Corrigendum: Implementing the Soil Enrichment Protocol at Scale: Opportunities for an Agricultural Carbon Market
- Indigo Ag, Boston, MA, United States
High-quality agricultural carbon credits that incentivize regenerative practices can help address climate change through greenhouse gas (GHG) abatement and CO2 sequestration. Generating large volumes of such credits requires rigorous crediting methodologies. The Soil Enrichment Protocol (SEP) by the Climate Action Reserve (CAR) aims to unlock this type of crediting potential. The SEP includes new expert-driven standards for validating the use of soil biogeochemical modeling to generate credits. Technical experts at Indigo Ag participated in the SEP working group and are supporting implementation of the first project, CAR 1459_RP1, on hundreds of thousands of acres in the US. The authors share their thoughts on new approaches enabled by the SEP as both contributors to the theory behind and practitioners of these approaches. The SEP enables scalable, high-quality credits through four main advances: (1) allowing flexibility in the use of biogeochemical models that meet explicit performance requirements, (2) enabling a new approach to field-level, modeled baselines, (3) supporting a hybrid approach of credit generation using both soil measurement and modeling, and (4) requiring a new type of credit uncertainty quantification that accounts for multiple sources of uncertainty. Together these advances support agricultural credit quantification that enables payments to offset transitional costs for growers, at large enough scales to create a robust market, with a level of rigor that ensures any credited emission reductions have real climate impact. Innovations in soil analyses, advances in research, and improvements in data collection could further improve the potential for agricultural carbon credits to scale.
Introduction
To ensure long-term success at a global scale, carbon markets must be based on confidence in driving emissions reductions. This confidence requires rigorous and transparent protocol standards for greenhouse gas (GHG) mitigation and CO2 sequestration to generate high-quality carbon credits, defined as being realistically baselined, additional, and permanent, among other criteria (see Table 1). Since the use of an offset credit involves the allowance of an equal emission elsewhere on Earth, offset credits that do not equate to equivalent amounts of emissions reductions (i.e., are not real) result in direct environmental harm. Confidence is critical; if credits are not rigorous, science-based, and transparent in their methods; if low-quality, unverified credits are transacted in the market, it can cast doubt on the entire market and the incentive structure can collapse.
Recently, multiple entities (including Indigo Ag) have either explored or actively launched programs to generate agricultural carbon credits due to the potential for regenerative practices, such as cover cropping and reduced tillage, to reduce GHG emissions and sequester carbon in soils (Paustian et al., 2016). To support large-scale practice change, growers need to be directly compensated for the carbon credits they generate on their operations. Implementing regenerative practices often involves transitional costs, such as changes to equipment to plant into heavier residue, or ongoing operational costs, such as annual purchases of cover crop seeds. Carbon credit payments generated by these activities could reduce financial barriers to adoption. Currently, adoption rates of regenerative practices in many regions remain low; for example, <2% of growers used cover crops and <12% practiced no-till in 2017 [United States Department of Agriculture National Agricultural Statistics Service (NASS), 2017]. Agricultural carbon credits have an opportunity to increase regenerative practice adoption, by incentivizing regenerative management strategies that minimize GHG emissions while maximizing carbon sequestration and co-beneficial outcomes to soil and crops.
Carbon markets have developed since the 1990s through voluntary efforts, such as independent project registries, and regulatory mechanisms, such as the Kyoto Protocol and the California cap-and-trade program [International Carbon Reduction and Offset Alliance (ICROA), 2020]. There is broad consensus around a suite of quality criteria that should underpin any credible offset program [Offset Quality Initiative (OQI), 2008]. Individual programs, such as the Climate Action Reserve (CAR), maintain their own programmatic standards built around these quality criteria, and then adopt individual protocols and methodologies for specific project activities, such as forestry or agricultural land management (Climate Action Reserve Offset Program Manual, 2021). Several protocols have been adopted to address implementation and monitoring of GHG mitigation projects on agricultural lands, including the CAR Nitrogen Management Protocol (Climate Action Reserve Nitrogen Management Protocol Version 1.0, 2012) and California Compliance Offset Protocol for Rice Cultivation Projects (COP RCP) (Compliance Offset Protocol Rice Cultivation Projects, 2015). Except for livestock manure digestion projects, these past efforts have not resulted in large-scale projects or significant credit volume. This lack of scale is at least partly a result of a reliance on the combined precedent of industrial emission reduction methodologies and forestry-focused land use methodologies, which proved ineffective in creating scalable agricultural projects. Additionally, those protocols faced significant challenges with farmer engagement and data collection, partly because either the programs or protocols were highly prescriptive and left little room for innovation by project aggregators or for growers to be responsive to changes in market demand for certain crops. For example, the 2015 COP RCP generated zero credits despite stable demand and above average prices in the California compliance offset market (Air Resources Board Offset Credit Issuance, 2020; Compliance Offset Protocol Rice Cultivation Projects, 2015). This protocol generated zero credits because of high data burdens, grower resistance to monitoring and data collection requirements, and limited opportunity to practically aggregate multiple fields into a single project. The protocol was also limited in geographic scope to rice growing regions in California, the Mississippi River delta, and Louisiana.
The CAR Soil Enrichment Protocol v1.0 (SEP) aims to generate high-quality carbon credits at scale by providing opportunities for projects to continually benefit from scientific advancements in agricultural sampling methods and soil biogeochemical modeling, while supporting credit generation at a grower-beneficial cadence (Climate Action Reserve Soil Enrichment Protocol Version 1.0, 2020). Indigo participated in the stakeholder working group for the CAR-led development of the SEP. The registry engaged with expert working groups that represented multiple perspectives, including growers, scientists, environmental NGOs, and industry representatives, and included public comment periods. This process resulted in an independently validated, publicly available, and scalable methodology with four critical advances from previous protocols: (1) a flexible approach in soil biogeochemical model use within a single project, (2) a new approach to generate field-level, modeled baselines, (3) a combined measurement and modeling approach to credit generation, and (4) a novel uncertainty quantification approach. These advances enable the SEP to yield high-quality carbon credits from the quantification and verification of GHG emission reductions associated with soil enrichment projects on agricultural lands.
Protocols like the SEP do not exist in a vacuum; national policies can play a key role in accelerating their impact. In the US, the Growing Climate Solutions Act aims to provide clarity around acceptable standards for agricultural carbon and GHG measurement and verification. If implemented as proposed, this act will reduce confusion on the quality and value of various carbon programs that farmers can choose from Growing Climate Solutions Act (2020). If quality standards are set too low, however, the problem would not be solved for growers because the USDA standard would not align with the minimum quality demanded by the wider carbon market. Another proposal, the establishment of a carbon bank, could encourage both farmers and project developers to invest in project implementation. There are many ways that this idea could be implemented (e.g., a buyer of last resort, transition payments, no interest loans for capital costs, etc.), but the basic concept of providing stable financing to encourage climate smart agricultural practices is helpful if the structure and timing of financing benefits growers and drives practice change. Lastly, policies that discourage regenerative practice adoption could be changed, such as Federal crop insurance rules, which, in some cases, penalize the use of cover crops.
In this perspective article, we share how the SEP supports a more robust carbon market in agriculture, as well as our learnings to date from operating as a CAR SEP project developer for a project on hundreds of thousands of acres. We also discuss how innovations in commercial soil analyses, advances in agroecosystem and soil biogeochemical modeling, and targeted research can further reduce uncertainties, increase inclusivity, and strengthen the quality of agricultural soil carbon credits at a global scale.
Quantifying Agricultural Carbon Sequestration and GHG Emissions At Scale Requires Leveraging The Latest Science
Climate and environmental issues require pressing action and the science behind regenerative agriculture has reached a level of maturity where we can implement net beneficial solutions today (Pittelkow et al., 2015; Griscom et al., 2017; National Academies of Sciences, 2019; Oldfield et al., 2019; Paustian et al., 2020). Although many regenerative agriculture areas remain in need of additional research, the SEP provides a framework whereby these needs are not a bottleneck. Instead, the protocol balances the current state of scientific knowledge supporting each credit issuance iteration with standards that allow continual advancements in agricultural research, soil measurement methods, and biogeochemical modeling. These standards focus on the use of soil biogeochemical model simulations, realistic and adaptive baselines, the combined use of soil measurement with modeling to generate credits, and the quantification of credit uncertainty. The SEP also employs standards to ensure that carbon credited meets a 100-year permanence period (see Supplementary Materials for details).
New CAR SEP Model Guidance Standards Increase Soil Biogeochemical Modeling Flexibility and Ensure Verifiable Use for a Given SEP Project
Model requirements described in the stand-alone CAR SEP Model Guidance define new standards for the use of peer-reviewed published experimental data to validate the capacity of a model to simulate the practice changes, crop types and biophysical settings in a SEP project (Climate Action Reserve Requirements and Guidance for Model Calibration, Validation, Uncertainty, and Verification for Soil Enrichment Products Version 1.0, 2020). In previous crediting protocols such as the CARB Compliance forest offset protocol, models were not permitted to change model parameterizations or undergo updates during a project [California Air Resources Board (CARB) U.S. Forest Projects Compliance Offset Program, 2015]. Instead, models were typically approved for use individually based on expert input and the general robustness of evidence for model performance in peer-reviewed publications. Furthermore, crediting methods did not require quantification of model prediction error, and thus provided no mechanism to account for model performance in credit estimations.
In contrast, the SEP Model Guidance allows any model to be used in a SEP project if the specific model validation requirements are demonstrated, documented, and reviewed in a separate report prior to credit issuance (Climate Action Reserve Soil Enrichment Protocol Version 1.0, 2020). The SEP model validation report ensures each model version and associated parameter sets demonstrate appropriate calibration, validation, and model uncertainty quantification for the specific SEP project in which the model will be used, as defined by the project crop types, practice changes, soil properties, and geographies. These standards allow model recalibration and version improvements to be used over the project duration. Critically, independent expert-review, CAR approval, and public availability are required of every validation report to demonstrate that these standards are met. This requirement helps ensure that all model performance for SEP projects is transparent, and that associated data are available to the scientific community for side-by-side comparisons. In the development of the Model Guidance document, one of the critical challenges was how to best support verification entities who may not have the expertise needed to ensure that a soil biogeochemical model was used appropriately. SEP Model Guidance standards aim to balance rigor with practicality in implementation, requiring the engagement of expert review to verify model performance, but not requiring this expertise from verifiers. Verifiers are instead allowed to reference an approved model validation report to confirm model application in each project, checking that model versions, required information, and parameter sets match their application to quantify credits within a project.
The SEP Enables a Baseline Approach That Is Better Suited to the Dynamics of Agricultural Lands
Carbon credits are quantified as the difference in emissions that occurred in the project scenario and the baseline scenario—i.e., emissions that would have occurred in the counterfactual world in which the project did not incentivize a change. Methodologies for managed lands previously relied on two approaches to establish a project baseline: a static approach – wherein samples or historical data are used to create a single baseline that is used for several (often 5 or 10) years or an entire project, or a forward-modeling approach – wherein referential historical data is used to create a model projection (e.g., linear) of baseline emissions that is referenced to evaluate credits earned each year of the project. With re-baselining occurring at increments of 5 years or longer, neither approach accounts for variables, such as changes in weather or market demands, that may change grower choices practices, such as crop rotations. This limits the ability of such protocols to quantify offsets accurately and dynamically at scale. Recent work highlighted the potential to over-estimate emissions reductions from deforestation and forest degradation projects (REDD+) in the Brazilian Amazon (West et al., 2020). Significant changes in market forces reduced the expected level of deforestation during the project early implementation phase, but the model baselines were quantified with historical data and did not account for these changes. As a project developer, Indigo is now undertaking the first implementation of the new baseline approach in the SEP that generates what we term a Just-in-Time Adaptive Baseline (JITAB)—inspired by the Just-In-Time Adaptive Intervention approach in behavioral science (Nahum-Shani et al., 2018; Hardeman et al., 2019). This approach creates a baseline using static historic data and incorporates dynamic modeling to re-calculate the baseline every year in response to ongoing real-world changes in project crop rotation and weather (see Supplementary Materials for further discussion).
A Hybrid Sampling and Modeling Approach Enables Annual Quantification of Large Projects
Before the CAR SEP, agricultural GHG emission mitigation methodologies relied either on narrow use of models, solely on direct soil measurement, or on use of narrowly applicable default emission factors (Climate Action Reserve Nitrogen Management Protocol Version 1.0, 2012; Compliance Offset Protocol Rice Cultivation Projects, 2015). Direct measurement-only approaches have significant barriers to scale: they are expensive, struggle to handle short-term fluxes in soil organic carbon (SOC) content and trace gases, and can take a long time to deliver results (e.g., often a minimum of 5–10 years). Moreover, setting baselines for measurement-only projects requires either paired control plots which are infeasible at scale, or regional benchmarks for which there are not yet sufficient data. Using soil biogeochemical models exclusively might be less expensive but would only produce low-quality credits as the models would not be anchored to the reality of the individual site(s), even if provided accurate and spatially resolved soils, climate, and management data. Default emissions factor approaches do see common use in crediting protocols as they are the most straightforward and least expensive quantification option. However, they also have narrow scope of application, low field-level accuracy, and are unable to adapt to changing conditions and management. The SEP requirements provide a framework to overcome these limitations using a hybrid approach that balances in-field soil sampling and analysis with biogeochemical models that can be continually improved and validated (Climate Action Reserve Requirements and Guidance for Model Calibration, Validation, Uncertainty, and Verification for Soil Enrichment Products Version 1.0, 2020), as well as default equations for non-CO2 GHG sources (N2O and CH4) that provide an alternative option when models cannot be validated for these trace gas fluxes. This approach enables scalability; for example, in Indigo's first project under the SEP, we are implementing a process that would use soil core measurements a quarter of all project fields. Combining soil measurements with modeling requires high-quality data yet enables wide-scale GHG estimation on project fields that accounts for and incentivizes the reduction of model uncertainty via improved model performance.
SEP Uncertainty Quantification Requirements Allow Innovative Post-stratification of Sampling Points and New Approaches for Handling Missing Data
The SEP requires uncertainty quantification to include multiple sources of uncertainty, including project sampling design uncertainty and uncertainty arising from model used in credit quantification. The benefits of this approach are twofold: one, model prediction error is explicitly represented in quantification of emission reductions and removals, allowing models to be used with measurable confidence to generate credits for the first time in an offset project protocol; and two, combining both sources of uncertainty enable post-stratification statistical methods able to meet the unique challenges of monitoring emission reductions in agricultural soils.
Agricultural soils can be highly heterogeneous both within fields and regions, and soil properties vary significantly across space and time (Wuest, 2014). Carbon credit projects that estimate climate benefits using a random, representative sampling points have highly uncertain climate benefit measures within a project unless many soil samples are pulled due to this inherent heterogeneity (Franzleubbers, 2010). One way to reduce the number of soil samples without increasing uncertainty is to divide the project lands into non-overlapping groups, called “strata,” to draw a random sample in each stratum independently of the other strata, and to allocate more samples to strata that are expected to be more heterogeneous in the outcome variable (Cochran, 1977; Holt and Smith, 1979). The SEP, like many methodologies, encourages stratification.
For the estimation tasks in the SEP, stratification is challenging for two reasons. First, there are multiple outcome variables (i.e., emissions reductions in various gases) and the ideal stratification may vary significantly for one outcome variable to another. Second, practice changes made by growers are important stratification variables, as they differ substantially in their average reduction in emissions. Many growers, however, do not know what practice change they will make upon joining the program, or those they will make in the subsequent years, making it difficult to assign land to strata at enrollment. Indigo is forming strata after creating a random sample of the project because of these challenges, a technique is called post-stratification (Holt and Smith, 1979). Like pre-stratification, post-stratification can reduce variance, if strata are homogeneous in their outcome variable. Post-stratification can also reduce bias introduced by “missing soil samples” that were planned but not taken due to unforeseen challenges including temporary floods, tall crops, frozen soil, grazing cattle, and samples lost or damaged in shipment. Bias from these missing samples is mitigated if strata are formed such that the observed samples are representative of their stratum. This is useful in large sampling campaigns like those in SEP projects because the planned samples appear in thousands of fields that are accessible for sampling for just a few months per year.
New Technologies and Research Coupled With Carbon Credits Can Accelerate The Implementation of Regenerative Agriculture At The Global Scale
While the SEP provides a path forward for large-scale projects to quantify agricultural credits, it is also designed to advance with the state of the science. Several areas of research and innovation can improve projects, implementation, and the quality of agricultural carbon credits so that agricultural soils can realize their full potential as a negative emissions technology to mitigate climate change on a global scale (summarized in Table 2). Smith et al. provide a comprehensive assessment of soil measurement, reporting, and verification in the context of greenhouse gas removal projects (Smith et al., 2020). In this section, we discuss innovations that can reduce the cost of estimating baseline emissions, collecting data, and verifying offsets, could make agricultural carbon credits more accessible to all producers and enable greater adoption of environmentally beneficial practices. We highlight three of these areas: (1) improving soil analyses, (2) continuing research and updating models to better account for all soil gains and losses (including erosion and inorganic carbon), and (3) improving the accessibility and management of agricultural data.
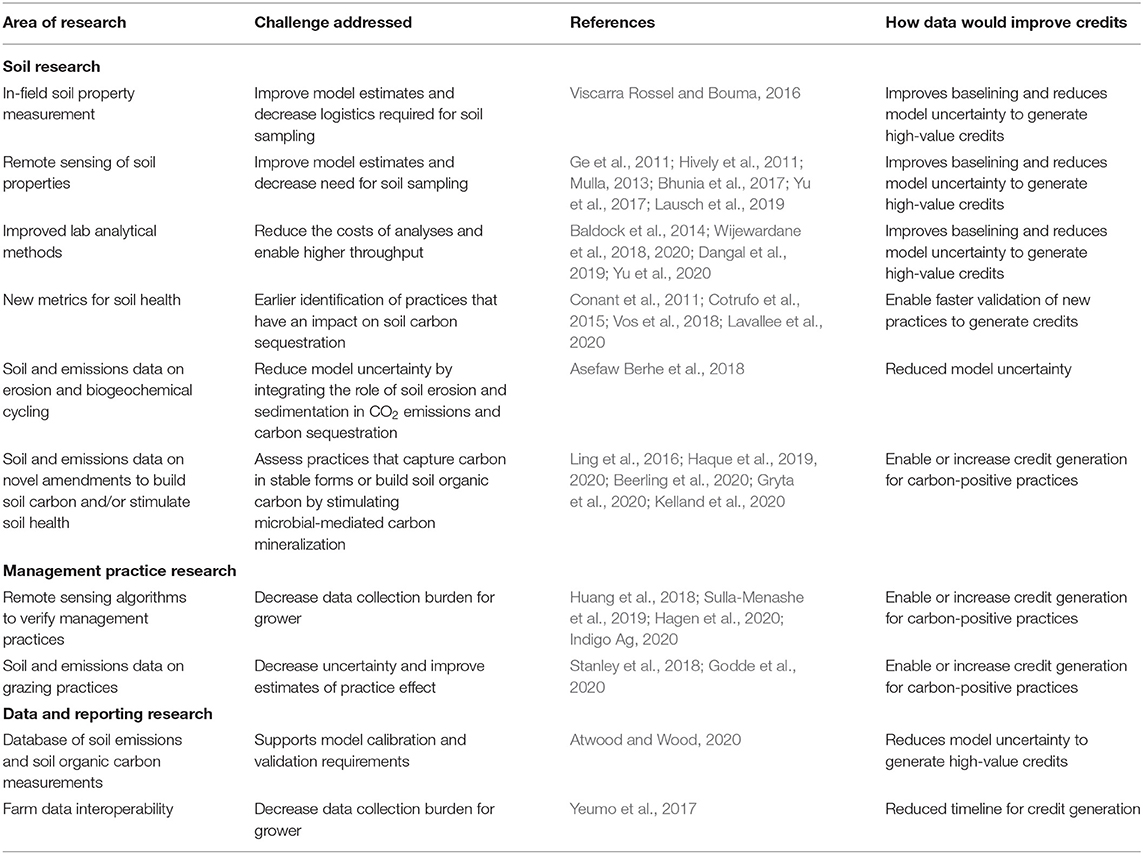
Table 2. Landscape of research and technologies that could accelerate the impact of agriculture as a tool to reduce net GHG emissions.
Innovations in Soil Analyses Can Improve Scale and Throughput
Conventional soil carbon quantification methods require soil core sample collection, transportation, and multi-step lab analyses to deliver accurate and precise results. In practice, separate analyses are required to characterize soil carbon by dry combustion, bulk density by soil weight over volume, texture by hydrometer or pipet method, and pH by pH-meter. While these methods are trusted and widely adopted, they can be time consuming, labor intensive, and expensive. Novel rapid and accurate soil measurement technologies would revolutionize soil carbon sampling and measurement systems. Remote sensing of soil properties would be the most impactful advance, but current methods have not demonstrated strong performance for soil organic carbon (R2 > 0.85) or are limited to fields with bare soil—a requirement that is hard to meet in no-till systems with residue and cover crops (Bhunia et al., 2017; Yu et al., 2017). In-field soil property measurements through probes or scanners still require in-person visit fields but would reduce sample shipping and tracking logistical needs (Viscarra Rossel and Bouma, 2016). Finally, improvements in lab analyses could decrease costs and already show promise in the near-term based on published data on spectroscopic techniques and existing spectral databases (Baldock et al., 2014; Yu et al., 2020) (further detail in Supplementary Material).
Research and Methodology Updates Could Improve Accounting for All Potential Soil Carbon Gains and Losses
Further research is critical to elucidate how key practices and crop systems impact GHG emissions and soil carbon sequestration. Recognizing this, Indigo has undertaken a long-term research effort, the Soil Carbon Experiment, described in the Supplementary Material. External research has also illustrated how methodologies and biogeochemical models could be improved to better account for carbon enrichment or loss in diverse soils. The methods described in this article and in the SEP primarily focus on organic carbon flux. The SEP allows for use of amendments such as biochar, which includes a component of inorganic carbon, to enhance carbon sequestration provided that the carbon remains in the project area. Properly quantifying and verifying the inorganic carbon stocks, however, is challenging as it is not currently estimated by biogeochemical models. Similarly, soil erosion is an important factor to accurately estimate the benefit of practices such as no-till and cover cropping (Asefaw Berhe et al., 2018). Although some models like EPIC and RZWQM2 account for erosion, many do not. Updating models and methods to better account for these sources and losses of carbon could further incentivize adoption of regenerative practices. Recent research has also highlighted ways that soils could be used to capture carbon through enhanced rock weathering, in which the soil-captured carbon eventually travels through waterways and deposits on the ocean floor (Beerling et al., 2020). This type of sequestration has benefits in terms of greater certainty of permanence but would require a new methodology or significant revision to the SEP as the ultimate location of the carbon goes outside the bounds of the fields within a project.
Improved Data Collection Methods Can Reduce Barriers to Entry for Growers
The volume of data required to create high-quality credits cannot be understated. Precise, verifiable, and traceable soil and agronomic data must be collected from grower fields and grower records to keep uncertainty low. These documentation requirements represent significant investment from the grower and the project developer, both in interview time and in the effort to properly clean and assess the data for quality. Multiple ag-tech companies, including Indigo, have created software tools to support soil and grower data collection. Even so, the burden of data collection remains a challenge for growers as many farm records remain undigitized. Remote sensing technology can be leveraged as a gap-filling measure to help reduce the burden of historical data collection across project fields (Ge et al., 2011; Huang et al., 2018; Sulla-Menashe et al., 2019). Although growers must still corroborate the data and the project developer must still verify eligible practice changes have occurred, remote sensing could provide initial crop type and practice data across project fields for current and previous seasons. Research investments that improve and validate remote sensing tools for field-level practice determination can play a significant role in realizing its potential to decrease the data collection burden.
Conclusion
In this perspective article, we explored the theoretical underpinnings and key advancements of carbon crediting under the CAR SEP– a flexible modeling approach, a new approach to baselining, a hybrid measurement and modeling approach to credit generation, and a new approach to uncertainty quantification. Thanks to growing voluntary carbon market incentives and rigorous protocols like the SEP, there are financial mechanisms to reward producers who implement land management practices that sequester additional carbon in soil and mitigate GHG emissions. Agricultural carbon credits can be further enhanced by improving grower data collection through remote sensing, funding research on high-throughput and accurate soil quantification technologies, and leveraging research insights in comprehensive calibration-validation databases. It is important to acknowledge that agricultural offsets, while impactful, must be complemented by critical emissions reductions and natural climate solutions across all sectors to mitigate climate change (Griscom et al., 2017). However, wide-scale adoption of regenerative agriculture can serve as a viable, low-cost, and co-beneficial component of a global climate change impact reduction effort. By continuing to leverage scientific advancements and cutting-edge research, we can improve upon model uncertainties, issue high-quality credits, and implement this negative emissions technology at scale.
Data Availability Statement
The original contributions generated for the study are included in the article/Supplementary Material, further inquiries can be directed to the corresponding author.
Author Contributions
AJ and AK contributed to manuscript ideation, primary writing, and editing. MM, MD, and EC contributed to writing, editing, and review. CB and GP contributed to writing and review. DH contributed to review. All authors contributed to the article and approved the submitted version.
Conflict of Interest
All authors are employed by Indigo Agriculture.
Supplementary Material
The Supplementary Material for this article can be found online at: https://www.frontiersin.org/articles/10.3389/fclim.2021.686440/full#supplementary-material
References
Air Resources Board Offset Credit Issuance (2020). Available online at: ww2.arb.ca.gov/our-work/programs/compliance-offset-program/arb-offset-credit-issuance (accessed March 12, 2021).
Asefaw Berhe, A., Barnes, R. T., Six, J., and Marín-Spiotta, E. (2018). Role of soil erosion in biogeochemical cycling of essential elements: carbon, nitrogen, and phosphorus. Annu. Rev. 46, 521–548. doi: 10.1146/annurev-earth-082517-010018
Atwood, L. W., and Wood, S. (2020). AgEvidence: Agro-environmental responses of conservation agricultural practices in the US Midwest published from 1980 to 2017. Knowledge Network for Biocomplexity.
Baldock, J. A., Hawke, B., Sanderman, J., and Macdonald, L. M. (2014). Predicting contents of carbon and its component fractions in Australian soils from diffuse reflectance mid-infrared spectra. Soil Res. 51, 577–595. doi: 10.1071/SR13077
Beerling, D. J., Kantzas, E. P., Lomas, M. R., Wade, P., Eufraiso, R. M., Renforth, P., et al. (2020). Potential for large-scale CO2 removal via enhanced rock weathering with croplands. Nature 583, 242–248. doi: 10.1038/s41586-020-2448-9
Bhunia, G. S., Shit, P. K., and Pourghasemi, H. R. (2017). Soil organic carbon mapping using remote sensing techniques and multivariate regression model. Geocarto Int. 34, 215–226. doi: 10.1080/10106049.2017.1381179
California Air Resources Board (CARB) U.S. Forest Projects Compliance Offset Program (2015). Available online at: https://ww2.arb.ca.gov/our-work/programs/compliance-offsetprogram/compliance-offset-protocols/us-forest-projects/2015 (accessed May 5, 2021).
Climate Action Reserve Nitrogen Management Protocol Version 1.0 (2012). Reducing Nitrous Oxide Emissions Through Improved Nitrogen Management in Crop Production. Available online at: https://www.climateactionreserve.org/wp-content/uploads/2012/06/Nitrogen_Management_Project_Protocol_V1.01.pdf (accessed May 7, 2021).
Climate Action Reserve Offset Program Manual (2021). Available online at: https://www.climateactionreserve.org/wp-content/uploads/2021/03/Reserve_Offset_Program_Manual_March_2021.pdf (accessed May 2, 2021).
Climate Action Reserve Requirements Guidance for Model Calibration Validation, Uncertainty, Verification for Soil Enrichment Products Version 1.0. (2020). Available online at: https://www.climateactionreserve.org/wp-content/uploads/2020/10/SEP-Model-Requirements-and-Guidance-v1.0a.pdf (accessed May 7, 2021).
Climate Action Reserve Soil Enrichment Protocol Version 1.0 (2020). Available online at: https://www.climateactionreserve.org/wp-content/uploads/2020/10/Soil-Enrichment-Protocol-V1.0.pdf (accessed March 25, 2021).
Compliance Offset Protocol Rice Cultivation Projects (2015). Available online at: https://ww2.arb.ca.gov/sites/default/files/classic/cc/capandtrade/protocols/rice/riceprotocol2015.pdf (accessed March 25, 2021).
Conant, R. T., Ryan, M. G., Ågren, G. I., Birge, H. E., Davidson, E. A., Eliasson, P. E., et al. (2011). Temperature and soil organic matter decomposition rates - synthesis of current knowledge and a way forward. Global Change Biol. 17, 3392–3404 doi: 10.1111/j.1365-2486.2011.02496.x
Cotrufo, M. F., Soong, J. L., Horton, A. J., Campbell, E. E., Haddix, M. H., Wall, D. L., et al. (2015). Soil organic matter formation from biochemical and physical pathways of litter mass loss. Nat. Geosci. 8, 776–779. doi: 10.1038/ngeo2520
Dangal, S. R. S., Sanderman, J., Wills, S., and Ramirez-Lopez, L. (2019). Accurate and precise prediction of soil properties from a large mid-infrared spectral library. Soil Syst. 3:11. doi: 10.3390/soilsystems3010011
Franzleubbers, A. J. (2010). Achieving soil organic carbon sequestration with conservation agricultural systems in the southeastern United States. Soil Sci. Soc. Am. J. 74, 347–357. doi: 10.2136/sssaj2009.0079
Ge, Y., Thomasson, A., and Sui, R. (2011). Remote sensing of soil properties in precision agriculture: a review. Front. Earth Sci. 5:229–238 doi: 10.1007/s11707-011-0175-0
Godde, C. M., de Boer, I. J. M., Ermgassen, E., Herrero, M., van Middelaar, C. E., and Muller, A. (2020). Soil carbon sequestration in grazing systems: managing expectations. Climatic Change 161, 385–391. doi: 10.1007/s10584-020-02673-x
Griscom, B. W., Adams, P. W., Ellis, R. A., Houghton, G., Lomax, D. A., Miteva, W. H., et al. (2017). Natural climate solutions. Proc. Natl. Acad. Sci. U.S.A. 114, 11645–11650. doi: 10.1073/pnas.1710465114
Gryta, A., Frac, M., and Oszust, K. (2020). Genetic and metabolic diversity of soil microbiome in response to exogenous organic matter amendments. Agronomy 10:546. doi: 10.3390/agronomy10040546
Hagen, S. C., Delgado, G., Ingraham, P., Cooke, I., Emery, R. P., et al. (2020). Mapping conservation management practices and outcomes in the corn belt using the operational tillage information system (OpTIS) and the denitrification–decomposition (DNDC) model. Land 9:408. doi: 10.3390/land9110408
Haque, F., Santos, R., and Chiang, Y. W. (2020). CO2 sequestration by wollastonite-amended agricultural soils – An Ontario field study. Int. J. Greenhouse Gas Control 97:103017. doi: 10.1016/j.ijggc.2020.103017
Haque, F., Santos, R., Dutta, A., Thimmanagari, M., and Chiang, Y. W. (2019). Co-benefits of wollastonite weathering in agriculture: CO2 sequestration and promoted plant growth. ACS Omega 4, 1425–1433. doi: 10.1021/acsomega.8b02477
Hardeman, W., Houghton, J., Lane, K., Jones, A., and Naughton, F. (2019). A systematic review of just-in-time adaptive interventions (JITAIs) to promote physical activity. Int. J. Behav. Nutr. Phys. Act 16:31. doi: 10.1186/s12966-019-0792-7
Hively, W. D., McCarty, G. W., Reeves, J. B., Lang, M. W., Oesterling, R. A., and Delwiche, S. R. (2011). Use of airborne hyperspectral imagery to map soil properties in tilled agricultural fields. Appl. Environ. Soil Sci. 2011:358193. doi: 10.1155/2011/358193
Holt, D., and Smith, T. (1979). Post stratification. J. R. Stat. Soc. Ser. A, 142, 33–46. doi: 10.2307/2344652
Huang, Y., Chen, Z. X., Yu, T., Huang, X. Z., and Gu, X. F. (2018). Agricultural remote sensing big data: management and applications. J. Int. Ag. 17, 1915–1931. doi: 10.1016/S2095-3119(17)61859-8
Indigo Ag (2020). Regeneration on the American Farm Progress Report. Available online at: https://www.indigoag.com/hubfs/Progress%20Report/Indigo_Progress_Report_July_2020.pdf (accessed March 25, 2021).
International Carbon Reduction Offset Alliance (ICROA), (2020). Evolution of Voluntary Carbon Market Graphic. Available online at: https://voluntarycarbonmarket.org/docs/VCM-Interactive-PDF-Version-1-With-Introduction.pdf (accessed May 2, 2021).
Kelland, M. E., Wade, P. W., Lewis, A. L., Taylor, L. L., Sarkar, B., Andrews, M. G., et al. (2020). Increased yield and CO2 sequestration potential with the C4 cereal Sorghum bicolor cultivated in basaltic rock dust-amended agricultural soil. Glob Change Biol. 26, 3658–3676. doi: 10.1111/gcb.15089
Lausch, A., Baade, J., Bannehr, L., Borg, E., Bumberger, J., Chabrilliat, S., et al. (2019). Linking remote sensing and geodiversity and their traits relevant to biodiversity—Part I: soil characteristics. Remote Sens. 11:2356. doi: 10.3390/rs11202356
Lavallee, J., Soong, J. L., and Cotrufo, M. F. (2020). Conceptualizing soil organic matter into particulate and mineral-associated forms to address global change in the 21st century. Global Change Biol. 26, 261–273. doi: 10.1111/gcb.14859
Ling, N., Zhu, C., Xue, C., Chen, H., Duan, Y., Peng, C., et al. (2016). Insight into how organic amendments can shape the soil microbiome in long-term field experiments as revealed by network analysis. Soil Biol. Biochem. 99, 137–149. doi: 10.1016/j.soilbio.2016.05.005
Mulla, D. (2013). Twenty-five years of remote sensing in precision agriculture: key advances and remaining knowledge gaps. Biosyst. Eng. 114, 358–371. doi: 10.1016/j.biosystemseng.2012.08.009
Nahum-Shani, I., Smith, S. N., Spring, B. J., Collins, L. M., Witkiewitz, K., Tewari, A., et al. (2018). Just-in-time adaptive interventions (JITAIs) in mobile health: key components and design principles for ongoing health behavior support. Ann. Behav. Med. 52:446. doi: 10.1007/s12160-016-9830-8
National Academies of Sciences Engineering, and Medicine. (2019). Negative Emissions Technologies and Reliable Sequestration: A Research Agenda. Washington, DC: The National Academies Press.
Offset Quality Initiative (OQI) (2008). Ensuring Offset Quality: Integrating High-Quality Greenhouse Gas Offsets into North American Cap-and-Trade Policy. Available online at: https://www.c2es.org/site/assets/uploads/2008/07/ensuring-offsetquality.pdf (accessed May 2, 2021).
Oldfield, E. E., Bradford, M. A., and Wood, S. A. (2019). Global meta-analysis of the relationship between soil organic matter and crop yields. Soil 5, 15–32. doi: 10.5194/soil-5-15-2019
Paustian, K., Chenu, C., Conant, R., Cotrufo, F., Lal, R., Smith, P., et al. (2020). Climate mitigation potential of regenerative agriculture is significant! Regenerative Agriculture Foundation June 2020 Report
Paustian, K., Lehmann, J., Ogle, S., Reay, D., Robertson, G. P., and Smith, P. (2016). Climate-smart soils. Nature 532, 49–57. doi: 10.1038/nature17174
Pittelkow, C. M., Liang, X., Linquist, B. A., van Groenigen, K. J., Lee, J., Lundy, M. E., et al. (2015). Productivity limits and potentials of the principles of conservation agriculture. Nature 517, 365–368. doi: 10.1038/nature13809
Smith, P., Soussana, J. F., Angers, D., Schipper, L., Chenu, C., Rasse, D. P., et al. (2020). How to measure, report, and verify soil carbon change to realize the potential of soil carbon sequestration for atmospheric greenhouse gas removal. Global Change Biol. 26, 219–241. doi: 10.1111/gcb.14815
Stanley, P., Rowntree, J. E., Beede, D., DeLonge, M. S., and Hamm, M. W. (2018). Impacts of soil carbon sequestration on life cycle greenhouse gas emissions in Midwestern USA beef finishing systems. Agric. Syst. 162, 249–258. doi: 10.1016/j.agsy.2018.02.003
Sulla-Menashe, D., Ma, K. F., Braswell, B. H., Friedl, M., and Horn, O. (2019). Providing Growers with Real-Time Crop Health Information Using NASA's Harmonized Landsat Sentinel-2 Product. Abstract retrieved from Abstracts in American Geophysical Union, Fall Meeting 2019 database (Abstract No. GC23H-1451).
United States Department of Agriculture National Agricultural Statistics Service (NASS) (2017). Census of Agriculture. Available online at: nass.usda.gov/AgCensus (accessed May 4, 2021).
Viscarra Rossel, R. A., and Bouma, J. (2016). Soil sensing: a new paradigm for agriculture. Agric. Syst. 148, 71–74. doi: 10.1016/j.agsy.2016.07.001
Vos, C., Jaconi, A., Jacobs, A., and Don, A. (2018). Hot regions of labile and stable soil organic carbon in Germany – Spatial variability and driving factors. Soil 4, 153–167. doi: 10.5194/soil-4-153-2018
West, T., Börner, J., Sills, E. O., and Kontoleon, A. (2020). Overstated carbon emission reductions from voluntary REDD+ projects in the Brazilian Amazon. Proc. Natl. Acad. Sci. U.S.A 117, 24188–24194. doi: 10.1073/pnas.2004334117
Wijewardane, N. K., Ge, Y., Sanderman, J., and Ferguson, R. (2020). Fine grinding is needed to maintain the high accuracy of MIR spectroscopy for soil property estimation. Soil Sci. Soc. Am. J. 85, 263–272. doi: 10.1002/saj2.20194
Wijewardane, N. K., Ge, Y., Wills, S., and Libohova, Z. (2018). Predicting physical and chemical properties of U.S. soils with a mid-infrared reflectance spectral library. Soil Sci. Soc. Am. J. 82, 722–731. doi: 10.2136/sssaj2017.10.0361
Wuest, S. (2014). Seasonal variation in soil organic carbon. Soil Sci. Soc. Am. J. 78, 1442–1447. doi: 10.2136/sssaj2013.10.0447
Yeumo, E., Alaux, M., Arnaud, E., Aubin, S., Baumann, U., Buche, P., et al. (2017). Developing data interoperability using standards: a wheat community use case. 6:1843. doi: 10.12688/f1000research.12234.2
Yu, H., Kong, B., Wang, G., Du, R., and Qie, G. (2017). Prediction of soil properties using a hyperspectral remote sensing method. Arch. Agronomy Soil Sci. 64, 546–559. doi: 10.1080/03650340.2017.1359416
Keywords: negative emissions technology, agriculture, soil, carbon offset, carbon credit, regenerative agriculture
Citation: Jackson Hammond AA, Motew M, Brummitt CD, DuBuisson ML, Pinjuv G, Harburg DV, Campbell EE and Kumar AA (2021) Implementing the Soil Enrichment Protocol at Scale: Opportunities for an Agricultural Carbon Market. Front. Clim. 3:686440. doi: 10.3389/fclim.2021.686440
Received: 26 March 2021; Accepted: 27 May 2021;
Published: 21 June 2021.
Edited by:
Phil Renforth, Heriot-Watt University, United KingdomReviewed by:
Andy D. Robertson, Shell, United StatesRafael Mattos Dos Santos, University of Guelph, Canada
Copyright © 2021 Jackson Hammond, Motew, Brummitt, DuBuisson, Pinjuv, Harburg, Campbell and Kumar. This is an open-access article distributed under the terms of the Creative Commons Attribution License (CC BY). The use, distribution or reproduction in other forums is permitted, provided the original author(s) and the copyright owner(s) are credited and that the original publication in this journal is cited, in accordance with accepted academic practice. No use, distribution or reproduction is permitted which does not comply with these terms.
*Correspondence: Ashok A. Kumar, YWprdW1hciYjeDAwMDQwO2luZGlnb2FnLmNvbQ==