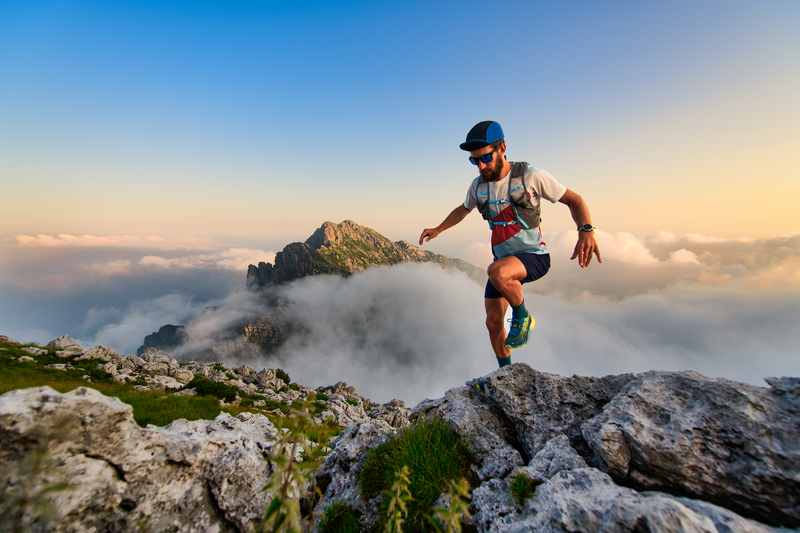
95% of researchers rate our articles as excellent or good
Learn more about the work of our research integrity team to safeguard the quality of each article we publish.
Find out more
TECHNOLOGY AND CODE article
Front. Clim. , 06 May 2021
Sec. Carbon Dioxide Removal
Volume 3 - 2021 | https://doi.org/10.3389/fclim.2021.656108
This article is part of the Research Topic Scaling-Up Negative Emissions: The Power of Leveraging Policy, Philanthropy, Purchasing and Investment View all 12 articles
To prevent the global average temperature from increasing more than 1.5°C and lower the concentration of greenhouse gases (GHGs) in the atmosphere, most emissions trajectories necessitate the implementation of strategies that include both GHG mitigation and negative emissions technologies (NETs). For NETs, there are unique research challenges faced by both CO2 capture and utilization to scale in an economically feasible manner. Starting with incumbent methods, wherein CO2 is recovered from a high concentration source, and moving toward CO2 capture from more widely available dilute sources, we outline how CO2 capture systems interface with downstream utilization in flow reactors. To provide a real-world point of comparison, we analyze CO2 sourcing for Air Company's CO2-to-alcohols pilot and demonstration scale deployments in Brooklyn, New York, USA and Calgary, Alberta, Canada as case studies. The degree of reduction in atmospheric CO2 depends on product alcohol usage; for example, use as a fixed chemical feedstock provides longer term emissions reduction than as a fuel, which is eventually oxidized. Lastly, we discuss the barriers that are present for economic scale-up of CO2 capture and utilization technologies broadly.
Anthropogenic climate change is perhaps the most significant existential challenge that humanity faces today (Mora et al., 2018; Gills and Morgan, 2020). A rapid increase in utilization of fossil fuels since the industrial revolution has increased the concentration of greenhouse gases (GHGs) in the atmosphere at a faster rate than has been observed previously (Peng et al., 1983; Etheridge et al., 1996; Lacis et al., 2010). The overwhelming majority of scientific evidence points to this increase in atmospheric GHGs, specifically carbon dioxide, being the cause of the changing global climate (Oreskes, 2004; Hartmann et al., 2013). Historically, there has been an equilibrium between CO2 sequestration via photosynthesis and CO2 emissions by biodegradation and other natural mechanisms that gradually removed CO2 from the atmosphere, transforming Earth's atmosphere into the habitable one that we now rely on (Des Marais, 2000; Gonzalez Hernandez and Sheehan, 2020). Burning fossil fuels to power today's society introduces a new, rapid flux of CO2 into the atmosphere that natural photosynthesis can no longer compensate (Grace, 2004; Le Quéré et al., 2018).
Renewable technologies must be developed and deployed to bring equilibrium back to the global carbon cycle (Holdren et al., 1980; Goreau, 1990; Gielen et al., 2019). There are several renewable approaches to CO2 emissions mitigation, and ultimately the planet will require a diversified portfolio as no single technology could reasonably abate global CO2 emissions alone (Moriarty and Honnery, 2012; Fasihi et al., 2019; Realmonte et al., 2019). Many viable solutions rely on the increased utilization of renewable (wind, solar, hydroelectric, and others) energy with low lifecycle CO2 emissions intensity (Sims, 2004). Displacing fossil fuel-based electricity generation with renewables indirectly reduces GHG emissions by preventing them from being emitted in the first place. Carbon capture and storage (CCS) technologies, on the other hand, provide direct CO2 emissions reduction by sequestering CO2 from anthropogenic sources or from the air (Snæbjörnsdóttir et al., 2020). Lastly, carbon capture and utilization (CCU) affords both direct and indirect emissions reduction by directly utilizing captured CO2 as a reactant to make a product, which in turn displaces the product's fossil-derived counterpart. Together, the latter two technologies are known as carbon capture, utilization, and storage (CCUS). CCUS is a subset of negative emissions technologies (NETs), which is defined for the purposes of this article as technologies that reduce atmospheric GHG concentrations below the concentration that would occur without the technology (McLaren, 2012).
The utility of NETs increases over time as we implement measures to reduce global GHG emissions. In today's world where coal is still burned for electricity, it is in many cases more advantageous to replace these most polluting emitters with renewable energy to prevent their CO2 emissions in the first place (Gaffney et al., 2020). Once the “lowest hanging fruit” is taken by removing the worst emitters, the relative difficulty of mitigating CO2 increases. At this intermediate point, the utility of NETs to capture CO2 from clean emitters is comparable to deploying additional indirect CO2 emissions reduction measures. Ultimately, when most electricity generation is done renewably (which introduces additional energy storage challenges that further enable several CCU technologies) there will still be substantial CO2 emissions in several areas, such as the chemical and aerospace industries, agriculture, aviation, and cement production (Fasihi et al., 2019). At this point, NETs will be required to maintain equilibrium in the global carbon cycle. As of 2020, we need to remove more than 170 gigatons of CO2 from the atmosphere to remain under the target of 1.5°C of warming by 2100 (Johansson et al., 2020). This is the equivalent of more than 5 times the total amount of CO2 emitted globally in 2019 (Friedlingstein et al., 2020).
Currently, the most widely commercialized systems that capture CO2 recover it from a high-purity source, such as hydrogen production or sugar fermentation (de Assis Filho et al., 2013). These CO2 recovery (CR) systems utilize feed streams with typically >95% CO2 concentration, requiring minimal impurity removal to increase the concentration to >99%, while compressing to provide a liquefied CO2 product for ease of transport and use. In contrast, point source CO2 capture (PSC) uses a more dilute feed stream, such as natural gas flue gas which is ~4–16% CO2 in N2 and O2 from the air (Jiang et al., 2019). A testament to the energetic implications of CO2 concentration on NET efficiency, much PSC research focuses on source gases with higher CO2 concentrations (Li et al., 2011; Baker et al., 2017). Lower concentration feed streams necessitate use of a higher volume sorption system, and typically uses the industry standard monoethanolamine (MEA) CO2 scrubber. Direct air capture (DAC), on the other hand, captures CO2 from the air at ~416 ppm which is much more energetically challenging than either CR or PSC (Ren et al., 2021). A key differentiator between these technologies is the concentration of CO2 in their source material, which dictates the feedstock mass and energy required for separation.
In this article, we present an analysis of CO2 capture technologies as they interface with downstream continuous flow CO2 utilization systems. We provide an industrial perspective by discussing the advantages and challenges to deploying flow reactors downstream from CR at high-purity point sources in the Air Company pilot reactor in New York, as compared to PSC from the flue gas of a natural gas-fired power plant using an amine absorption-based system at the Air Company demonstration reactor in Calgary. In doing so, we highlight the trajectory for deployment of CO2 capture technologies more broadly, when coupled with utilization as these emerging fields and respective technologies scale. Lastly, we discuss the lessons learned from these deployments as they relate to integrated CO2 capture and utilization systems and their associated technological and infrastructural barriers.
All three major categories of CO2 capture technologies are subject to the same development constraints as other chemical processes. To progress from conceptual idea, to a proof of concept, followed by a benchtop prototype, then pilot, demonstration, and small commercial plant is highly capital intensive and requires research and development infrastructure of its own. As they are both still the subject of heavy R&D, the economics for both PSC and DAC do not reach the low cost point that CR has achieved. Given the long chemical scale-up and development cycles and the fundamentally higher energy requirement for PSC and DAC, CR is the lowest hanging fruit today as a CO2 source to provide suitable feedstock for CO2 utilization. On the other hand, CR has the lowest potential for scale and long-term CO2 emissions reduction since there are limited sources; many are dependent on industries powered by fossil fuels that can be replaced with renewable alternatives, such as H2 production (Table 1).
Table 1. Typical reported energy consumption and potential scale for CO2 capture technologies as defined by their source concentration.
CR acts on CO2-rich gases produced during processes such as fermentation and is fully commercially available (Haszeldine et al., 2018). Due to its source gas containing >90% CO2, it can function without high thermal energy input to capture and release CO2 from a sorbent and is typically fully powered by electricity. This gives it the best economics of all the CO2 capture technologies today, as evidenced by its widespread use to produce CO2 for sale. Due to its high concentration feedstock, CR is the most entropically and energetically favorable CO2 capture technology by a large margin as shown in Table 1. However, because CR has the lowest potential for scale for global CO2 removal, it is insufficient to meet decarbonization goals over the long term if not used in tandem with PSC and DAC. Due in part to its low potential for scale and need for future technological improvements, there is a dearth of scientific literature and policy-based focus on CR. For this reason, it makes the most pragmatic sense to prioritize deployment of CR in locations where there are concentrated CO2 streams being emitted as the lowest-hanging fruit in the NET portfolio. These deployments can be done rapidly while simultaneously continuing to scale PSC and DAC technologies.
PSC predominantly acts on post-combustion point sources, such as natural gas combined cycle (NGCC) plants with CO2 concentrations of 4–16% (Jiang et al., 2019; see Table 1). Currently, PSC is operating at industrial scales, but its poor economics prevent widespread deployment, prompting further R&D in laboratories and pilot plants to reduce capital and energy costs (Table 2). Several PSC pilots show significant promise to further these goals, with innovations such as corrosion inhibitors helping to reduce heat duty from 5.0 to 1.8 GJ/ton CO2 and with projected return on investment within 2.5 years (Idem et al., 2015; Shirmohammadi et al., 2018). Current challenges are centered around optimizing adsorbent capacity at the high temperatures and low CO2 concentrations present in flue gas streams (Divekar et al., 2020). Unfortunately, many otherwise promising improvements in PSC (including selective membranes in combined cycles reaching 90% capture rates) require expensive retrofitting of plants, which may deter commercial deployment (Turi et al., 2017).
Table 2. Summary of technologies under R&D to improve the economics of PSC, including their current stage of development and reported technology readiness level (TRL).
PSC typically uses the byproduct gas of fossil fuel combustion as a source of CO2, raising concerns that overreliance on PSC may enable continued fossil fuel dependence. In contrast, DAC has been proposed as a mechanism that minimizes the need for infrastructural change or fossil fuel dependence from its inception (Lackner et al., 1999). As DAC removes CO2 from air, with a concentration that is orders of magnitude lower than that of CR and PSC, it requires the highest energy input of the technologies studied (Breyer et al., 2019). One of its strengths as a component of a diversified portfolio of NETs is in offsetting distributed emissions, such as those from aviation and agriculture. Areas for improvement of DAC technology primarily focus on decreasing energy requirements for CO2 desorption (see Table 3). Of the high temperature and low temperature DAC systems, low temperature thus far achieves lower heat supply costs (Fasihi et al., 2019). Thicker absorbent films, thinner monolithic walls, and adsorbents with higher efficiency at ambient air conditions can decrease the required energy of temperature vacuum swing adsorption (Sinha et al., 2017).
Table 3. Selected technologies under R&D to improve the economics of DAC, including current stage of development and reported TRL.
Critics argue that both PSC and DAC delay an inevitable transition to renewables, and thus increase the societal costs associated with pollution (Jacobson, 2019, 2020). There are scenarios in which this concern is understandable; some DAC deployment trajectories require a quarter of global energy demand by the end of the century (Realmonte et al., 2019). However, the warming targets in the Paris Agreement can only be met if NETs are part of the portfolio of climate solutions deployed (Haszeldine et al., 2018), making it imperative to deploy both low-carbon energy generation and NETs. The timing and trajectory of NET deployment is critical to reconcile both sides of the discussion. In the near term, NETs have the most impact by displacing the processes that are both most CO2 intensive, thereby maximizing both direct and indirect CO2 emissions, and have no renewable replacement in the foreseeable future.
We propose a trajectory for deployment of CO2 capture technologies that follows this approach, and examples on the pilot and commercial demonstration scales using continuous flow CO2 conversion systems are described below. The stranded sources of concentrated CO2 are urgent to capture, but not as important in the long-term as our ability to remove CO2 from the air. Correspondingly, the energy and capital intensity of DAC make it more economic to first pursue lower-hanging fruit, especially when today's renewable energy infrastructure does not provide adequate heat to power DAC systems without burning fossil fuels (Holmes et al., 2013; Keith et al., 2018), but it is critical that large-scale R&D efforts lower these expenses in the future. Proper timing for both renewable electricity and NETs is critical to maximize societal benefit.
Air Company's CO2-to-alcohols commercial pilot plant in Brooklyn, New York is a distillery that utilizes CO2 delivered from CR-sourced sites, including fermentation facilities, to produce ethanol. The ethanol is distilled, mixed, and bottled on-site to produce spirits and hand sanitizer. The facility is powered by a mixture of offsite wind turbines and utility-scale solar photovoltaics, enabling ethanol production from CO2, H2O, and renewable electricity. Figure 1A is a photograph of Air Company's CO2 conversion pilot plant in the facility, with a NEL H-series H2O electrolysis system and a fixed bed flow reactor for CO2 hydrogenation. A flow chart of the process can be seen in Figure 1B; in brief, CO2 and H2 are compressed, heated, and fed into the 16-foot fixed bed flow reactor. The reactor is filled with a novel and proprietary heterogeneous catalyst that has not yet been reported in literature and is developed and synthesized on the kg-scale in Air Company's facilities, enabling stable and continuous conversion of CO2 and H2 into ethanol. The gaseous products are passed through a condenser assembly, which separates the room-temperature crude ethanol aqueous liquid and gases (Sarp et al., 2021). Room temperature gases are recycled into the reactor for further conversion. The facility and systems are further adaptable to accommodate CO2 electrolysis when that technology is at an appropriate commercial stage of development (Chen et al., 2018).
Figure 1. Air Company pilot plant in New York that utilizes CO2 sourced from CR, (A) photograph of the electrolyzer for H2 production (left) and CO2 hydrogenation system (right), and (B) block flow diagram of the processes taking place in the facility.
After the CO2 conversion process, the crude ethanol is filtered and distilled to produce a neutral spirit that is ~95% ethanol by volume, the remaining 5% being water with <300 ppm net of all impurities by gas chromatography (GC), and meets all requirements for United States Pharmacopeia (USP) grade. In this facility, the CO2 fed into the reactor is captured offsite via CR powered by renewables, which typically requires 120 kWh/ton, together with ~30 kWh/ton for transportation. CR is a continuous flow system that delivers CO2 as a liquid at high pressure, ensuring consistent supply and eliminating concern about the rate or variability of CO2 use in flow reactors downstream.
Defining NETs as technologies that reduce atmospheric GHG concentrations below that which would occur without the technology, lifecycle assessment is based on a cradle-to-gate analysis (McLaren, 2012). Under optimal production conditions and operating at capacity, to produce 1 kg of ethanol as a functional unit, a minimum of 1.91 kg of CO2 and 0.26 kg of H2 is required. Depending on the lifecycle analysis methodology, a reasonable carbon footprint for the captured CO2 is ~ −1.78 kgCO2e given that the CO2 used in the process would otherwise be emitted to the atmosphere (Müller et al., 2020). The primarily wind and solar power for the facility has average lifecycle emissions of 10 gCO2e/kWh (Sovacool, 2008). Water electrolysis consumes 81 kWh/kg H2, which equates to 0.21 kgCO2e (NEL Hydrogen, 2020). Compression, heating, cooling, and distillation are all powered by electricity or waste heat and net ~8 kWh/kg ethanol. Amortized material of construction emissions over production lifetime averages 40 gCO2e/kg ethanol, accounting for unoptimized system mass (Sheehan, 2021). This results in an estimated carbon footprint of ~-1.45 kgCO2e/kg ethanol, though a more detailed and thorough lifecycle analysis that includes cradle-to-grave considerations is the subject of a future study that is currently underway.
Especially when transportation GHG emissions are minimal as to keep the net CO2e <100 kg per ton of CO2 captured, CR is an ideal capture medium for downstream flow CO2 conversion. The CO2 is delivered as a liquid, which has a constant vapor pressure. This is helpful in flow systems if there are one or more stages of compression prior to introduction of CO2 to other reactants. The suction pressure of these compressors must remain constant for optimum operation and to ensure adequate compressor lifetime, and severe variation in inlet CO2 pressure or temperature can cause challenges that prompt plant shutdown. CR eliminates these operational variables and is economic without substantial subsidy, which makes it a model system for integration with flow CO2 conversion systems in the near-term despite its limited long-term utility.
Unlike fermentation processes, NGCC power plants release flue gas streams with CO2 concentrations of 4–16%. In this case, CO2 is a harmful byproduct of electricity production. PSC from NGCC power plants takes place today using a commercial monoethanolamine (MEA) process, in which CO2 is absorbed by liquid MEA at high pressure and low temperature and stripped from the MEA at low pressure and high temperature. Air Company's Calgary commercial CO2-to-alcohols demonstration plant is deployed at the Shepard Energy Center, an 860 MW NGCC power plant. The NGCC byproduct flue gas containing 6.6% CO2 is fed into the MEA adsorption system operated by the Alberta Carbon Conversion Technology Center (ACCTC), shown in the left on the photograph in Figure 2A. The product from the capture system, water-saturated CO2 (98%), is then pumped into the Air Company building. Tail gas is typically emitted from amine CO2 capture systems, which contains ~1.2% CO2 that is not captured because it is too energy-intensive to do so. The water content of the captured CO2 could have implications for the efficacy of the system, and a knockout drum dryer is used for its removal. After compression, the CO2 is combined with H2 and introduced into a reactor similar to, but significantly larger than, the Air Company pilot plant. While H2 was supplied via tube trailer in the interim, construction of an integrated facility with a H2O electrolyzer powered by renewable electricity is nearly complete. Table 4 shows a summary of the gas inlet parameters between the pilot and demonstration facilities.
Figure 2. Air Company plant in Calgary utilizing CO2 sourced from PSC at a NGCC power plant, (A) photograph of the CO2 conversion system, and (B) block flow diagram of the processes taking place in the facility.
Table 4. Summary of inlet parameters and approximate energy cost for CO2 capture coupled with pilot and production scale downstream flow reactors.
For the Air Company pilot and commercial demonstration facilities as well as deployments for larger CO2 utilization systems by us and others in the future, plant economics will play a major role in the technology used for CO2 capture. Our experiences suggest that the hypothesis to target the high-concentration CO2 emitters first is valid, but the GHG reduction of these sources is limited. This calls for research to reduce the capital costs, energy requirements, and improve product characteristics (e.g., temperature, pressure) for PSC and DAC at-scale. In our case, using a CR-sourced pilot reactor and PSC-sourced commercial demonstration reactor, the biggest barrier to use of DAC was the large capital expenditure for small units (on the order of 1–10 tons per day of CO2). Innovations in materials science and sorbent materials that drive down the capital cost of small DAC units would be hugely beneficial for distributed deployment, especially in industries where customers are willing to pay a substantial premium for modular and distributed DAC, thus offsetting its comparably larger operational expense.
Beyond capital expenditure and operational considerations, the necessity of retrofits for certain PSC technologies could represent key barrier to PSC deployment and receives little academic attention (Koelbl et al., 2014). Due in part to the costs of retrofits and the abundance of non-retrofittable power plants, DAC has surprisingly been identified as the less expensive option compared to PSC in one third of NGCC plants. Further select cases (e.g., microalgae cultivation) also make DAC energetically competitive despite being the furthest from commercial availability (Mangram, 2012; Wilcox et al., 2017; Azarabadi and Lackner, 2020; Hirsch and Foust, 2020). There is no fundamental reason why both DAC and PSC systems also cannot deliver product CO2 with the same consistency, pressures, and temperatures as CR to optimize integration with downstream flow reactors. Beyond facilitating retrofits, research that improves the feedstock input and CO2 output tolerances of DAC and PSC technologies could further accelerate commercialization.
Locations for geological storage and measures to mitigate leakage also represent key barriers to scaling NET outside CR, PSC, and DAC technologies themselves that must be addressed (Koelbl et al., 2014; von Strandmann et al., 2019). Since CCS does not produce a physical, saleable end product, exploration into further profitable NET opportunities, mass production and innovative infrastructural development, financial incentives, and international policy will be necessary to reach emissions targets (Honegger and Reiner, 2018; Hirsch and Foust, 2020; Olfe-Kräutlein, 2020). The high startup costs associated with early CCU deployment may potentially be overcome by following successful disruptive innovation models in electric vehicles (EVs) and EV infrastructure that move from high-end to mass markets to scale pragmatically, for example, as Tesla has done (Chen and Perez, 2018).
Ultimately, widespread CO2 capture and utilization will be needed to meet emissions targets, but these technologies alone will not save us. Real infrastructural change to facilitate an economic trajectory of CO2 capture deployment is required. Similar to the way the hardware and software for EVs existed prior to its currently accelerating adoption due to cultural and political changes, these fundamental pieces exist for CO2 capture. CO2 utilization technologies now provide an additional incentive to build the required infrastructure on local and global scales.
The original contributions presented in the study are included in the article/supplementary material, further inquiries can be directed to the corresponding author.
GP performed research on CO2 capture technologies, compiled information in Tables 1, 2, and drafted the main body of the article. SS conceptualized the research, the facilities in Figures 1, 2, and supervised the work. Both authors wrote and revised the manuscript.
GP acknowledges the New York State Energy Research and Development Authority (NYSERDA) for their Clean Energy Internship Program. SS acknowledged the Ontario Centre of Innovation for Phase 1 and 2 awards under their Solutions 2030 Challenge (Nos. 29828 and 31826), and NASA Centennial Challenges for a Phase 1 award from their CO2 Conversion Challenge.
All authors are employees of Air Company.
We thank the Air Company operations team, Gregory Constantine, Jackson Constantine, Morgan Sparkes, Nicholas Steinke, Jodi Taylor, Joshua Anderson, Santiago Gonzalez Hernandez, Dr. Chi Chen, and Dean Pawulski, for their work on the facilities described in this article. We also acknowledge Dr. Marcius Extavour and the NRG COSIA Carbon XPrize team, Dr. John Sirman, and Dr. Aref Najafi for their discussions and support of CCUS technologies.
Azarabadi, H., and Lackner, K. S. (2020). Postcombustion capture or direct air capture in decarbonizing US natural gas power? Environ. Sci. Technol. 54, 5102–5111. doi: 10.1021/acs.est.0c00161
Baker, R. W., Freeman, B., Kniep, J., Wei, X., and Merkel, T. (2017). CO2 capture from natural gas power plants using selective exhaust gas recycle membrane designs. Int. J. Greenhouse Gas Control. 66, 35–47. doi: 10.1016/j.ijggc.2017.08.016
Breyer, C., Fasihi, M., Bajamundi, C., and Creutzig, F. (2019). Direct air capture of CO2: a key technology for ambitious climate change mitigation. Joule 3, 2053–2057. doi: 10.1016/j.joule.2019.08.010
Broehm, M., Strefler, J., and Bauer, N. (2015). Techno-economic review of direct air capture systems for large scale mitigation of atmospheric CO2. Available at SSRN 2665702. doi: 10.2139/ssrn.2665702
Chen, C., Kotyk, J. F. K., and Sheehan, S. W. (2018). Progress toward commercial application of electrochemical carbon dioxide reduction. Chem 4, 2571–2586. doi: 10.1016/j.chempr.2018.08.019
Chen, Y., and Perez, Y. (2018). “Business model design: lessons learned from Tesla Motors,” in Towards a Sustainable Economy, ed P. da Costa, and D. Attias (Cham: Springer), 53–69. doi: 10.1007/978-3-319-79060-2_4
de Assis Filho, R. B., Danielski, L., de Carvalho, F. R., and Stragevitch, L. (2013). Recovery of carbon dioxide from sugarcane fermentation broth in the ethanol industry. Food Bioprod. Process. 91, 287–291. doi: 10.1016/j.fbp.2012.09.009
Des Marais, D. J. (2000). When did photosynthesis emerge on Earth? Science 289, 1703–1705. doi: 10.1126/science.289.5485.1703
Divekar, S., Dasgupta, S., Arya, A., Gupta, P., Singh, S., and Nanoti, A. (2020). Improved CO2 recovery from flue gas by layered bed Vacuum Swing Adsorption (VSA). Sep. Purif. Technol. 234, 115594. doi: 10.1016/j.seppur.2019.05.036
Etheridge, D. M., Steele, L. P., Langenfelds, R. L., Francey, R. J., Barnola, J. M., and Morgan, V. I. (1996). Natural and anthropogenic changes in atmospheric CO2 over the last 1000 years from air in Antarctic ice and firn. J. Geophys. Res. Atmos. 101, 4115–115528. doi: 10.1029/95JD03410
Fasihi, M., Efimova, O., and Breyer, C. (2019). Techno-economic assessment of CO2 direct air capture plants. J. Clean. Prod. 224, 957–980. doi: 10.1016/j.jclepro.2019.03.086
Fitzgerald, F. D., Hume, S. A., McGough, G., and Damen, K. (2014). Ferrybridge CCPilot100+ operating experience and final test results. Energy Procedia 63, 6239–6251. doi: 10.1016/j.egypro.2014.11.655
Friedlingstein, P., O'Sullivan, M., Jones, M. W., Andrew, R. M., Hauck, J., Olsen, A., et al. (2020). Global carbon budget 2020. Earth Syst. Sci. Data 12, 3269–3340. doi: 10.5194/essd-12-3269-2020
Gaffney, F., Deane, J. P., Drayton, G., Glynn, J., and Gallachóir, B. P. (2020). Comparing negative emissions and high renewable scenarios for the European power system. BMC Energy 2, 1–3. doi: 10.1186/s42500-020-00013-4
Gielen, D., Boshell, F., Saygin, D., Bazilian, M. D., Wagner, N., and Gorini, R. (2019). The role of renewable energy in the global energy transformation. Energy Strategy Rev. 24, 38–50. doi: 10.1016/j.esr.2019.01.006
Gills, B., and Morgan, J. (2020). Global climate emergency: after COP24, climate science, urgency, and the threat to humanity. Globalizations 17, 885–902. doi: 10.1080/14747731.2019.1669915
Goeppert, A., Czaun, M., Prakash, G. S., and Olah, G. A. (2012). Air as the renewable carbon source of the future: an overview of CO2 capture from the atmosphere. Energy Environ. Sci. 5, 7833–7853. doi: 10.1039/c2ee21586a
Gonzalez Hernandez, S., and Sheehan, S. W. (2020). Comparison of carbon sequestration efficacy between artificial photosynthetic carbon dioxide conversion and timberland reforestation. MRS Energy Sust. 7:32. doi: 10.1557/mre.2020.32
Grace, J. (2004). Understanding and managing the global carbon cycle. J. Ecol. 92, 189–202. doi: 10.1111/j.0022-0477.2004.00874.x
Guo, H., Li, C., Shi, X., Li, H., and Shen, S. (2019). Nonaqueous amine-based absorbents for energy efficient CO2 capture. Appl. Energy 239, 725–734. doi: 10.1016/j.apenergy.2019.02.019
Hartmann, D. L., Tank, A. M., Rusticucci, M., Alexander, L. V., Brönnimann, S., Charabi, Y. A., et al. (2013). “Observations: atmosphere and surface,” in Climate Change 2013 the Physical Science Basis: Working Group I Contribution to the Fifth Assessment Report of the Intergovernmental Panel on Climate Change (Cambridge: Cambridge University Press), 159–254.
Haszeldine, R. S., Flude, S., Johnson, G., and Scott, V. (2018). Negative emissions technologies and carbon capture and storage to achieve the Paris Agreement commitments. Philos. Trans. R. Soc. A. 376:20160447. doi: 10.1098/rsta.2016.0447
Hirsch, E., and Foust, T. (2020). Policies and programs available in the United States in support of carbon capture and utilization. Energy L. J. 41:91. Available online at: https://heinonline.org/HOL/LandingPage?handle=hein.journals/energy41&div=13&id=&page=
Holdren, J. P., Morris, G., and Mintzer, I. (1980). Environmental aspects of renewable energy sources. Ann. Rev. Energy 5, 241–291.
Holmes, G., Nold, K., Walsh, T., Heidel, K., Henderson, M. A., Ritchie, J., et al. (2013). Outdoor prototype results for direct atmospheric capture of carbon dioxide. Energy Procedia 37, 6079–6095. doi: 10.1016/j.egypro.2013.06.537
Honegger, M., and Reiner, D. (2018). The political economy of negative emissions technologies: consequences for international policy design. Clim. Policy 18, 306–321. doi: 10.1080/14693062.2017.1413322
Idem, R., Supap, T., Shi, H., Gelowitz, D., Ball, M., Campbell, C., et al. (2015). Practical experience in post-combustion CO2 capture using reactive solvents in large pilot and demonstration plants. Int. J. Greenhouse Gas. Control. 40, 6–25. doi: 10.1016/j.ijggc.2015.06.005
Ishimoto, Y., Sugiyama, M., Kato, E., Moriyama, R., Tsuzuki, K., and Kurosawa, A. (2017). Putting costs of direct air capture in context. FCEA Working Paper Series: 002. doi: 10.2139/ssrn.2982422
Jacobson, M. Z. (2019). The health and climate impacts of carbon capture and direct air capture. Energy Environ. Sci. 12, 3567–3574. doi: 10.1039/C9EE02709B
Jacobson, M. Z. (2020). “Evaluation of coal and natural gas with carbon capture as proposed solutions to global warming, air pollution, and energy security,” in 100% Clean, Renewable Energy and Storage for Everything (New York, NY: Cambridge University Press), 347–388. doi: 10.1017/9781108786713
Jiang, L., Gonzalez-Diaz, A., Ling-Chin, J., Roskilly, A. P., and Smallbone, A. J. (2019). Post-combustion CO2 capture from a natural gas combined cycle power plant using activated carbon adsorption. Appl. Energy 245, 1–5. doi: 10.1016/j.apenergy.2019.04.006
Johansson, D. J., Azar, C., Lehtveer, M., and Peters, G. P. (2020). The role of negative carbon emissions in reaching the Paris climate targets: the impact of target formulation in integrated assessment models. Environ. Res. Lett. 15:124024. doi: 10.1088/1748-9326/abc3f0
Keith, D. W., Holmes, G., Angelo, D. S., and Heidel, K. (2018). A process for capturing CO2 from the atmosphere. Joule 2, 1573–124094. doi: 10.1016/j.joule.2018.05.006
Koelbl, B. S., van den Broek, M. A., Faaij, A. P., and van Vuuren, D. P. (2014). Uncertainty in carbon capture and storage (CCS) deployment projections: a cross-model comparison exercise. Clim. Change 123, 461–476. doi: 10.1007/s10584-013-1050-7
Lacis, A. A., Schmidt, G. A., Rind, D., and Ruedy, R. A. (2010). Atmospheric CO2: principal control knob governing earth's temperature. Science 330, 356–359. doi: 10.1126/science.1190653
Lackner, K., Ziock, H. J., and Grimes, P. (1999). Carbon Dioxide Extraction From Air: Is It an Option? Santa Fe, NM: Los Alamos National Lab.
Lackner, K. S. (2013). The thermodynamics of direct air capture of carbon dioxide. Energy 50, 38–46. doi: 10.1016/j.energy.2012.09.012
Le Quéré, C., Andrew, R. M., Friedlingstein, P., Sitch, S., Hauck, J., Pongratz, J., et al. (2018). Global carbon budget 2018. Earth Syst. Sci. Data 10, 2141–2194. doi: 10.5194/essd-10-2141-2018
Lee, W. R., Hwang, S. Y., Ryu, D. W., Lim, K. S., Han, S. S., et al. (2014). Diamine-functionalized metal–organic framework: exceptionally high CO2 capacities from ambient air and flue gas, ultrafast CO2 uptake rate, and adsorption mechanism. Energy Environ. Sci. 7, 744–751. doi: 10.1039/C3EE42328J
Li, H., Ditaranto, M., and Berstad, D. (2011). Technologies for increasing CO2 concentration in exhaust gas from natural gas-fired power production with post-combustion, amine-based CO2 capture. Energy 36, 1124–1133. doi: 10.1016/j.energy.2010.11.037
Lim, J., Choi, W., Mok, J., and Seo, Y. (2018). Clathrate-based CO2 capture from CO2-rich natural gas and biogas. ACS Sus. Chem. Eng. 6, 5627–5635. doi: 10.1021/acssuschemeng.8b00712
Mangram, M. E. (2012). The globalization of Tesla Motors: a strategic marketing plan analysis. J. Strateg. Mark. 20, 289–312. doi: 10.1080/0965254X.2012.657224
McLaren, D. (2012). A comparative global assessment of potential negative emissions technologies. Process Saf. Environ. Prot. 90, 489–500. doi: 10.1016/j.psep.2012.10.005
Möllersten, K., Yan, J., and Moreira, J. R. (2003). Potential market niches for biomass energy with CO2 capture and storage—opportunities for energy supply with negative CO2 emissions. Biomass Bioenerg. 25, 273–285. doi: 10.1016/S0961-9534(03)00013-8
Mora, C., Spirandelli, D., Franklin, E. C., Lynham, J., Kantar, M. B., Miles, W., et al. (2018). Broad threat to humanity from cumulative climate hazards intensified by greenhouse gas emissions. Nat. Clim. Change 8, 1062–1071. doi: 10.1038/s41558-018-0315-6
Moriarty, P., and Honnery, D. (2012). What is the global potential for renewable energy? Renew. Sust. Energy Rev. 16, 244–252. doi: 10.1016/j.rser.2011.07.151
Müller, L. J., Kätelhön, A., Bringezu, S., McCoy, S., Suh, S., Edwards, R., et al. (2020). The carbon footprint of the carbon feedstock CO2. Energy Environ. Sci. 13, 2979–2992. doi: 10.1039/d0ee01530j.
NEL Hydrogen (2020). H Series Hydrogen Generation Systems Specification Sheet, Revision G. Available online at: https://nelhydrogen.com/wp-content/uploads/2020/03/H-Series-Spec-Sheet-Rev-G.pdf (accessed March 29, 2021).
Olfe-Kräutlein, B. (2020). Advancing CCU technologies pursuant to the SDGs: a challenge for policy making. Front. Energy Res. 8:198. doi: 10.3389/fenrg.2020.00198
Olivier, J. G., Schure, K. M., and Peters, J. A. (2017). Trends in Global CO2 and Total Greenhouse Gas Emissions. The Hague: PBL Netherlands Environmental Assessment Agency.
Oreskes, N. (2004). The scientific consensus on climate change. Science 306, 1686. doi: 10.1126/science.1103618
Park, J., Suh, B. L., and Kim, J. (2020). Computational design of a photoresponsive metal–organic framework for post combustion carbon capture. J. Phys. Chem. C 124, 13162–13167. doi: 10.1021/acs.jpcc.0c01878
Peng, T. H., Broecker, W. S., Freyer, H. D., and Trumbore, S. (1983). A deconvolution of the tree ring based δ13C record. J. Geophys. Res. Oceans 88, 3609–3620. doi: 10.1029/JC088iC06p03609
Realmonte, G., Drouet, L., Gambhir, A., Glynn, J., Hawkes, A., Köberle, A. C., et al. (2019). An inter-model assessment of the role of direct air capture in deep mitigation pathways. Nat. Commun. 10, 1–2. doi: 10.1038/s41467-019-10842-5
Ren, S., Aldahri, T., Liu, W., and Liang, B. (2021). CO2 mineral sequestration by using blast furnace slag: from batch to continuous experiments. Energy 214:118975. doi: 10.1016/j.energy.2020.118975
Sadiq, M. M., Batten, M. P., Mulet, X., Freeman, C., Konstas, K., Mardel, J. I., et al. (2020). A pilot-scale demonstration of mobile direct air capture using metal-organic frameworks. Adv. Sust. Syst. Adv. Sust. Syst. 4:2000101. doi: 10.1002/adsu.202000101
Sarp, S., Gonzalez Hernandez, S., Chen, C., and Sheehan, S. W. (2021). Alcohol production from carbon dioxide: methanol as a fuel and chemical feedstock. Joule 5, 59–76. doi: 10.1016/j.joule.2020.11.005
Sheehan, S. W. (2021). Electrochemical methane production from CO2 for orbital and interplanetary refueling. iScience 24:102230. doi: 10.1016/j.isci.2021.102230
Shirmohammadi, R., Soltanieh, M., and Romeo, L. M. (2018). Thermoeconomic analysis and optimization of post-combustion CO2 recovery unit utilizing absorption refrigeration system for a natural-gas-fired power plant. Environ. Prog. Sust. Energy 37, 1075–1084. doi: 10.1002/ep.12866
Sims, R. E. (2004). Renewable energy: a response to climate change. Sol. Energy 76, 9–17. doi: 10.1016/S0038-092X(03)00101-4
Sinha, A., Darunte, L. A., Jones, C. W., Realff, M. J., and Kawajiri, Y. (2017). Systems design and economic analysis of direct air capture of CO2 through temperature vacuum swing adsorption using MIL-101 (Cr)-PEI-800 and mmen-Mg2 (dobpdc) MOF adsorbents. Ind. Eng. Chem. Res. 56, 750–764. doi: 10.1021/acs.iecr.6b03887
Snæbjörnsdóttir, S. Ó., Sigfússon, B., Marieni, C., Goldberg, D., Gislason, S. R., and Oelkers, E. H. (2020). Carbon dioxide storage through mineral carbonation. Nat. Rev. Earth Environ. 1, 90–102. doi: 10.1038/s43017-019-0011-8
Sovacool, B. K. (2008). Valuing the greenhouse gas emissions from nuclear power: a critical survey. Energy Policy 36, 2950–2963. doi: 10.1016/j.enpol.2008.04.017
Turi, D. M., Ho, M., Ferrari, M. C., Chiesa, P., Wiley, D. E., and Romano, M. C. (2017). CO2 capture from natural gas combined cycles by CO2 selective membranes. Int. J. Greenhouse Gas Control 61, 168–183. doi: 10.1016/j.ijggc.2017.03.022
von Strandmann, P. A., Burton, K. W., Snæbjörnsdóttir, S. O., Sigfússon, B., Aradóttir, E. S., Gunnarsson, I., et al. (2019). Rapid CO2 mineralisation into calcite at the CarbFix storage site quantified using calcium isotopes. Nat. Commun. 10, 1–7. doi: 10.1038/s41467-019-10003-8
Voskian, S., and Hatton, T. A. (2019). Faradaic electro-swing reactive adsorption for CO2 capture. Energy Environ. Sci. 12, 3530–3547. doi: 10.1039/C9EE02412C
Wilcox, J., Psarras, P. C., and Liguori, S. (2017). Assessment of reasonable opportunities for direct air capture. Environ. Res. Lett. 12:065001. doi: 10.1088/1748-9326/aa6de5
Keywords: carbon dioxide utilization, direct air capture, carbon recovery, carbon capture, solar fuels, emissions to liquids, ethanol, flow chemistry
Citation: Pace G and Sheehan SW (2021) Scaling CO2 Capture With Downstream Flow CO2 Conversion to Ethanol. Front. Clim. 3:656108. doi: 10.3389/fclim.2021.656108
Received: 21 January 2021; Accepted: 06 April 2021;
Published: 06 May 2021.
Edited by:
Phil Renforth, Heriot-Watt University, United KingdomReviewed by:
Volker Sick, University of Michigan, United StatesCopyright © 2021 Pace and Sheehan. This is an open-access article distributed under the terms of the Creative Commons Attribution License (CC BY). The use, distribution or reproduction in other forums is permitted, provided the original author(s) and the copyright owner(s) are credited and that the original publication in this journal is cited, in accordance with accepted academic practice. No use, distribution or reproduction is permitted which does not comply with these terms.
*Correspondence: Stafford W. Sheehan, c3RhZmZAYWlyY29tcGFueS5jb20=
Disclaimer: All claims expressed in this article are solely those of the authors and do not necessarily represent those of their affiliated organizations, or those of the publisher, the editors and the reviewers. Any product that may be evaluated in this article or claim that may be made by its manufacturer is not guaranteed or endorsed by the publisher.
Research integrity at Frontiers
Learn more about the work of our research integrity team to safeguard the quality of each article we publish.