rain barrels could be a highly efficient solution, even considering climate change (Saldarriaga et al., 2020). The authors also established that these solutions were feasible, depending on available space, such as parking lots, parks, and institutional buildings. Furthermore, in the study, better results were yielded in Bogota than for Medellin, as the latter has steeper slopes and less available space (Bustos and Saldarriaga, 2017). Therefore, for densely populated areas, the implementation of small-scale SUDS is proposed, as a public policy for environmental authorities. In fact, many guides published worldwide have established certain limitations for SUDS location, such as highly-impervious areas, reduced land availability for surface structures, smaller green areas, and emphasis on the need for planned, communal, open public space for new developments (Kellager and Laughlan, 2005; Government of the Hong Kong Especial Administrative Region, 2013; Department of Environmental Conservation, 2015; Woods Ballard et al., 2015). Cultural and socio-economic situation of the densely populated neighborhoods of Manizales allows to conclude that private initiatives of the community are not feasible. Thus, it is required that authorities in charge of network management to implement public policies for this type of infrastructures. As such, European legislation for the compulsory installation of SUDS for all new developments might be mentioned, thus removing the right to automatic sewer connections for housing, causing dependence on authority approvals. In Colombia, SUDS are still an option for builders, although as mentioned previously, many are already implementing individual SUDS solutions. There are no records of those solutions impeding city plan and design solutions for storm water management.
Storm flooding in the city of Manizales has become more frequent since its documentation began, and different phenomena have been registered to be associated with these, such as landslides and fluvial floods. The precipitation associated with said floods are short-lived events of significant magnitude, which coincides with that documented by different authors, on an international level. In Manizales, the problem could be categorized as of an intermediate, as it is becoming more frequent and generates concern in the community and among the environmental authorities. It was found that the increase in runoff volumes was closely associated with city development, which has been accompanied by high levels of basin impermeabilization and the disappearance of a large natural streams.
The precipitation events that could be correlated with runoff floods presented characteristics which included short duration and significant magnitude, which corresponded with the fact that microbasins in the city are mostly small, and have low concentration times. This added to the steep slopes produces quick runoff flows that generate peak flows far beyond those contemplated in the original drainage infrastructure designs, as well as short evacuation times. This means that floods are generated quickly in the city, but also, that they are evacuated rapidly. Flows, then, are supercritical with very high energy levels, which cause considerable damage to drainage infrastructure and soils and coverages, such as landslides and trees falling. These types of situations generate great concern regarding runoff flooding with environmental authorities, as these precipitations also imply their constant attention. The above concern is also ratified on the global level, in an attempt to control flow velocities and the generation of peak flows, which is the only variable currently verified in national Colombian regulations, and to perform said controls at the source.
This implies the need for the evaluation of SUDS techniques, which may adapt to this type of environment (small basins with steep, dense slopes with little space in which to install large interventions). In the present study, surface runoff was modeled via the SWMM tool, version 5.1.011, in a study basin with the characteristics proposed. The model appropriately simulated runoff in the area of study, with a satisfactory adjustment level above 0.8 for calibration, and above 0.5 for validation, evaluated via the Nash-Sutcliffe indicator. Additionally, the model appropriately simulated the hydric balances with volumetric errors between ±10–20% for calibration events and ±10–30% in validation. With said model, the hydraulic behavior mentioned above was corroborated, in which normal design precipitation (in accordance with the norm) provokes almost immediate flooding, evacuation times of maximum 1 h, and input power of almost all pipes and manholes located in very dense, waterproof, steep areas with steep slopes or brusque slope changes.
With the model calibrated, various types of SUDS were incorporated that could be implemented on high slopes with little space, and green roofs were tested, as were water deposits (cisterns and water barrels) and bioretention cells. These were specifically observed in a subzone with steep slopes that could be evaluated under critical conditions. For each, their capacity to mitigate flood volume, peak flow of the inundation hydrogram, flooding initiation time, and the ability to change flow velocities in pipes were evaluated. The SUDS were evaluated using 2 year return period precipitation, with a critical temporal distribution and a duration of 25 min, which is very normal precipitation in the zone.
Cisterns performed well, and achieved a decrease in peak flows, and especially in flood volume, above that requested in Colombian regulations. However, total attenuation was not achieved, as it was difficult to find space for cisterns. Cisterns were shown to be very efficient in rain water management, and this is a practice that is being employed a great deal by owners of large tracts of land. For this reason, it is imperative to stimulate the use of these types of devices, and that the municipality include them in key areas.
On the other hand, small commercial rain barrels (500, 600, 1,000, and 1,100) did not reach the expected results, as storage was too minimal to be effective in the majority of cases, owing to high flow velocities. This type of barrel is easy to install, cheap, and requires little space, but have the notable disadvantage that they must be installed by homeowners, which implies that they must also be saddled with their maintenance, which is impractical. However, it is an alternative considered appropriate, considering the minimal space available in the area. As such, two more robust alternatives were contemplated: 2,000 l barrels in the maximum area possible, and 1,000 l barrels stacked two-high. These alternatives, unlike the previous options, achieved significant results in the reduction of flooding volumes in manholes, peak flood flows generated, and decreased flood times. In fact, for several areas with lower flow, total attenuation was achieved. Also, the 1,000 l barrels, stacked two high, was evaluated, acting together with bioretention cells, with which flooding was entirely prevented.
Green roofs performed well, as in all cases, output flows were delayed. This type of SUDS considerably mitigates flooding, with decreases in excess of 25 % of the peak flow. Its effectiveness owes to the fact that it is able to retain or store intense rain, as is the case with design rain. It is also notable that this does not require additional planting areas. Similarly, however, green roofs require homeowner permission and their commitment to maintenance.
As a general conclusion green roofs can be considered in new areas and high areas because are most effective but also costly than other techniques. Rain barrels in dense packed areas are effective if they are configured 1,000 l barrels stacked two high or 2,000 l, and as a municipality program.
For those alternatives that return flows to the drainage system, as was the case of the SUDS modeled in this particular study, one might consider the use of rain gardens or bioretention cells, which may be efficient for the complete attenuation of flood flows. These, additionally, have the advantage that they may use the green space in gardens or on sidewalks and offer potential aesthetic improvements. As such, their incorporation is recommended, as urban improvements, as soon as possible.
These alternatives are proposed as tools for urban planners and constructors to consider when designing drainage networks. In the case of the SUDS evaluated, consider those which favor lags over storage for drainage on steep slopes. Complementary techniques will still be studied for each proposal.
The raw data supporting the conclusions of this article will be made available by the authors, without undue reservation.
All authors listed have made a substantial, direct, and intellectual contribution to the work and approved it for publication.
The authors declare that the research was conducted in the absence of any commercial or financial relationships that could be construed as a potential conflict of interest.
All claims expressed in this article are solely those of the authors and do not necessarily represent those of their affiliated organizations, or those of the publisher, the editors and the reviewers. Any product that may be evaluated in this article, or claim that may be made by its manufacturer, is not guaranteed or endorsed by the publisher.
This article is based on the results of the thesis entitled, proposal for a sustainable urban drainage system for high slope mountain basins, carried out in the master's program in Hydraulic Resource Engineering.
Aguilar Gómez, M. (2010). Transformación de La Estructura Verde En Una Ciudad Intermedia Tropical Andina Caso Ecoparques Manizales = [Transformation of Green Structure in an Intermediate Tropical Andean City: Case Ecoparks Manizales]. Manizales: Inédito.
Ashbolt, S., Aryal, S., Petrone, K., McIntosh, B., Maheepala, R., Chowdhury, S., et al. (2013). Can stormwater harvesting restore pre-development flows in urban catchments in south east Queensland? Water Sci. Technol. 67, 446–451. doi: 10.2166/wst.2012.582
Baptiste, A. K., Foley, C., and Smardon, R. (2015). Understanding urban neighborhood differences in willingness to implement gi measures: a casestudy of Syracuse, NY. Landsc. Urban Plan. 136, 1–12. doi: 10.1016/j.landurbplan.2014.11.012
Burns, M. J., Fletcher, T. D., Duncan, H. P., Hatt, B. E., Ladson, A. R., and Walsh, C. J. (2015). The performance of rainwater tanks for stormwater retention and water supply at the household scale: an empirical study. Hydrol. Proc. 29, 152–160. doi: 10.1002/hyp.10142
Bustos, K., and Saldarriaga, J. (2017). “Test of a methodology for storm tank location in sectors of Bogotá and Medellín cities,” in XV Seminario Iberoamericano de Redes de Agua y Drenaje, SEREA2017 (Guanajuato).
Cabezas Suárez, C., and Zambrano Nájera, J. Z. (2016). “Evaluación del impacto de los cambios de usos del suelo sobre la hidrología de cuencas urbanas caso de aplicación cuenca san luis,” in 7o Encuentro de Semilleros de Investigación—Nodo Caldas Red Regional de Semilleros de Investigación –RREDSI (Manizales).
Campisano, A., and Modica, C. (2015). Appropriate resolution timescale to evaluate water saving and retention potential of rainwater harvesting for toilet flushing in single houses. J. Hydroinform. 17, 331–346. doi: 10.2166/hydro.2015.022
Carson, T., Keeley, M., Marasco, D. E., McGillis, W., and Culligan, P. (2015). Assessing methods for predicting green roof rainfall capture: a comparison between full-scale observations and four hydrologic models. Urban Water J. 14, 589–603. doi: 10.1080/1573062X.2015.1056742
Carter, T., and Jackson, R. C. (2007). Vegetated roofs for stormwater management at multiple spatial scales. Landsc. Urban Plan. 80, 84–94. doi: 10.1016/j.landurbplan.2006.06.005
Chaosakul, T., Koottatep, T., and Irvine, K. (2013). Low impact development modeling to assess localized flood reduction in Thailand. J. Water Manage. Model. R246–18, 337–353. doi: 10.14796/JWMM.R246-18
Cohen, J., Field, R. P., Tafuri, A. N., and Ports, M. A. (2012). Cost comparison of conventional gray combined sewer overflow control infrastructure versus a green/gray combination. J. Sustain. Water Built Environ. 138, 534–540. doi: 10.1061/(ASCE)IR.1943-4774.0000432
Corporación OSSO Universidad EAFIT (2011). Base de Datos de Pérdidas Históricas En Colombia (Periodo 1970-2011). Available online at: http://online.desinventar.org.
Correa, O., and Duque, E. (2015). “Investigación geotécnica en la zona urbana (rellenos, geología, inventario de deslizamientos),” in Simposio Interdisciplinar Sobre Adaptación y Gestión Local Del Riesgo de Desastres (Manizales), 46–51.
Delgado, V., Zambrano, J., and Vélez Upegui, J. J. (2020). Short-Term Temperature variability in Manizales, Colombia. Sometido Para Eval. Hydrol. Rev. Vinculos 17, 17091. doi: 10.14483/2322939X.17091
Delgado, V., Zambrano, J., and Vélez, J. J. (n.d.). The Knowledge of the Spatial-Temporal Rainfall Patterns as a Tool for Storm-Design: Case Study. Manizales
Department of Environmental Conservation (2015). New York Stormwater Management Design Manual. New York, NY: Department of Environmental Conservation.
Ertan, S., and Çelik, R. N. (2021). The assessment of urbanization effect and sustainable drainage solutions on flood hazard by GIS. Sustainability 13, 2293. doi: 10.3390/su13042293
Feng, B., Zhang, Y., and Bourke, R. (2021). Urbanization impacts on flood risks based on urban growth data and coupled flood models. Nat. Haz. 106, 613–627. doi: 10.1007/s11069-020-04480-0
Fletcher, T. D., Shuster, W., Hunt, W. F., Ashley, R., Butler, D., Arthur, S., et al. (2015). SUDS, LID, BMPs, WSUD and more – the evolution and application of terminology surrounding urban drainage. Urban Water J. 12, 1–18. doi: 10.1080/1573062X.2014.916314
Giraldo, M. (2017). Caracterización de Las Propiedades Del Suelo y Su Influencia En La Hidrología de La Cuenca San Luis En La Ciudad de Manizales - Caldas. Manizales: Universidad Nacional de Colombia.
Gómez Valentín, M. (2006). Curso de Hidrología Urbana. Barcelona: Distribuidora Alfambra de Papelería.
Government of the Hong Kong Especial Administrative Region (2013). Stormwater Drainage Manual Planning, Design and Management. Hong Kong: Government of the Hong Kong Especial Administrative Region.
Griffiths, J. A. (2017). “Sustainable urban drainage,” in Encyclopedia of Sustainable Technologies, 403–13. doi: 10.1016/B978-0-12-409548-9.10203-9
Hilten, R. N., Lawrence, T. M., and Tollner, E. W. (2008). Modeling stormwater runoff from green roofs with HYDRUS-1D. J. Hydrol. 358, 288–293. doi: 10.1016/j.jhydrol.2008.06.010
Hirabayashi, Y., Mahendran, R., Koirala, S., Konoshima, L., Yamazaki, D., Watanabe, S., et al. (2013). Global flood risk under climate change. Nat. Clim. Change 3, 816–821. doi: 10.1038/nclimate1911
Holman-Dodds, J. K., Bradley, A. A., and Potter, K. W. (2003). Evaluation of hydrologic benefits of infiltration based urban storm water management. J. Am. Water Resourc. Assoc. 39, 205–215. doi: 10.1111/j.1752-1688.2003.tb01572.x
Hoyos, B., and Felipe, A. (2003). Análisis Del Comportamiento de La Red de Alcantarillado Combinado de La Cuenca de La Quebrada San Luís. Manizales: Universidad Nacional de Colombia.
Hromadka, V. T., and Whitley, R. J. (1989). Stochastic Integral Equations and Rainfall-Runoff Models. Berlin: Springer. doi: 10.1007/978-3-642-49309-6
Huang, Q., Wang, J., Li, M., Fei, M., and Dong, J. (2017). Modeling the influence of urbanization on urban pluvial flooding: a scenario-based case study in Shanghai, China. Nat. Haz. 87, 1035–1055. doi: 10.1007/s11069-017-2808-4
IDEA. (2005). Cuencas experimentales urbanas en Colombia, Caso Manizales. Fase 1. Caracterizaci3n e instrumentación. Manizales, Caldas.
Jha, A. K., Bloch, R., and Lamond, J. (2012). Cities and Flooding: A Guide to Integrated Urban Flood Risk Management for the 21st Century. Washington, DC: World Bank. doi: 10.1596/978-0-8213-8866-2
Johannessen, B. G., Hamouz, V., Gragne, A. S., and Muthanna, T. M. (2019). The transferability of SWMM model parameters between green roofs with similar build-up. J. Hydrol. 569, 816–828. doi: 10.1016/j.jhydrol.2019.01.004
Kasmin, H., Stovin, V. R., and Hathway, E. A. (2010). Towards a generic rainfall-runoff model for green roofs. Water Sci. Technol. 62, 898–905. doi: 10.2166/wst.2010.352
Kellager, R. B. B., and Laughlan, C. S. (2005). Use of SUDS in High Density Developments. Guidance Manual. Report SR 666. Wallingford, CT: HR Wallingford limited.
Krebs, G., Kokkonen, T., Valtanen, M., Koivusalo, H., and Setälä, H. (2013). A high resolution application of a stormwater management model (SWMM) using genetic parameter optimization. Urban Water J. 10, 394–410. doi: 10.1080/1573062X.2012.739631
Krebs, G., Kuoppamäki, K., Kokkonen, T., and Koivusalo, H. (2016). Simulation of green roof test bed runoff. Hydrol. Proc. 30, 250–262. doi: 10.1002/hyp.10605
Locatelli, L., Mark, O., Mikkelsen, P. S., Arnbjerg-Nielsen, K., Jensen, M. B., and Binning, P. J. (2014). Modelling of green roof hydrological performance for urban drainage applications. J. Hydrol. 519, 3237–3248. doi: 10.1016/j.jhydrol.2014.10.030
Metselaar, K. (2012). Water retention and evapotranspiration of green roofs and possible natural vegetation types. Resourc. Conserv. Recycl. 64, 49–55. doi: 10.1016/j.resconrec.2011.12.009
Ministerio de Vivienda Ciudad y Territorio (2017). Resolución 330 de 2017 RAS 2017. Reglamento Técnico Del Sector de Agua Potable y Saneamiento Basico. Bogotá: Ministerio de Vivienda Ciudad y Territorio.
Palla, A., and Gnecco, I. (2015). Hydrologic modeling of low impact development systems at the urban catchment scale. J. Hydrol. 528, 361–368. doi: 10.1016/j.jhydrol.2015.06.050
Pareja, J. F., and Osorio, J. I. (1997). Estudio Hidrologico de Caldas, Revision y Actualizacion de Las Curvas de Intensidad - Duracion - Frecuencia. Manizales: Universidad Nacional de Colombia; Sede Manizales; Facultad de Ingenieria y Arquitectura.
Rey Valencia, R., and Marcela, D. (2019). Propuesta de Sistema de Drenaje Urbano Sostenible Para Cuencas de Montaña Con Alta Pendiente. Manizales: Universidad Nacional de Colombia.
Rincón, D., Velez, J., and Chang, P. (2015). “Spatio-Temporal description of the rainfall in the andean city of Manizales (Colombia) for storm design,” in E-Proceedings of the 36th IAHR World Congress: Deltas of the Future and What Happens Upstream (The Hague), 5437–5441.
Rosa, D. J., Clausen, J. C., and Dietz, M. E. (2015). Calibration and verification of SWMM for low impact development. J. Am. Water Resourc. Assoc. 51, 746–757. doi: 10.1111/jawr.12272
Rossman, L. A. (2015). Storm Water Management Model User's Manual, Version 5.1. United States Environment Protection Agency. Cincinnati: EPA.
Rossman, L. A. (2016). Storm Water Management Model SWMM. Cincinnati: National Risk Management Research Laboratory, U.S. Environmental Protection Agency EPA.
Rossman, L. A., and Huber, W. C. (2016). Storm Water Management Model Reference Manual Volume III–Water Quality. Washington, DC: US Environmental Protection Agency, Office of Research and Development.
Saldarriaga, J., Salcedo, C., Solarte, L., Pulgarín, L., Rivera, M. L., Camacho, M., et al. (2020). Reducing flood risk in changing environments: optimal location and sizing of stormwater tanks considering climate change. Water 12, 2491. doi: 10.3390/w12092491
Sample, D. J., and Liu, J. (2014). Optimizing rainwater harvesting systems for the dual purposes of water supply and runoff capture. J. Clean. Product. 75, 174–194. doi: 10.1016/j.jclepro.2014.03.075
Steffen, J., Jensen, M., Pomeroy, C. A., and Burian, S. J. (2013). Water supply and stormwater management benefits of residential rainwater harvesting in U.S. cities. J. Am. Water Resourc. Assoc. 49, 810–824. doi: 10.1111/jawr.12038
Stephens, K. A., Graham, P., and Reid, D. (2002). Stormwater Planning: A Guidebook for British Columbia. Vancouver, CA: British Columbia Ministry of Water, Land and Air Protection.
Stovin, V., Vesuviano, G., and Kasmin, H. (2012). The hydrological performance of a green roof test bed under UK climatic conditions. J. Hydrol. 414–415, 148–61. doi: 10.1016/j.jhydrol.2011.10.022
Toronto and Region Conservation Authority. (2010). Low Impact Development Stormwater BMP Fact Sheets. Toronto, ON: Toronto and Region Conservation Authority.
Tsihrintzis, A. V., and Hamid, R. (2001). Runoff quality prediction from small urban catchments using SWMM. Hydrol. Proc. 12, 311–29. doi: 10.1002/(SICI)1099-1085(199802)12:2<311::AID-HYP579>3.0.CO;2-R
UN CORPOCALDAS. (2014). CDIAC—Centro de Datos e Indicadores Ambientales de Caldas 2014. Available online at: http://cdiac.manizales.unal.edu.co/
United States Environmental Protection Agency (2005). National Management Measures to Control Nonpoint Source Pollution from Urban Areas, EPA Document, EPA-841-B-05-004. Cincinnati: United States Environmental Protection Agency.
Upegui, V., Julián, J., Alzate, M. O., Méndez, N. D., and Aristizabal Zuluaga, B. H. (2015). Entendimiento de Fenómenos Ambientales Mediante Análisis de Datos. Manizales: Universidad Nacional de Colombia Sede Manizales.
USDA-ARS (2008). Sitio Web STEWARDS (Sustaining The Earth's Watersheds—Agricultural Research Database System). Washington: USDA-ARS.
Vélez Upegui, V., Julián, J., and Gutiérrez, A. B. (2011). Estimación del tiempo de concentración y tiempo de rezago en la cuenca experimental urbana de la quebrada san luis, manizales (estimation of the time of concentration and the lag time at San Luis Creek Basin, Manizales). Dyna 165, 58–71.
Villalonga, V. M. (2016). Rehabilitación de Redes de Alcantarillado Mediante Técnicas LID, Usando SWMM5 En Un Caso Real. Barcelona: Universitat Politècnica de Catalunya.
Villarreal, E. L. (2007). Runoff detention effect of a sedum green-roof. Hydrol. Res. 38, 99–105. doi: 10.2166/nh.2007.031
Ward, S., Memon, F. A., and Butler, D. (2012). Performance of a large building rainwater harvesting system. Water Res. 46, 5127–5134. doi: 10.1016/j.watres.2012.06.043
Warsta, L., Niemi, T. J., Taka, M., Krebs, G., Haahti, K., Koivusalo, H., et al. (2017). Development and application of an automated subcatchment generator for SWMM using open data. Urban Water J. 14, 954–963. doi: 10.1080/1573062X.2017.1325496
Keywords: tropical Andean city, storm floods, rainfall intensity, flood risk, urban hydrology
Citation: Zambrano Nájera J and Rey DM (2022) Assessment of Storm Floods in a Small Tropical Andean Basins. Front. Clim. 4:760894. doi: 10.3389/fclim.2022.760894
Received: 19 August 2021; Accepted: 06 June 2022;
Published: 08 August 2022.
Edited by:
Sirkku Juhola, University of Helsinki, FinlandReviewed by:
Mariana Gutierres Arteiro Da Paz, National Institute of Space Research (INPE), BrazilCopyright © 2022 Zambrano Nájera and Rey. This is an open-access article distributed under the terms of the Creative Commons Attribution License (CC BY). The use, distribution or reproduction in other forums is permitted, provided the original author(s) and the copyright owner(s) are credited and that the original publication in this journal is cited, in accordance with accepted academic practice. No use, distribution or reproduction is permitted which does not comply with these terms.
*Correspondence: Jeannette Zambrano Nájera, amR6YW1icmFub25hQHVuYWwuZWR1LmNv
†These authors have contributed equally to this work
Disclaimer: All claims expressed in this article are solely those of the authors and do not necessarily represent those of their affiliated organizations, or those of the publisher, the editors and the reviewers. Any product that may be evaluated in this article or claim that may be made by its manufacturer is not guaranteed or endorsed by the publisher.
Research integrity at Frontiers
Learn more about the work of our research integrity team to safeguard the quality of each article we publish.