- 1ASRC Federal Data Solutions, Contractor to the U.S. Geological Survey (USGS) Earth Resources Observation and Science (EROS) Center, Sioux Falls, SD, United States
- 2U.S. Geological Survey (USGS) Earth Resources Observation and Science (EROS) Center, Sioux Falls, SD, United States
California has, in recent years, become a hotspot of interannual climatic variability, recording devastating climate-related disturbances with severe effects on tree resources. Understanding the patterns of tree cover change associated with these events is vital for developing strategies to sustain critical habitats of endemic and threatened vegetation communities. We assessed patterns of tree cover change, especially the effects of the 2012–2016 drought within the distribution range of blue oak (Quercus douglasii), an endemic tree species to California with a narrow geographic extent. We utilized multiple, annual land-cover and land-surface change products from the U.S. Geological Survey (USGS) Land Change Monitoring, Assessment and Projection (LCMAP) project along with climate and wildfire datasets to monitor changes in tree cover state and condition and examine their relationships with interannual climate variability between 1985 and 2016. Here, we refer to a change in tree cover class without a land-cover change to another class as “conditional change.” The unusual drought of 2012–2016, accompanied by anomalously high temperatures and vapor pressure deficit, was associated with exceptional spikes in the amount of both fire and non-fire induced tree cover loss and tree cover conditional change, especially in 2015 and 2016. Approximately 1,266 km2 of tree cover loss and 617 km2 of tree cover conditional change were recorded during that drought. Tree cover loss through medium to high severity fires was especially large in exceptionally dry and hot years. Our study demonstrates the usefulness of the LCMAP products for monitoring the effects of climatic extremes and disturbance events on both thematic and conditional land-cover change over a multi-decadal period. Our results signify that blue oak woodlands may be vulnerable to extreme climate events and changing wildfire regimes. Here, we present early evidence that frequent droughts associated with climate warming may continue to affect tree cover in this region, while drought interaction with wildfires and the resulting feedbacks may have substantial influence as well. Consequently, efforts to conserve the blue oak woodlands, and potentially other vegetation communities in the Western United States, may benefit from consideration of climate risks as well as the potential for climate-fire and vegetation feedbacks.
Introduction
Compounding effects from more frequent climatic extremes and changing disturbance regimes are increasing the probability of widespread transformation of terrestrial ecosystems, prompting the need for more improved assessments in support of management efforts as these threats are expected to amplify across vegetation communities worldwide. California has in recent years become a hotspot of interannual climatic variability, recording several climate-related disturbances with severe effects on tree resources and critical habitats of endemic and threatened species and the wildlife that depends on them. In particular, California's drought of 2012–2016 has been reported as one of the most consequential droughts in over a century (Griffin and Anchukaitis, 2014; Robeson, 2015; Lund et al., 2018). Millions of trees suffered severe effects of the drought including extensive crown defoliation, foliage die-back, and massive die-offs (Byer and Jin, 2017; Paz-Kagan et al., 2017; Stephenson et al., 2018). Recently, wildfires have been increasing at an alarming rate in the western United States (Westerling, 2016). Consequently, this severe historical drought in California (Lund et al., 2018) exacerbated fire activity, leading to more tree deaths. Understanding the patterns of tree cover change associated with extreme climate events is vital for developing mitigation and adaptation strategies to sustain ecosystem services and for projecting future effects of climate and land-use change on vegetation communities.
Following the 2012–2016 drought, several studies have examined effects of the drought on forests and woodlands across California. Pile et al. (2019) reported that the drought and bark beetle outbreaks resulted in elevated tree mortality across the southern Sierra Nevada, with small-sized pine trees the hardest hit. According to Fettig et al. (2019) nearly 49% of trees died within a network of national forests across central and southern Sierra Nevada between 2014 and 2017. From various accounts, the 2012–2016 drought was a warmer drought, meaning that the drought occurred under warmer than historically normal temperatures (Lund et al., 2018; Goulden and Bales, 2019; Field et al., 2020). This warmer drought, which lasted several years, triggered excessive deep soil drying and extreme moisture stress in trees leading to massive tree deaths throughout the Sierra Nevada (Asner et al., 2016; Goulden and Bales, 2019; Madakumbura et al., 2020). On California's Santa Cruz Island, the drought resulted in extensive die-off of bishop pine (Pinus muricata D. Don) forests (Taylor et al., 2019).
However, the distribution range of blue oak (Quercus douglasii Hook. & Arn.) has received far less attention, limiting our ability to understand how this iconic native vegetation community may respond to warming climate and changing wildfire regimes. Like elsewhere in the state, the range of blue oak, an endemic tree species to California with a narrow geographic extent, has in recent decades been experiencing more severe droughts with warmer climate, increased land-use change, wildfires, and insect-pest outbreaks. However, species with a narrow range of distribution, such as blue oak, are of particular conservation concern because they are increasingly threatened by climate warming (Morueta-Holme et al., 2010; Cartwright, 2019). Blue oak woodlands are a rare remnant of old-growth endemic woodlands and one of the largest and most biologically diverse ecosystems of California (McDonald, 1990; Reiner and Craig, 2011; Stahle et al., 2013). Despite the importance of this unique ecosystem, there is a lack of information on the negative consequences of the recent drought on tree cover as well as the long-term patterns of tree cover change in the area. A more recent study on the drought's effect on tree mortality was limited to a small subset of blue oak woodlands (Das et al., 2020). In a 2017 post-drought survey in the Sequoia National Park, Das et al. (2020) estimated that 19% of standing blue oak and 14% of all trees were killed during that drought, with blue oak basal area dropping by 26%. Meanwhile, landscape-wide studies using bioclimate modeling to project the vulnerability of blue oak distribution relied on climate and hydrologic factors, precluding important factors such as wildfire disturbance (Kueppers et al., 2005; Brown et al., 2018).
Disturbance and extreme climatic events may lead to changes in tree cover state or condition, depending on the type, and intensity of the event. While tree cover conditional change denotes a change in tree cover class without a conversion to another land-cover class, such change may render tree cover more susceptible to state change upon subsequent disturbances, even of lower intensity. Therefore, to better understand the effects of climatic extremes and disturbance events on woodlands, it is critical to identify both where these events lead to complete tree cover loss and where they lead to conditional change. Nonetheless, assessment of these types of change are often constrained by lack of data products capable of detecting both land-cover and land-surface change consistently in space and time. To overcome this data constraint, we leveraged a new land-cover and land-surface change dataset from the U.S. Geological Survey (USGS) Land Change Monitoring, Assessment and Projection (LCMAP) project to identify annual tree cover declines, emphasizing both tree cover loss and tree cover conditional change. The annual LCMAP Collection 1.0 data suite, spanning 1985–2017, offers new opportunity to detect changes in land cover across the conterminous United States (CONUS) at a 30 × 30 m spatial resolution over a multi-decadal time span (Brown et al., 2020). In particular, multiple LCMAP products can be integrated to monitor not only thematic change but also conditional change in land cover. Hence, LCMAP presents a unique and exciting opportunity for embarking on medium-to-long-term ecological studies probing different types of change in land cover that accompany disturbances and climate variability.
Changes in tree cover are not only related to but are also indicators of important ecological processes including tree mortality, reduced productivity, loss of tree-held carbon, and ecosystem degradation. Therefore, assessing patterns of tree cover change and their linkages to drought and drought-related disturbances such as wildfire is a prerequisite for developing effective strategies to conserve the blue oak woodlands ecosystem. To this end, we combined LCMAP datasets along with climate and wildfire datasets to monitor changes in tree cover state and tree cover condition and examine their relationships with interannual climate variability for the 1985–2016 study period. With emphasis on the recent statewide extreme drought of 2012–2016 (Goulden and Bales, 2019; Pile et al., 2019), we specifically addressed the following research questions:
• How did patterns of tree cover loss and tree cover conditional change within blue oak species range during the 2012–2016 drought compare with those of other years in the study period?
• Was the 2012–2016 drought associated with unusual patterns in fire-induced and non-fire related tree cover loss and tree cover conditional change?
• What are the interannual relationships between climate and both fire-induced and non-fire related tree cover loss and tree cover conditional change?
• What are the relationships between fire-induced tree cover loss and non-fire tree cover loss and conditional change?
Materials and Methods
Study Area
Our study area encompassed the blue oak woodlands of California (Figure 1). This landscape, which is dominated by Quercus douglasii Hook. & Arn., is an endemic woodland and one of the largest and most biologically diverse ecosystems in California (Reiner and Craig, 2011; Stahle et al., 2013). In an earlier forest inventory report, Waddell and Barrett (2005) found that blue oak woodland was the most common hardwood forest type in California. Nonetheless, as Stahle et al. (2013) rightly pointed out this unique ecosystem has received far less attention than their neighboring conifer forests. Blue oak woodlands, measuring ~1.2 million hectares, cover a mosaic of woodland and savanna that encircles California's Central Valley; and the species are distributed along the foothill portions of the Cascades, Sierra Nevada, and Coast Ranges (McDonald, 1990; Waddell and Barrett, 2005). This landscape thus constitutes the lower forest border between grasslands in the valley and mid-slope conifer forests, with a fairly wide elevation ranging from 50–1,800 m (McDonald, 1990; Stahle et al., 2001).
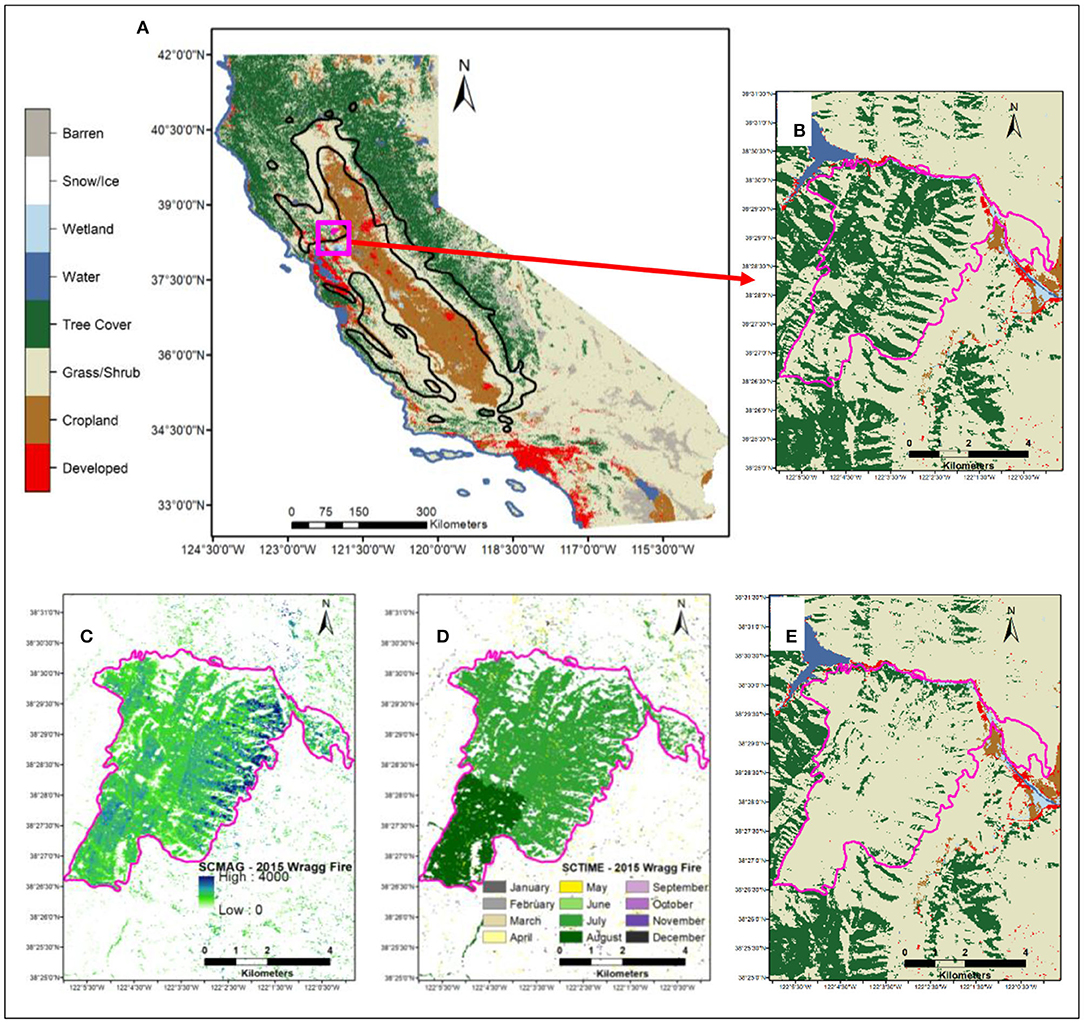
Figure 1. Map of study area showing: (A) LCMAP Primary Land Cover (LCPRI,) of 1985 of California indicating location of blue oak species range (black boundary); (B) LCPRI of 2014 (pre-fire land cover) zoomed to location of the Wragg fire of 2015 (pink polygon, from MTBS fire perimeter database); (C) LCMAP Change Magnitude (SCMAG) indicating spectral change and the degree of change associated with the Wragg fire of 2015; (D) LCMAP Time of Spectral Change (SCTIME) indicating timing of spectral change (day of year) of CCDC model breaks following the Wragg fire in 2015; (E) LCPRI of 2016 (post-fire land cover) indicating tree cover loss to grass/shrub following the Wragg fire of 2015 (pink polygon, from MTBS fire perimeter database).
The area has a Mediterranean climate characterized by hot dry summers and cool wet winters. Most precipitation typically occurs between October and March (McDonald, 1990). As a slow growing, winter deciduous tree, blue oak grows on dry, loamy, gravelly, or rocky soils with an understory dominated by annual grasses and forbs (McDonald, 1990; Waddell and Barrett, 2005). Despite historical threats mainly from clearing and grazing, canopy-dominants aging 150 to more than 400 years are still common in remnant old-growth blue oak landscapes, especially on rugged terrain in remote areas (Stahle et al., 2001; Reiner and Craig, 2011). The species range has been projected to decrease considerably because of reduced precipitation, climate warming, and regeneration constraints (Kueppers et al., 2005; Davis et al., 2016; Brown et al., 2018). As a result, the threat posed by the changing climate including frequent climatic extremes such as droughts and their interactions with disturbances (e.g., wildfires) will remain a significant challenge for conservation of the study area.
Data
Land-Cover and Land-Change Dataset
For this study, land cover and land change data at an annual frequency were important for determining the location and timing of change on a yearly basis. We obtained land cover and land surface change products from the LCMAP initiative, Collection 1.0, from the USGS Earth Resources Observation and Science (EROS) Center. LCMAP products are based on the USGS implementation of the Continuous Change Detection and Classification (CCDC) algorithm (Zhu and Woodcock, 2014) on the Landsat archive (Collection 1) to monitor land cover and land-cover change (Brown et al., 2020). The LCMAP product suite has 10 science datasets consisting of five land cover and five land surface change products (https://doi.org/10.5066/P9W1TO6E). The land cover products are named Primary Land Cover (LCPRI), Land Cover Change (LCACHG), Primary Land Cover Confidence (LCPCONF), Secondary Land Cover (LCSEC), and Secondary Land Cover Confidence (LCSCONF). The land surface change products are named Time of Spectral Change (SCTIME), Change Magnitude (SCMAG), Time Since Last Change (SCLAST), Spectral Stability Period (SCSTAB), and Spectral Model Quality (SCMQA). Detailed description of these products can be found in Brown et al. (2020). In this study we used LCPRI, LCACHG, LCPCONF, SCTIME, and SCMAG.
In this study, Tree Cover describes a class in the land cover legend of LCMAP, which is often called “forest” in other mapping efforts. We used the annual LCPRI product to identify the tree cover class and the LCACHG indicated how much and where tree cover changed each year and the land cover categories that tree cover changed into when there was tree cover loss. For each calendar year, we obtained tree cover loss from the LCACHG product by selecting all pixels that changed from tree cover class to a new land cover class that year. In this study, we use the term tree cover conditional change to describe a change in tree cover condition, indicated by the spectral change product (SCMAG), without a land cover change to another class. Tree cover conditional change could result from thinning, windthrow, insect attacks, moisture stress, wildfire, and other disturbances that reduce tree cover but not to below the 10% threshold tree cover used in the LCMAP classification of tree cover class. For each calendar year, we obtained tree cover conditional change by selecting tree cover pixels with SCMAG values >0 for which the LCACHG indicates no class change before and after the CCDC model break. We also used LCACHG to obtain tree cover gain for each calendar year by selecting pixels that changed from other class to tree cover class that year. We spatially overlaid the SCTIME, which has the time of change information per the CCDC model break, to identify the day of change for each changed pixel. Changed pixels in tree cover–grass/shrub transitional segments do not have day of change information in the SCTIME product because the transition is more gradual with no one specific time. We spatially overlaid the LCPCONF with the LCACHG product to obtain changed pixels in the transitional segments. In the LCPCONF product, tree cover gain and tree cover loss have codes 151 and 152, respectively. Therefore, tree cover change for each calendar year comprised change identified by both the CCDC model breaks and change in the tree cover–grass/shrub transitional segments.
Fire Dataset
We obtained fire perimeter and burn severity data for all large wildfires (> 404 ha) from the Monitoring Trends in Burn Severity (MTBS, http://www.mtbs.gov/) project (Eidenshink et al., 2007). Timing of fires were obtained from the fire ignition and extinction dates in the MTBS fire perimeter data. We rasterized the fire perimeter polygon shapefile to obtain burn date information for each burned pixel. Fire severity is a measure of the degree of lethal effects of fire on vegetation such as mortality and biomass consumption; and on soil such as ash, char, and mineral soil. MTBS maps fire severity based on Landsat derived delta normalized burn ratio (dNBR) that compares post- and pre-fire conditions. MTBS burned area categorizes fire severity into six classes, 1–6. We only included in our analysis fire severity classes 2–4, denoting low severity—class 2, medium severity—class 3, and high severity–class 4. Eidenshink et al. (2007) provide details on pre-processing and specific information on burn severity classification in MTBS.
We partitioned annual tree cover loss and tree cover conditional change into those related to fire and non-fire causes by overlaying with burned area information from the MTBS burned area data. Thus, rather than analyze annual area burned we looked at the area of tree cover loss and tree cover conditional change by fire, which could be interpreted as proxies for tree mortality. We overlaid the tree cover loss and day of change (SCTIME) layers with the MTBS burned area and burn date raster layers to obtain fire-induced tree cover loss for each calendar year from 1985 to 2016. Tree cover loss pixels were labeled as fire-induced if the SCTIME occurs on or after the MTBS fire start date. Because tree cover loss from the tree cover-grass/shrub transitional segments do not have day of change information, pixels were labeled as fire-induced if they spatially coincided with the burned pixels in the MTBS burn area data for each year. The same procedure above was followed to obtain fire-induced tree cover conditional change. In both cases we included change one-year post-fire to account for delayed tree mortality after fire. Similarly, we overlaid fire-induced tree cover loss (and tree cover conditional change) with MTBS burn severity to obtain tree cover loss (and tree cover conditional change) by fire severity class for calendar each year. A flowchart summarizing the entire process for generating fire-induced and non-fire related tree cover loss and tree cover conditional change is shown in Figure 2.
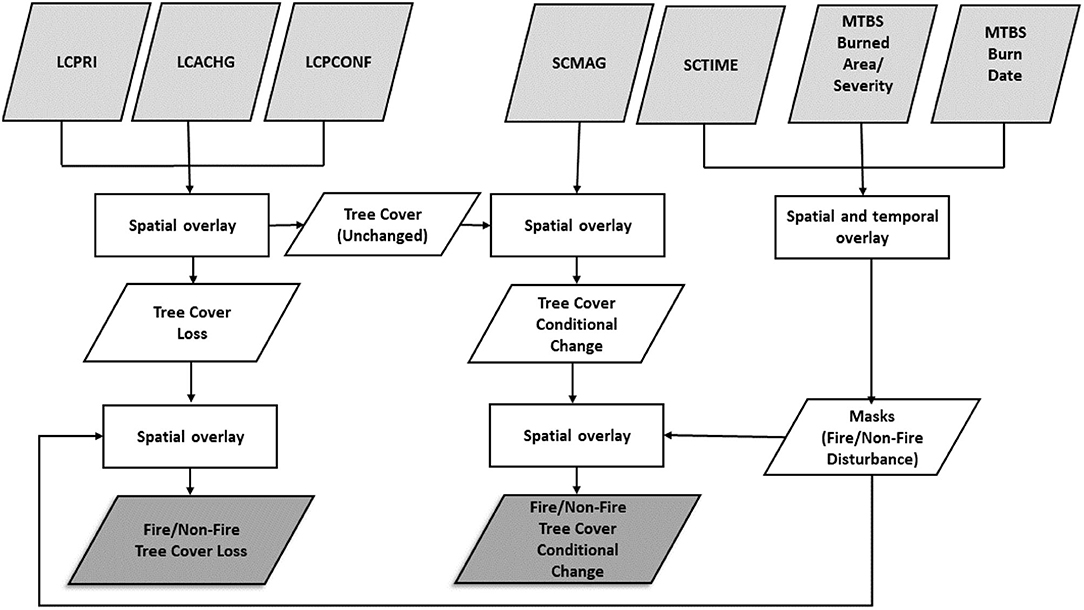
Figure 2. A flowchart of methods to generate fire-induced and non-fire related tree cover loss and tree cover conditional change from LCMAP annual land-cover and land-surface change products and Monitoring Trends in Burn Severity (MTBS) wildfire datasets. LCPRI, primary land cover; LCACHG, land cover change; LCPCONF, primary land cover confidence; SCTIME, time of spectral change; SCMAG, change magnitude.
Climate Data
We used a monthly gridded meteorological dataset at 1/24° spatial resolution (≈ 4 km) of precipitation, maximum vapor pressure deficit (VPDmax), and maximum temperature (Tmax) from Parameter-elevation Regression on Independent Slopes Model (PRISM, https://prism.nacse.org/; Christopher et al., 2002). We obtained a monthly gridded dataset of climatic water deficit at 1/120° spatial resolution (≈800 m) based on methods described by Dobrowski et al. (2013). Inputs for the climatic water deficit data were PRISM—derived temperature, precipitation, and humidity, soil water holding capacity from POLARIS (Chaney et al., 2016), and 10-m wind and downward shortwave radiation from the North American Land Data Assimilation System (NLDAS2; Mitchell et al., 2004).
Boundary Shapefile of Blue Oak Species Range
Boundary shapefile of blue oak species range was obtained from Digital Representations of Tree Species Range Maps from “Atlas of United States Trees” (https://web.archive.org/web/20170127093428/https://gec.cr.usgs.gov/data/little/).
Analysis Methods
Comparing Patterns of Tree Cover Loss and Tree Cover Conditional Change During the 2012–2016 Drought With Other Years
To characterize interannual climate variability, we used the PRISM climate datasets to calculate annual and seasonal summaries and standardized climatic anomalies. Climate anomalies are effective indicators of regional meteorological fluctuations, including droughts, by eliminating the underlying spatial variability in long-term climate data and emphasizing the temporal deviations. We calculated standardized climatic anomalies as the ratio of anomaly to the standard deviation of the climatological mean. The climatological mean was based on the reference period 1982–2011. Summarized climate variables consisting of total precipitation, mean maximum vapor pressure deficit, mean maximum temperature, and mean climatic water deficit for the growing season (June–August), winter (December–February), fire season (May–October), calendar year (January–December) and the water year. The water year was defined as the 12-month period from Oct 1st through September 30th of the following year. All climate variables were calculated for each PRISM grid cell and then summarized for the entire study area.
We summarized annual changes in tree cover across the study area for the period 1985–2016. We lacked stratified sample reference data to calculate statistically based area estimates; henceforth all our area estimates of tree cover change were based on pixel count only in the LCMAP land-cover dataset. Annual tree cover estimates were graphed along with standardized anomalies of the four climate variables; climatic water deficit, maximum temperature, precipitation amount, and vapor pressure summarized for each year 1985–2016.
Patterns of Fire-Induced and Non-fire Tree Cover Loss and Tree Cover Conditional Change During 2012–2016 Drought
We generated time series graphs of non-fire and fire-related tree cover loss and tree cover conditional change for the study area. We also generated time series maps of climate anomalies to indicate the spatial pattern of drought across blue oak species range during the 2012–2016 drought.
Interannual Relationships Between Climate and Both Fire-Induced and Non-fire Tree Cover Loss and Conditional Change
To determine the relationships between interannual climate variability and tree cover declines, we used Pearson rank correlation to assess the association between annual climate anomalies and annual area of both fire-induced and non-fire tree cover loss and conditional change across the study area. Correlations were significant at p-values ≤ 0.05.
Relationships Between Fire-Induced Tree Cover Loss and Non-fire Tree Cover Loss and Conditional Change
We used Pearson rank correlation to determine if annual area of fire-induced tree cover loss was associated with non-fire tree cover loss and conditional change. Correlations were significant at p-values ≤ 0.05.
To characterize the spatial pattern of tree cover declines during the drought period, we counted pixels of tree cover in 2011 that changed to tree cover loss and tree cover conditional change in each year during that period. We summarized these changed pixels as a percent of the pre-drought tree cover pixel count in 2011 for each grid cell and each year. Grid cells measured 1/120° spatial resolution (≈800 m) corresponding to raster resolution of the PRISM-based climatic water deficit. We evaluated if the spatial patterns of fire-induced tree cover loss during the most consequential fires of 2015 (the largest and the most widespread fire of the study period) were related to the pattern of non-fire tree cover loss and non-fire tree cover conditional change during the drought. We summarized our gridded percent non-fire tree cover loss from 2012 to 2014 to obtain mean percent non-fire tree cover loss per grid cell before the fire. Similarly, we summarized our gridded percent non-fire tree cover conditional change from 2012 to 2014 to obtain mean percent of non-fire tree cover conditional change per grid cell. We then used Spearman rank correlation to test for associations between percent fire-induced tree cover loss of 2015 and both percent non-fire tree cover loss and non-fire tree cover conditional change.
Results
Comparing Patterns of Tree Cover Loss and Tree Cover Conditional Change During the 2012–2016 Drought With Other Years
The proportion of the study area that experienced tree cover decline varied considerably throughout the 32-year study period (1985–2016). The study area experienced prolonged drought conditions during 2012–2016, with higher temperatures and vapor pressure deficit as well as lower precipitation and intensification of moisture stress. These drought conditions were more severe in 2014–2015 (Figure 3A). This multi-year severe drought was associated with exceptionally high levels of tree cover loss and tree cover conditional change, particularly in 2015 and 2016 (Figure 3B). Among all the seasons over which our climate data were summarized, we generally found the strongest relationship between annual tree cover change and climate anomalies summarized over the water year. Thus, hereafter all results of climate anomalies refer to the water year throughout rest of this paper.
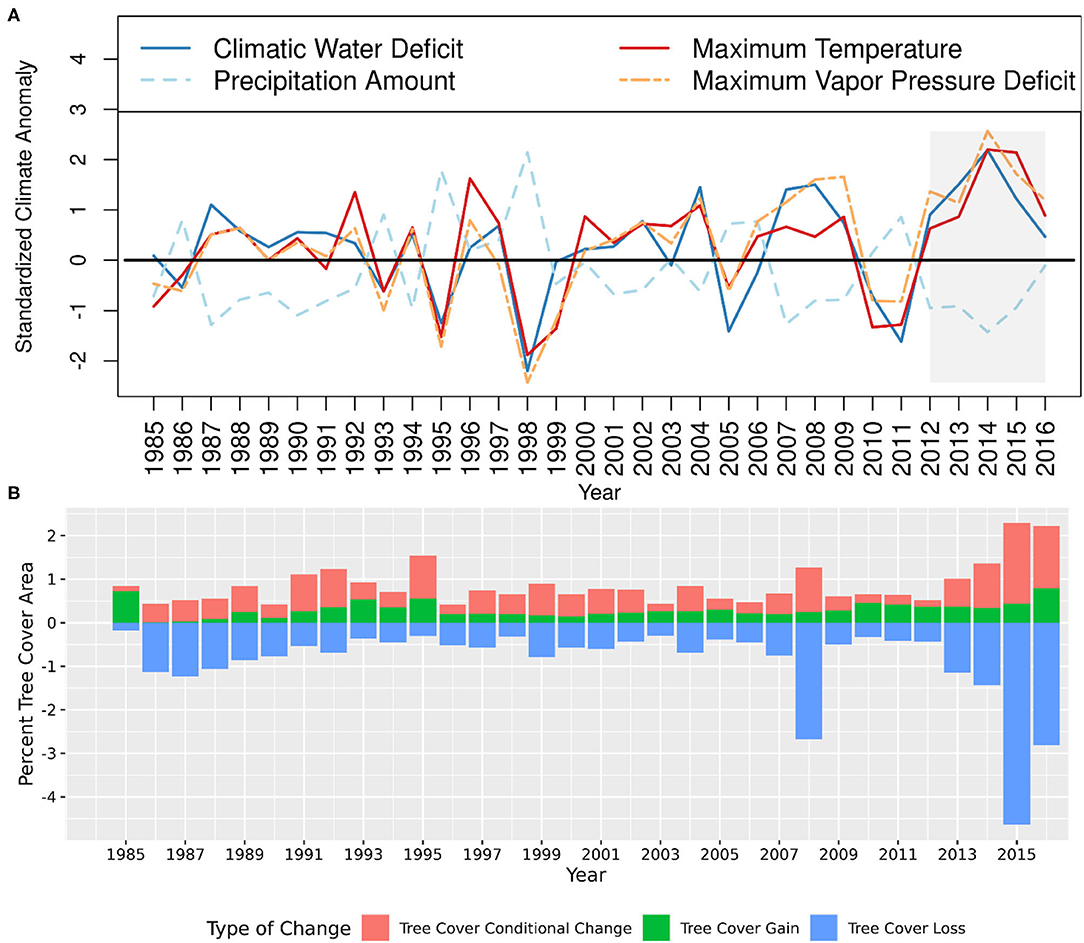
Figure 3. (A) Annual time series of standardized climate anomalies across blue oak woodlands, 1985–2016. Note the prolonged drying and warming beginning 2012; and (B) Interannual variability in the amount of tree cover change across blue oak woodlands, 1985–2016. Tree cover change were derived from LCMAP products. Percent tree cover area on y-axis is the area of change expressed as a percentage of the original tree cover area in 1985.
For the entire study period, the mean annual tree cover loss, as a percentage of the base year tree covered area in 1985, was 0.9% year−1. During the 2012–2016 drought, the mean annual tree cover loss was 2.1%, reaching 4.6 and 2.8% in 2015 and 2016, respectively (Figure 3B). Thus, tree cover loss in 2015 and 2016 were ~5.2 and 3.2 times the longer-term mean. According to the land-cover maps, a cumulative 1,266 km2 of tree cover was lost during the 5-year drought, which constitutes ~37% of all tree cover loss during the 32-year study period.
Similarly, the mean annual tree cover conditional change, as a percentage of the original tree covered area in 1985, was 0.6% year−1 during the study period. However, during the 2012–2016 drought, the mean annual tree cover conditional change was 1.0%, reaching 1.9% and 1.4% in 2015 and 2016, respectively (Figure 3B). Hence, tree cover conditional change in 2015 and 2016 were ~3.3 and 2.6 times the longer-term mean, respectively. According to the land-cover maps, ~617 km2 tree cover conditional change were recorded during the five-year drought, which constitutes ~28% of all tree cover conditional change during the 32-year study period. The years 1987–1991 and 2007–2009 were also identified as drought years but with lesser drought intensity and relatively less decline in tree cover (Figures 3A,B). The 2007–2009 drought was associated with the third highest record of tree cover loss during the study period.
Patterns of Fire-Induced and Non-fire Tree Cover Loss and Tree Cover Conditional Change During 2012–2016 Drought
Spatial patterns of the standardized climatic anomalies indicate that the drought and hotter conditions of 2012–2016 were widespread throughout blue oak woodlands (Figure 4). At the peak of the drought, in 2014 and 2015, standardized anomalies of climatic water deficit, maximum temperature, and maximum vapor pressure deficit exceeded twice their longer-term means (Figures 3, 4).
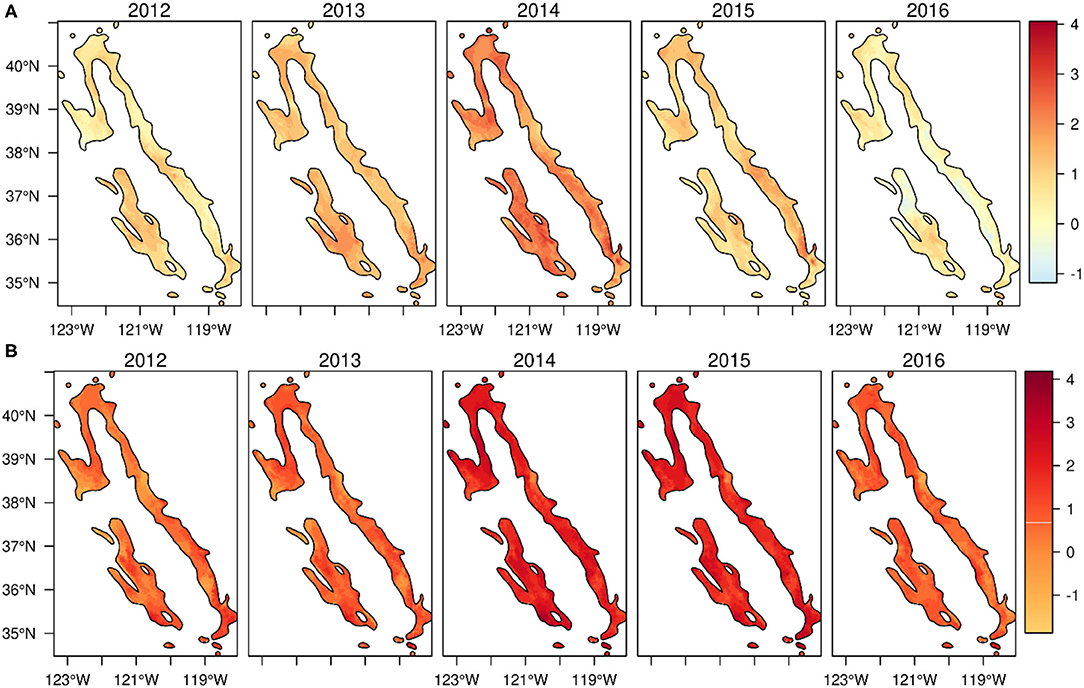
Figure 4. Time series maps showing the spatial pattern of standardized anomaly (z-score) of (A) climatic water deficit (PRISM 800-m resolution) and (B) maximum temperature (PRISM 4-km resolution) across blue oak woodlands of California during 2012 and 2016.
This hotter drought was accompanied by sharp increases in both fire and non-fire related tree cover loss and tree cover conditional change, with all of them recording their largest annual area in 2015 (Figure 5). From 2012 to 2016 there were sustained increases in non-fire tree cover loss and conditional change, both attaining their maximum values in 2015. In contrast, fire-induced tree cover loss remained low until a sudden jump to a record high in 2015, and then a slight decrease in 2016. The 2015 spike in fire-induced tree cover loss, measuring ~330 km2, distinctly contrasts that of any other year. Although 1987–1991 and 2007–2009 also experienced drought, the former drought events were associated with such relatively less declines in tree cover by fire. Nonetheless, the 2007–2009 drought was associated with considerable increase in fire-induced tree cover loss particularly in 2008 (Figure 5). This spike, which is the second largest in pixel-count area of annual fire-induced tree cover loss, measured ~245 km2 and represents about 2.0% of the tree covered area in the 1985 base year. Annual area of non-fire tree cover loss was generally greater than fire-induced tree cover loss, except in 2008 when the area of fire-induced tree cover loss (245 km2) was substantially greater than non-fire tree cover loss (95 km2).
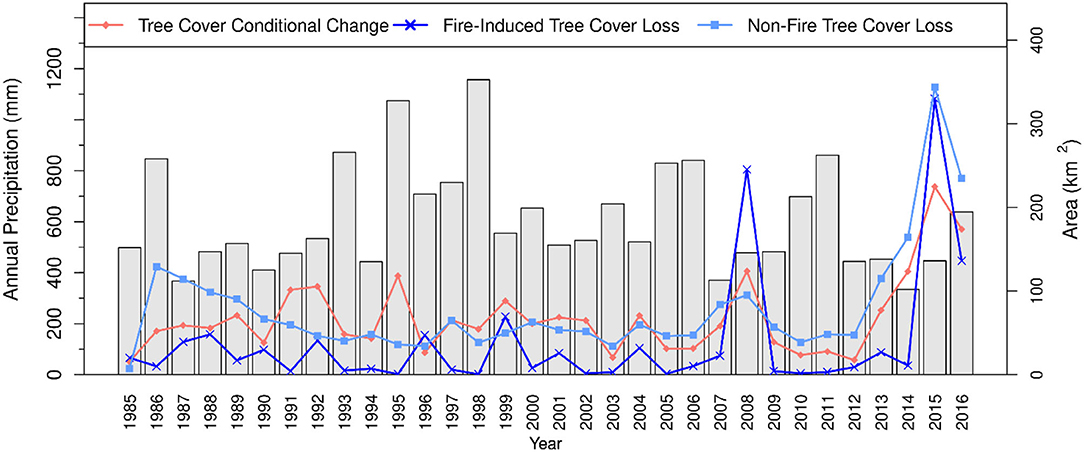
Figure 5. Time series plot showing non-fire and fire-related tree cover change and their interrelationships with interannual precipitation variability across blue oak woodlands, 1985–2016.
Interannual Relationships Between Climate and Both Fire-Induced and Non-fire Tree Cover Loss and Conditional Change
Figure 6 shows the relationships between annual time series climatic anomalies and both fire-induced and non-fire tree cover loss and tree cover conditional change. Annual area of fire-induced tree cover loss had a moderate positive association with water year climatic water deficit, maximum temperature, and maximum vapor pressure deficit (r = 0.35, 0.36, and 0.39, respectively). Similarly, annual area of fire-induced tree cover loss by medium-high fire severity had a moderate positive association with climatic water deficit, maximum temperature, and maximum vapor pressure deficit (r = 0.35, 0.35, and 0.39, respectively). We observed remarkably large increases in tree cover loss through medium—high severity fires that were coincident with anomalously drier and hotter years of 2008, 2015, and 2016 (Figure 7). In 2015, the area of fire-induced tree cover loss by medium—high severity fires were distinctly high, amounting to ~2.3% (280 km2) of the total tree covered area in the 1985 base year (Figure 7). We found moderate positive association between annual area of fire-induced tree cover conditional change and climatic water deficit only (r = 0.41). Annual area of non-fire tree cover loss had a moderate positive association with climatic water deficit, maximum temperature, and maximum vapor pressure deficit (r = 0.41, 0.5, and 0.47, respectively). Annual area of non-fire tree cover conditional change had a moderate positive association only with maximum temperature (r = 0.36). All correlations were significant at p-values < 0.05.
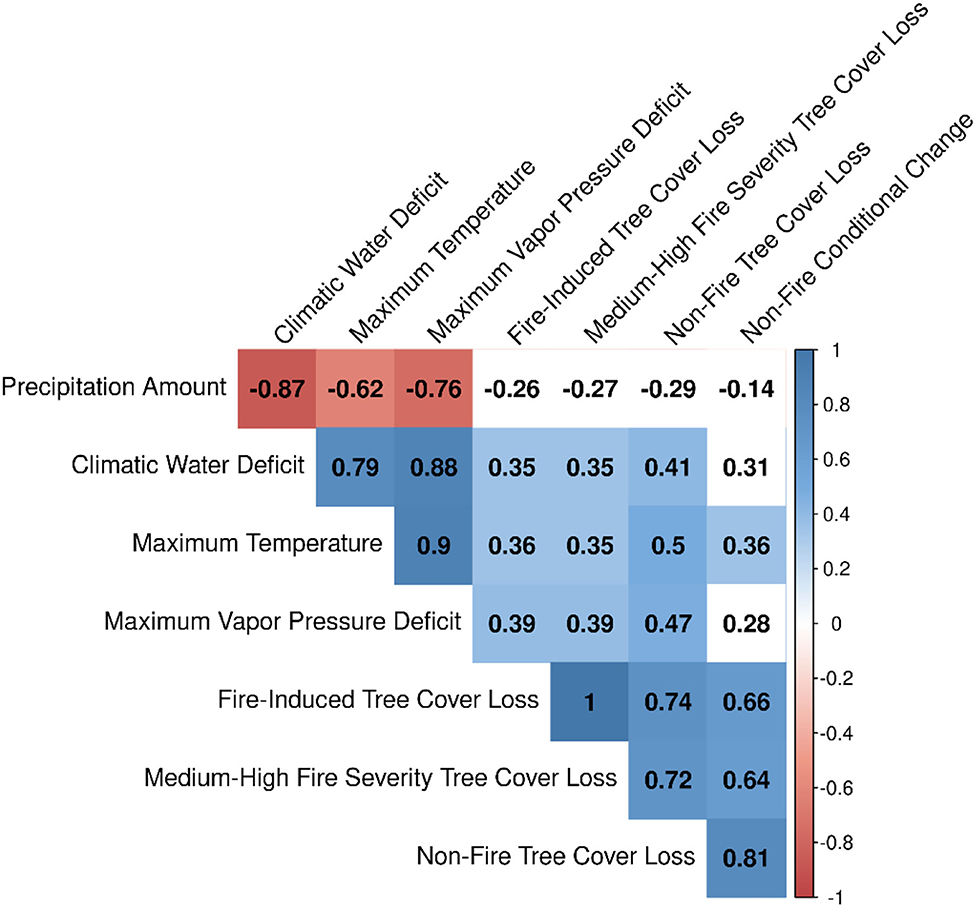
Figure 6. Correlation matrix showing associations among annual area of tree cover change and climate anomalies for the period 1985–2016. Correlations were significant at p-values <0.05. Relationships that are non-significant are colored in white.
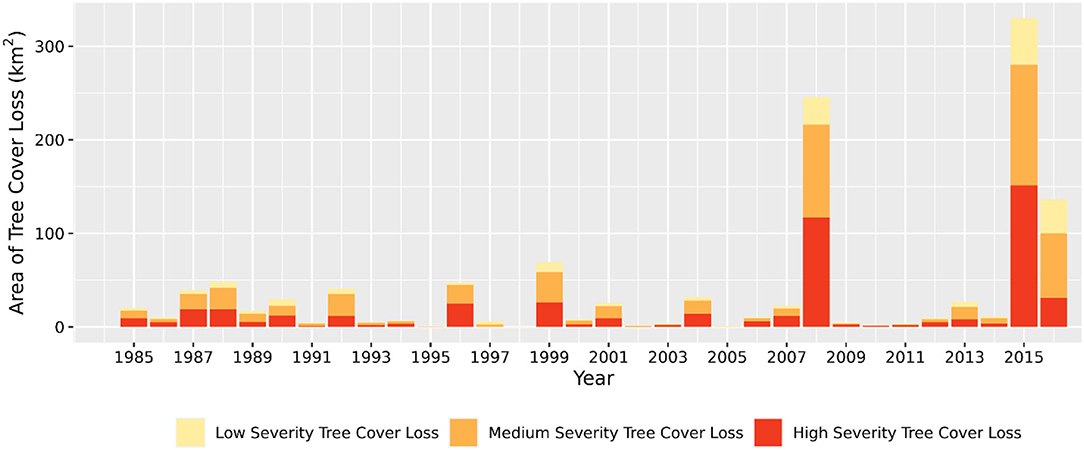
Figure 7. Interannual variability in fire-induced tree cover loss showing sudden increases in tree cover loss by medium–high severity fires in 2008, 2015, and 2016 across blue oak woodlands. Percent tree cover area on y-axis is the area of change expressed as a percentage of the original tree cover area in 1985.
Relationships Between Fire-Induced Tree Cover Loss and Non-fire Tree Cover Loss and Conditional Change Across Blue Oak Woodlands
The annual area of fire-induced tree cover loss was positively correlated with annual area of both non-fire tree cover loss (r = 0.74, p < 0.05) and non-fire tree cover conditional change (r = 0.66, p < 0.05, Figure 6). During the 2012–2016 drought, non-fire tree cover loss and tree cover conditional change were spatially widespread, starting from 2012 and gradually expanding through most of the study area by 2016 (Figure 8). Fire-induced tree cover loss was mainly clustered in few isolated locations along the eastern, the north-western and south-western portions across the landscape. The Spearman rank correlation between gridded percent fire-induced tree cover loss and both percent non-fire tree cover loss and non-fire tree cover conditional change were not significant (r =-0.19 and −0.06, respectively).
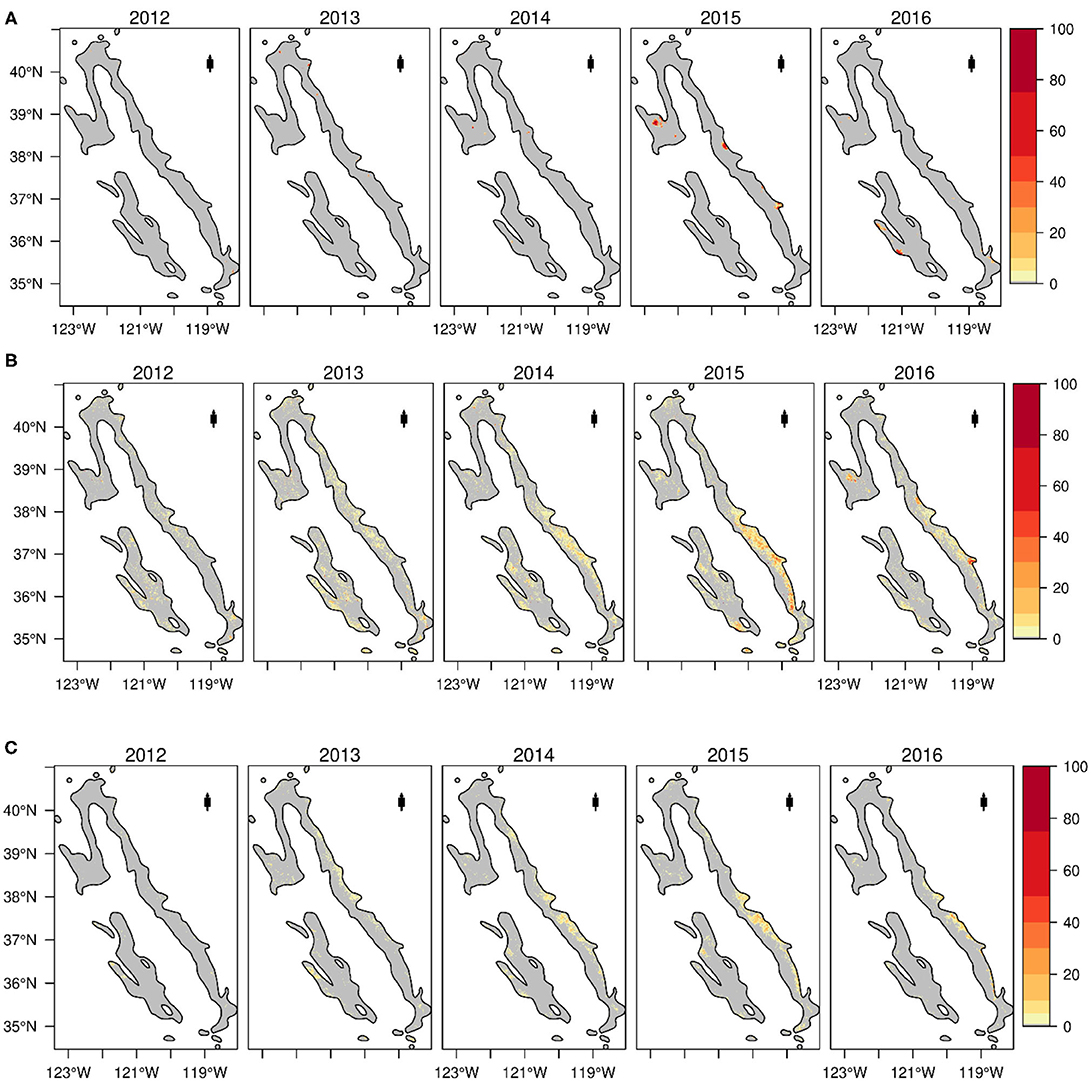
Figure 8. Time series maps showing the spatial pattern of (A) Percent fire-induced tree cover loss; (B) Percent non-fire related tree cover loss; and (C) Percent non-fire related tree cover conditional change across blue oak woodlands during the 2012–2016 drought.
Discussion
This study documented a surge in tree cover decline in California's blue oak woodlands during the 2012–2016 drought. Our results show that the 2012–2016 drought was associated with exceptionally high levels of tree cover loss and tree cover conditional change, with these declines in tree cover peaking in 2015. This multi-year drought was accompanied by extremely high temperatures, especially in 2014 and 2015, making it the hottest drought in the study period, and perhaps in more than a century (Lund et al., 2018). During the drought, we observed exceptional increases in both fire and non-fire related tree cover loss and tree cover conditional change, both peaking in 2015. Across the study area, annual estimates of both fire and non-fire tree cover loss and tree cover conditional change were associated with interannual variability in climate anomalies, in which exceptionally dry and hot years coincided with spikes in both fire and non-fire tree cover loss and tree cover conditional change. Moreover, unusually hot drought years were associated with dramatic increases in the area of fire-induced tree cover loss by medium-high severity fires. Annual area of fire-induced tree cover loss had a positive association with the area of both non-fire tree cover loss and non-fire tree cover conditional change. These results indicate that the hot, multi-year drought of 2012–2016 led to an escalation in tree cover decline, by both fire and non-fire causes, in blue oak woodlands that was unprecedented during the study period. Nonetheless, the spatial pattern of fire-induced tree cover loss of the largest fire of the study period in 2015 had no significant association with the pre-fire non-fire tree cover loss and non-fire tree cover conditional change. Here, we posit that elevated tree mortality in early years of the drought may have enhanced productivity of understory grass and forbs thereby creating abundant fine fuels capable of catalyzing the large fires in 2015. This result further emphasizes that it is not only land-cover change but its complex interactions with ignition sources, vegetation types, climate, and topography that influence the geographic variability of wildfires.
Our observation of elevated tree cover decline is consistent with tree mortality studies in parts of blue oak woodlands and in other vegetation communities across California during the 2012–2016 drought. In a post-drought survey in 2017 in the Sequoia National Park, a portion of blue oak species range, Das et al. (2020) reported that about 19% of standing blue oak trees and 14% of all trees were killed during that drought. In blue oak species, mortality was high across all tree size classes, with a higher mortality rate in trees over 40 cm at breast height (Das et al., 2020). In the Klamath Mountains of northern California, Bost et al. (2019) found a three-fold increase in the area of canopy decline during the drought. According to Tubbesing et al. (2020) ~1–5 % of the aboveground live tree biomass across California's forested lands died between 2012 and 2017. Our results showing record high tree cover decline of the study period during the drought, particularly around 2015 and 2016, concur with the patterns of tree mortality in other studies elsewhere in California during that drought (Goulden and Bales, 2019; Pile et al., 2019; Taylor et al., 2019). Fettig et al. (2019) found that most tree mortality in the central and southern Sierra Nevada occurred in 2015 and 2016. Our results indicate that the increased levels of both fire and non-fire related tree cover declines were accompanied by record uptick in all climatic anomalies, namely, high climatic water deficit, maximum temperature, vapor pressure deficit, and low precipitation, especially in 2014 and 2015. These findings support similar studies reporting that with climate warming, excessive evaporative demand and intensified drought stresses are expected to be accompanied by more severe tree mortality due to the combined effects of drought stress, wildfires, and insect-pest outbreaks (Williams et al., 2013; Field et al., 2020).
Non-fire tree cover declines were more prevalent in the years prior to and during the largest fire-induced tree cover declines in 2015. Our climatic anomalies time series also showed that the years 2014–2015 were particularly hot and dry. Hence, we infer that tree cover declines created gaps that enhanced grass and forb productivity, and thus created more homogenous fuels, which in concert with the harshest drought conditions in 2014–2015, fomented the largest fires observed in 2015. It has been reported in some other ecosystems that non-fire tree mortality, such as those from insect outbreaks, do not necessary increase fire hazard (Hart et al., 2015; Meigs et al., 2016). Meigs et al. (2016) attribute the reduced fire risk in such instances to negative feedback effect in which tree death by insect outbreaks dampen subsequent fire severity by reducing the amount of live fuels susceptible to fire. Nonetheless, we observed a positive association between the annual area of fire-induced tree cover loss and the area of non-fire related tree cover loss and tree cover conditional change. Furthermore, annual area of non-fire tree cover loss and conditional change, that may be the result of insect attack on trees, were positively associated with tree cover loss by medium-high severity fires. These results indicate that cumulative non-fire tree cover declines from early years of the drought, in 2012–2014, may have increased fuel load, especially fine fuels from understory grass and forbs, and increased fire risk leading to larger and more severe wildfires that we observed in 2015 and 2016. By generating large, contiguous combustible biomass, drought-induced tree mortality can interact with subsequent fires to create greater potential for more catastrophic fires (Ruthrof et al., 2016; Stephens et al., 2018). Our results highlight the potential for the combined influences of drought-induced tree cover declines and warmer multi-year droughts to increase wildfire activity, leading to more rapid tree cover loss. Notwithstanding, we found no significant spatial correlations between fire-induced tree cover loss and non-fire tree cover declines during the large fires of 2015. This observation underscores the fact that it is the confounding effects of land cover along with other controlling factors including ignition sources, vegetation types, climate, and topography that determine the spatial variability of fire effects on vegetation.
We found unusual surge in annual area of tree cover loss through medium-high severity fires coincided with anomalously drier and hotter years. This finding is consistent with that of Crockett and Westerling (2018), who reported that intensified drought stress were associated with larger and more severe wildfires and greater tree mortality in recent droughts across the western United States. Historically, the area burned and the proportion of high-severity wildfires have been increasing in the western United States since the mid-1980s (Westerling, 2016; Singleton et al., 2019). This increasing trend of fire activity, that has been linked to climate, is expected to continue in a warmer and drier future climate (Liu and Wimberly, 2015; Coop et al., 2020; Higuera and Abatzoglou, 2021). Our findings indicate that frequent hotter and drier climate in the future could lead to more tree cover loss, catalyzed by more catastrophic fires; with severe effects on rare and critical habitats such as the blue oak woodlands.
The distribution range of blue oak trees is expected to shrink due to greater warming and less precipitation in the future (Kueppers et al., 2005). Future droughts may lead to more catastrophic consequences because frequent hotter droughts may amplify fire–vegetation feedbacks, making the vegetation community more susceptible to irreversible vegetation transitions (Odion et al., 2010; Hurteau et al., 2014; Tepley et al., 2017; Coop et al., 2020). Already, there are reports that fire may be detrimental to blue oak regeneration and the survival of small saplings (Das et al., 2020). Using a bioclimate modeling approach, Brown et al. (2018) projected that more of the distribution range of blue oak will become vulnerable in the future due to frequent declines in precipitation. Our findings signal a concerning future where multi-year droughts under elevated temperatures may trigger more severe forest die-off, pathogens, fire regimes, and insect outbreaks (Williams et al., 2013; Cobb et al., 2017; Field et al., 2020). Extreme droughts may cause selective tree deaths in vegetation communities, thereby altering vegetation architecture and biodiversity (Paz-Kagan et al., 2017). For instance, in the central and southern Sierra Nevada, drought-induced tree mortality killed a majority of the larger trees, and altered composition of regeneration (Fettig et al., 2019). Our study shows an alarming level of tree cover loss also occurred in the lower elevations of blue oak woodlands during the 2012–2016 record-setting drought. The medium to long-term ecological fallout in terms of the structure and composition of this ecosystem could be a priority for future research. Moreover, the drought-propelled spike in tree cover loss has the potential to increase fragmentation due to patchiness of the fire-induced tree cover loss observed across the landscape. The potential for fire-driven vegetation shifts will be enhanced by declines in the area climatically suitable for tree recruitment because of harsher post-disturbance climate (Davis et al., 2020). Moreover, a drier and warmer post-fire environment in concert with high severity fires can severely inhibit forest recovery, thereby reducing resilience of ecosystems to fire (Johnstone et al., 2016; Rodman et al., 2020).
Large map-derived areas of disturbance-related tree cover conditional change highlighted in this study by the LCMAP data products indicates that tree cover loss based only on thematic land cover change is likely under-reporting this type of phenomenon, with considerable implications for carbon accounting and other ecosystem goods and services. Moreover, interannual variability in tree cover conditional change was highly related to tree cover loss, indicating that early detection of subtle changes in tree cover condition may be vital to support proactive resource management decisions aimed at minimizing complete tree cover losses. We have demonstrated how the annual LCMAP data suite supports the assessment of thematic and conditional change in tree cover, and our results attest to the usefulness of the dataset to monitor the effects of climatic extremes and disturbance events on tree cover on multi-decadal time span. Thus, LCMAP provides unique observations of medium- to long-term land cover and land change at a crucial era where improved understanding of the effects of our changing climate and disturbance regimes on terrestrial ecosystems is paramount. Therefore, the LCMAP monitoring approach offers a promising capability toward improved characterization and estimation of tree cover change in the United States across space and time.
Conclusion
Our results revealed elevated decline in tree cover in blue oak woodlands during the major 2012–2016 drought. Overall, ~1,266 km2, constituting about 37% of the cumulative tree cover loss in the 32-year study period, were lost during the five-year drought. Additionally, ~617 km2, constituting about 28% of the cumulative tree cover conditional change in the 32-year study period, were recorded during that drought. The record decline in tree cover in blue oak woodlands specifically in 2015–2016 occurred in the fourth and fifth year of a multi-year drought beginning in 2012 that resulted in significant tree cover losses due to fire and non-fire causes in the context of a hotter drought than seen in prior decades. Our findings have important implications on the long-term vulnerability of this iconic vegetation community, and its ecosystem goods and services. The unusual increases in both fire and non-fire related tree cover declines during this drought event portend that the compounding effects of fire and climate variability may enable rapid vegetation shifts in the studied region. More specifically, our findings signify that blue oak woodlands may be vulnerable to extreme climate events and changing wildfire regimes. Here, we present early evidence that frequent droughts associated with climate warming may continue to affect tree cover in this region, while drought interaction with wildfires and the resulting feedbacks may have significant influence as well. As a result, efforts to conserve the blue oak woodlands, and potentially other vegetation communities in the Western United States, may benefit from consideration of climate risks as well as the potential for climate—fire and vegetation feedbacks.
Data Availability Statement
Publicly available datasets were analyzed in this study. This data can be found at LCMAP product suite https://doi.org/10.5066/P9W1TO6E, Monitoring Trends in Burn Severity http://www.mtbs.gov/, PRISM climate dataset https://prism.nacse.org/.
Author Contributions
Conceptualization: JB, HT, RA, and FD. Methodology: FD, RA, HT, and JB. Formal analysis and writing—original draft preparation: FD. Writing—review and editing: FD, JB, RA, and HT. Supervision: JB and RA. All authors contributed to the article and approved the submitted version.
Funding
This research was funded by the U.S. Geological Survey National Land Imaging (NLI) program under contract 140G0119C0001.
Disclaimer
Any use of trade, firm, or product names is for descriptive purposes only and does not imply endorsement by the U.S. Government.
Conflict of Interest
The authors declare that the research was conducted in the absence of any commercial or financial relationships that could be construed as a potential conflict of interest.
Acknowledgments
We thank the academic editor and the reviewers who helped us substantially improve the manuscript. We thank Dr. Todd J. Hawbaker for his helpful comments which improved earlier drafts of this manuscript.
References
Asner, G. P., Brodrick, P. G., Anderson, C. B., Vaughn, N., Knapp, D. E., and Martin, R. E. (2016). Progressive forest canopy water loss during the 2012–2015 California drought. Proc. Natl. Acad. Sci. U.S.A. 113, E249–E255. doi: 10.1073/pnas.1523397113
Bost, D. S., Reilly, M. J., Jules, E. S., DeSiervo, M. H., Yang, Z., and Butz, R. J. (2019). Assessing spatial and temporal patterns of canopy decline across a diverse montane landscape in the Klamath Mountains, CA, USA using a 30-year Landsat time series. Landsc. Ecology 34, 2599–2614. doi: 10.1007/s10980-019-00907-7
Brown, B. J., McLaughlin, B. C., Blakey, R. V., and Morueta-Holme, N. (2018). Future vulnerability mapping based on response to extreme climate events: dieback thresholds in an endemic California oak. Divers. Distrib. 24, 1186–1198. doi: 10.1111/ddi.12770
Brown, J. F., Tollerud, H. J., Barber, C. P., Zhou, Q., Dwyer, J. L., Vogelmann, J. E., et al. (2020). Lessons learned implementing an operational continuous United States national land change monitoring capability: The Land Change Monitoring, Assessment, and Projection (LCMAP) approach. Remote Sens. Environ. 238:111356. doi: 10.1016/j.rse.2019.111356
Byer, S., and Jin, Y. (2017). Detecting drought-induced tree mortality in Sierra Nevada forests with time series of satellite data. Remote Sens. 9:929. doi: 10.3390/rs9090929
Cartwright, J. (2019). Ecological islands: conserving biodiversity hotspots in a changing climate. Front. Ecol. Environ. 17, 331–339. doi: 10.1002/fee.2058
Chaney, N. W., Wood, E. F., McBratney, A. B., Hempel, J. W., Nauman, T. W., Brungard, C. W., et al. (2016). POLARIS: a 30-meter probabilistic soil series map of the contiguous United States. Geoderma 274, 54–67. doi: 10.1016/j.geoderma.2016.03.025
Christopher, D., Wayne, P. G., George, H. T., Gregory, L. J., and Phillip, P. (2002). A knowledge-based approach to the statistical mapping of climate. Clim. Res. 22, 99–113. doi: 10.3354/cr022099
Cobb, R. C., Ruthrof, K. X., Breshears, D. D., Lloret, F., Aakala, T., Adams, H. D., et al. (2017). Ecosystem dynamics and management after forest die-off: a global synthesis with conceptual state-and-transition models. Ecosphere 8:e02034. doi: 10.1002/ecs2.2034
Coop, J. D., Parks, S. A., Stevens-Rumann, C. S., Crausbay, S. D., Higuera, P. E., Hurteau, M. D., et al. (2020). Wildfire-driven forest conversion in Western North American landscapes. BioScience 70, 659–673. doi: 10.1093/biosci/biaa061
Crockett, J. L., and Westerling, A. L. (2018). Greater temperature and precipitation extremes intensify Western U.S. droughts, wildfire severity, and sierra Nevada tree mortality. J. Clim. 31, 341–354. doi: 10.1175/JCLI-D-17-0254.1
Das, A. J., Ampersee, N. J., Pfaff, A. H., Stephenson, N. L., Swiecki, T. J., Bernhardt, E. A., et al. (2020). Tree mortality in blue oak woodland during extreme drought in Sequoia National Park, California. Madroño 66, 164–175. doi: 10.3120/0024-9637-66.4.164
Davis, F. W., Sweet, L. C., Serra-Diaz, J. M., Franklin, J., McCullough, I., Flint, A., et al. (2016). Shrinking windows of opportunity for oak seedling establishment in southern California mountains. Ecosphere 7:e01573. doi: 10.1002/ecs2.1573
Davis, K. T., Higuera, P. E., Dobrowski, S. Z., Parks, S. A., Abatzoglou, J. T., Rother, M. T., et al. (2020). Fire-catalyzed vegetation shifts in ponderosa pine and Douglas-fir forests of the western United States. Environ. Res. Let. 15:12. doi: 10.1088/1748-9326/abb9df
Dobrowski, S. Z., Abatzoglou, J., Swanson, A. K., Greenberg, J. A., Mynsberge, A. R., Holden, Z. A., et al. (2013). The climate velocity of the contiguous United States during the 20th century. Global Change Biol. 19, 241–251. doi: 10.1111/gcb.12026
Eidenshink, J., Schwind, B., Brewer, K., Zhu, Z.-L., Quayle, B., and Howard, S. (2007). A project for monitoring trends in burn severity. Fire Ecol. 3, 3–21. doi: 10.4996/fireecology.0301003
Fettig, C. J., Mortenson, L. A., Bulaon, B. M., and Foulk, P. B. (2019). Tree mortality following drought in the central and southern Sierra Nevada, California, U.S. Forest Ecol. Manage. 432, 164–178. doi: 10.1016/j.foreco.2018.09.006
Field, J. P., Breshears, D. D., Bradford, J. B., Law, D. J., Feng, X., and Allen, C. D. (2020). Forest management under megadrought: urgent needs at finer scale and higher intensity. Front. For. Glob. Change 3:502669. doi: 10.3389/ffgc.2020.502669
Goulden, M. L., and Bales, R. C. (2019). California forest die-off linked to multi-year deep soil drying in 2012–2015 drought. Nat. Geosci. 12, 632–637. doi: 10.1038/s41561-019-0388-5
Griffin, D., and Anchukaitis, K. J. (2014). How unusual is the 2012–2014 California drought? Geophys. Res. Lett. 41, 9017–9023. doi: 10.1002/2014GL062433
Hart, S. J., Schoennagel, T., Veblen, T. T., and Chapman, T. B. (2015). Area burned in the western United States is unaffected by recent mountain pine beetle outbreaks. Proc. Natl. Acad. Sci. U.S.A. 112, 4375–4380. doi: 10.1073/pnas.1424037112
Higuera, P. E., and Abatzoglou, J. T. (2021). Record-setting climate enabled the extraordinary 2020 fire season in the western United States. Glob. Change Biol. 27, 1–2. doi: 10.1111/gcb.15388
Hurteau, M. D., Bradford, J. B., Fulé, P. Z., Taylor, A. H., and Martin, K. L. (2014). Climate change, fire management, and ecological services in the southwestern US. For. Ecol. Manage. 327, 280–289. doi: 10.1016/j.foreco.2013.08.007
Johnstone, J. F., Allen, C. D., Franklin, J. F., Frelich, L. E., Harvey, B. J., Higuera, P. E., et al. (2016). Changing disturbance regimes, ecological memory, and forest resilience. Front. Ecol. Environ. 14:1311. doi: 10.1002/fee.1311
Kueppers, L. M., Snyder, M. A., Sloan, L. C., Zavaleta, E. S., and Fulfrost, B. (2005). Modeled regional climate change and California endemic oak ranges. Proc. Natl. Acad. Sci. U.S.A. 102, 16281–16286. doi: 10.1073/pnas.0501427102
Liu, Z., and Wimberly, M. C. (2015). Climatic and landscape influences on fire regimes from 1984 to 2010 in the Western United States. PLoS ONE 10:e0140839. doi: 10.1371/journal.pone.0140839
Lund, J., Medellin-Azuara, J., Durand, J., and Stone, K. (2018). Lessons from California's 2012–2016 drought. J. Water Resour. Plan. Manage. 144:4018067. doi: 10.1061/(ASCE)WR.1943-5452.0000984
Madakumbura, G. D., Goulden, M. L., Hall, A., Fu, R., Moritz, M. A., Koven, C. D., et al. (2020). Recent California tree mortality portends future increase in drought-driven forest die-off. Environ. Res. Lett. 15:124040. doi: 10.1088/1748-9326/abc719
McDonald, P. M. (1990). “Quercus douglasii Hook & Arn. Blue oak,” in Silvics of North America, eds R. M. Burns, and B. H. Honkala (Washington, DC: U.S. Department of Agriculture, Forest Service), 631–639.
Meigs, G. W., Zald, H. S. J., Campbell, J. L., Keeton, W. S., and Kennedy, R. E. (2016). Do insect outbreaks reduce the severity of subsequent forest fires? Environ. Res. Lett. 11:45008. doi: 10.1088/1748-9326/11/4/045008
Mitchell, K. E., Lohmann, D., Houser, P. R., Wood, E. F., Schaake, J. C., Robock, A., et al. (2004). The multi-institution North American Land Data Assimilation System (NLDAS): utilizing multiple GCIP products and partners in a continental distributed hydrological modeling system. J. Geophys. Res. 109:D07S90. doi: 10.1029/2003JD003823
Morueta-Holme, N., Fløjgaard, C., and Svenning, J.-C. (2010). Climate change risks and conservation implications for a threatened small-range mammal species. PLoS ONE 5:e10360. doi: 10.1371/journal.pone.0010360
Odion, D. C., Moritz, M. A., and DellaSala, D. A. (2010). Alternative community states maintained by fire in the Klamath Mountains, USA. J. Ecol. 98, 96–105. doi: 10.1111/j.1365-2745.2009.01597.x
Paz-Kagan, T., Brodrick, P. G., Vaughn, N. R., Das, A. J., Stephenson, N. L., Nydick, K. R., et al. (2017). What mediates tree mortality during drought in the southern Sierra Nevada. Ecol. Appl. 27, 2443–2457. doi: 10.1002/eap.1620
Pile, L. S., Meyer, M. D., Rojas, R., Roe, O., and Smith, M. T. (2019). Drought impacts and compounding mortality on forest trees in the southern Sierra Nevada. Forests 10:237. doi: 10.3390/f10030237
Reiner, R., and Craig, A. (2011). Conservation easements in California blue oak woodlands: testing the assumption of livestock grazing as a compatible use. Nat. Areas J. 31, 408–413. doi: 10.3375/043.031.0411
Robeson, S. M. (2015). Revisiting the recent California drought as an extreme value. Geophys. Res. Lett. 42, 6771–6779. doi: 10.1002/2015GL064593
Rodman, K. C., Veblen, T. T., Battaglia, M. A., Chambers, M. E., Fornwalt, P. J., Holden, Z. A., et al. (2020). A changing climate is snuffing out post-fire recovery in montane forests. Glob. Ecol. Biogeogr. 29, 2039–2051. doi: 10.1111/geb.13174
Ruthrof, K. X., Fontaine, J. B., Matusick, G., Breshears, D. D., Law, D. J., Powell, S., et al. (2016). How drought-induced forest die-off alters microclimate and increases fuel loadings and fire potentials. Int. J. Wildland Fire 25, 819–830. doi: 10.1071/WF15028
Singleton, M. P., Thode, A. E., Sánchez Meador, A. J., and Iniguez, J. M. (2019). Increasing trends in high-severity fire in the southwestern USA from 1984 to 2015. For. Ecol. Manage. 433, 709–719. doi: 10.1016/j.foreco.2018.11.039
Stahle, D. W., Griffin, R. D., Meko, D. M., Therrell, M. D., Edmondson, J. R., Cleaveland, M. K., et al. (2013). The ancient Blue Oak woodlands of California: longevity and hydroclimatic history. Earth Interact. 17, 1–23. doi: 10.1175/2013EI000518.1
Stahle, D. W., Therrell, M. D., Cleaveland, M. K., Cayan, D. R., Dettinger, M. D., and Knowles, N. (2001). Ancient blue oak reveal human impact on San Francisco Bay salinity. Eos. Trans. Am. Geophys. Union 82, 141–145. doi: 10.1029/EO082i012p00141
Stephens, S. L., Collins, B. M., Fettig, C. J., Finney, M. A., Hoffman, C. M., Knapp, E. E., et al. (2018). Drought, tree mortality, and wildfire in forests adapted to frequent fire. BioScience 68, 77–88. doi: 10.1093/biosci/bix146
Stephenson, N. L., Das, A. J., Ampersee, N. J., Cahill, K. G., Caprio, A. C., Sanders, J. E., et al. (2018). Patterns and correlates of giant sequoia foliage dieback during California's 2012–2016 hotter drought. For. Ecol. Manage. 419-420, 268–278. doi: 10.1016/j.foreco.2017.10.053
Taylor, A., Biswas, T., Randall, J. M., Klausmeyer, K., and Cohen, B. (2019). Parched pines: a quantitative comparison of two multi-year droughts and associated mass mortalities of bishop pine (Pinus muricata) on Santa Cruz Island, California. Remote Sens. Ecol. Conserv. 6, 20–34. doi: 10.1002/rse2.123
Tepley, A. J., Thompson, J. R., Epstein, H. E., and Anderson-Teixeira, K. J. (2017). Vulnerability to forest loss through altered postfire recovery dynamics in a warming climate in the Klamath Mountains. Glob. Change Biol. 23, 4117–4132. doi: 10.1111/gcb.13704
Tubbesing, C. L., Lara, J. D., Battles, J. J., Tittmann, P. W., and Kammen, D. M. (2020). Characterization of the woody biomass feedstock potential resulting from California's drought. Sci. Rep. 10:1096. doi: 10.1038/s41598-020-57904-z
Waddell, K. L., and Barrett, T. M. (2005). Oak Woodlands and Other Hardwood Forests of California, 1990s. Portland, OR: US Department of Agriculture, Forest Service, Pacific Northwest Research Station, 245. doi: 10.2737/PNW-RB-245
Westerling, A. L. (2016). Increasing western US forest wildfire activity: sensitivity to changes in the timing of spring. Philos. Trans. R. Soc. Lond., B, Biol. Sci. 371:20150178. doi: 10.1098/rstb.2015.0178
Williams, A. P., Allen, C. D., Macalady, A. K., Griffin, D., Woodhouse, C. A., Meko, D. M., et al. (2013). Temperature as a potent driver of regional forest drought stress and tree mortality. Nat. Clim. Change 3, 292–297. doi: 10.1038/nclimate1693
Keywords: disturbance, tree cover change, climatic extremes, wildfire, LCMAP
Citation: Dwomoh FK, Brown JF, Tollerud HJ and Auch RF (2021) Hotter Drought Escalates Tree Cover Declines in Blue Oak Woodlands of California. Front. Clim. 3:689945. doi: 10.3389/fclim.2021.689945
Received: 01 April 2021; Accepted: 01 June 2021;
Published: 29 June 2021.
Edited by:
Miao Yu, Nanjing University of Information Science and Technology, ChinaReviewed by:
Artan Hysa, Epoka University, AlbaniaXu Yue, Nanjing University of Information Science and Technology, China
This work is authored by Francis Kwabena Dwomoh, Jesslyn F Brown, Heather J Tollerud and Roger F. Auch on behalf of the U.S Government and, as regards Dwomoh, Brown, Tollerud and Auch, and the U.S Government, is not subject to copyright protection in the United States. Foreign and other copyrights may apply. This is an open-access article distributed under the terms of the Creative Commons Attribution License (CC BY). The use, distribution or reproduction in other forums is permitted, provided the original author(s) and the copyright owner(s) are credited and that the original publication in this journal is cited, in accordance with accepted academic practice. No use, distribution or reproduction is permitted which does not comply with these terms.
*Correspondence: Francis K. Dwomoh, ZmR3b21vaCYjeDAwMDQwO2NvbnRyYWN0b3IudXNncy5nb3Y=