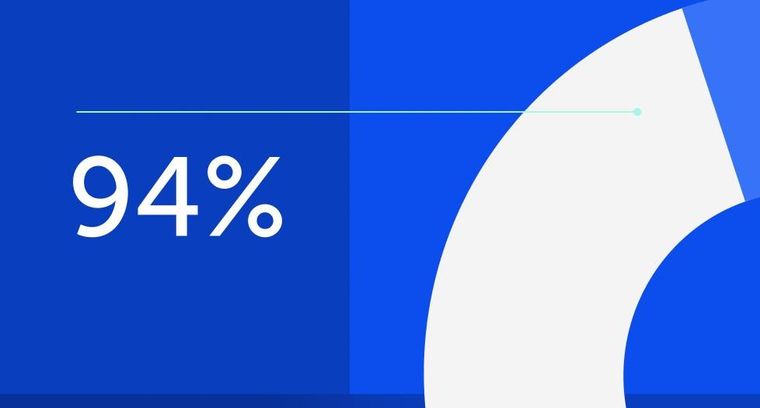
94% of researchers rate our articles as excellent or good
Learn more about the work of our research integrity team to safeguard the quality of each article we publish.
Find out more
PERSPECTIVE article
Front. Clim., 17 January 2022
Sec. Carbon Dioxide Removal
Volume 3 - 2021 | https://doi.org/10.3389/fclim.2021.820204
This article is part of the Research TopicEnhanced Weathering and Synergistic Combinations with Other CDR MethodsView all 12 articles
Enhanced weathering is a promising approach to remove carbon dioxide from the atmosphere. However, it may also pose environmental risks through the release of heavy metals, in particular nickel and chromium. In this perspective article I explore the potential role of plants in modulating these heavy metal fluxes. Agricultural basaltic soils may be valuable study sites in this context. However, the effect of biomass harvesting on the accumulation of heavy metals is currently not well studied. Mostly caused by different parent rock concentrations, there is a large variability of heavy metal concentrations in basaltic and ultramafic soils. Hence, to minimize environmental risks of enhanced weathering, basalts with low heavy metal concentrations should be favored. Existing phytoremediation strategies may be used to “phytoprevent” the accumulation of nickel and chromium released from enhanced weathering in soils. As a result, elevated nickel and chromium concentrations in rocks must not preclude enhanced weathering in all settings. In particular, hyperaccumulating plants could be used as part of a crop rotation to periodically remove heavy metals from soils. Enhanced weathering could also be employed on fields or forests of (non-hyper) accumulating plants that have a high primary production of biomass. Both approaches may have additional synergies with phytomining or bioenergy carbon capture and storage, increasing the total amount of carbon dioxide drawdown and at the same time preventing heavy metal accumulation in soils.
Limiting global warming to below 1.5 °C will require the development and implementation of carbon dioxide removal (CDR) technologies to offset residual and potential overshoot emissions (Fuss et al., 2018; IPCC, 2018). Among other CDR approaches (Fuss et al., 2018), terrestrial enhanced weathering (TEW) of silicate rocks has the potential to limit the accumulation of CO2 in the atmosphere through the acceleration of natural weathering processes. Although TEW removes only ~0.3–1.1 tCO2 per ton of rock (Köhler et al., 2010; Moosdorf et al., 2014; Strefler et al., 2018), when scaled up to large application rates (e.g. 40 t ha−1; Beerling et al., 2020) it can remove between 0.5 and 4 GtCO2 yr−1 (Fuss et al., 2018; Beerling et al., 2020; Goll et al., 2021) or even up to 95 GtCO2 yr−1 (Strefler et al., 2018). Co-benefits of EW include (i) increasing the alkalinity of natural waters which ameliorates ocean acidification and decreasing aragonite saturation (Taylor et al., 2016), (ii) stabilizing the soil pH which benefits plants and reduces emissions of other greenhouse gases (Kantola et al., 2017), (iii) improving soil hydrology (de Oliveira Garcia et al., 2020), and (iv) the release of nutrients which stimulates further biological carbon sequestration (Goll et al., 2021). Carbon dioxide removal through TEW does not require underground storage in geological reservoirs but still stores CO2 in stable non-biological reservoirs such as the marine alkalinity pool or marine/pedogenic carbonates. Furthermore, selling carbon credits from TEW may also support livelihoods in the Global South and help progress toward multiple sustainable development goals (Smith et al., 2019).
Although models suggest that TEW could be a suitable CDR strategy (Strefler et al., 2018; Beerling et al., 2020; Goll et al., 2021), and pot and sediment core experiments detect increasing cation fluxes or bioavailable concentrations (Anda et al., 2009, 2013, 2015; ten Berge et al., 2012; Renforth et al., 2015; Amann et al., 2020), first field trials of TEW in agricultural settings do not detect an enhanced weathering signal in drainage waters (Andrews and Taylor, 2019). This is problematic because drainage waters integrate weathering signals over their catchment and would therefore be ideal to quantify TEW processes. Possible reasons include the retardation of weathering signals by the soil exchangeable cation pool (Pogge von Strandmann et al., 2021), precipitation of solutes in pedogenic carbonate (Haque et al., 2019, 2020b,c) and other secondary minerals, as well as the incorporation of released cations into biomass (Andrews and Taylor, 2019). Support for the latter mechanism can be drawn from (i) the fertilization potential of TEW implying cation uptake by plants (Hartmann et al., 2013; Amann and Hartmann, 2018; Goll et al., 2021), (ii) detectable cation flux responses in TEW experiments in forests (Peters et al., 2004; Shao et al., 2016; Taylor et al., 2021), and (iii) the observation that biomass constitutes a missing sink in weathering flux mass balances (von Blanckenburg et al., 2021).
Suitable rocks for enhanced weathering include basalt and dunite, an ultramafic rock mostly comprised of olivine. Both rocks, particularly dunite, often have elevated concentrations of Ni and Cr (Alloway, 2012). Alternatives with lower HM concentrations exist, for example wollastonite (Peters et al., 2004; Shao et al., 2016; Haque et al., 2019, 2020b,c; Taylor et al., 2021). However, they can be limited by availability (~0.1Gt global wollastonite reserves; USGS, 2021) or price. Upon release through weathering, Ni and Cr have the tendency to be incorporated into secondary phases or adsorb onto surfaces (Alloway, 2012). Hence, TEW may result in accumulation of these heavy metals in soils and biomass which can be toxic to living organisms at high concentrations (Miranda et al., 2009; Alloway, 2012; Hartmann et al., 2013; Beerling et al., 2018; Amann et al., 2020). For example, in an experiment using an agricultural soil core, ~99% of Ni and Cr released from olivine weathering are retained in the soil, limiting addition of olivine to soils to 95 t ha−1 before permissible Ni concentrations are exceeded (Renforth et al., 2015). However, plants also take up trace elements like Ni and Cr (Pulford and Watson, 2003; Etim, 2012), which might result in accumulation of these elements in periodically harvested and removed biomass. As a result, grass grown on basaltic soils may have Ni and Cr concentrations of 2.2 and 0.3 ppm (Néel et al., 2007), and 11–39 and 0.4–7.1 ppm when grown on ultramafic soils (Miranda et al., 2009). While this may have implications for food safety, it could also mean that concentrations could remain at safe levels in both soil and biomass.
Clearly, both the quantification of TEW fluxes as well as TEW related heavy metal (HM) cycling require a deeper understanding of how plants interact with the solutes released through TEW. Focusing on the latter aspect, in this perspective article I explore the lessons from existing literature on HM in basaltic soils as well as using phytoremediation strategies to “phytoprevent” accumulation of HM in soils. Phytoprevention might prove useful since understanding and mitigating detrimental environmental consequences of TEW is an important prerequisite for its large-scale implementation. It is also important from a global climate justice perspective, as TEW might be employed in countries of the Global South (Strefler et al., 2018; Beerling et al., 2020; Goll et al., 2021) that have not substantially contributed to the climate crisis.
Soils formed from ultramafic and basaltic parent materials exist in various locations where these rocks occur naturally. Many of these soils are well studied, giving the opportunity to derive implications for TEW. Although basalt weathers (and removes CO2 from the atmosphere) much slower than ultramafic lithologies (Strefler et al., 2018), I focus on basalt due to the high abundance of basalt outcrops (Hartmann and Moosdorf, 2012), its phosphorous fertilization potential (Gillman et al., 2002; Hartmann et al., 2013), and lower HM concentrations of ~130 ppm Ni and ~250 ppm Cr compared to ~2000 ppm Ni and ~2300 ppm Cr in ultramafic rocks like dunite (Alloway, 2012). As an upper limit for acceptable concentrations of Ni and Cr in soils I use 100 ppm and 200 ppm respectively, which are broadly representative of average limits throughout the EU (Tóth et al., 2016).
Globally, Ni and Cr concentrations in basaltic soils vary over two (Ni) to three (Cr) orders of magnitude (Figure 1). Some soils fall below, and some above, these thresholds. The main factor controlling soil concentrations is the composition of the parent material (Tra and Egashira, 2001; Dœlsch et al., 2006a,b; de Oliveira et al., 2011; Mendoza-Grimón et al., 2014; Cabral Pinto et al., 2015; Cox et al., 2017; Mazhari et al., 2017; Vlček et al., 2017; Fabrício Neta et al., 2018; Memoli et al., 2018; Iskandar et al., 2019; Bocardi et al., 2020; Wang et al., 2020). This is expected given the tendency of Ni and Cr to be retained in soils (Alloway, 2012). Similarly, reflecting elevated parent materials concentrations, soils formed from ultramafic rocks have much higher concentrations of Ni and Cr – on average ~2000 ppm Ni and ~2750 ppm Cr (Kierczak et al., 2021). Only few studies assess the impact of biology on HM concentrations in basaltic soils. On La Réunion, Ni and Cr concentrations are similar in cultivated and uncultivated soils (Dœlsch et al., 2006b), though it is not clear whether this is due to vegetation having no effect, or a canceling out of HM removal in biomass and inputs from fertilizer which can contain HM like Ni (McMurtry et al., 1995). At Mount Vesuvius, plant cover determines the HM fraction that is reducible or oxidizable mostly through its control on soil organic matter quality and associated impacts on pH, oxidation state and element mobility (Memoli et al., 2018). Although few studies report the composition of the parent material, some find HM concentrations in soils that are lower than in the parent material (McMurtry et al., 1995; Wang et al., 2020), indicating the presence of a HM sink.
Figure 1. Chromium and Ni concentrations in soils derived from basaltic rocks. The chosen acceptable limits for agricultural soils are representative of average limits throughout the EU (Tóth et al., 2016), although both higher or lower values are adopted into legislature in various countries (Tóth et al., 2016; Iskandar et al., 2019). For studies where individual sample data were not reported, the mean as well as the range of results (stippled lines) are shown. Where reported, the standard deviation around the mean (error bars) is plotted as well. (a) Tra and Egashira, 2001, (b) Amaral et al., 2006, (c) Dœlsch et al., 2006a, (d) de Oliveira et al., 2011, (e) Fabrício Neta et al., 2018, (f) Cabral Pinto et al., 2015, (g) Rodriguez-Espinosa et al., 2015, (h) Cox et al., 2017, (i) Mazhari et al., 2017, (j) Memoli et al., 2018, (k) Ma et al., 2019, (l) Vlček et al., 2017, (m) Mendoza-Grimón et al., 2014, (n) Iskandar et al., 2019, (o) Bocardi et al., 2020, (p) Wang et al., 2020, (q) Tóth et al., 2016.
This analysis shows that, first, there is large variability of Ni and Cr concentrations in soils formed from basalt (Figure 1), which is also valid for soils formed from ultramafic rocks (Kierczak et al., 2021). Second, much of this variability seems to depend on the composition of the parent material. This highlights that if HM exposure is to be minimized based exclusively on parent rock composition, the variability within the respective rock classes should be taken into account, favoring rocks with low concentrations in both rock types (i.e. also differentiating between high and low HM concentrations in basalts). Thus, choosing basalt or ultramafic rocks becomes a decision based on other factors like the required rate of CO2 uptake or nutrient requirements, rather than environmental risk alone. Third, the extent to which biology effects buildup of HM in basaltic soils is currently poorly understood. Given this limited state of knowledge and the fact that additional sinks of Ni and Cr exist at least in some settings (McMurtry et al., 1995; Wang et al., 2020), further studies assessing the effect of different types of vegetation cover and agricultural practices on HM concentrations in basaltic (and ultramafic) soils would be valuable in the TEW context.
Although high concentrations of HM in ultramafic soils make them toxic for many plants, they are also a niche habitat for plant species that are adapted to coping with these conditions by either excluding HM from, or (hyper-)accumulating HM in, their plant tissues (Kierczak et al., 2021). While hyperaccumulation has probably evolved as a defense mechanism of plants against herbivores (Rascio and Navari-Izzo, 2011), excluders use one or several separate strategies to either break down contaminants or make them less bioavailable (e.g. phytodegradation, rhizodegradation, and phytostabilization; Etim, 2012; Yadav et al., 2021).
In phytoremediation, appropriate plants are used to decontaminate soils or water bodies polluted from anthropogenic impacts either by extracting HM or making them less bioavailable. To remove metals from soils, phytoextracting plants that accumulate metals of interest, ideally in the above ground biomass, are particularly useful (Pulford and Watson, 2003; Vara Prasad and de Oliveira Freitas, 2003; Etim, 2012; Yadav et al., 2021). These plants are usually separated into hyperaccumulators having a metal concentration of >1000 ppm of some HM (including Ni and Cr) in their dried biomass (Baker and Brooks, 1989) and non-hyperaccumulators that have lower HM concentrations but may still accumulate HM from soils (called accumulators here). Hyperaccumulating plants usually have a lower biomass production (Pulford and Watson, 2003). As a result, accumulating plants of lower HM concentration but high biomass production can be just as effective at removing HM from soils.
In phytoremediation, plants are used to treat soils after they have been contaminated. The existence of these approaches suggests that they could be used preemptively to prevent accumulation and contamination of soils with HM released from TEW (Haque et al., 2020a) – termed “phytoprevention” here. In combination with TEW, this seems a promising approach given that plant species have been identified that (hyper-)accumulate Ni and Cr from soils and waters. While several hundred Ni hyperaccumulating taxa have been identified (Lone et al., 2008), Cr hyperaccumulation is generally scarce (Han et al., 2004; Singh et al., 2013; Teuchies et al., 2013; van der Ent et al., 2013) due to the low bioavailability of Cr(III) (dominating over Cr(IV) in most soils; Kotaś and Stasicka, 2000; Alloway, 2012). In the context of phytoprevention, I outline two synergistic approaches between phytoprevention and TEW below, as well a third approach to remove HM from effluent waters. A comprehensive review of potentially suitable species is beyond the scope of this perspective article, but a selection of promising species for each approach can be found in Table 1.
Table 1. Selected plants that have been used to remove Cr and/or Ni from soils or water and might be useful in one of the three phytoprevention scenarios outlined in the main text.
In approach one, TEW is employed on agricultural fields (including for food production) and hyperaccumulating plants periodically remove Ni and Cr from the soils as part of a crop rotation. Many hyperaccumulators can reach metal concentrations of 1–3% of Ni. Given that their ashes may contain 12% to >20% of Ni, this approach might also enable the commercial retrieval of the metals initially contained in the basalt or ultramafic rocks, i.e. phytomining (Chaney et al., 2007; Alloway, 2012) in addition to limiting HM buildup in soil and biomass. Although some examples like southern cutgrass (Leersia hexandra) or Chinese brake (Pteris vittate; in hydroponic experiments) of Cr hyperaccumulation exist (Zhang et al., 2007; Kalve et al., 2011), it is not clear whether this strategy could work for Cr due to its limited bioavailability.
In approach two, managed fields or forests of accumulating plants, ideally grown for non-food applications, are used for TEW. While the concentrations of HM in these plants are lower, their larger biomass production may still make them suitable for phytoprevention. This approach has potential synergies with bioenergy carbon capture and storage (BECCS) discussed in the next section. Low translocation from roots to above ground biomass may limit HM extraction efficiency for some of these plants or require laborious root harvesting (Pulford et al., 2001; Pulford and Watson, 2003; Were et al., 2017; Ranieri et al., 2020). An additional benefit of this approach is that many involved plant species, like bamboo and willow, stabilize soils and prevent erosion, decreasing the risk of spreading potential contamination to adjacent areas (Riddell-Black, 1994; Were et al., 2017). Intercropping accumulating plants with hyperaccumulating plants may increase overall crop metal accumulation, protect the accumulating plants, and increase overall biomass production (Kidd et al., 2015; Bian et al., 2020).
In the third approach, hyperaccumulating aquatic freshwater plants are used to remove HM from surface runoff and/or groundwater springs or wells to prevent contamination of rivers and freshwater resources. Many terrestrial hyperaccumulators like Indian mustard (Brasicca juncea) or Chinese brake (Pteris vittate) presented in approach one can also extract HM from hydroponic solutions (Diwan et al., 2008; Kalve et al., 2011; Ansari et al., 2015) enlarging the pool of suitable species.
Using accumulating plants of high biomass production for phytoprevention of HM pollution from TEW, i.e. approach two above, has the benefit that this biomass might be used for other CDR approaches like BECCS. For example, bamboo has a high net primary productivity of 12-26 t ha−1 yr−1 (Nath et al., 2015). Willows can be frequently harvested and yield as much as 10–15 t ha−1 dry biomass (Pulford and Watson, 2003). Both plants have been suggested as energy feedstocks in BECCS (Fajardy and Mac Dowell, 2017; Quader and Ahmed, 2017; Albanito et al., 2019). Removal of nutrients from soils through harvested biomass results in soils that are often nutrient deficient in terms of P and K in bamboo forests (Guan et al., 2017) and forests in general (Thiffault et al., 2011), limiting biomass production. As a result, combining TEW and phytoprevention with BECCS may produce a synergistic system where TEW supplies additional nutrients, increases growth rates, and CO2 fixation of the BECCS feedstock. At the same time, regular harvesting of HM bearing biomass could phytoprevent the accumulation of HM in soils. While increased HM concentrations in biomass may pose challenges to bioenergy power plants from a pollution point of view, it may be possible to extract these metals through phytomining (Edgar et al., 2021).
For all three approaches, plants should be chosen that are adjusted to the local climate conditions, favoring native plant varieties (Arreghini et al., 2017; Wei et al., 2021). Further research and a large database of plants that can potentially ameliorate HM contamination would therefore greatly benefit the management of environmental consequences of TEW.
Phytoremediation draws on a few other strategies, which may also be relevant in the context of phytoprevention. Examples are the use of rhizobacteria (Yadav et al., 2021) or mycorrhiza (Coninx et al., 2017) that may encourage plant growth, and increase the bioavailability and uptake efficiency of metals. The latter effect could also be achieved by adding chelating agents like EDTA to soils (Lim et al., 2004; Wu et al., 2004), although this should be viewed with caution and tested meticulously as the increased bioavailability and element mobility might promote runoff of HM into surface and groundwaters (Cooper et al., 1999; Pulford and Watson, 2003; Wu et al., 2004). Another interesting potential phytoprevention strategy common in phytoremediation is using phytostabilizing plants, which may have a similar effect as biochar in that they reduce HM bioavailability and runoff (Amann and Hartmann, 2018).
Upper limits of environmentally safe addition of olivine or basalt to soils may easily be calculated based on the cumulative amount of rocks added and their HM concentrations (Renforth et al., 2015). However, this may overestimate the environmental impact in the presence of additional HM sinks. In principle, a limit to TEW application at which HM do not accumulate in soils may be calculated from the heavy metal fluxes in biomass and rock:
Where u is the uptake efficiency of HM in biomass, and d the fraction of source rock dissolved in the given period. For example, in a simplified case where u and d are 1 (biomass HM concentration as high as it is at high soil concentrations, source rock dissolved completely), bamboo could extract the Cr equivalent of up to ~55 t basalt ha(−1) yr(−1), and mustard family plants the Ni equivalent of ~180 t basalt ha(−1) yr(−1). In practice, this would require a more realistic parametrization of weathering (d) and plant HM uptake (u) processes at the given soil and source rock conditions, including of concentrations of HM in biomass at lower concentrations in soils. Potentially, elevated HM concentrations in rocks must not preclude TEW in all settings: If HM are continuously removed from soils through phytoprevention, even prolonged TEW might not exceed acceptable HM thresholds in either soil, drainage water, or harvested biomass. Overall, the approach of phytoprevention highlights the need for TEW studies to assess the fate of HM, as well as weathering fluxes in general, in terms of all input and output fluxes including biomass.
Publicly available datasets were analyzed in this study. The data sources are referenced in the article.
The author confirms being the sole contributor of this work and has approved it for publication.
The publication costs were covered by ETH Zurich.
The author declares that the research was conducted in the absence of any commercial or financial relationships that could be construed as a potential conflict of interest.
All claims expressed in this article are solely those of the authors and do not necessarily represent those of their affiliated organizations, or those of the publisher, the editors and the reviewers. Any product that may be evaluated in this article, or claim that may be made by its manufacturer, is not guaranteed or endorsed by the publisher.
I would like to thank Prof. Dr. Jens Hartmann for discussing this idea with me, encouraging me to write this paper, and his constructive suggestions on how it may be improved. I further thank Prof. Dr. Derek Vance, Dr. Jörg Rickli, and Clara Leonie Bütow for editing, Lydia Ruth Bailey for proofreading an early version of this manuscript, and finally Dr. Fatima Haque, Dr. Rafael Mattos Dos Santos, and Dr. Sofie Thijs for their constructive reviews.
Albanito, F., Hastings, A., Fitton, N., Richards, M., Martin, M., and Mac Dowell, N. (2019). Mitigation potential and environmental impact of centralized versus distributed BECCS with domestic biomass production in Great Britain. GCB Bioenergy. 11, 1234–1252. doi: 10.1111/gcbb.12630
Alloway, B. J. (2012). Heavy Metals in Soils - Trace Metals and Metalloids in Soils and their Bioavailability. Springer. doi: 10.1007/978-94-007-4470-7
Amann, T., and Hartmann, J. (2018). Ideas and perspectives: Synergies from co-deployment of negative emission technologies. Biogeosc. Discus. 16, 2949–2960. doi: 10.5194/bg-16-2949-2019
Amann, T., Hartmann, J., Struyf, E., Oliveira Garcia, D. e, Fischer, W., Janssens, E. K., et al. (2020). Enhanced Weathering and related element fluxes - A cropland mesocosm approach. Biogeosciences. 17, 103–119. doi: 10.5194/bg-17-103-2020
Amaral, A., Cruz, J., Cunha, R., and Rodrigues, A. (2006). Baseline levels of metals in volcanic soils of the Azores (Portugal). Soil Sediment Contamin. 15, 123–130. doi: 10.1080/15320380500506255
Anda, M., Shamshuddin, J., and Fauziah, C. I. (2013). Increasing negative charge and nutrient contents of a highly weathered soil using basalt and rice husk to promote cocoa growth under field conditions. Soil Tillage Res. 132, 1–11. doi: 10.1016/j.still.2013.04.005
Anda, M., Shamshuddin, J., and Fauziah, C. I. (2015). Improving chemical properties of a highly weathered soil using finely ground basalt rocks. Catena. 124, 147–161. doi: 10.1016/j.catena.2014.09.012
Anda, M., Shamshuddin, J., Fauziah, C. I., and Omar, S. R. S. (2009). Dissolution of ground basalt and its effect on oxisol chemical properties and cocoa growth. Soil Sci. 174, 264–271. doi: 10.1097/SS.0b013e3181a56928
Andrews, M. G., and Taylor, L. L. (2019). Combating climate change through enhanced weathering of agricultural soils. Elements, 15, 253–258. doi: 10.2138/gselements.15.4.253
Ansari, M. K. A., Ahmad, A., Umar, S., Zia, M. H., Iqbal, M., and Owens, G. (2015). Genotypic variation in phytoremediation potential of indian mustard exposed to nickel stress: a hydroponic study. Int. J. Phytoremed. 17, 135–144. doi: 10.1080/15226514.2013.862206
Arreghini, S., de Cabo, L., Serafini, R., and de Iorio, A. F. (2017). Effect of the combined addition of Zn and Pb on partitioning in sediments and their accumulation by the emergent macrophyte Schoenoplectus californicus. Environ. Sci. Pollut. Res. 24, 8098–8107. doi: 10.1007/s11356-017-8478-7
Baker, A. J. M., and Brooks, R. R. (1989). Terrestrial higher plants which hyperaccumulate metallic elements - a review of their distribution, ecology and phytochemistry. Biorecovery. 1, 81–126.
Bani, A., Pavlova, D., Echevarria, G., Mullaj, A., Reeves, R. D., Morel, J. L., et al. (2010). Nickel hyperaccumulation by the species of Alyssum and Thlaspi (Brassicaceae) from the ultramafic soils of the Balkans. Botanica Serbica. 34, 3–14. Available online at: https://botanicaserbica.bio.bg.ac.rs/arhiva/pdf/2010_34_1_502_full.pdf
Beerling, D. J., Kantzas, E. P., Lomas, M. R., Wade, P., Eufrasio, R. M., Renforth, P., et al. (2020). Potential for large-scale CO 2 removal via enhanced rock weathering with croplands. Nature. 583, 242–248. doi: 10.1038/s41586-020-2448-9
Beerling, D. J., Leake, J. R., Long, S. P., Scholes, J. D., Ton, J., Nelson, P. N., et al. (2018). Farming with crops and rocks to address global climate, food and soil security. Nat. Plants. 4, 138–147. doi: 10.1038/s41477-018-0108-y
Bian, F., Zhong, Z., Zhang, X., Yang, C., and Gai, X. (2020). Bamboo – An untapped plant resource for the phytoremediation of heavy metal contaminated soils. Chemosphere. 246, 125750. doi: 10.1016/j.chemosphere.2019.125750
Bocardi, J. M. B., Pletsch, A. L., Melo, F., and Quinaia, S. P. (2020). Quality reference values for heavy metals in soils developed from basic rocks under tropical conditions. J. Geochem. Explor. 217, 106591. doi: 10.1016/j.gexplo.2020.106591
Brooks, R. R., Lee, J., and Jaffre, T. (1974). Some New Zealand and new caledonian plant accumulators of nickel. J. Ecol. 62, 493–499. doi: 10.2307/2258995
Cabral Pinto, M., da Silva, E. F., Silva, M. M. G., and Melo-Gonçalves, P. (2015). Heavy metals of Santiago Island (Cape Verde) top soils: Estimated Background Value maps and environmental risk assessment. J. Afri. Earth Sci. 101, 162–176. doi: 10.1016/j.jafrearsci.2014.09.011
Chaney, R. L., Angle, J. S., Broadhurst, C. L., Peters, C. A., Tappero, R. V., and Sparks, D. L. (2007). Improved understanding of hyperaccumulation yields commercial phytoextraction and phytomining technologies. J. Environ. Qual. 36, 1429–1443. doi: 10.2134/jeq2006.0514
Coninx, L., Martinova, V., and Rineau, F. (2017). Mycorrhiza-Assisted Phytoremediation. Adv. Botan. Res. 83, 127–188. doi: 10.1016/bs.abr.2016.12.005
Cooper, E. M., Sims, J. T., Cunningham, S. D., Huang, J. W., and Berti, W. R. (1999). Chelate-assisted phytoextraction of lead from contaminated soils. J. Environ. Qual. 28, 1709–1719. doi: 10.2134/jeq1999.00472425002800060004x
Cox, S. F., Rollinson, G., and McKinley, J. M. (2017). Mineralogical characterisation to improve understanding of oral bioaccessibility of Cr and Ni in basaltic soils in Northern Ireland. J. Geochem. Explor. 183, 166–177. doi: 10.1016/j.gexplo.2017.02.006
de Oliveira Garcia, W., Amann, T., Hartmann, J., Karstens, K., Popp, A., and Boysen, L. R. (2020). Impacts of enhanced weathering on biomass production for negative emission technologies and soil hydrology. Biogeosciences. 17, 2107–2133. doi: 10.5194/bg-17-2107-2020
de Oliveira, S., Pessenda, L. C., Gouveia, S. E., and Favaro, D. I. (2011). Heavy metal concentrations in soils from a remote oceanic island Fernando de Noronha, Brazil. Anais Acade. Brasileira Ciencias. 83, 1193–1206. doi: 10.1590/S0001-37652011005000042
Dhir, B., Sharmila, P., Pardha Saradhi, P., and Nasim, S. A. (2009). Physiological and antioxidant responses of Salvinia natans exposed to chromium-rich wastewater. Ecotoxicol. Environ. Safety. 72, 1790–1797. doi: 10.1016/j.ecoenv.2009.03.015
Diwan, H., Ahmad, A., and Iqbal, M. (2008). Genotypic variation in the phytoremediation potential of indian mustard for chromium. Environ. Manage. 41, 734–741. doi: 10.1007/s00267-007-9020-3
Dœlsch, E., Saint Macary, H., and Van de Kerchove, (2006b). Sources of very high heavy metal content in soils of volcanic island (La Réunion). J. Geochem. Explor. 88, 194–197. doi: 10.1016/j.gexplo.2005.08.037
Dœlsch, E., Van de Kerchove, V., and Saint Macary, H. (2006a). Heavy metal content in soils of Réunion (Indian Ocean). Geoderma. 134, 119–134. doi: 10.1016/j.geoderma.2005.09.003
Dushenkov, V., Nanda Kumar, P. B. A., Motto, H., and Raskin, I. (1995). Rhizofiltration: the use of plants to remove heavy metals from aqueous streams. Environ. Sci. Technol. 29, 1239–1245. doi: 10.1021/es00005a015
Edgar, N., Fabián, F. L., Julián Mario, P. C., and Ileana, R. (2021). Coupling plant biomass derived from phytoremediation of potential toxic-metal-polluted soils to bioenergy production and high-value by-products-a review. Appl. Sci. 11, 1–35. doi: 10.3390/app11072982
Etim, E. E. (2012). Phytoremediation and its mechanisms: a review. Int. J. Environ. Bioenergy. 2, 120–136. Available online at: http://www.modernscientificpress.com/Journals/ViewArticle.aspx?gkN1Z6Pb60HNQPymfPQlZIsaO1oMajYkT5i8/SIthV/i1509l3XqlgX4XSDiXBec
Fabrício Neta, A. D. B., do Nascimento, C. W. A., Biondi, C. M., van Straaten, P., and Bittar, S. M. B. (2018). Natural concentrations and reference values for trace elements in soils of a tropical volcanic archipelago. Environ. Geochem. Health. 40, 163–173. doi: 10.1007/s10653-016-9890-5
Fajardy, M., and Mac Dowell, N. (2017). Can BECCS deliver sustainable and resource efficient negative emissions? Energy Environ. Sci. 10, 1389–1426. doi: 10.1039/C7EE00465F
Fazal, S., Zhang, B., and Mehmood, Q. (2015). Biological treatment of combined industrial wastewater. Ecol Eng. 84, 551–558. doi: 10.1016/j.ecoleng.2015.09.014
Fuentes, I. I., Espadas-Gil, F., Talavera-May, C., Fuentes, G., and Santamaría, J. M. (2014). Capacity of the aquatic fern (Salvinia minima Baker) to accumulate high concentrations of nickel in its tissues, and its effect on plant physiological processes. Aquatic Toxicol. 155, 142–150. doi: 10.1016/j.aquatox.2014.06.016
Fuss, S., Lamb, W. F., Callaghan, M. W., Hilaire, J., Creutzig, F., Amann, T., et al. (2018). Negative emissions - Part 2: costs, potentials and side effects. Environ. Res. Lett. 13, 063002. doi: 10.1088/1748-9326/aabf9f
Gillman, G. P., Burkett, D. C., and Coventry, R. J. (2002). Amending highly weathered soils with finely ground basalt rock. Appl. Geochem. 17, 987–1001. doi: 10.1016/S0883-2927(02)00078-1
Goll, D. S., Ciais, P., Amann, T., Buermann, W., Chang, J., Eker, S., et al. (2021). Potential CO2 removal from enhanced weathering by ecosystem responses to powdered rock. Nat Geosci. 14, 545–549. doi: 10.1038/s41561-021-00798-x
Guan, F., Xia, M., Tang, X., and Fan, S. (2017). Spatial variability of soil nitrogen, phosphorus and potassium contents in Moso bamboo forests in Yong'an City, China. Catena. 150, p. 161–172. doi: 10.1016/j.catena.2016.11.017
Han, F. X., Sridhar, B. B. M., Monts, D. L., and Su, Y. (2004). Phytoavailability and toxicity of trivalent and hexavalent chromium to Brassica juncea. New Phytol. 162, 489–499. doi: 10.1111/j.1469-8137.2004.01027.x
Haque, F., Chiang, Y. W., and Santos, R. M. (2020a). Risk assessment of Ni, Cr, and Si release from alkaline minerals during enhanced weathering. Open Agric. 5, 166–175. doi: 10.1515/opag-2020-0016
Haque, F., Santos, R. M., and Chiang, Y. W. (2020b). CO2 sequestration by wollastonite-amended agricultural soils – An Ontario field study. Int. J. Greenhouse Gas Control. 97, 103017. doi: 10.1016/j.ijggc.2020.103017
Haque, F., Santos, R. M., and Chiang, Y. W. (2020c). Optimizing inorganic carbon sequestration and crop yield with wollastonite soil amendment in a microplot study. Front. Plant Sci. 11, 1–12. doi: 10.3389/fpls.2020.01012
Haque, F., Santos, R. M., Dutta, A., Thimmanagari, M., and Chiang, Y. W. (2019). Co-benefits of wollastonite weathering in agriculture: CO2 sequestration and promoted plant growth. ACS Omega. 4, 1425–1433. doi: 10.1021/acsomega.8b02477
Hartmann, J., and Moosdorf, N. (2012). The new global lithological map database GLiM: a representation of rock properties at the Earth surface. Geochem. Geophys. Geosyst. 13, 1–37. doi: 10.1029/2012GC004370
Hartmann, J., West, A. J., Renforth, P., Köhler, P., De La Rocha, C. L., Wolf-Gladrow, D. A., et al. (2013). Enhanced chemical weathering as a geoengineering strategy to reduce atmospheric carbon dioxide, supply nutrients, and mitigate ocean acidification. Rev. Geophys. 51, 113–149. doi: 10.1002/rog.20004
Ingole, N. W., and Bhole, A. G. (2003). Removal of heavy metals from aqueous solution by water hyacinth (Eichhornia crassipes). J. Water Supply. Res. Technol. AQUA. 52, 119–128. doi: 10.2166/aqua.2003.0012
IPCC, (2018). Summary for Policy makers, in Global Warming of 1, 5.°C. An IPCC Special Report on the impacts of global warming of 1.5°C above pre-industrial levels and related global greenhouse gas emission pathways, in the context of strengthening the global response to the threat of climate change. World Meteorological Organization, Geneva, Switzerland, p. 32.
Iskandar, Darmawan Sudadi, U., and Mulyanto, B. (2019). Ambient concentration of soil heavy metals in Indonesian tertiary and quaternary geologic formations: an explorative study. In: IOP Conference Series: Earth and Environmental Science. Vol. 399, p. 012017. doi: 10.1088/1755-1315/399/1/012017
Jaffr,é, T., and Schmid, M. (1974). Accumulation du nickel par une Rubiacée de Nouvelle-Calédonie, Psychotria douarrei (G. Beauvisage) Däniker: Comptes Rendus de l'Académie des Sciences. Série D: Sci. Naturel. 278, 1727–1730.
Jaffré, T., Brooks, R. R., Lee, J., and Reeves, R. D. (1976). Sebertia acuminata: a hyperaccumulator of nickel from New Caledonia. Science. 193, 579–580. doi: 10.1126/science.193.4253.579
Jaffré, T., Pillon, Y., Thomine, S., and Merlot, S. (2013). The metal hyperaccumulators from New Caledonia can broaden our understanding of nickel accumulation in plants. Front. Plant Sci. 4, 1–7. doi: 10.3389/fpls.2013.00279
Kalve, S., Sarangi, B. K., Pandey, R. A., and Chakrabarti, T. (2011). Arsenic and chromium hyperaccumulation by an ecotype of Pteris vittata - prospective for phytoextraction from contaminated water and soil. Current Sci. 100, 888–894. Available online at: https://www.jstor.org/stable/24076481
Kantola, I. B., Masters, M. D., Beerling, D. J., Long, S. P., and DeLucia, E. H. (2017). Potential of global croplands and bioenergy crops for climate change mitigation through deployment for enhanced weathering. Biol. Lett. 13, 20160714. doi: 10.1098/rsbl.2016.0714
Kidd, P., Mench, M., Alvarez-Lopez, V., Bert, V., Dimitriou, I., Friesl-Hanl, W., et al. (2015). Agronomic Practices for Improving Gentle Remediation of Trace Element-Contaminated Soils. Int. J. Phytoremed. 17, 1005–1037. doi: 10.1080/15226514.2014.1003788
Kidd, P. S., and Monterroso, C. (2005). Metal extraction by Alyssum serpyllifolium ssp. lusitanicum on mine-spoil soils from Spain. Sci. Total Environ. 336, 1–11. doi: 10.1016/j.scitotenv.2004.06.003
Kierczak, J., Pietranik, A., and Pedziwiatr, A. (2021). Ultramafic geoecosystems as a natural source of Ni, Cr, and Co to the environment: a review. Sci Total Environ. 755, 142620. doi: 10.1016/j.scitotenv.2020.142620
Köhler, P., Hartmann, J., and Wolf-Gladrow, D. A. (2010). Geoengineering potential of artificially enhanced silicate weathering of olivine. In: Proceedings of the National Academy of Sciences of the United States of America. vol.107, 20228–pp. 20233. doi: 10.1073/pnas.1000545107
Kotaś, J., and Stasicka, Z. (2000). Chromium occurrence in the environment and methods of its speciation. Environ. Pollut. 107, 263–283. doi: 10.1016/S0269-7491(99)00168-2
Lim, J. M., Salido, A. L., and Butcher, D. J. (2004). Phytoremediation of lead using Indian mustard (Brassica juncea) with EDTA and electrodics. Microchem. J. 76, 3–9. doi: 10.1016/j.microc.2003.10.002
Lone, M. I., He, Z. L., Stoffella, P. J., and Yang, X. E. (2008). Phytoremediation of heavy metal polluted soils and water: Progresses and perspectives. J. Zhejiang Univ. Sci. B. 9, 210–220. doi: 10.1631/jzus.B0710633
Ma, Q., Han, L., Zhang, J., Zhang, Y., Lang, Q., Li, F., et al. (2019). Environmental risk assessment of metals in the volcanic soil of Changbai mountain. Int. J. Environ. Res. Public Health. 16, 2047. doi: 10.3390/ijerph16112047
Macci, C., Doni, S., Peruzzi, E., Bardella, S., Filippis, G., Ceccanti, B., et al. (2013). A real-scale soil phytoremediation. Biodegradation. 24, 521–538. doi: 10.1007/s10532-012-9608-z
Mazhari, S. A., Sharifiyan Attar, R., and Haghighi, F. (2017). Heavy metals concentration and availability of different soils in Sabzevar area, NE of Iran. J. Afr. Earth Sci. 134, 106–112. doi: 10.1016/j.jafrearsci.2017.06.017
McMurtry, G. M., Wiltshire, J. C., and Kauahikaua, J. P. (1995). Heavy metal anomalies in coastal sediments of Oahu, Hawaii. Pacific Sci. 49, 452–470.
Memoli, V., Eymar, E., García-Delgado, C., Esposito, F., Santorufo, L., and De Marco, A. (2018). Total and fraction content of elements in volcanic soil: Natural or anthropogenic derivation. Sci. Total Environ. 625, 16–26. doi: 10.1016/j.scitotenv.2017.12.223
Mendoza-Grimón, V., Hernández-Moreno, J. M., Rodríguez Martín, J. A., Fernández-Vera, J. R., and Palacios-Díaz, M. P. (2014). Trace and major element associations in basaltic ash soils of El Hierro Island. J. Geochem. Explor. 147, 277–282. doi: 10.1016/j.gexplo.2014.06.010
Miranda, M., Benedito, J. L., Blanco-Penedo, I., López-Lamas, C., Merino, A., and López-Alonso, M. (2009). Metal accumulation in cattle raised in a serpentine-soil area: Relationship between metal concentrations in soil, forage and animal tissues. J. Trace Elements Med. Biol. 23, 231–238. doi: 10.1016/j.jtemb.2009.03.004
Mishra, K., and Tripathi, B. D. (2009). Accumulation of chromium and zinc from aqueous solutions using water hyacinth (Eichhornia crassipes). J. Hazard. Mater. 164, 1059–1063. doi: 10.1016/j.jhazmat.2008.09.020
Moosdorf, N., Renforth, P., and Hartmann, J. (2014). Carbon dioxide efficiency of terrestrial enhanced weathering. Environ. Sci. Technol. 48, 4809–4816. doi: 10.1021/es4052022
Nath, A. J., Lal, R., and Das, A. K. (2015). Managing woody bamboos for carbon farming and carbon trading. Global Ecol. Conserv. 3, 654–663. doi: 10.1016/j.gecco.2015.03.002
Néel, C., Soubrand-Colin, M., Piquet-Pissaloux, A., and Bril, H. (2007). Mobility and bioavailability of Cr, Cu, Ni, Pb and Zn in a basaltic grassland: comparison of selective extractions with quantitative approaches at different scales. Appl. Geochem. 22, 724–735. doi: 10.1016/j.apgeochem.2006.11.008
Pandharipande, S., and Gadpayle, P. (2016). Phytoremediation studies for removal of copper and chromium using azolla pinnata and water hyacinth. Int. J. Innov. Res. Sci. Eng. Technol. 5, 7078–7083. doi: 10.15680/IJIRSET.2016.0505064
Panneerselvam, B., and Priya, K. S. (2021). Phytoremediation potential of water hyacinth in heavy metal removal in chromium and lead contaminated water. Int. J. Environ. Analyt. Chem. 1–16. doi: 10.1080/03067319.2021.1901896
Peters, S. C., Blum, J. D., Driscoll, C. T., and Likens, G. E. (2004). Dissolution of wollastonite during the experimental manipulation of Hubbard Brook Watershed. Biogeochemistry. 67, 309–329. doi: 10.1023/B:BIOG.0000015787.44175.3f
Pogge von Strandmann, P. A. E., Renforth, P., West, A. J., Murphy, M. J., Luu, T. H., and Henderson, G. M. (2021). The lithium and magnesium isotope signature of olivine dissolution in soil experiments. Chem. Geol. 560, 120008. doi: 10.1016/j.chemgeo.2020.120008
Prado, C., Rodríguez-Montelongo, L., González, J. A., Pagano, E. A., Hilal, M., and Prado, F. E. (2010). Uptake of chromium by Salvinia minima: effect on plant growth, leaf respiration and carbohydrate metabolism. J. Hazard. Mater. 177, 546–553. doi: 10.1016/j.jhazmat.2009.12.067
Pulford, I. D., and Watson, C. (2003). Phytoremediation of heavy metal-contaminated land by trees - a review. Environ. Int. 29, 529–540. doi: 10.1016/S0160-4120(02)00152-6
Pulford, I. D., Watson, C., and McGregor, S. D. (2001). Uptake of chromium by trees: prospects for phytoremediation. Environ. Geochem. Health. 23, 307–311. doi: 10.1023/A:1012243129773
Quader, M. A., and Ahmed, S. (2017). Bioenergy with carbon capture and storage (BECCS): Future prospects of carbon-negative technologies. Elsevier Inc., p. 91–140. doi: 10.1016/B978-0-12-805423-9.00004-1
Ranieri, E., Tursi, A., Giuliano, S., Spagnolo, V., Ranieri, A. C., and Petrella, A. (2020). Phytoextraction from chromium-contaminated soil using moso bamboo in mediterranean conditions. Water, Air, Soil Pollut. 231, 1–12. doi: 10.1007/s11270-020-04759-9
Rascio, N., and Navari-Izzo, F. (2011). Heavy metal hyperaccumulating plants: How and why do they do it? And what makes them so interesting? Plant Sci. 180, 169–181. doi: 10.1016/j.plantsci.2010.08.016
Rathore, S. S., Shekhawat, K., Dass, A., Kandpal, B. K., and Singh, K. (2019). Phytoremediation mechanism in indian mustard (Brassica juncea) and its enhancement through agronomic interventions. Proc. Nation. Acad. Sci. India Section B – Biol. Sci. 89, 419–427. doi: 10.1007/s40011-017-0885-5
Renforth, P., von Strandmann, P. P., and Henderson, G. M. (2015). The dissolution of olivine added to soil: Implications for enhanced weathering. Appl. Geochem. 61, 109–118. doi: 10.1016/j.apgeochem.2015.05.016
Riddell-Black, D. (1994). Heavy metal uptake by fast growing willow species, in Willow vegetation filters for municipal wastewaters and sludges. A biological purification system. Uppsala: Swedish University of Agricultural Sciences, p. 145–151.
Rodriguez-Espinosa, P. F., Jonathan, M. P., Morales-García, S. S., Villegas, L. E. C., Martínez-Tavera, E., Muñoz-Sevilla, N. P., et al. (2015). Metal enrichment of soils following the April 2012–2013 eruptive activity of the Popocatépetl volcano, Puebla, Mexico. Environ. Monitor. Assess. 187, 1–7. doi: 10.1007/s10661-015-4938-z
Saha, P., Shinde, O., and Sarkar, S. (2017). Phytoremediation of industrial mines wastewater using water hyacinth. Int. J. Phytoremed. 19, 87–96. doi: 10.1080/15226514.2016.1216078
Shao, S., Driscoll, C. T., Johnson, C. E., Fahey, T. J., Battles, J. J., and Blum, J. D. (2016). Long-term responses in soil solution and stream-water chemistry at Hubbard Brook after experimental addition of wollastonite. Environ. Chem. 13, 528–540. doi: 10.1071/EN15113
Shukla, O. P., Juwarkar, A. A., Singh, S. K., Khan, S., and Rai, U. N. (2011). Growth responses and metal accumulation capabilities of woody plants during the phytoremediation of tannery sludge. Waste Manage. 31, 115–123. doi: 10.1016/j.wasman.2010.08.022
Singh, H. P., Mahajan, P., Kaur, S., Batish, D. R., and Kohli, R. K. (2013). Chromium toxicity and tolerance in plants. Environ. Chem. Lett. 11, 229–254. doi: 10.1007/s10311-013-0407-5
Smith, P., Adams, J., Beerling, D. J., Beringer, T., Calvin, K. V., Fuss, S., et al. (2019). Land-management options for greenhouse gas removal and their impacts on ecosystem services and the sustainable development goals. Ann. Rev. Environ. Resour. 44, 255–286. doi: 10.1146/annurev-environ-101718-033129
Strefler, J., Amann, T., Bauer, N., Kriegler, E., and Hartmann, J. (2018). Potential and costs of carbon dioxide removal by enhanced weathering of rocks. Environ. Res. Lett. 13, 034010. doi: 10.1088/1748-9326/aaa9c4
Taufikurahman, T., Pradisa, M. A. S., Amalia, S. G., and Hutahaean, G. E. M. (2019). Phytoremediation of chromium (Cr) using Typha angustifolia L., Canna indica L. and Hydrocotyle umbellata L. in surface flow system of constructed wetland: IOP Conference Series. Earth Environ. Sci. 308, 012020. doi: 10.1088/1755-1315/308/1/012020
Taylor, L. L., Driscoll, C. T., Groffman, P. M., Rau, G. H., Blum, J. D., and Beerling, D. J. (2021). Increased carbon capture by a silicate-treated forested watershed affected by acid deposition. Biogeosciences. 18, 169–188. doi: 10.5194/bg-18-169-2021
Taylor, L. L., Quirk, J., Thorley, R. M. S., Kharecha, P. A., Hansen, J., Ridgwell, A., et al. (2016). Enhanced weathering strategies for stabilizing climate and averting ocean acidification. Nat. Clim. Change. 6, 402–406. doi: 10.1038/nclimate2882
ten Berge, H. F. M., van der Meer, H. G., Steenhuizen, J. W., Goedhart, P. W., Knops, P., and Verhagen, J. (2012). Olivine weathering in soil, and its effects on growth and nutrient uptake in ryegrass (Lolium perenne L.): a pot experiment. PLoS ONE. 7, 1–8. doi: 10.1371/journal.pone.0042098
Teuchies, J., Jacobs, S., Oosterlee, L., Bervoets, L., and Meire, P. (2013). Role of plants in metal cycling in a tidal wetland: Implications for phytoremidiation. Sci. Total Environ. 445, 146–154. doi: 10.1016/j.scitotenv.2012.11.088
Thiffault, E., Hannam, K. D., Paré, D., Titus, B. D., Hazlett, P. W., Maynard, D. G., et al. (2011). Effects of forest biomass harvesting on soil productivity in boreal and temperate forests-A review. Environ. Rev. 19, 278–309. doi: 10.1139/a11-009
Tóth, G., Hermann, T., Da Silva, M. R., and Montanarella, L. (2016). Heavy metals in agricultural soils of the European Union with implications for food safety. Environ. Int. 88, 299–309. doi: 10.1016/j.envint.2015.12.017
Tra, H. T. L., and Egashira, K. (2001). Status of heavy metals in agricultural soils of Vietnam. Soil Sci. Plant Nutr. 47, 419–422. doi: 10.1080/00380768.2001.10408405
van der Ent, A., Baker, A. J. M., Reeves, R. D., Pollard, A. J., and Schat, H. (2013). Hyperaccumulators of metal and metalloid trace elements: facts and fiction. Plant and Soil. 362, 319–334. doi: 10.1007/s11104-012-1287-3
Vara Prasad, M. N., and de Oliveira Freitas, H. M. (2003). Metal hyperaccumulation in plants - Biodiversity prospecting for phytoremediation technology. Electr. J. Biotechnol. 6, 285–321. doi: 10.2225/vol6-issue3-fulltext-6
Verma, K., Tewari, S., and Rai, J. P. N. (2008). Ion exchange during heavy metal bio-sorption from aqueous solution by dried biomass of macrophytes. Bioresource Technol. 99, 1932–1938. doi: 10.1016/j.biortech.2007.03.042
Vlček, V., Jurička, D., and Míkov,á, J. (2017). Heavy metal concentration in selected soils and sediments of Livingston Island, Deception Island, King George Island, James Ross Island (Antarctica). Czech Polar Rep. 7, 18–33. doi: 10.5817/CPR2017-1-3
von Blanckenburg, F., Schuessler, J. A., Bouchez, J., and Frings, P. J. (2021). Rock weathering and nutrient cycling along an erodosequence. Am. J. Sci. 321, 1111–1163. doi: 10.2475/08.2021.01
Wang, H., Li, X., Chen, Y., Li, Z., Hedding, D. W., Nel, W., et al. (2020). Geochemical behavior and potential health risk of heavy metals in basalt-derived agricultural soil and crops: a case study from Xuyi County, eastern China. Sci Total Environ. 729, 139058. doi: 10.1016/j.scitotenv.2020.139058
Wei, Z., Van Le, Q., Peng, W., Yang, Y., Yang, H., Gu, H., et al. (2021). A review on phytoremediation of contaminants in air, water and soil. J. Hazard. Mater. 403, 123658. doi: 10.1016/j.jhazmat.2020.123658
Were, F. H., Wafula, G. A., and Wairungu, S. (2017). Phytoremediation using bamboo to reduce the risk of chromium exposure from a contaminated tannery site in Kenya. J. Health Pollut. 7, 12–25. doi: 10.5696/2156-9614-7.16.12
Wu, L. H., Luo, Y. M., Xing, X. R., and Christie, P. (2004). EDTA-enhanced phytoremediation of heavy metal contaminated soil with Indian mustard and associated potential leaching risk. Agric. Ecosyst. Environ. 102, 307–318. doi: 10.1016/j.agee.2003.09.002
Yadav, M., Singh, G., and Jadeja, R. N. (2021). Phytoremediation for heavy metal removal. Pollut. Water Manage. 128–150. doi: 10.1002/9781119693635.ch6
Zaranyika, M. F., and Ndapwadza, T. (1995). Uptake of Ni, Zn, Fe, Co, Cr, Pb, Cu and Cd by water hyacinth (eichhornia crassipes) in mukuvisi and manyame rivers, Zimbabwe: Journal of Environmental Science and Health. Part A: Environ. Sci. Eng. Toxicol. 30, 157–169. doi: 10.1080/10934529509376193
Zhang, X. H., Liu, J., Huang, H. T., Chen, J., Zhu, Y. N., and Wang, D. Q. (2007). Chromium accumulation by the hyperaccumulator plant Leersia hexandra Swartz. Chemosphere. 67, 1138–1143. doi: 10.1016/j.chemosphere.2006.11.014
Keywords: enhanced weathering, heavy metals, chromium, nickel, phytoprevention, phytoremediation, hyperaccumulating plants, basalt
Citation: Suhrhoff TJ (2022) Phytoprevention of Heavy Metal Contamination From Terrestrial Enhanced Weathering: Can Plants Save the Day? Front. Clim. 3:820204. doi: 10.3389/fclim.2021.820204
Received: 22 November 2021; Accepted: 20 December 2021;
Published: 17 January 2022.
Edited by:
Sara Vicca, University of Antwerp, BelgiumReviewed by:
Fatima Haque, University of Guelph, CanadaCopyright © 2022 Suhrhoff. This is an open-access article distributed under the terms of the Creative Commons Attribution License (CC BY). The use, distribution or reproduction in other forums is permitted, provided the original author(s) and the copyright owner(s) are credited and that the original publication in this journal is cited, in accordance with accepted academic practice. No use, distribution or reproduction is permitted which does not comply with these terms.
*Correspondence: Tim Jesper Suhrhoff, amVzcGVyLnN1aHJob2ZmQGVyZHcuZXRoei5jaA==
Disclaimer: All claims expressed in this article are solely those of the authors and do not necessarily represent those of their affiliated organizations, or those of the publisher, the editors and the reviewers. Any product that may be evaluated in this article or claim that may be made by its manufacturer is not guaranteed or endorsed by the publisher.
Research integrity at Frontiers
Learn more about the work of our research integrity team to safeguard the quality of each article we publish.