- 1Bioresource Science and Engineering Group, Environmental Sciences Division, Oak Ridge National Laboratory, Oak Ridge, TN, United States
- 2Natural Resource Ecology Laboratory, Colorado State University, Fort Collins, CO, United States
Climate stabilization plans rely heavily on advanced bioenergy and bioproducts for substitution of fossil-based energy sources and materials, and increasingly, for negative emissions via the direct sequestration of biogenic carbon. Yet, there remain persistent, largely unresolved critiques of bioenergy assessment methodology, particularly in the areas of land use and biogenic carbon accounting. The concept of “additional carbon” calls for evaluating the climate performance of bio-based systems by whether feedstock production creates measurable new local agro-ecosystem uptake of carbon from the atmosphere. This concept is challenging to operationalize for first-generation biofuels, and has largely been advanced as a negative critique. However, carbon additionality is more straightforward to establish—and less critical to overall system mitigation performance—in advanced bioenergy systems. In this Perspective, I review the additional carbon critique, and why it is analytically challenging to address in first-generation biofuel systems based on conventional food crops with large existing markets. Next, I make a case that carbon additionality (1) is more readily achievable with cellulosic feedstocks, (2) is more directly observable for dedicated biomass crops, and (3) is not a strict requirement for achieving net mitigation in carbon-negative bio-based systems. I end by discussing how centering atmosphere–ecosystem carbon exchanges in bio-based system assessment could create new opportunities for enterprise-scale performance monitoring and verification, augmenting and diversifying the current reliance on model-based life-cycle assessment approaches.
Introduction
While electrification and renewable electricity generation have made great headway in recent years, more than a quarter of all energy-related emissions will likely require a different decarbonization approach (Davis et al., 2018). Additionally, after years of accelerating greenhouse gas (GHG) emissions, most scenarios for achieving the temperature targets of the Paris Agreement now also include the wide-scale deployment of negative emissions (Vuuren et al., 2018). It is expected that biomass will pay a key role in climate stabilization as a feedstock for renewable transportation fuels (Fulton et al., 2015), industrial heat and power (Butnar et al., 2020), carbon-negative energy production (Fuss et al., 2014), and bio-product manufacturing (Fuhrman et al., 2020) in some combination.
Biofuels and bioenergy production are among the most well-studied bio-based systems, and have been a leading topic of life-cycle assessment (LCA) research and methodological development for more than four decades (Silva et al., 1978). However, there remains significant controversy around the climate change mitigation value of such systems, particularly with respect to land use and feedstock production (DeCicco and Schlesinger, 2018). Conventional LCA is a bottom-up approach that seeks to tabulate all cradle-to-grave GHG emissions associated with the supply chain of providing a good or service. If the total life-cycle emissions of bioenergy production and use are less than that of the competing conventional fossil-derived energy source, then emissions savings (mitigation) are inferred when bioenergy use replaces the fossil energy source. Emissions of biomass-derived “biogenic” CO2 from bioenergy conversion and end use are often assumed to be carbon-neutral a priori (DeCicco et al., 2016), on the grounds that such carbon was recently fixed from the atmosphere during feedstock production, and an equivalent amount of carbon will be fixed again when the feedstock is subsequently re-grown. Changes in land use or land management for feedstock production are accounted for in terms of changes in above- or belowground ecosystem carbon stocks (Sheehan et al., 2003; Fargione et al., 2008), but biogenic carbon fluxes from the atmosphere into the feedstock and then back to the atmosphere during conversion and use are usually presupposed, or excluded from emissions accounting entirely.
Carbon Additionality
The concept of “additional carbon” suggests that the mitigation value of a bioenergy system is fundamentally dependent on, and should be evaluated explicitly in terms of, increased net photosynthetic uptake of atmospheric carbon in feedstock-producing agro-ecosystems (Searchinger, 2010; DeCicco, 2013; Haberl, 2013). Carbon uptake is usually understood to specifically mean net ecosystem production (NEP) (DeCicco, 2013). “A fundamental property of ecosystems” (Lovett et al., 2006) and “a central concept in C-cycling research” (Chapin et al., 2006), NEP reflects the difference between gross photosynthetic carbon uptake (i.e., gross primary production, or GPP) and carbon losses via ecosystem respiration (Re):
It can alternately be defined in terms of net primary production (NPP, i.e., net photosynthetic uptake by plants after correcting for their autotrophic respiration) and heterotrophic respiration (Rh):
In systems with negligible inorganic carbon sources or sinks, NEP represents the total net CO2-C uptake from the atmosphere by the ecosystem. Note however that the net ecosystem carbon balance (NECB) of an agricultural system is also affected by removals of carbon through the harvest (Harv) of grain or biomass:
Proponents of carbon additionality assessment suggest that simplistic a priori assumptions of biomass carbon neutrality can mask carbon accounting baseline errors or unintended consequences from bioenergy systems. Production of first-generation biofuels from corn, soy, or sugarcane in the absence of additional NEP suggests that these feedstocks are simply being diverted from existing commodity markets. This undermines the basis of mitigation claims from such systems, and could lead to unintended consequences from compensatory agricultural extensification or intensification elsewhere [e.g., indirect land use change (ILUC)], or an overall reduction in food calorie production (Searchinger et al., 2015). Production of advanced bioenergy from cellulosic biomass feedstocks without increased NEP suggests that carbon is being “mined” from feedstock-producing ecosystems or sourced at the expense of future ecosystem carbon sequestration, and thus the benefits of reduced fossil fuel emissions are counteracted by a reduced ecosystem carbon sink (Searchinger et al., 2017; Schlesinger, 2018).
The concept of carbon additionality is illustrated in Figure 1 by comparing reference-case (“ref”) agricultural land management and fossil coal combustion for energy (Figure 1A) to an alternative bioenergy scenario (“bio”) where coal is displaced by biomass sourced from agricultural residue collection (Figure 1B). Carbon fluxes associated with grain harvest and use for food or animal feed are assumed to be unchanged between the reference and stover-bioenergy scenarios, and thus are excluded from the accounting below for simplicity. The remaining relevant exchanges of carbon with the atmosphere in the reference case (ΔCatm,ref) consist of point-source emissions from coal combustion for energy (Eref) and net carbon uptake by agro-ecosystems during business-as-usual agricultural production (NEPref):
Conventional bioenergy systems seek to mitigate climate change through displacing fossil energy use with alternative biologically-derived energy sources. In the alternative bioenergy case, excluding upstream supply chain emissions (e.g., emissions associated with fertilizer production or farm operations) for simplicity, bioenergy feedstock production affects ecosystem carbon uptake (NEPbio), and coal emissions are replaced with emissions of biogenic carbon from biomass combustion (Ebio):
As described previously in Field et al. (2020), achieving net climate change mitigation (i.e., net reduction in atmospheric carbon load) from such a system implies:
Coal combustion releases CO2 to the atmosphere at a rate of 24.4 g C MJ−1 on a lower heating-value basis as per the GREET model (Wang, 1996). Combusting corn stover produces CO2 at a roughly equivalent rate (25 g C MJ−1, based on data from Tanger et al., 2013). Because energy system emissions are roughly equivalent in both cases (Ebio ≈ Eref), Equation (7) simplifies to:
In other words, any net mitigation from a bioenergy system is dependent on increased NEP flux from the atmosphere to the agricultural landscape where the biomass feedstock is produced. As per Equation (3), if the increase in carbon removal from new biomass harvest exceeds the increase in net carbon uptake by the system (NEP), then the biomass carbon is not fully “additional,” but rather comes at the cost of reduced ecosystem carbon storage (NECB). Thus, carbon additionality refocuses assessment from tracking changes in ecosystem carbon stocks to tracking equivalent changes in atmosphere-ecosystem carbon flux.
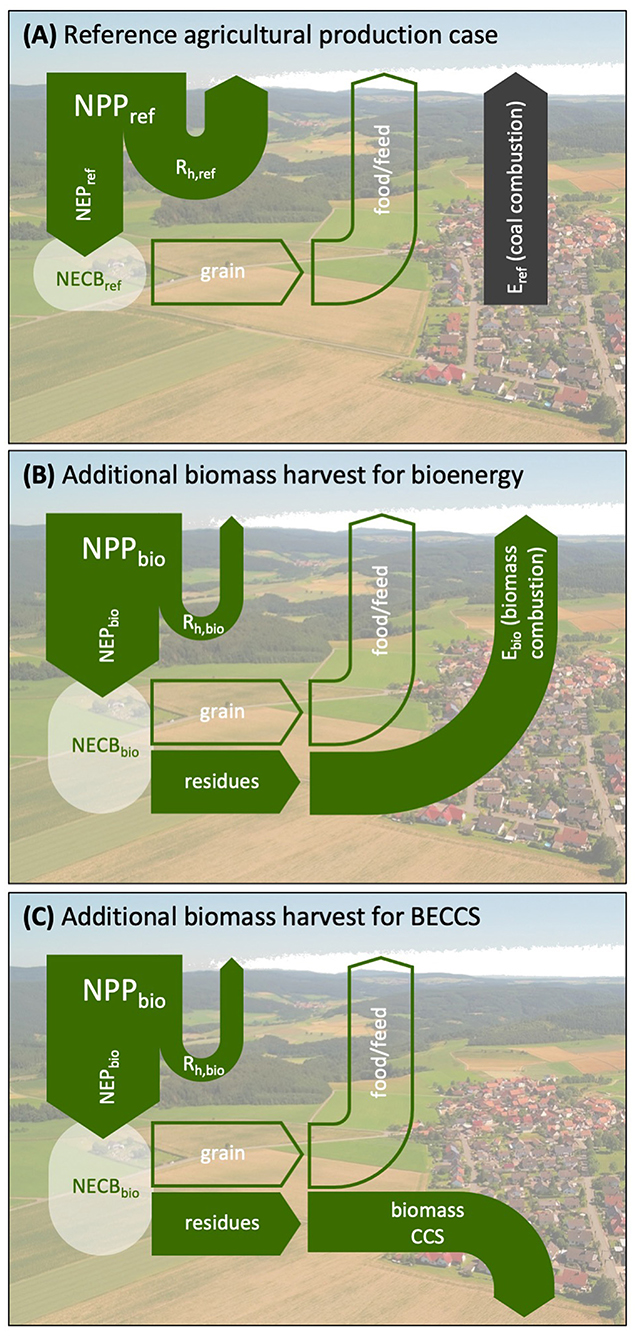
Figure 1. Carbon exchanges with the atmosphere in the production of conventional and carbon-negative bioenergy (“bio”) from new agricultural residue harvest, as compared to a reference case (“ref”). Fluxes of photosynthesis-derived “biogenic” carbon are shown in green; fossil carbon emissions from fossil fuel use in black. Net carbon exchange between the atmosphere and the agricultural landscape (net ecosystem production, or NEP) reflects the difference between net primary production (NPP) and heterotrophic respiration (Rh). (A) The reference case includes atmosphere–ecosystem fluxes from business-as-usual agricultural grain production, plus coal combustion for energy. (B) Conventional bioenergy production from biomass sourced from new agricultural residue collection, which displaces reference-case coal combustion. (C) A bioenergy with carbon capture and storage (BECCS) variant of scenario B, in which biogenic CO2 from biomass combustion is geologically sequestered.
An illustrative quantitative example is developed in Table 1. Reference-case ecosystem fluxes associated with corn grain production (“ref”) are taken from Cates and Jackson (2019) for a 3-year experiment in Wisconsin. That study estimates a NEP value of 5.6 Mg C ha−1 y−1 and grain export of 5.3 Mg C ha−1 y−1 averaged across all cover crop treatments, which together imply a small positive residual NECB (0.3 Mg C ha−1 y−1). A whole-plant silage harvest treatment from the same study is analogous to the case of adding stover harvest for bioenergy production (“bio1”). NPP was reduced slightly (−1.2 Mg C ha−1 y−1) under that management system, but carbon harvest increased by 3.4 Mg C ha−1 y−1. The measured heterotrophic respiration rate was unchanged compared to the reference case over this relatively short experimental timeframe, which implies no increase in NEP (in fact a small decrease). Over this short time horizon, there is no system-level benefit to the atmosphere from trading coal emissions for stover biomass emissions, since that stover production was not associated with additional net agro-ecosystem carbon uptake (i.e., no carbon additionality), but rather came at the cost of reduced NECB (i.e., reduced litter and soil carbon in the system).
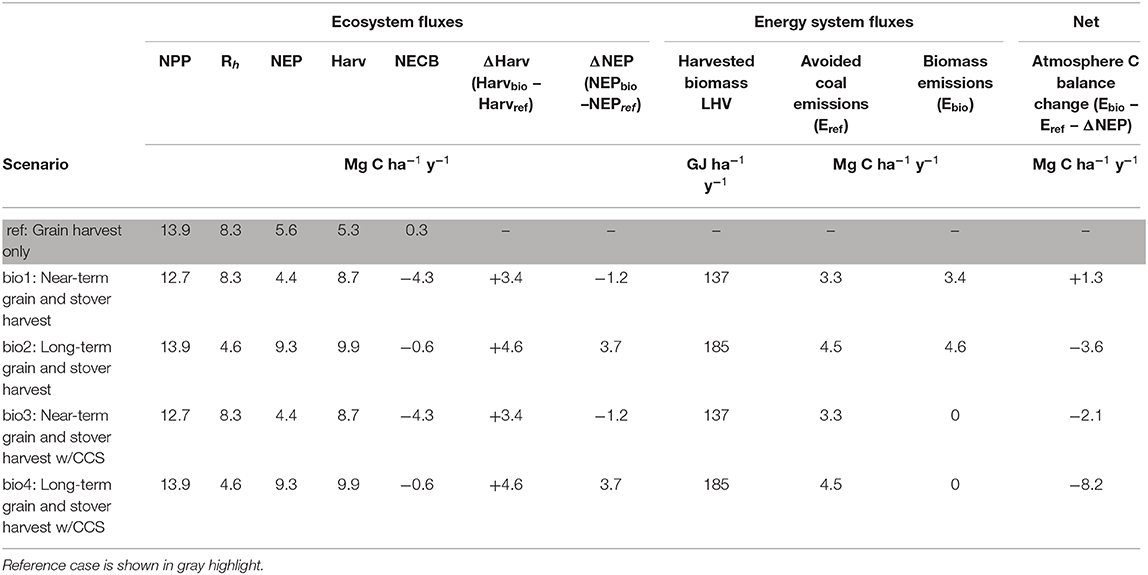
Table 1. Illustrative example of changes to ecosystem–atmosphere and energy system–atmosphere carbon fluxes in response to corn stover collection for bioenergy production.
However, over longer time-frames we would expect Rh rates to drop as soil organic matter levels reach a new equilibrium in response to stover removal (Kim et al., 2018). To construct a hypothetical longer-term equilibrium stover removal scenario (“bio2”) we assume no reduction in long-term productivity with stover harvest, and that only 20% of the carbon in harvested stover would have been stabilized as soil organic matter had it been retained. The remaining 80% of the harvested stover carbon is “additional” since it would otherwise be respired back to the atmosphere during stover decomposition, and its harvest increases agro-ecosystem NEP by decreasing Rh (Searchinger, 2010). When used for energy production, the harvested biomass carbon displaces a roughly-equivalent amount of carbon from coal combustion, and the net atmosphere carbon load is decreased by approximately the amount of long-term NEP increase from feedstock production. Note that carbon additionality is distinct from the idea of ecosystem carbon sequestration, and feedstock production can be partially additional, i.e., lead to increased local agro-ecosystem carbon uptake from the atmosphere (NEP) despite some reduction in ecosystem carbon storage (NECB, as is illustrated in the “bio2” case).
Carbon Additionality Challenges for First-Generation Biofuels
NEP and net ecosystem exchange (NEE, which is equivalent to NEP, but calculated from the perspective of the atmosphere and thus uses the opposite sign convention) have been measured in variety of bioenergy feedstock-producing landscapes via eddy covariance techniques (Skinner and Adler, 2010; Gelfand et al., 2011; Zeri et al., 2011, 2013; Drewer et al., 2012; Bernier and Paré, 2013; Zenone et al., 2013; Wagle et al., 2015; Sharma et al., 2017; Abraha et al., 2018). However, such data is seldom used directly in life-cycle assessments or other estimates of system-level GHG mitigation in bio-based systems. Though the concept of additional carbon is straightforward, its assessment in first-generation biofuel systems is not necessarily so.
Corn, soy, and sugarcane are fungible food commodities with large global markets, subject to large-scale supply and demand trends and market perturbations independent of biofuel production (De Kleine et al., 2017). As such, any changes in cultivation area, management intensity, or agricultural technology development associated with the scale-up of biofuel production must first be isolated from those background trends and perturbations in food and feed markets before they can be attributed to the biofuel sector. This requires detailed market analysis and modeling (Oladosu et al., 2011; Khanna et al., 2020), and the resulting estimates of biofuel performance are very heavily influenced by the conditions of a fundamentally unobservable “no-biofuel” counterfactual reference case (Babcock, 2009; Koponen et al., 2018). In addition, much of the crop mass used in first-generation biofuel production ends up in useful co-products such as corn oil, distillers grains, or soy meal, further entwining biofuel production with existing markets and introducing more dependencies around the arbitrary choice of co-product allocation method (Finnveden et al., 2009; Malça and Freire, 2010). Thus, establishing carbon additionality in such systems is more an economic and LCA attribution problem (relying on economic modeling, trade analysis, LCA allocation conventions, etc.) than an issue of carbon cycle measurement per se. As such, previous studies of carbon additionality from first-generation biofuels are necessarily coarse in their spatial and temporal scale, limited by model resolution and data availability to evaluating regional- or national-scale trends over multi-year periods. While this can shed light on the sustainability of the industry as a whole, it has limited value for the design, optimization, or verification of individual bioenergy systems.
Despite these challenges around verifying carbon additionality in first-generation biofuel systems, steady improvements in bioenergy production technology are paving the way to advanced system designs that might circumvent much of this ambiguity. Biofuel production from non-edible woody and herbaceous “cellulosic” biomass has been a major area of research since the US Renewable Fuel Standard was expanded in 2007 (Steiner and Buford, 2016; Peters, 2018). Compared to first-generation biofuels, advanced bioenergy systems have a) multiple routes to increased NEP, b) more identifiable atmosphere–ecosystem feedstock fluxes, and c) more opportunities for direct enterprise-level carbon sequestration. While these ideas are developed below in the context of bioenergy, there are many commonalities for the production of bio-plastics, mass timber, and other elements of the wider developing bioeconomy.
Multiple Routes to Increased NEP in Cellulosic Biomass Production
There are multiple potential routes to feedstock production in existing agricultural landscapes that increase NEP while avoiding wide-scale indiscriminate land use change. As per Equation (2), NEP can be increased via increasing NPP, decreasing Rh, or a combination thereof. These are consistent with the concept of “sustainable intensification,” which seeks to increase the per-area productivity of agricultural systems through increased crop growth and/or reduced waste (Tilman et al., 2011; Heaton et al., 2013; Yang et al., 2018; Mouratiadou et al., 2020).
Input intensification (e.g., greater use of fertilizers and irrigation) and adoption of higher-yielding crop varieties are conventional routes to increased agricultural NPP, though Heaton et al. (2013) review additional opportunities for bioenergy-focused sustainable intensification. They define temporal intensification as cultivating additional crops during the fallow portion of existing crop rotations, for example, growing winter oilseeds within conventional cotton-based rotations in the southeastern US (Kumar et al., 2020). Such approaches can have important co-benefits including reduced erosion, increased soil carbon, and reduced nutrient losses (Tonitto et al., 2006; Jian et al., 2020). Spatial intensification of agricultural landscapes involves converting under-utilized or unsustainably-cultivated land to dedicated energy crops. This might include “marginal” land of intermediate productivity (Gelfand et al., 2013) or agricultural land that has previously been degraded (Tilman et al., 2006), abandoned (Campbell et al., 2008), or placed into conservation easements (Gelfand et al., 2011). This could also include sub-field scale integration of perennial energy grasses in areas of problematic topography or soils, which currently produce negative economic returns under conventional crops and contribute disproportionately to erosion and nutrient leaching (Brandes et al., 2018). Perennial grasses can achieve higher NPP than many annual crops due in part to a longer growing season (Dohleman and Long, 2009). Eddy flux covariance studies have observed dedicated energy grasses such as Miscanthus and switchgrass to have approximately double the average annual NEP of conventional corn–soy rotations (Zeri et al., 2011, 2013) or continuous corn cultivation (Abraha et al., 2018). However, such NEP results are highly sensitive to prior land use and time since energy crop establishment (Abraha et al., 2018), and some studies show substantially lower NEP values for those crops (Skinner and Adler, 2010; Drewer et al., 2012), suggesting significant regional- and site-level variability.
Alternately, cellulosic feedstocks can be produced from harvesting agricultural residues such as corn stover (Domínguez-Escribá and Porcar, 2010; Heaton et al., 2013; Mouratiadou et al., 2020) that would otherwise largely be respired back to the atmosphere. Such feedstocks are additional to the extent that they increase agro-ecosystem NEP by reducing Rh (Searchinger, 2010). Stover removal is not completely additional as it does lead to some reduction in soil carbon levels (Xu et al., 2019), particularly in the short-term as demonstrated by both modeling studies (Kim et al., 2018) and carbon flux measurements (Cates and Jackson, 2019). Soil organic matter is foundational to soil health and fertility (Campbell et al., 2018), and extreme organic matter loss can compromise agricultural system function (Tiessen et al., 1994). Erosion control and soil moisture management impose further constraints on the amount of residue that can be sustainably removed in different settings (Graham et al., 2007). However, these constraints might be lessened to some degree with the co-adoption of complementary conservation practices such as tillage intensity reduction or winter cover-cropping (Kim et al., 2018; Qin et al., 2018).
Identifying Ecosystem–Atmosphere Exchange with Dedicated Bioenergy Crops
Dedicated perennial energy crops will likely be the largest source of cellulosic biomass feedstocks for a future US advanced bioeconomy (U.S. Department of Energy, 2016). Large federal research programs support the development of improved varieties of perennial energy grasses such as switchgrass, Miscanthus, and energycane, and short-rotation woody crops such as poplar (Steiner and Buford, 2016; Peters, 2018). Subsidies have also been offered to encourage their establishment in the landscapes around bioenergy facilities (Miao and Khanna, 2017). These dedicated bioenergy feedstock crops have often not previously been domesticated or improved, and lack large existing markets. As such, any future development and deployment of such crops can be confidently attributed to the bioenergy and bioproducts sectors.
The uniqueness of these dedicated crops also creates opportunities to cheaply and transparently monitor their growth and performance using remote sensing (RS) techniques. RS is widely used to map the extent of conventional crops in the US at fine spatial scales (Boryan et al., 2011), and has been applied to track expansion of corn cultivation during the growth of the ethanol industry (Wright and Wimberly, 2013; Wright et al., 2017; Lark et al., 2020). Differentiation of grassy land covers such as native grassland, managed pasture, hay production, and dedicated bioenergy grasses has historically been problematic for RS-based land use mapping (Kline et al., 2013). However, advanced methods show promise for identifying warm-season grasses (Wang et al., 2014, 2017) and even individual species such as Miscanthus (Xin and Adler, 2019) in cellulosic bioenergy production landscapes. Further refinement of such methods may enable precise, transparent, and low-cost mapping of dedicated energy crop plantings, as well as the previous land uses they replaced.
Beyond just land cover, RS is also increasingly applied to assess ecosystem carbon stocks and fluxes directly. Recent advances support using solar-induced florescence to sense GPP, lidar to measure standing biomass, and column CO2 concentration measurement and source/sink inversion modeling to estimate NEP (Xiao et al., 2019). Gu et al. (2012) have used RS techniques to produce high-resolution maps of NEP under current land cover, in order to identify low-productivity marginal lands to target for conversion to bioenergy crops. Many bioenergy critiques (Righelato and Spracklen, 2007; Haberl, 2013; Searchinger et al., 2017) focus not on the carbon value of current-day land use, but rather on the “opportunity cost” of producing bioenergy in lieu of reforestation or alternate land-based “natural climate solutions” (Griscom et al., 2017). However, RS approaches are also beginning to be used in the assessment and monitoring of NEP provided by such natural solutions (Gerlein-Safdi et al., 2020). Together, these methods may enable the direct observation of carbon additionality by tracking the carbon uptake of land before and after conversion to dedicated energy crops, and in comparison to alternative natural solutions. This would transform many nuanced sustainability questions that are currently subject of scenario analysis and model-based inference into a matter of direct observation and measurement at relatively fine spatial scales.
Carbon Additionality in Carbon-Negative Systems
Traditional bioenergy systems aim to achieve climate benefits principally through the displacement of fossil emissions. However, advanced bioenergy and other bio-based systems increasingly target the sequestration of biogenic carbon in soils, geological reservoirs, and durable bio-based products, termed “carbon management” (Canadell and Schulze, 2014), “negative emissions,” or “carbon dioxide removal.” Perennial feedstock crop cultivation promotes sequestration of soil organic carbon (Qin et al., 2016) in amounts that significantly affect system-level climate performance (Yang and Tilman, 2020). New research suggests that enhanced rock weathering can be widely deployment on croplands for additional sequestration of inorganic carbon (Beerling et al., 2020). A variety of “carbon negative” bioenergy production technologies have also been proposed including the co-production of biochar soil amendments (Lehmann, 2007) or pyrolysis liquids for geological sequestration (Schmidt et al., 2019), and point-source carbon capture and storage (CCS) applied to biomass power plants (Fuss et al., 2014; Sanchez et al., 2015) and biorefineries (Field et al., 2020; Gelfand et al., 2020; Hanssen et al., 2020).
These various negative emissions options have different implications for system-level mitigation performance. Ethanol fermentation produces a CO2 byproduct, the sequestration of which creates additional mitigation beyond the fossil fuel displacement value of the main fuel product. This can be viewed as increasing the carbon efficiency of the system, i.e., achieving greater climate benefits per unit of feedstock consumed (Field et al., 2020). Further, in a bioenergy with carbon capture and storage (BECCS) system, the same mass of carbon can both displace fossil emissions via bioenergy production, and be captured and sequestered via CCS (Figure 1C), thus effectively doing double-duty from a mitigation perspective. In the simplified examples illustrated in Figure 1C and quantified in Table 1, the addition of CCS to the near-term stover removal scenario (“bio3”) prevents CO2 from biomass combustion from being re-emitted back to the atmosphere. As a result, the system achieves net climate benefit compared to the reference case due to the avoidance of emissions from coal combustion, even in the absence of carbon additionality of the biomass feedstock. When CCS is applied to the carbon-additional long-term stover removal scenario (“bio4”), substantial mitigation is achieved via both displacing coal emissions, and from the geological sequestration of biogenic carbon (effectively creating a carbon pump from the atmosphere to the geosphere). Adding CCS introduces additional parasitic energy requirements that are not considered in this simplified example, though analysis of a hybrid fuel-and-power production system concept suggests that CCS integration can approximately double overall net system mitigation performance (Liu et al., 2011).
Similar logic is potentially applicable to other bio-based systems as well. For example, mass timber production may have mitigation value through both the displacement of emissions-intensive conventional building materials (steel, concrete, etc.) and via the sequestration of biogenic carbon in the timber itself.
Discussion
Bioenergy and bioproduct assessment has been heavily reliant on model-intensive LCA approaches subject to large and potentially irresolvable methodological uncertainties (Warner et al., 2013; DeCicco et al., 2016). However, as advanced bio-based supply chains become more distinct from conventional agricultural production, and more reliant on mitigation via the direct sequestration of biogenic carbon, new opportunities arise to directly observe feedstock-related carbon fluxes which have previously been the source of much critique and controversy. Remote sensing of additional ecosystem carbon uptake at the scale of feedstock-sheds would establish a data-rich foundation for monitoring individual bioeconomy enterprises without the need for bespoke, resource-intensive studies (Field et al., 2018).
Direct observation of atmosphere–ecosystem carbon exchange does not address all feedstock-related sustainability critiques or serve as a full replacement for conventional bio-based system LCA. Those conventional approaches are still needed to calculate supply-chain emissions associated with upstream fertilizer production and farm operations, for example. However, such emissions are typically modest in cellulosic systems. In contrast, the measurement of ecosystem–atmosphere exchanges centers the more contentious issues of land availability, system scale, and biogenic emissions accounting in ways that conventional LCA cannot. There are other system-level effects such as ILUC that exist largely outside the provenance of individual feedstock producers, bioenergy companies, or even many policy jurisdictions, and which cannot directly be observed (Babcock, 2009). But even there, RS approaches can help constrain the underlying land use change modeling with observational estimates of any existing agricultural production being displaced by feedstock crops.
Climate benefits are not a guaranteed outcome of bio-based systems, but rather the result of systems thinking and design—including innovations in technology, assessment, and policy—to maximize mitigation potential while minimizing the risk of unintended consequences. Prime and even marginal arable land are a finite resource, and arbitrarily-wide deployment of any land-based mitigation approach will at some point conflict with the food system (Fuhrman et al., 2020; Stenzel et al., 2021) and/or with biodiversity preservation (Stoy et al., 2018; Seddon et al., 2019). In light of these challenges, some recommend taking a highly precautionary approach to the development and deployment of bio-based systems (Searchinger, 2010; DeCicco and Schlesinger, 2018). However, centering the observation of atmosphere–ecosystem carbon exchanges in bio-based system assessment may provide a different path. The assessment community might take inspiration from the imperative of “ecological forecasting,” which calls for a near-term iterative approach to ecological modeling that can be continuously evaluated and updated in light of the flood of new measurements becoming available in that field (Clark et al., 2001; Dietze et al., 2018). Similarly, a greater focus on observed atmosphere–ecosystem carbon exchange in bio-based system assessment could support near-term iterative performance evaluation for individual bio-based enterprises or land-use policies, in support of sustainable decarbonization.
Data Availability Statement
The original contributions presented in the study are included in the article/supplementary material, further inquiries can be directed to the corresponding author/s.
Author Contributions
JF devised and wrote the manuscript.
Funding
JF was funded by The Center for Bioenergy Innovation, a U.S. Department of Energy Research Center supported by the Office of Biological and Environmental Research in the DOE Office of Science (grant# DE-AC05-00OR22725); The Southeast Partnership for Advanced Renewables from Carinata, funded by the U.S. Department of Agriculture National Institute of Food and Agriculture (USDA/NIFA grant# 2017-68005-26807); and USDA/NIFA grant# 2017-67019-26327.
Conflict of Interest
The author declares that the research was conducted in the absence of any commercial or financial relationships that could be construed as a potential conflict of interest.
Acknowledgments
I thank M. Francesca Cotrufo for her encouragement on this topic.
References
Abraha, M., Hamilton, S. K., Chen, J., and Robertson, G. P. (2018). Ecosystem carbon exchange on conversion of Conservation Reserve Program grasslands to annual and perennial cropping systems. Agric. For. Meteorol. 253–254, 151–160. doi: 10.1016/j.agrformet.2018.02.016
Babcock, B. (2009). Measuring unmeasurable land-use changes from biofuels. Iowa Ag Rev. 15, 4–6. Available online at: https://lib.dr.iastate.edu/iowaagreview/vol15/iss3/2
Beerling, D. J., Kantzas, E. P., Lomas, M. R., Wade, P., Eufrasio, R. M., Renforth, P., et al. (2020). Potential for large-scale CO 2 removal via enhanced rock weathering with croplands. Nature 583, 242–248. doi: 10.1038/s41586-020-2448-9
Bernier, P., and Paré, D. (2013). Using ecosystem CO2 measurements to estimate the timing and magnitude of greenhouse gas mitigation potential of forest bioenergy. GCB Bioenergy 5, 67–72. doi: 10.1111/j.1757-1707.2012.01197.x
Boryan, C., Yang, Z., Mueller, R., and Craig, M. (2011). Monitoring US agriculture: the US Department of Agriculture, National Agricultural Statistics Service, Cropland Data Layer Program. Geocarto Int. 26, 341–358. doi: 10.1080/10106049.2011.562309
Brandes, E., McNunn, G. S., Schulte, L. A., Muth, D. J., VanLoocke, A., and Heaton, E. A. (2018). Targeted subfield switchgrass integration could improve the farm economy, water quality, and bioenergy feedstock production. GCB Bioenergy 10, 199–212. doi: 10.1111/gcbb.12481
Butnar, I., Broad, O., Rodriguez, B. S., and Dodds, P. E. (2020). The role of bioenergy for global deep decarbonization: CO2 removal or low-carbon energy? GCB Bioenergy 12, 198–212. doi: 10.1111/gcbb.12666
Campbell, E. E., Field, J. L., and Paustian, K. (2018). “Modelling soil organic matter dynamics as a soil health indicator,” in Managing Soil Health for Sustainable Agriculture Burleigh Dodds Series In Agricultural Science, ed. D. Reicosky (Cambridge, UK: Burleigh Dodds Science Publishing).
Campbell, J. E., Lobell, D. B., Genova, R. C., and Field, C. B. (2008). The global potential of bioenergy on abandoned agriculture lands. Environ. Sci. Technol. 42, 5791–5794. doi: 10.1021/es800052w
Canadell, J. G., and Schulze, E. D. (2014). Global potential of biospheric carbon management for climate mitigation. Nat. Commun. 5:5282. doi: 10.1038/ncomms6282
Cates, A. M., and Jackson, R. D. (2019). Cover crop effects on net ecosystem carbon balance in grain and silage maize. Agron. J. 111, 30–38. doi: 10.2134/agronj2018.01.0045
Chapin, F. S., Woodwell, G. M., Randerson, J. T., Rastetter, E. B., Lovett, G. M., Baldocchi, D. D., et al. (2006). Reconciling carbon-cycle concepts, terminology, and methods. Ecosystems 9, 1041–1050. doi: 10.1007/s10021-005-0105-7
Clark, J. S., Carpenter, S. R., Barber, M., Collins, S., Dobson, A., Foley, J. A., et al. (2001). Ecological forecasts: an emerging imperative. Science 293, 657–660. doi: 10.1126/science.293.5530.657
Davis, S. J., Lewis, N. S., Shaner, M., Aggarwal, S., Arent, D., Azevedo, I. L., et al. (2018). Net-zero emissions energy systems. Science 360:eaas9793. doi: 10.1126/science.aas9793
De Kleine, R. D., Wallington, T. J., Anderson, J. E., and Kim, H. C. (2017). Commentary on “carbon balance effects of US biofuel production and use,” by DeCicco et al. (2016). Clim. Change 144, 111–119. doi: 10.1007/s10584-017-2032-y
DeCicco, J. M. (2013). Biofuel's carbon balance: doubts, certainties and implications. Clim. Change 121, 801–814. doi: 10.1007/s10584-013-0927-9
DeCicco, J. M., Liu, D. Y., Heo, J., Krishnan, R., Kurthen, A., and Wang, L. (2016). Carbon balance effects of U.S. biofuel production and use. Clim. Change 138, 667–680. doi: 10.1007/s10584-016-1764-4
DeCicco, J. M., and Schlesinger, W. H. (2018). Opinion: Reconsidering bioenergy given the urgency of climate protection. Proc. Natl. Acad. Sci. 115, 9642–9645. doi: 10.1073/pnas.1814120115
Dietze, M. C., Fox, A., Beck-Johnson, L. M., Betancourt, J. L., Hooten, M. B., Jarnevich, C. S., et al. (2018). Iterative near-term ecological forecasting: Needs, opportunities, and challenges. PNAS 115, 1424–1432. doi: 10.1073/pnas.1710231115
Dohleman, F. G., and Long, S. P. (2009). More productive than maize in the midwest: how does miscanthus do it? Plant Physiol. 150, 2104–2115. doi: 10.1104/pp.109.139162
Domínguez-Escribá, L., and Porcar, M. (2010). Rice straw management: the big waste. Biofuels Bioprod. Biorefin. 4, 154–159. doi: 10.1002/bbb.196
Drewer, J., Finch, J. W., Lloyd, C. R., Baggs, E. M., and Skiba, U. (2012). How do soil emissions of N2O, CH4 and CO2 from perennial bioenergy crops differ from arable annual crops? GCB Bioenergy 4, 408–419. doi: 10.1111/j.1757-1707.2011.01136.x
Fargione, J., Hill, J., Tilman, D., Polasky, S., and Hawthorne, P. (2008). Land clearing and the biofuel carbon debt. Science 319, 1235–1238. doi: 10.1126/science.1152747
Field, J. L., Evans, S. G., Marx, E., Easter, M., Adler, P. R., Dinh, T., et al. (2018). High-resolution techno–ecological modelling of a bioenergy landscape to identify climate mitigation opportunities in cellulosic ethanol production. Nat. Energy 3, 211–219. doi: 10.1038/s41560-018-0088-1
Field, J. L., Richard, T. L., Smithwick, E. A., Cai, H., Laser, M. S., LeBauer, D. S., et al. (2020). Robust paths to net greenhouse gas mitigation and negative emissions via advanced biofuels. Proc. Natl. Acad. Sci. 117, 21968–21977 doi: 10.1073/pnas.1920877117
Finnveden, G., Hauschild, M. Z., Ekvall, T., Guinée, J., Heijungs, R., Hellweg, S., et al. (2009). Recent developments in life cycle assessment. J. Environ. Manag. 91, 1–21. doi: 10.1016/j.jenvman.2009.06.018
Fuhrman, J., McJeon, H., Patel, P., Doney, S. C., Shobe, W. M., and Clarens, A. F. (2020). Food–energy–water implications of negative emissions technologies in a +1.5°C future. Nat. Clim. Change 10, 920–927. doi: 10.1038/s41558-020-0876-z
Fulton, L. M., Lynd, L. R., Körner, A., Greene, N., and Tonachel, L. R. (2015). The need for biofuels as part of a low carbon energy future. Biofuels Bioprod. Biorefin. 9, 476–483. doi: 10.1002/bbb.1559
Fuss, S., Canadell, J. G., Peters, G. P., Tavoni, M., Andrew, R. M., Ciais, P., et al. (2014). Betting on negative emissions. Nat. Clim. Change 4, 850–853. doi: 10.1038/nclimate2392
Gelfand, I., Hamilton, S. K., Kravchenko, A. N., Jackson, R. D., Thelen, K. D., and Robertson, G. P. (2020). Empirical evidence for the potential climate benefits of decarbonizing light vehicle transport in the U.S. with bioenergy from purpose-grown biomass with and without BECCS. Environ. Sci. Technol. 54, 2961–2974. doi: 10.1021/acs.est.9b07019
Gelfand, I., Sahajpal, R., Zhang, X., Izaurralde, R. C., Gross, K. L., and Robertson, G. P. (2013). Sustainable bioenergy production from marginal lands in the US Midwest. Nature 493, 514–517. doi: 10.1038/nature11811
Gelfand, I., Zenone, T., Jasrotia, P., Chen, J., Hamilton, S. K., and Robertson, G. P. (2011). Carbon debt of conservation reserve program (CRP) grasslands converted to bioenergy production. PNAS 108, 13864–13869. doi: 10.1073/pnas.1017277108
Gerlein-Safdi, C., Keppel-Aleks, G., Wang, F., Frolking, S., and Mauzerall, D. L. (2020). Satellite monitoring of natural reforestation efforts in China's drylands. One Earth 2, 98–108. doi: 10.1016/j.oneear.2019.12.015
Graham, R. L., Nelson, R., Sheehan, J., Perlack, R. D., and Wright, L. L. (2007). Current and potential U.S. corn stover supplies. Agron. J. 99, 1–11. doi: 10.2134/agronj2005.0222
Griscom, B. W., Adams, J., Ellis, P. W., Houghton, R. A., Lomax, G., Miteva, D. A., et al. (2017). Natural climate solutions. Proc. Natl. Acad. Sci. 114, 11645–11650. doi: 10.1073/pnas.1710465114
Gu, Y., Wylie, B. K., Zhang, L., and Gilmanov, T. G. (2012). Evaluation of carbon fluxes and trends (2000–2008) in the Greater Platte River Basin: a sustainability study for potential biofuel feedstock development. Biomass Bioenergy 47, 145–152. doi: 10.1016/j.biombioe.2012.09.048
Haberl, H. (2013). Net land-atmosphere flows of biogenic carbon related to bioenergy: towards an understanding of systemic feedbacks. GCB Bioenergy 5, 351–357. doi: 10.1111/gcbb.12071
Hanssen, S. V., Daioglou, V., Steinmann, Z. J. N., Doelman, J. C., Van Vuuren, D. P., Huijbregts, M., et al. (2020). The climate change mitigation potential of bioenergy with carbon capture and storage. Nat. Clim. Change 10, 1023–1029. doi: 10.1038/s41558-020-0885-y
Heaton, E. A., Schulte, L. A., Berti, M., Langeveld, H., Zegada-Lizarazu, W., Parrish, D., et al. (2013). Managing a second-generation crop portfolio through sustainable intensification: Examples from the USA and the EU. Biofpr 7, 702–714. doi: 10.1002/bbb.1429
Jian, J., Du, X., Reiter, M. S., and Stewart, R. D. (2020). A meta-analysis of global cropland soil carbon changes due to cover cropping. Soil Biol. Biochem. 143:107735. doi: 10.1016/j.soilbio.2020.107735
Khanna, M., Wang, W., and Wang, M. (2020). Assessing the additional carbon savings with biofuel. Bioenerg. Res. 13, 1082–1094. doi: 10.1007/s12155-020-10149-0
Kim, S., Zhang, X., Dale, B., Reddy, A. D., Jones, C. D., Cronin, K., et al. (2018). Corn stover cannot simultaneously meet both the volume and GHG reduction requirements of the renewable fuel standard. Biofuels Bioprod. Biorefin. 12, 203–212. doi: 10.1002/bbb.1830
Kline, K. L., Singh, N., and Dale, V. H. (2013). Cultivated hay and fallow/idle cropland confound analysis of grassland conversion in the Western Corn Belt. PNAS 110, E2863–E2863. doi: 10.1073/pnas.1306646110
Koponen, K., Soimakallio, S., Kline, K. L., Cowie, A., and Brandão, M. (2018). Quantifying the climate effects of bioenergy – choice of reference system. Renew. Sustain. Energy Rev. 81, 2271–2280. doi: 10.1016/j.rser.2017.05.292
Kumar, S., Seepaul, R., Mulvaney, M. J., Colvin, B., George, S., Marois, J. J., et al. (2020). Brassica carinata genotypes demonstrate potential as a winter biofuel crop in South East United States. Indus. Crops Prod. 150:112353. doi: 10.1016/j.indcrop.2020.112353
Lark, T. J., Spawn, S. A., Bougie, M., and Gibbs, H. K. (2020). Cropland expansion in the United States produces marginal yields at high costs to wildlife. Nat. Commun. 11:4295. doi: 10.1038/s41467-020-18045-z
Liu, G., Williams, R. H., Larson, E. D., and Kreutz, T. G. (2011). Design/economics of low-carbon power generation from natural gas and biomass with synthetic fuels co-production. Energy Proc. 4, 1989–1996. doi: 10.1016/j.egypro.2011.02.080
Lovett, G. M., Cole, J. J., and Pace, M. L. (2006). Is net ecosystem production equal to ecosystem carbon accumulation? Ecosystems 9, 152–155. doi: 10.1007/s10021-005-0036-3
Malça, J., and Freire, F. (2010). Uncertainty analysis in biofuel systems. J. Indus. Ecol. 14, 322–334. doi: 10.1111/j.1530-9290.2010.00227.x
Miao, R., and Khanna, M. (2017). Effectiveness of the biomass crop assistance program: roles of behavioral factors, credit constraint, and program design. Appl. Econ. Perspect. Policy 39, 584–608. doi: 10.1093/aepp/ppx031
Mouratiadou, I., Stella, T., Gaiser, T., Wicke, B., Nendel, C., Ewert, F., Hilst, F., and van der (2020). Sustainable intensification of crop residue exploitation for bioenergy: opportunities and challenges. GCB Bioenergy 12, 71–89. doi: 10.1111/gcbb.12649
Oladosu, G., Kline, K., Uria-Martinez, R., and Eaton, L. (2011). Sources of corn for ethanol production in the United States: a decomposition analysis of the empirical data. Biofuels Bioprod. Bioref. 5, 640–653. doi: 10.1002/bbb.305
Peters, N. K. (2018). U.S. Department of Energy Bioenergy Research Centers: 10-Year Retrospective. Breakthroughs and Impacts, 2007-2017. Washington, D.C. (United States): USDOE Office of Science (SC), Biological and Environmental Research (BER); Oak Ridge, TN (United States): Oak Ridge National Lab. (ORNL), Available online at: https://www.osti.gov/biblio/1471705 (accessed August 31, 2020).
Qin, Z., Canter, C. E., Dunn, J. B., Mueller, S., Kwon, H., Han, J., et al. (2018). Land management change greatly impacts biofuels' greenhouse gas emissions. GCB Bioenergy 10, 370–381. doi: 10.1111/gcbb.12500
Qin, Z., Dunn, J. B., Kwon, H., Mueller, S., and Wander, M. M. (2016). Soil carbon sequestration and land use change associated with biofuel production: empirical evidence. GCB Bioenergy 8, 66–80. doi: 10.1111/gcbb.12237
Righelato, R., and Spracklen, D. V. (2007). Carbon mitigation by biofuels or by saving and restoring forests? Science 317, 902–902. doi: 10.1126/science.1141361
Sanchez, D. L., Nelson, J. H., Johnston, J., Mileva, A., and Kammen, D. M. (2015). Biomass enables the transition to a carbon-negative power system across western North America. Nat. Clim. Change 5, 230–234. doi: 10.1038/nclimate2488
Schlesinger, W. H. (2018). Are wood pellets a green fuel? Science 359, 1328–1329. doi: 10.1126/science.aat2305
Schmidt, H.-P., Anca-Couce, A., Hagemann, N., Werner, C., Gerten, D., Lucht, W., et al. (2019). Pyrogenic carbon capture and storage. GCB Bioenergy 11, 573–591. doi: 10.1111/gcbb.12553
Searchinger, T. (2010). Biofuels and the need for additional carbon. Environ. Res. Lett. 5:024007. doi: 10.1088/1748-9326/5/2/024007
Searchinger, T., Edwards, R., Mulligan, D., Heimlich, R., and Plevin, R. (2015). Do biofuel policies seek to cut emissions by cutting food? Science 347, 1420–1422. doi: 10.1126/science.1261221
Searchinger, T. D., Beringer, T., and Strong, A. (2017). Does the world have low-carbon bioenergy potential from the dedicated use of land? Energy Policy 110, 434–446. doi: 10.1016/j.enpol.2017.08.016
Seddon, N., Turner, B., Berry, P., Chausson, A., and Girardin, C. A. J. (2019). Grounding nature-based climate solutions in sound biodiversity science. Nat. Clim. Change 9:84. doi: 10.1038/s41558-019-0405-0
Sharma, S., Rajan, N., Cui, S., Casey, K., Ale, S., Jessup, R., et al. (2017). Seasonal variability of evapotranspiration and carbon exchanges over a biomass sorghum field in the Southern U.S. Great Plains. Biomass Bioenergy 105, 392–401. doi: 10.1016/j.biombioe.2017.07.021
Sheehan, J., Aden, A., Paustian, K., Killian, K., Brenner, J., Walsh, M., et al. (2003). Energy and environmental aspects of using corn stover for fuel ethanol. J. Indus. Ecol. 7, 117–146. doi: 10.1162/108819803323059433
Silva, J. G. D., Serra, G. E., Moreira, J. R., Conçalves, J. C., and Goldemberg, J. (1978). Energy balance for ethyl alcohol production from crops. Science 201, 903–906. doi: 10.1126/science.201.4359.903
Skinner, R. H., and Adler, P. R. (2010). Carbon dioxide and water fluxes from switchgrass managed for bioenergy production. Agric. Ecosyst. Environ. 138, 257–264. doi: 10.1016/j.agee.2010.05.008
Steiner, J. J., and Buford, M. A. (2016). The origin of the USDA regional biomass research centers. Bioenerg. Res. 9, 379–383. doi: 10.1007/s12155-016-9736-0
Stenzel, F., Greve, P., Lucht, W., Tramberend, S., Wada, Y., and Gerten, D. (2021). Irrigation of biomass plantations may globally increase water stress more than climate change. Nat. Commun. 12:1512. doi: 10.1038/s41467-021-21640-3
Stoy, P. C., Ahmed, S., Jarchow, M., Rashford, B., Swanson, D., Albeke, S., et al. (2018). Opportunities and trade-offs among BECCS and the food, water, energy, biodiversity, and social systems nexus at regional scales. BioScience 68, 100–111. doi: 10.1093/biosci/bix145
Tanger, P., Field, J. L., Jahn, C. E., DeFoort, M. W., and Leach, J. E. (2013). Biomass for thermochemical conversion: targets and challenges. Front. Plant Sci 4:218. doi: 10.3389/fpls.2013.00218
Tiessen, H., Cuevas, E., and Chacon, P. (1994). The role of soil organic matter in sustaining soil fertility. Nature 371, 783–785. doi: 10.1038/371783a0
Tilman, D., Balzer, C., Hill, J., and Befort, B. L. (2011). Global food demand and the sustainable intensification of agriculture. Proc. Natl. Acad. Sci. 108, 20260–20264. doi: 10.1073/pnas.1116437108
Tilman, D., Hill, J., and Lehman, C. (2006). Carbon-negative biofuels from low-input high-diversity grassland biomass. Science 314, 1598–1600. doi: 10.1126/science.1133306
Tonitto, C., David, M. B., and Drinkwater, L. E. (2006). Replacing bare fallows with cover crops in fertilizer-intensive cropping systems: a meta-analysis of crop yield and N dynamics. Agric. Ecosyst. Environ. 112, 58–72. doi: 10.1016/j.agee,.2005.07.003
U.S. Department of Energy (2016). “2016 billion-ton report: advancing domestic resources for a thriving bioeconomy, volume 1: economic availability of feedstocks,” in eds. M. H. Langholtz, B. J. Stokes, and L. M. Eaton (Oak Ridge, TN: Oak Ridge National Laboratory), 448.
Vuuren, D. P., van Stehfest, E., Gernaat, D. E. H. J., Berg, M., van den Bijl, D. L., Boer, H. S., et al. (2018). Alternative pathways to the 1.5 °C target reduce the need for negative emission technologies. Nat. Clim. Change 8, 391–397. doi: 10.1038/s41558-018-0119-8
Wagle, P., Kakani, V. G., and Huhnke, R. L. (2015). Net ecosystem carbon dioxide exchange of dedicated bioenergy feedstocks: switchgrass and high biomass sorghum. Agric. For. Meteorol. 207, 107–116. doi: 10.1016/j.agrformet.2015.03.015
Wang, C., Fan, Q., Li, Q., SooHoo, W. M., and Lu, L. (2017). Energy crop mapping with enhanced TM/MODIS time series in the BCAP agricultural lands. ISPRS J. Photogram. Remote Sens. 124, 133–143. doi: 10.1016/j.isprsjprs.2016.12.002
Wang, C., Zhong, C., and Yang, Z. (2014). Assessing bioenergy-driven agricultural land use change and biomass quantities in the U.S. Midwest with MODIS time series. JARS 8:085198. doi: 10.1117/1.JRS.8.085198
Wang, M. (1996). GREET - Transportation Fuel Cycles Model: Methodology and Use. Argonne National Laboratory: US Department of Energy.
Warner, E., Zhang, Y., Inman, D., and Heath, G. (2013). Challenges in the estimation of greenhouse gas emissions from biofuel-induced global land-use change. Biofuels Bioprod. Bioref. 8, 114–125. doi: 10.1002/bbb.1434
Wright, C. K., Larson, B., Lark, T. J., and Gibbs, H. K. (2017). Recent grassland losses are concentrated around U.S. ethanol refineries. Environ. Res. Lett. 12:044001. doi: 10.1088/1748-9326/aa6446
Wright, C. K., and Wimberly, M. C. (2013). Recent land use change in the Western Corn Belt threatens grasslands and wetlands. PNAS 110, 4134–4139. doi: 10.1073/pnas.1215404110
Xiao, J., Chevallier, F., Gomez, C., Guanter, L., Hicke, J. A., Huete, A. R., et al. (2019). Remote sensing of the terrestrial carbon cycle: a review of advances over 50 years. Remote Sens. Environ. 233:111383. doi: 10.1016/j.rse.2019.111383
Xin, Y., and Adler, P. R. (2019). “Mapping miscanthus using multi-temporal convolutional neural network and google earth engine,” in Proceedings of the 3rd ACM SIGSPATIAL International Workshop on AI for Geographic Knowledge Discovery GeoAI 2019 (New York, NY, USA: Association for Computing Machinery), 81–84.
Xu, H., Sieverding, H., Kwon, H., Clay, D., Stewart, C., Johnson, J. M. F., et al. (2019). A global meta-analysis of soil organic carbon response to corn stover removal. GCB Bioenergy 11, 1215–1233. doi: 10.1111/gcbb.12631
Yang, Y., and Tilman, D. (2020). Soil and root carbon storage is key to climate benefits of bioenergy crops. Biofuel Res. J. 7, 1143–1148. doi: 10.18331/BRJ2020.7.2.2
Yang, Y., Tilman, D., Lehman, C., and Trost, J. J. (2018). Sustainable intensification of high-diversity biomass production for optimal biofuel benefits. Nat. Sustain. 1, 686–692. doi: 10.1038/s41893-018-0166-1
Zenone, T., Gelfand, I., Chen, J., Hamilton, S. K., and Robertson, G. P. (2013). From set-aside grassland to annual and perennial cellulosic biofuel crops: effects of land use change on carbon balance. Agric. For. Meteorol. 182–183, 1–12. doi: 10.1016/j.agrformet.2013.07.015
Zeri, M., Anderson-Teixeira, K., Hickman, G., Masters, M., DeLucia, E., and Bernacchi, C. J. (2011). Carbon exchange by establishing biofuel crops in Central Illinois. Agric. Ecosyst. Environ. 144, 319–329. doi: 10.1016/j.agee.2011.09.006
Keywords: biogenic carbon, biofuels, additional carbon, life-cycle assessment, bioproducts, BECCS, mitigation, negative emissions
Citation: Field JL (2021) Revisiting “Additional Carbon”: Tracking Atmosphere–Ecosystem Carbon Exchange to Establish Mitigation and Negative Emissions From Bio-Based Systems. Front. Clim. 3:603239. doi: 10.3389/fclim.2021.603239
Received: 05 September 2020; Accepted: 16 March 2021;
Published: 13 April 2021.
Edited by:
Miguel Brandão, Royal Institute of Technology, SwedenReviewed by:
Rafael Mattos Dos Santos, University of Guelph, CanadaEmanuele Lugato, Joint Research Centre (JRC), Italy
Copyright © 2021 Field. This is an open-access article distributed under the terms of the Creative Commons Attribution License (CC BY). The use, distribution or reproduction in other forums is permitted, provided the original author(s) and the copyright owner(s) are credited and that the original publication in this journal is cited, in accordance with accepted academic practice. No use, distribution or reproduction is permitted which does not comply with these terms.
*Correspondence: John L. Field, ZmllbGRKTEBvcm5sLmdvdg==