- 1Department of Earth and Planetary Sciences, Kyushu University, Fukuoka, Japan
- 2Atmosphere-Ocean Research Institute, The University of Tokyo, Kashiwa, Japan
Introduction: A subdecadal (i.e. , three-year running mean) variation over the tropical Pacific is very distinctively observed in the 2000s.
Results and methods: Here, we have demonstrated that sea surface temperature (SST) anomalies in the tropical Atlantic contribute to forming high ocean-temperature anomalies in the tropical Pacific in the early 2000s by performing partial data assimilation of a global climate model. Low SSTs over the equatorial Atlantic change the Walker circulation, and the associated weakening of the Pacific trade winds raises the equatorial SST on subdecadal timescales. At the same time, a high SST anomaly is also generated in the off-equatorial North Pacific through deepening of the upper ocean thermocline due to an accompanying anticyclonic surface wind anomaly aloft. While the subtropical North Atlantic SSTs may help the subdecadal warming in the equatorial Pacific, the resultant SST anomalies show a one-year delay in the phase transition and are modestly accompanied by ocean thermocline deepening.
Discussion: It roughly follows the IMRaD format.
Introduction
The tropical Pacific climate is an energetic driver of global climate variability and change (Meehl et al., 2013, 2016a; e.g., Kosaka and Xie, 2013; Fyfe et al., 2016). For example, the sea surface temperature (SST) changes over the tropical Pacific, as a part of the Interdecadal Pacific Oscillation (IPO) (Trenberth and Hurrell, 1994), predominantly modulate the global warming tendency (e.g., Kosaka and Xie, 2013). In particular, the tropical Pacific SST variability on subdecadal to decadal timescales, representing a broad peak in power spectra (Newman, 2007; Sun et al., 2017), plays an important role in near-term (i.e., decadal) climate changes (Meehl et al., 2016b). As of today, however, near-term climate prediction usually has limited degrees of predictability for the tropical Pacific (e.g., Doblas-Reyes et al., 2011, 2013; Kim et al., 2012; Mochizuki et al., 2012; Smith et al., 2018, 2019, 2020) and for the slowdown of the global warming tendency in the 2000s (Easterling and Wehner, 2009). Given that the present predictive skill is less than a decade ahead in near-term climate prediction, climate variability on subdecadal timescales should attract much attention in the areas of applied studies, such as those on fisheries, agriculture, marine ecosystems, and natural disasters.
When focusing on subdecadal timescales, recently, Mochizuki and Watanabe (2019) found subdecadal climate variability that was distinctive in the 2000s over the tropical Pacific and was rarely observed in preceding decades. The subdecadal variation has usually been observed as a periodic fluctuation in the twentieth century, showing an ocean heat content (OHC) anomaly with an eastward evolution along the equator and a subsequent slow westward propagation in the off-equatorial North Pacific (Capotondi and Alexander, 2001; Tourre et al., 2001, 2005). As a result, the spatial pattern of SSTs is similar to the so-called eastern Pacific El Niño Southern Oscillation (EP-ENSO) events. On the other hand, this process may not sufficiently explain the distinctive fluctuations observed in the 2000s, which show a similar spatial pattern to that of the so-called central Pacific ENSO (CP-ENSO) (e.g., Ashok et al., 2007; Kug et al., 2009). In the early 2000s, for example, a high temperature anomaly in the upper ocean along the equator was rapidly formed with a deep ocean thermocline around the international dateline, and it maintained its position rather than propagating further eastward (Mochizuki and Watanabe, 2019). The noticeably high OHC (and SST) can also be observed in the off-equatorial area together with the high OHC (and SST) along the equator in the 2000s. This is not limited to an issue of physical mechanism but also can contribute to an issue of climate prediction by modulating the hindcast skill of the tropical and global climates on subdecadal timescales. Whereas, the dominant subdecadal variations in the tropical Pacific represent large dependency on the periods as above, for example, our global climate model used for the decadal prediction experiments in the Coupled Model Intercomparison Project Phase 5 (CMIP5) always simulates periodic fluctuations in the tropical OHC as the dominant subdecadal variability. Consequently, our CMIP5 decadal hindcasts fail to simulate the distinctive fluctuations even several years in advance particularly in the 2000s rather than in the twentieth century century (Mochizuki and Watanabe, 2019).
In addition to possible feedback in the Pacific climate system, possible remote influences from other basins attract much attention and may contribute to surface wind changes in the tropical Pacific (Cai et al., 2019; Wang, 2019). The equatorial and subtropical North Atlantic Oceans are two major areas in which SST changes largely impact the Pacific Ocean. On seasonal to interannual timescales, for example, the SST anomaly in the equatorial Atlantic where the Atlantic Niño dominates (Zebiak, 1993) affects the Pacific ENSO through changes in the Walker circulation (Wang, 2006; Rodríguez-Fonseca et al., 2009; Ding et al., 2012; Martín-Rey et al., 2014; Polo et al., 2015; Luo et al., 2017), even gives beneficial information for ENSO prediction (Martín-Rey et al., 2015). A robust influence of the Pacific ENSO is found in the subtropical North Atlantic and, in turn, thermal feedback tends to induce the CP-ENSO (Ham et al., 2013; Ding et al., 2017; Wang et al., 2017). The interbasin effect becomes stronger after the 1990s (Martín-Rey et al., 2014), possibly in relation to the phase of the Atlantic Multidecadal Oscillation (AMO) (Martín-Rey et al., 2018). Note that the seasonal cycle in climatology plays an important role in the interbasin interaction between the equatorial Pacific and Atlantic Oceans on seasonal to interannual timescales (e.g., Ham et al., 2013). Ham et al. (2013) demonstrated that the negative SST anomaly in the subtropical North Atlantic in the boreal spring induces the positive SST anomaly in the tropical Pacific in the following autumn and winter, through the changes of the Inter-Tropical Convergence Zones (ITCZs) of the Atlantic and Pacific. On decadal to interdecadal timescales, on the other hand, recent studies have indicated that the tropical Pacific trade winds, SSTs, and OHC are partly affected by the equatorial Atlantic climate on decadal to interdecadal timescales (McGregor et al., 2014; e.g., England et al., 2014; Chikamoto et al., 2015, 2016; Kucharski et al., 2016; Li et al., 2016). The tropical Pacific and Atlantic Oceans show an interbasin contrast formed by simultaneous changes in SSTs, coined Trans-Basin Variability (TBV) (McGregor et al., 2014; Chikamoto et al., 2015). Several studies have indicated that the subtropical North Atlantic SST anomalies, as part of the AMO variability, are also influential factors on the Pacific Ocean (Yu et al., 2015; Ruprich-Robert et al., 2017, 2021; Levine et al., 2018; Wu et al., 2019).
Here, we clarify the physical processes that result in the subdecadal climate changes distinctively observed over the tropical Pacific in the 2000s, particularly focusing on the contribution of the interbasin effects from the Atlantic Ocean. Special focus is also given to subdecadal timescales and two types of potential impacts from the Atlantic Ocean. As described above, the interbasin connection has thus far been primarily discussed on seasonal to interannual timescales and on decadal to interdecadal timescales to understand the major contributor to the ENSO impact and modulation mechanism of climate change, respectively. In the broad review by Cai et al. (2019), they indicated that we have a limited ability to clearly define interbasin interactions across the full range of interannual to decadal and longer timescales. Our analysis will contribute to filling a gap in our understanding of Atlantic impacts on the tropical Pacific Ocean by improving our knowledge of subdecadal variability.
In this study, we therefore perform two sets of partial data assimilation experiments using an atmosphere–ocean coupled climate model and ocean observations in specific areas of interest. Based on our present understanding of the interbasin connections, we focus on the potential impacts of the climate conditions in the equatorial and subtropical North Atlantic Ocean. We compare the results of these sets of ensemble simulations and discuss the relative importance of the two major contributors to stimulating interbasin teleconnections on subdecadal timescales.
Model experiments
The framework of data assimilation is the same as that used in Chikamoto et al. (2015, 2016, 2020), Mochizuki et al. (2016), and Johnson et al. (2018, 2020). We assimilate ocean temperature and salinity anomalies derived from a gridded objective analysis (Ishii and Kimoto, 2009) as observations using version 3.2 of the Model for Interdisciplinary Research on Climate (MIROC). The atmospheric component is a T42 spectral model with 20 levels, and the resolution of the ocean component is 1.4° in longitude and 0.56–1.4° in latitude with 44 vertical levels. We perform two sets of 10 ensembles of partial data assimilation during 1945–2010 using ocean anomalies over specific areas of the Atlantic Ocean: the equatorial Atlantic (10°S−10°N) in one experiment (referred to as EA) and the subtropical North Atlantic (north of 10 °N, except for the areas where sea ice exists) in the other experiment (referred to as NA). As described by Chikamoto et al. (2015), these partial data assimilation experiments simulate not only the atmospheric response but also the resultant atmosphere–ocean interactions due to SST anomalies assimilated over the Atlantic Ocean. As a good proxy for the observations, we also analyze the results of the data assimilation experiment using ocean anomalies over the entire global ocean (referred to as GL), which was used to define the initial conditions in the decadal climate prediction (Mochizuki et al., 2010).
Subdecadal SST variability in the tropical Pacific Ocean
As we focus on the subdecadal variability on longer timescales than commonly observed ENSO timescales, we analyze three-year running mean anomalies relative to the linear trend at each grid point during 1961–2010, as in Mochizuki and Watanabe (2019). We analyze the data assimilation results after 1961 with following the protocol of the decadal climate prediction, because the climate model may usually require a little time to fit to the observed climate sufficiently. Figure 1 shows the subdecadal SST variability over the Pacific and Atlantic Oceans. One-sided Student's t-test is applied to reject null hypothesis indicating that the ensemble mean value is positive or negative. In the GL simulation (Figure 1A), which is a good proxy for the observations, the spatial patterns of the SST anomalies over the Pacific at the beginning of the Twenty first Century(i.e., during 1999–2001) are similar to those observed when the IPO is in negative phases. The North Atlantic SST anomalies show a tripolar pattern similar to those usually observed with the North Atlantic Oscillation (NAO). In the equatorial Pacific, the low SST anomaly rapidly decayed, and warm water was generated around the international dateline in 2001–2003. This warm anomaly is slowly enhanced, showing a CP-ENSO–like spatial pattern in the Pacific Ocean. In addition, the Atlantic SST anomaly that forms the positive AMO-like pattern follows with a lag of a few years (e.g., 2003–2005). Overall, the SST anomalies in 2003–2005 represent a phase reversal of the subdecadal Pacific variability when compared to those in 1999–2001. Note that, for convenience, we describe the spatial patterns of the subdecadal SST variability using a suffix (i.e., -like) as above. The zonally elongated Pacific SST anomaly along the equator and the tripolar SST anomaly over the North Atlantic, referred to as the CP-ENSO-like and the North Atlantic tripolar patterns respectively, represent subdecadal variability rather than interannual or multidecadal variability. Mochizuki and Watanabe (2019) also showed a CP-ENSO-like SST pattern in the observed subdecadal variability over the tropical Pacific. Martin et al. (2019) found subdecadal variability showing an SST tripolar signal over the North Atlantic in climate models, which sometimes corresponds to the above AMO-like pattern.
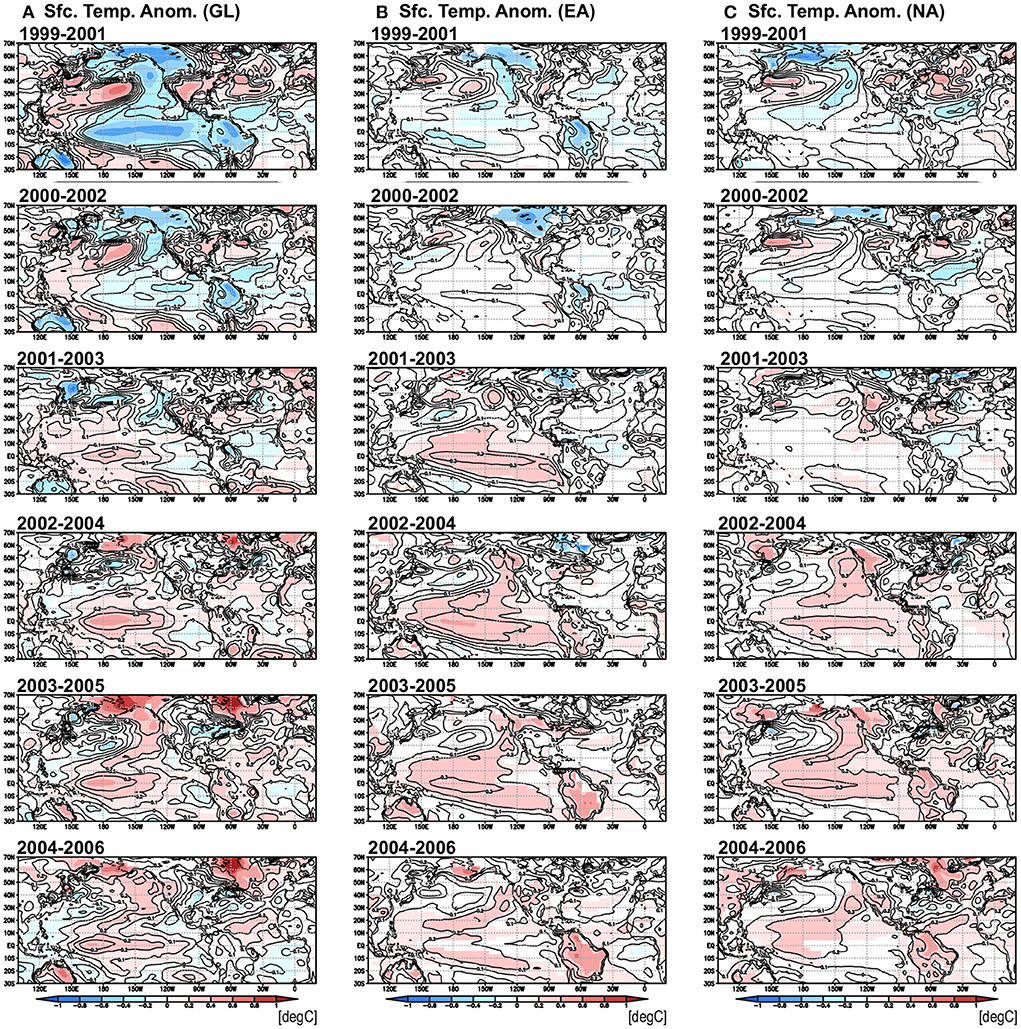
Figure 1. The three-year running mean anomalies of sea and land surface temperatures in the ensemble means of the (A) GL, (B) EA, and (C) NA simulations in the first half of the 2000s. Shaded areas represent significant anomalies at the 95% confidence level.
Even when we assimilate the ocean temperature and salinity only in the equatorial Atlantic (i.e., the EA simulation), the model reproduces strong warming of the equatorial Pacific after the period 2001–2003 (Figure 1B). The low SST anomaly in the tropical Atlantic, which is not accompanied by significant SST anomalies in the mid- and high-latitudes of the Atlantic Ocean, leads the tropical Pacific warming by a couple of years (e.g., 2000–2002). On the other hand, when we assimilate the ocean temperature and salinity only in the subtropical North Atlantic (i.e., the NA simulation), the model simulates a negative-positive-negative tripolar pattern of the North Atlantic SST anomalies up to the period 2001–2003 (Figure 1C) but hardly simulates the low SSTs in the equatorial Atlantic. The NA simulation also recreates the rapid warming of the tropical Pacific in 2002–2004, while the warming shows a one-year delay in the tropical Pacific relative to the observational proxy (i.e., the GL simulation). In reverse, the high SST anomalies in the equatorial and subtropical Atlantic in 2003-2005 are accompanied by the weakening of the high SST anomalies over the equatorial Pacific in 2004-2006 in the EA and NA simulations. Note that positive SST anomalies in the equatorial Pacific are surely weakened probably as a part of the subdecadal fluctuation but the phase is not reversed to representing negative values, mainly due to a basic framework of partial data assimilation. The assimilations of only specific areas (i.e., the EA and NA simulations) may not sufficiently control the long-term warming tendency globally, different from the GL simulation. As the global warming tendency is not observed as a rigidly constant rate, the slightly large warming tendency in the latter period can represent a positive SST anomaly relative to a long-term linear trend. The high climate sensitivity of the model leads to representing biased SSTs in the EA and NA simulations (i.e., slightly higher SST than in the GL simulation in the late 2000s) even when removing the linear trend before analyzing the data.
As indicated in Mochizuki and Watanabe (2019), the SST anomalies in the equatorial and off-equatorial Pacific correspond to the OHC rising. For example, during the period 2002–2004, when the equatorial Pacific SST anomaly is observed to be the strongest (Figure 1A), the high SST anomalies around the international dateline are found together with the deep ocean thermocline in the central and eastern equatorial Pacific (Figure 2D). At the same time, the high SST anomalies in the subtropical North Pacific are also accompanied by a high OHC with a deep ocean thermocline (Figure 2A).
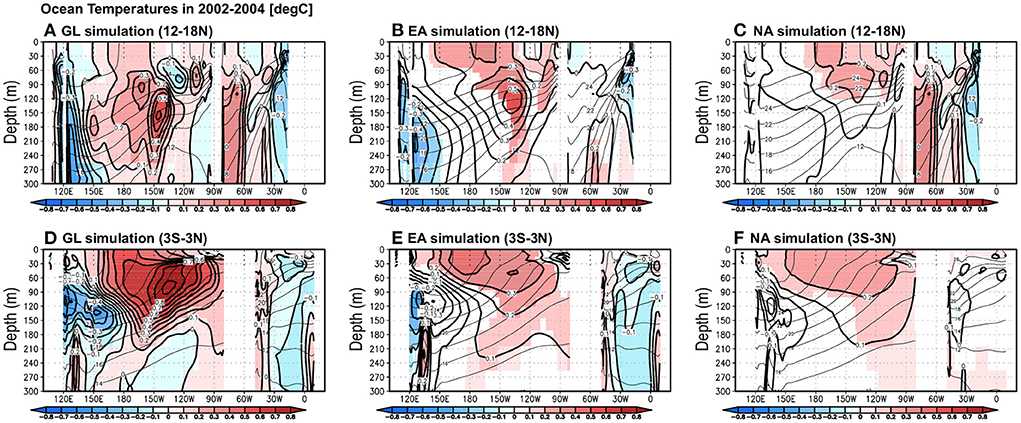
Figure 2. (Upper panels) Longitude-depth sections of ocean temperatures along 15°N in the ensemble means of the (A) GL, (B) EA, and (C) NA simulations. Plotted values are the three-year running mean anomalies (thick contours) and values (thin contours) averaged over 2002–2004. Shaded areas represent significant anomalies at the 95% confidence level (D–F). The same as in the upper panels, except for the ocean temperatures being plotted along the equator.
Both the EA and NA simulations strongly show a warm temperature anomaly at the sea surface rather than at the level of the thermocline along the equator (Figures 2E,F). Nevertheless, the EA simulation also indicates a small but significant warm temperature at the level of the thermocline in the central and eastern Pacific, suggesting a deepening of the ocean thermocline. The observed high OHC with a deep thermocline during 2002–2004 is due to the anomalous heat input from the subtropical areas, in addition to the eastward movement of the deep thermocline anomaly found around the western edge of the equatorial Pacific at the beginning of the twenty first century (Mochizuki and Watanabe, 2019). The EA simulation properly simulates the changes in the surface winds working to enhance the anomalous heat input from the subtropical areas, as described below. On the other hand, the formation of the deep thermocline anomaly in the western equatorial Pacific at the beginning of the twenty first century, prior to the equatorial Pacific warming, may not be necessarily simulated in the EA and NA simulations, since only the Atlantic Ocean state is directly controlled by the assimilation. The high temperature anomaly in the subtropics is also found to be strongest at the depth of the thermocline in the EA simulation (Figure 2B) as observed, while that in the NA simulation is concentrated at the sea surface (Figure 2C).
Changes in the Pacific and Atlantic atmospheres
The deepening of the ocean thermocline in both the equatorial and subtropical North Pacific (Figure 2) is due to changes in the surface winds aloft. During the period 2000–2002, when the equatorial Atlantic SST is below normal (Figure 1B), the Atlantic SST in the EA simulation stimulates significant changes in the Walker circulation along the equator over the central and eastern Pacific (Figure 3A). In particular, the anomalous westward winds at the sea surface should deepen the ocean thermocline along the equator (Figure 2E). These changes in the Walker circulation play a similar role to those on decadal or longer timescales (Chikamoto et al., 2016), while the subdecadal anomalies in Figures 1A,B clearly show the lagged relationship between the tropical Pacific and Atlantic SSTs; this is in contrast to the practically simultaneous SST contrast in the two basins referred to as TBV (McGregor et al., 2014; Chikamoto et al., 2015). Once the equatorial Pacific SST rises (e.g., 2001–2003), the zonal winds show anomalous divergence and convergence at the top and bottom of the troposphere, respectively, and the Walker circulation is significantly modified over the entire Pacific and Atlantic basins (Figure 3C). In addition to the changes along the equator, at the same time, the anomalous westward wind at the sea surface contributes to forming an anticyclonic wind curl anomaly in the subtropics of the central North Pacific (Figures 4A,B), which may work to deepen the ocean thermocline in the subtropics (Figure 2B) as a dynamical ocean response (Mochizuki and Watanabe, 2019).
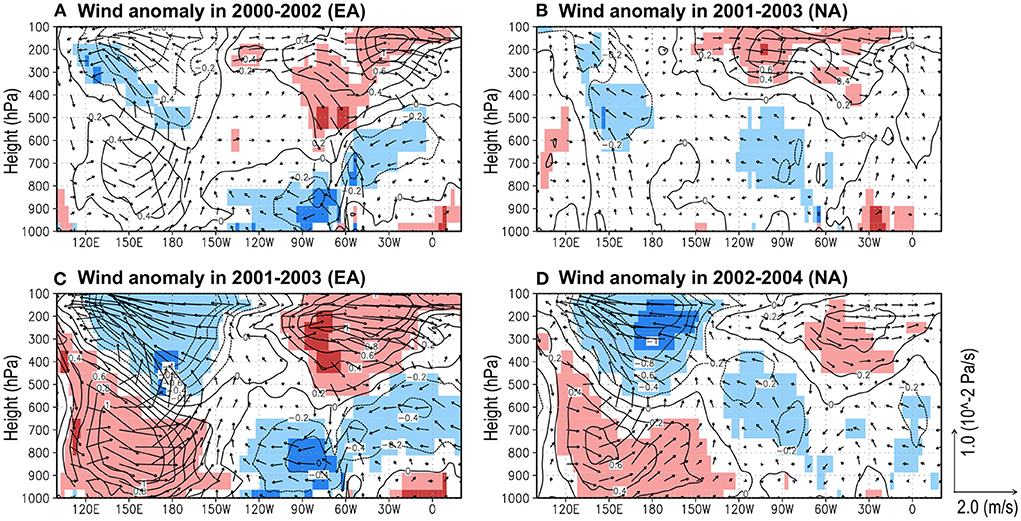
Figure 3. (Upper panels) Longitude-height sections of the zonal wind speed (contours) and the zonal and vertical winds (arrows) in the ensemble means of the (A) EA and (B) NA simulations. Plotted values are the three-year running mean anomalies just before the equatorial Pacific warming (i.e., 2000–2002 in the EA simulation and 2001–2003 in the NA simulation). Dark and light shades represent areas where the positive (red) and negative (blue) anomalies in the zonal wind speed are significant at the 99% and 90% confidence levels, respectively. (C,D) The same as in the upper panels, except for the three-year running mean anomalies being just after the equatorial Pacific warming (i.e., 2001–2003 in the EA simulation and 2002–2004 in the NA simulation).
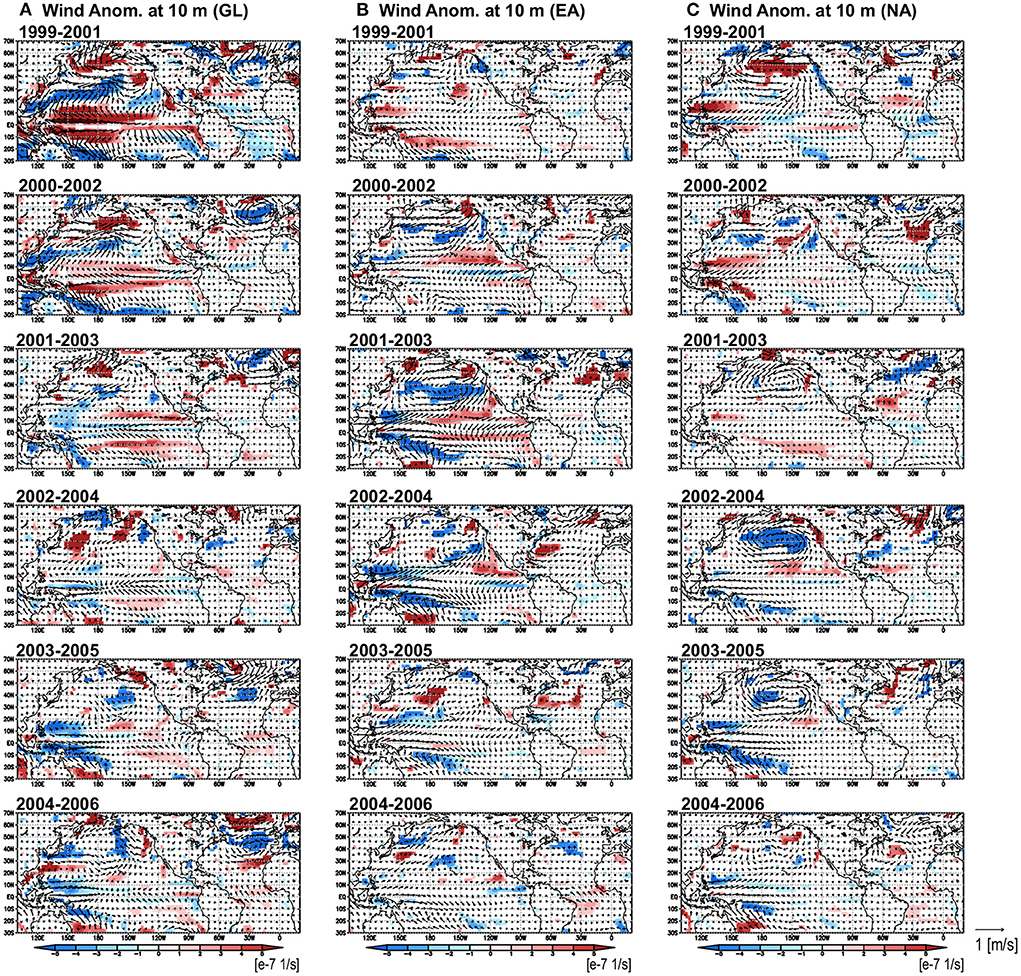
Figure 4. (A–C) The same as in Figure 1, except for being representative of the surface wind curl (shades) and surface zonal and meridional winds (arrows). The shaded areas are the only areas in which the surface wind curl anomalies are significant at the 90% confidence level. Positive and negative values of the surface wind curl values correspond to anticyclonic and cyclonic anomalies, respectively.
On the other hand, the NA simulation shows the low SST anomaly strongly in the subtropical Atlantic, as part of the negative-positive-negative tripolar pattern, during the period 2001–2003 just before the equatorial Pacific warming (Figure 1C). At this period, the Atlantic low SST hardly induces a significant anomaly in the surface wind curl over the Pacific Ocean (Figure 4C) nor significant changes in the zonal winds at the sea surface as a part of the Walker circulation along the equator (Figure 3B). Even after the equatorial Pacific warming (i.e., 2002–2004), the significant Walker circulation change is limited to the western and central Pacific Ocean (Figure 3D). Ham et al. (2013) indicated that a negative SST anomaly in the subtropical North Atlantic can work as a trigger of CP-ENSO on seasonal timescales. A similar mechanism in the seasonal evolution can modestly work for realizing the subdecadal SST anomalies in the NA simulation (Figure 5). When examining the seasonal mean states averaged over the period 2001–2003, for example, the low SST in the subtropical North Atlantic (Figure 5A) leads to an inactive Atlantic Inter-Tropical Convergence Zone (ITCZ) in the boreal spring (Figure 5B). The surface wind response over the northeastern subtropical Pacific represents an anticyclonic anomaly in the boreal summer (Figure 5D), suggesting an SST increase due to the southwesterly winds to the west (Figure 5C). High SSTs in the subtropics can logically result in enhancing the Pacific ITCZ in the boreal autumn (Figure 5E), but the atmospheric change is limited to an insignificant level. A possible weakening of the Pacific trade winds, therefore, can realize the high SSTs in the subtropical North Pacific (Figure 5F), as a thermal rather than dynamical response of the upper ocean (Figure 2F).
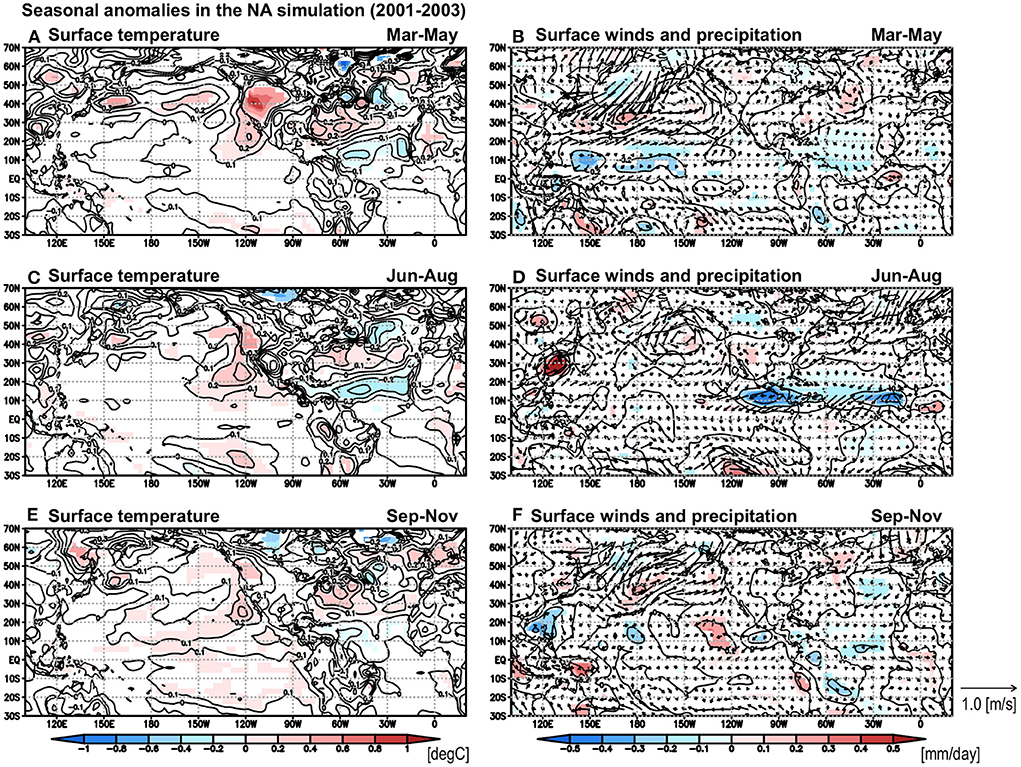
Figure 5. (Upper panels) Ensemble means of the (A) sea and land surface temperatures and (B) precipitation (contours) and zonal and meridional winds at the surface (arrows) in the NA simulation. Plotted values are the three-month running mean anomalies over March–May in 2001–2003. Shaded areas in panels (A,B) represent significant temperature anomalies at the 95% confidence level and significant precipitation anomalies at the 90% confidence level, respectively (Middle and lower panels). The same as in the upper panels, except for the three-month running mean anomalies being over June–August (C,D) and September–November (E,F).
Summary and discussion
We have demonstrated that SST anomalies in the equatorial and subtropical North Atlantic Ocean contributed to forming high ocean-temperature anomalies in the tropical Pacific in the early 2000s. We have gained knowledge of Atlantic impacts on the Pacific by validating the effects on subdecadal timescales in addition to seasonal to interannual and decadal to interdecadal timescales. The equatorial Atlantic SST predominantly works to generate the lagged SST contrast between the Atlantic and Pacific basins by changing the Walker circulation. This effect is similar to that on longer timescales, while the significant influence is not limited to the equator. The Atlantic SST can also directly change the surface winds in the subtropical North Pacific and modify the ocean thermocline. The subtropical North Atlantic SST may help subdecadal warming through similar changes working as a seasonal trigger of CP-ENSO.
In the partial data assimilation experiments, we specified the observed time-evolution of the ocean anomalies rather than an idealized pattern of SSTs that represents internal variability (e.g., Ruprich-Robert et al., 2017). To help our understanding of the observed anomalies used for the assimilations, we discuss the time series of the Atlantic SSTs simulated by the GL, EA and NA experiments. The subdecadal SST variations of the observational proxy (i.e., the GL simulation) are not necessarily synchronized in the equatorial and subtropical North Atlantic (red lines in Figure 6). Even when assimilating ocean observations in one area (i.e., the NA and EA simulations), the subdecadal SST variation is not reproduced well in other areas in which the ocean conditions are not directly assimilated. The time series of the subtropical North Atlantic SSTs in the EA simulation indicates long-term warming rather than the subdecadal oscillations found in the GL and NA simulations (Figure 6A). Similarly, the equatorial SSTs in the NA simulation hardly show the subdecadal fluctuation in the GL and EA simulations (Figure 6B). An additional set of 10 partial data assimilation ensembles using the ocean temperature and salinity only in the equatorial Pacific (EP; 10°S−10°N) should provide insight to understand the interbasin interaction between the Pacific and Atlantic Oceans on subdecadal timescales. The EP simulation simulates the subdecadal SST variations in the subtropical North Atlantic in good agreement with the GL and NA simulations (Figure 6A) (c.f., Martín-Rey et al., 2018), suggesting potential impact of the equatorial Pacific. Thus, the subtropical North Atlantic SST can work as an Atlantic feedback to the Pacific climate rather than being a simple one-way forcing. On the other hand, the equatorial Atlantic SST is not primarily controlled by the equatorial Pacific Ocean (Figure 6B) (c.f., Chang et al., 2006). Another factor likely contributes to realizing the subdecadal variation of the equatorial Atlantic SSTs, which play an important role in realizing the Atlantic impacts on the Pacific in addition to the slight warming tendency.
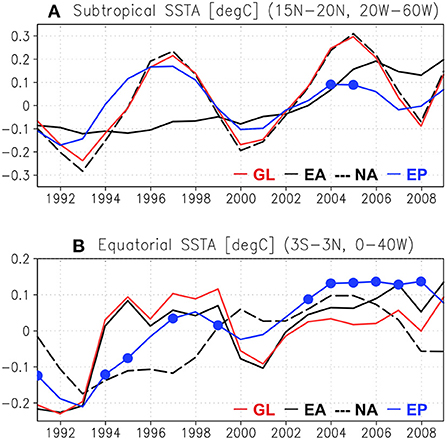
Figure 6. (A) The three-year running mean SST anomalies averaged over 15°N−20°N, 20°W−60°W. Red, solid black, dashed black, and blue lines represent the GL, EA, NA, and EP simulations, respectively. Blue circles represent the EP-derived SST anomalies that were significantly different from those of the GL simulation at the 95% confidence level. (B) The same as in panel (A), except for the SST anomalies being averaged over 3°S−3°N, 0–40°W.
We have validated the Atlantic impact on the Pacific climate through the Walker circulation changes, which work on both subdecadal timescales beyond the commonly observed ENSO variability. Note that the above process predominantly forms the subdecadal anomalies as the Pacific response only in the 2000s (Mochizuki and Watanabe, 2019). Since our analysis focuses on the 2000s, when the Pacific subdecadal variability is distinctively observed, the present analysis should be regarded as a case study rather than a discussion of a climate mode that is always observed as a dominant climate variability. To further explore the dependency on the period, we also discuss the time series of the tropical Pacific SSTs simulated by the GL, EA and NA experiments. Wavelet powers of the NINO3.4 (120°W−170°W, 5°S−5°N) SST show large peaks on subdedadal timescales in the EA and NA simulations (Figures 7B,C), suggesting that the three-year running mean anomalies can well represent the Atlantic contributions. In addition, it should be noted that these strong wavelet powers are simulated not only in the 2000s but also in the preceding decades. In other words, the Atlantic impacts potentially work also in other periods. On the other hand, the observational proxy shows two peaks in the wavelet powers with periods of 2–5 years and over 10 years in the preceding decades (Figure 7A). In the 2000s, the wavelet power related to the ENSO shows a broader peak even spreading over the subdecadal timescales (Figure 7A), and as a result the dominant timescale of the observed fluctuations considerably overlaps with that of the Atlantic impacts. Note that we can expect that the CP-ENSO events observed in 2002/2003 and 2004/2005 may be detected as a positive SST anomaly in the tropical Pacific in the three-year running mean field in a mathematical sense. In this regard, the subdecadal variability can be regarded as a statistical fluctuation, while the broad peak of wavelet powers suggests the Atlantic contributions to exciting subdecadal fluctuation in the tropical Pacific climate. The subdecadal variability discussed using the three-year running mean anomalies in this study may illustrate the low-frequency part and subdecadal modulation of the observed ENSO variability in the tropical Pacific rather than a specific mode independent on the ENSO. A close examination suggests that the observational proxy and the Atlantic contributions represent the slight differences in the spatial patterns of the Pacific SST anomalies in Figure 1. We can speculate that it can be due to the slight differences of the peaks of the wavelet power spectrum in the 2000s. The Atlantic impacts on the tropical Pacific on subdecadal timescales are sometimes simulated stronger over the off-equatorial region than the equatorial region. As in the case for IPO, in fact, the low-frequency variation in the Pacific usually shows SST anomaly widely spreading over the off-equator rather than narrowly concentrated onto the equator. Nevertheless, the changes in both the atmosphere and ocean along the equator (Figures 2, 3) verify the Atlantic impacts on the equatorial Pacific.
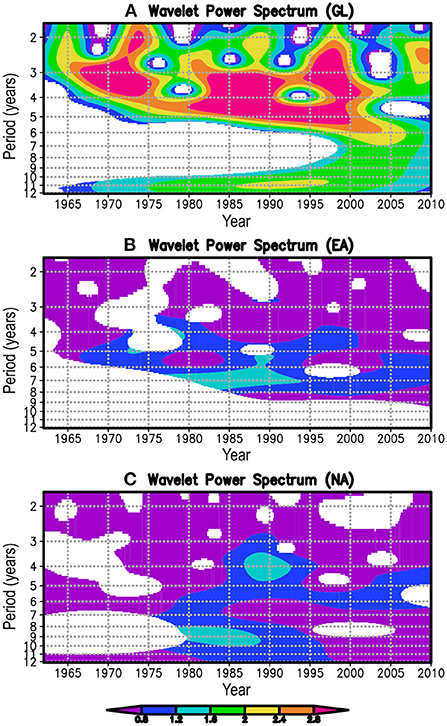
Figure 7. (A) Wavelet powers of the ensemble mean anomalies (rather than a single member anomalies) of the monthly mean NINO3.4 SST in the GL simulation. Shaded areas represent significant powers at the 95% confidence level relative to the powers of the one-order auto-regressive (AR1) model simulations. The confidence limit is based on the p-values defined by using 1000 sets of 10-member ensembles derived from each of the GL simulation and the AR1 simulations. These sets of ensembles of the GL simulation are obtained by random resampling with replacement using 10-member runs, and those of the AR1 simulations are due to random sampling using 10000-member AR1 runs. Panels (B,C) are the same as in panel (A), except for the EA and NA simulations, respectively.
The observed subdecadal variations represent noticeable dependency on the periods, and further studies to clarify the determining factors for selecting the dominant subdecadal variations in a specific period are now underway. We can speculate that changes in background states, which can modulate the ENSO events in the Pacific and the potential contribution of the tropical and subtropical Atlantic, may be a strong candidate for selecting the dominant variations in the actual climate on subdecadal timescales. Over the Atlantic Ocean, for example, Martín-Rey et al. (2018) put forward the role of the Atlantic background state in the emergence of diverse Atlantic Equatorial Modes, than in some cases can be forced by a previous ENSO added to a receptive tropical Atlantic mean state. CP-type ENSO events may be more likely under global warming conditions (Yeh et al., 2009). In addition to the warming trend, the observed AMO represents a positive peak in the mid-2000s (Power et al., 2013; Xu et al., 2017). A background state that effectively modulates the Atlantic impacts on the Pacific subdecadal variability is not clearly known; thus, the dominant process of interbasin connections in the future is still a debatable issue.
Data availability statement
The raw data supporting the conclusions of this article will be made available by the authors, without undue reservation.
Author contributions
TM decided research direction, performed experiments, analyzed data, made figures, and wrote the manuscript. MW led the research project. All authors contributed to the article and approved the submitted version.
Funding
This work is supported by JSPS KAKENHI Grant Numbers JP19H05703 and JP17K05661 and by MEXT programs JPMXD0717935457 and JPMXD0722680395.
Acknowledgments
The authors wish to thank Prof. M. Kimoto, Drs. T. Nishimura, M. Ishii, H. Tatebe, and Mr. H. Kanai for their support in performing the experiments. Thanks are also due to Drs. M. Kawamiya, F. Saito, H. Koyama, A. Yamazaki, and Y. Kawatani for their support in handling the simulation data.
Conflict of interest
The authors declare that the research was conducted in the absence of any commercial or financial relationships that could be construed as a potential conflict of interest.
Publisher's note
All claims expressed in this article are solely those of the authors and do not necessarily represent those of their affiliated organizations, or those of the publisher, the editors and the reviewers. Any product that may be evaluated in this article, or claim that may be made by its manufacturer, is not guaranteed or endorsed by the publisher.
References
Ashok, K., Behera, S. K., Rao, S. A., Weng, H., and Yamagata, T. (2007). El Niño Modoki and its possible teleconnection. J. Geophys. Res. 112, C11007. doi: 10.1029/2006JC003798
Cai, W., Wu, L., Lengaigne, M., Li, T., McGregor, S., Kug, J. S., et al. (2019). Pantropical climate interactions. Science 363, eaav4236. doi: 10.1126/science.aav4236
Capotondi, A., and Alexander, M. A. (2001). Rossby waves in the tropical North Pacific and their role in decadal thermocline variability. J. Phys. Oceanogr. 31, 3496–3515. doi: 10.1175/1520-0485(2002)031andlt;3496:RWITTNandgt;2.0.CO;2
Chang, P., Fang, Y., Saravanan, R., Ji, L., and Seidel, H. (2006). The cause of the fragile relationship between the Pacific El Niño and the Atlantic Niño. Nature 443, 324–328. doi: 10.1038/nature05053
Chikamoto, Y., Johnson, Z. F., Wang, S. Y. S., McPhaden, M. J., and Mochizuki, T. (2020). El Nino-Southern oscillation evolution modulated by Atlantic forcing. J. Geophys. Res. 125, e2020JC016318. doi: 10.1029/2020JC016318
Chikamoto, Y., Mochizuki, T., Timmermann, A., Kimoto, M., and Watanabe, M. (2016). Potential tropical Atlantic impacts on Pacific decadal climate trends. Geophys. Res. Lett. 43, 7143–7151. doi: 10.1002/2016GL069544
Chikamoto, Y., Timmerman, A., Luo, J. J., Mochizuki, T., Kimoto, M., Watanabe, M., et al. (2015). Skillful multi-year predictions of tropical trans-basin climate variability. Nat. Comms. 6, 6869. doi: 10.1038/ncomms7869
Ding, H., Keenlyside, N. S., and Larif, M. (2012). Impact of the equatorial Atlantic on the El Niño Southern Oscillation. Clim. Dyn. 38, 1965–1972. doi: 10.1007/s00382-011-1097-y
Ding, R., Li, J., Tseng, Y. H., Sun, C., and Zheng, F. (2017). Linking a sea level pressure anomaly dipole over North America to the central Pacific El Nino. Clim. Dyn. 49, 1321–1339. doi: 10.1007/s00382-016-3389-8
Doblas-Reyes, F. J., Andreu-Burillo, I., Chikamoto, Y., Garcia-Serrano, J., Guemas, V., Kimoto, M., et al. (2013). Initialized near-term regional climate change prediction. Nat. Comms. 4, 1715. doi: 10.1038/ncomms2704
Doblas-Reyes, F. J., Balmaseda, M. A., Weisheimer, A., and Palmer, T. N. (2011). Decadal climate prediction with the ECMWF coupled forecast system: impact of ocean observations. J. Geophys. Res. 116, D19111. doi: 10.1029/2010JD015394
Easterling, D. R., and Wehner, M. F. (2009). Is the climate warming or cooling? Geophys. Res. Lett. 36, L08706. doi: 10.1029/2009GL037810
England, M. H., McGregor, S., Spence, P., Meehl, G. A., Timmermann, A., Cai, W., et al. (2014). Recent intensification of wind-driven circulation in the Pacific and the ongoing warming hiatus. Nat. Clim. Change 4, 222–227. doi: 10.1038/nclimate2106
Fyfe, J. C., Meehl, G. A., England, M. H., Mann, M. E., Santer, B. D., Flato, G. M., et al. (2016). Making sense of the early-2000s warming slowdown. Nat. Clim. Change 6, 224–228. doi: 10.1038/nclimate2938
Ham, Y. G., Kug, J. S., Park, J. Y., and Jin, F. F. (2013). Sea surface temperature in the north tropical Atlantic as a trigger for El Nino/Southern Oscillation events. Nat. Geosci. 6, 112–116. doi: 10.1038/ngeo1686
Ishii, M., and Kimoto, M. (2009). Reevaluation of historical ocean heat content variations with time-varying XBT and MBT depth bias corrections. J. Oceanogr. 65, 287–299. doi: 10.1007/s10872-009-0027-7
Johnson, Z. F., Chikamoto, Y., Luo, J. J., and Mochizuki, T. (2018). Ocean impacts on Australian interannual to decadal precipitation variability. Climate 6, 61. doi: 10.3390/cli6030061
Johnson, Z. F., Chikamoto, Y., Wang, S. Y. S., McPhaden, M. J., and Mochizuki, T. (2020). Pacific decadal oscillation remotely forced by the equatorial pacific and the Atlantic Oceans. Clim. Dyn. 55, 789–811. doi: 10.1007/s00382-020-05295-2
Kim, H., Webster, P., and Curry, J. (2012). Evaluation of short-term climate change prediction in multi-model cmip5 decadal hindcasts. Geophys. Res. Lett. 39, L10701. doi: 10.1029/2012GL051644
Kosaka, Y., and Xie, S. P. (2013). Recent global-warming hiatus tied to equatorial Pacific surface cooling. Nature 501, 403–407.doi: 10.1038/nature12534
Kucharski F. F. Ikram F. Molteni R. Farneti I.-S. Kang H.-H. No M. P. (2016), Atlantic forcing of Pacific decadal variability. Clim. Dyn. 46, 2337–2351. 10.1007/s00382-015-2705-z .
Kug, J. S., Jin, F. F., and An, S. I. (2009). Two types of El Nino events: cold tongue El Nino and warm pool El Nino. J. Clim. 22, 1499–1515. doi: 10.1175/2008JCLI2624.1
Levine, A. F. Z., Frierson, D. M. W., and McPhaden, M. J. (2018). AMO forcing of multidecadal Pacific ITCZ variability. J. Clim. 31, 5749–5764. doi: 10.1175/JCLI-D-17-0810.1
Li, X., Xie, S. P., Gille, S. T., and Yoo, C. (2016). Atlantic-induced pan-tropical climate change over the past three decades. Nat. Clim. Change 6, 275–279. doi: 10.1038/nclimate2840
Luo, J. J., Liu, G., Hendon, H., Alves, O., and Yamagata, T. (2017). Inter-basin sources for two-year predictability of the multi-year La Nina event in 2010-2012. Sci. Rep. 7, 2276. doi: 10.1038/s41598-017-01479-9
Martin, T., Reintges, A., and Latif, M. (2019). Coupled North Atlantic subdecadal variability in CMIP5 models. J. Geophys. Res. 124, 2404–2417. doi: 10.1029/2018JC014539
Martín-Rey, M., Polo, I., Rodríguez-Fonseca, B., Losada, T., and Lazar, A. (2018). Is there evidence of changes in tropical Atlantic variability modes under AMO phases in the observational record? J. Clim. 31, 515–536. doi: 10.1175/JCLI-D-16-0459.1
Martín-Rey, M., Rodríguez-Fonseca, B., and Polo, I. (2015). Atlantic opportunities for ENSO prediction. Geophys. Res. Lett. 42, 6802–6810. doi: 10.1002/2015GL065062
Martín-Rey, M., Rodríguez-Fonseca, B., Polo, I., and Kusharski, F. (2014). On the atlantic-pacific ninos connection: a multidecadal modulated mode. Clim. Dyn. 43, 3163–3178. doi: 10.1007/s00382-014-2305-3
McGregor, S., Timmerman, A., Stuecker, M. F., England, M. H., Merrifield, M., Jin, F. F., et al. (2014). Recent Walker circulation strengthening and Pacific cooling amplified by Atlantic warming. Nat. Clim. Change 4, 888–892. doi: 10.1038/nclimate2330
Meehl, G. A., Hu, A., Arblaster, J. M., Fasullo, J., and Trenberth, K. E. (2013). Externally forced and internally generated decadal climate variability associated with the Interdecadal Pacific Oscillation. J. Clim. 26, 7298–7310. doi: 10.1175/JCLI-D-12-00548.1
Meehl, G. A., Hu, A., Santer, G. D., and Xie, S. P. (2016a). Contribution of the interdecadal pacific oscillation to twentieth-century global surface temperature trends, Nat. Clim. Change 6, 1005–1008. doi: 10.1038/nclimate3107
Meehl, G. A., Hu, A., and Teng, H. (2016b). Initialized decadal prediction for transition to positive phase of the interdecadal pacific oscillation. Nat. Comms. 7, 11718. doi: 10.1038/ncomms11718
Mochizuki, T., Chikamoto, Y., Kimoto, M., Ishii, M., Tatebe, H., Komuro, Y., et al. (2012). Decadal prediction using a recent series of MIROC global climate models. J. Meteorol. Soc. Japan 90A, 373–383. doi: 10.2151/jmsj.2012-A22
Mochizuki, T., Ishii, M., Kimoto, M., Chikamoto, Y., Watanabe, M., Nozawa, T., et al. (2010). Pacific decadal oscillation hindcasts relevant to near-term climate prediction. Proc. Natl. Acad. Sci. USA 107, 1833–1837. doi: 10.1073/pnas.0906531107
Mochizuki, T., Kimoto, M., Watanabe, M., Chikamoto, Y., and Ishii, M. (2016). Interbasin effects of the Indian Ocean on Pacific decadal climate change, Geophys. Res. Lett. 43, 7168–7175. doi: 10.1002/2016GL069940
Mochizuki, T., and Watanabe, M. (2019). Observed and hindcasted subdecadal variability of the tropical Pacific climate. ICES J. Marine Sci. 76, 1271–1279. doi: 10.1093/icesjms/fsz026
Newman, M. (2007). Interannual to decadal predictability of tropical and North Pacific sea surface temperatures. J. Clim. 20, 2333–2356. doi: 10.1175/JCLI4165.1
Polo, I., Martín-Rey, M., Rodriguez-Fonseca, B., Kucharski, F., and Mechoso, C. R. (2015). Processes in the Pacific La Niña onset triggered by the Atlantic Niño. Climate Dyn. 44, 115–131. doi: 10.1007/s00382-014-2354-7
Power, S., Delage, F., Chung, C., Kociube, G., and Keay, K. (2013). Robust twenty-first-century projections of El Niño and related precipitation variability. Nature 502, 541–545. doi: 10.1038/nature12580
Rodríguez-Fonseca, B., Polo, I., Gercia-Serrano, J., Losada, T., Mohino, E., Mechoso, C. R., et al. (2009). Are atlantic niños enhancing pacific enso events in recent decades? Geophys. Res. Lett., 36. doi: 10.1029/2009GL040048
Ruprich-Robert, Y., Moreno-Chamarro, E., Levine, X., Bellucci, A., Cassou, C., Castruccio, F., et al. (2021). Impacts of Atlantic multidecadal variability on the tropical Pacific: a multi-model study. NPJ Clim. Atm. Sci. 4, 1–11. doi: 10.1038/s41612-021-00188-5
Ruprich-Robert, Y., Msadek, R., Castruccio, F., Yeager, S., Delworth, T., Danabasoglu, G., et al. (2017). Assessing the climate impacts of the observed Atlantic multidecadal variability using the GFDL CM2.1 and NCAR CESM1 global coupled models. J. Clim. 30, 2810. doi: 10.1175/JCLI-D-16-0127.1
Smith, D. M., Eade, R., Scaife, A. A., Caron, L. P., Danabasoglu, G., DelSole, T. M., et al. (2019). Robust skill of decadal climate predictions. NPJ Clim. Atmos. Sci, 2, 13. doi: 10.1038/s41612-019-0071-y
Smith, D. M., Scaife, A. A., Eade, R., Athanasiadis, P., Bellucci, A., Bethke, I., et al (2020), North Atlantic climate far more predictable than models imply. Nature 583, 796–800. doi: 10.1038/s41586-020-2525-0.
Smith, D. M., Scaife, A. A., Hawkins, E., Bilbao, R., Boer, G. J., Caian, M., et al. (2018). Predicted chance that global warming will temporarily exceed 1.5 C. Geophys. Res. Lett. 45, 11–895. doi: 10.1029/2018GL079362
Sun, C., Kucharski, F., Li, J., Jin, F. F., Kang, I. S., Ding, R., et al. (2017). Western tropical Pacific multidecadal variability forced by the Atlantic multidecadal oscillation. Nat. Comms. 8, 15998. doi: 10.1038/ncomms15998
Tourre, Y. M., Cibot, C., Terray, L., White, W. B., and Dewitte, B. (2005). Quasi-decadal and inter-decadal climate fluctuations in the Pacific Ocean from a CGCM. Geophys. Res. Lett. 32, 7. doi: 10.1029/2004GL022087
Tourre, Y. M., Rajagopalan, B., Kushnir, Y., Barlow, M., and White, W. B. (2001). Patterns of coherent ocean decadal and interdecadal climate signals in the Pacific basin during the 20th century. Geophys. Res. Lett. 28, 2069–2072. doi: 10.1029/2000GL012780
Trenberth, K. E., and Hurrell, J. W. (1994). Decadal atmosphere-ocean variations in the Pacific. Clim. Dyn. 9, 303–319. doi: 10.1007/BF00204745
Wang, C. (2006). An overlooked feature of tropical climate: inter-Pacific-Atlantic variability. Geophys. Res. Lett. 33, L12702. doi: 10.1029/2006GL026324
Wang, C. (2019). Three-ocean interactions and climate variability: a review and perspective. Clim. Dyn. 53, 5119–5136. doi: 10.1007/s00382-019-04930-x
Wang, L., Yu, J. Y., and Paek, H. (2017). Enhanced biennial variability in the Pacific due to Atlantic capacitor effect. Nat. Comms. 8, 1–7. doi: 10.1038/ncomms14887
Wu, C. R., Lin, Y. F., and Qiu, B. (2019). Impact of the atlantic multidecadal oscillation on the pacific north equatorial current bifurcation. Sci. Rep. 9, 2162. doi: 10.1038/s41598-019-38479-w
Xu, K., Tam, C., Zhu, C., Liu, B., and Wang, W. (2017). CMIP5 projections of two types of El Niño and their related tropical precipitation in the twenty-first century. J. Clim. 30, 849–864. doi: 10.1175/JCLI-D-16-0413.1
Yeh, S. W., Kug, J. S., Dewitte, B., Kwon, M. H., Kirtman, B. P., Jin, F. F., et al. (2009). El Niño in a changing climate. Nature 461, 511–514. doi: 10.1038/nature08316
Yu, J., Kao, P., Paek, H., Hsu, H., Hung, C., Lu, M., et al. (2015). Linking emergence of the central Pacific El Niño to the atlantic multidecadal oscillation. J. Clim. 28, 651–662. doi: 10.1175/JCLI-D-14-00347.1
Keywords: subdecadal variability, interbasin connection, walker circulation, ocean thermocline, tropical climate, partial data assimilation
Citation: Mochizuki T and Watanabe M (2022) Atlantic impacts on subdecadal warming over the tropical Pacific in the 2000s. Front. Clim. 4:1040352. doi: 10.3389/fclim.2022.1040352
Received: 09 September 2022; Accepted: 07 November 2022;
Published: 22 November 2022.
Edited by:
Carlos R. Mechoso, University of California, Los Angeles, United StatesReviewed by:
Soon-Il A. N., Yonsei University, South KoreaJong-Seong Kug, Pohang University of Science and Technology, South Korea
Marta Martín-Rey, Complutense University of Madrid, Spain
Copyright © 2022 Mochizuki and Watanabe. This is an open-access article distributed under the terms of the Creative Commons Attribution License (CC BY). The use, distribution or reproduction in other forums is permitted, provided the original author(s) and the copyright owner(s) are credited and that the original publication in this journal is cited, in accordance with accepted academic practice. No use, distribution or reproduction is permitted which does not comply with these terms.
*Correspondence: Takashi Mochizuki, bW9jaGl6dWtpLnRha2FzaGkuODE3JiN4MDAwNDA7bS5reXVzaHUtdS5hYy5qcA==