- 1Robert Wood Johnson Medical School, Rutgers University, New Brunswick, NJ, United States
- 2Director of Clinical Division, Neuroscape Associate Professor, Neurology and Psychiatry, Weill Institute for Neurosciences & Kavli Institute for Fundamental Neuroscience, University of California, San Francisco, CA, United States
- 3Interdepartmental Neuroscience Program, Yale University, New Haven, CT, United States
- 4JelikaLite Corp., New York, NY, United States
Background: Small pilot studies have indicated that transcranial photobiomodulation (tPBM) may help alleviate symptoms of neurological conditions like depression, traumatic brain injury and Autism Spectrum Disorder (ASD).
Objective: To examine the effect of tPBM on the behavioral symptoms of ASD and brain electrophysiology in children aged 2–7.
Methods: We conducted an open label, one-arm study with 23 participants, aged 2–7, previously diagnosed with ASD. We delivered non-invasively to all participants pulses of near-infrared light (wavelength 850 nm, pulse 40 Hz) to cortical nodes of Default Mode Network, Broca and Wernicke areas, and occipital lobe of the brain, twice weekly for 10 weeks. The tPBM was delivered using an investigational medical device designed for this purpose. Changes in ASD symptoms were measured using pre- and post-intervention scores on the Childhood Autism Rating Scale (CARS-2, 2nd Edition). We collected electroencephalogram (EEG) data after each treatment session from all children who tolerated wearing the EEG cap to monitor changes in brain activity.
Results: The intervention resulted in a significant 7-point reduction in average CARS-2 scores (t = 10.23, p < .0001), along with decreased delta power and increased gamma and beta power in EEG readings. The increase in gamma power was statistically significant [t(14) = 2.30, p = 0.047]. Changes in EEG power were significantly correlated with the number of sessions (delta: r(192) = −0.18, p = .013; gamma: r(192) = .19, p = .007; beta: r(192) = .15, p = .04). Improvements in CARS-2 scores were negatively correlated with changes in delta and beta power (delta: r(15) = −.59, p = .020; beta: r(15) = −.54, p = .037). No moderate or severe side effects were reported.
Conclusion: This study supports the potential of tPBM as a safe and effective treatment for ASD, and it suggests that EEG measurements may serve as a useful biomarker for future research.
Trial Registration: https://clinicaltrials.gov/ct2/show/NCT04660552
Introduction
Autism Spectrum Disorder (ASD) is a neurodevelopmental condition, usually identified within the first two years of a child's life. It impacts a person's social and communication abilities and is marked by repetitive behaviors and language challenges. ASD affects all racial and ethnic groups and significantly influences the quality of life of affected children and their caregivers, with symptom severity and type varying greatly among affected individuals. Parents of children with ASD often face increased parenting stress, mental and physical health issues, and financial strain compared to parents of children without ASD (1). ASD poses a substantial financial burden on affected families, with recent studies estimating the average per capita lifetime cost of ASD to be over $3.5 million in the United States (2). ASD is a huge societal financial burden that continues to increase with the growing prevalence of ASD in the population. The Center for Disease Control (CDC) estimated that in 2020 the prevalence of ASD in children was 1 in 36, a nearly 90% increase from the 1 in 68 prevalence reported in 2010—with a yearly burden to the US economy expected to exceed $460 billion by 2025 (3, 4).
A full understanding of the pathogenesis of ASD remains elusive (5). However, it has been established that ASD can be hereditary in some instances, and having a close family member like a cousin or sibling with ASD or another mental health disorder increases the risk (3, 4). Numerous studies suggest that environmental contaminants, such as pesticides, heavy metals, and air pollutants, might contribute to ASD development (6, 7). Additionally, maternal diet and substance use during pregnancy are identified as risk factors (8).
Several theories have been proposed to explain the etiology of ASD, including mitochondrial dysfunction (5), neuroinflammation (9–11), overproduction of reactive oxygen species (ROS), abnormal immune responses, and impaired cellular metabolism (12). Mitochondrial dysfunction is present in as many as 80% of children diagnosed with ASD and it is correlated with a variety of ASD symptoms, such as developmental and cognitive disabilities, language impairment, gastrointestinal dysfunction, and fatigue (13, 14).
Neuroinflammation, specifically activation of microglia cells for an extended period, leads to the sustained production of inflammatory mediators for longer than usual. This long-term increase in inflammatory mediators contributes to loss of synaptic connections and neuronal cell death (9–11), processes that are associated with long range neuronal underconnectivity, a phenomenon reported in many autism studies (15–18). Relatedly, there is a demonstrated correlation between mitochondrial dysfunction and neuroinflammation, in which molecules derived from damaged mitochondria activate inflammatory pathways (19).
The prevalence in ASD of both under- and over-connectivity of the default mode network (DMN) might be related to mitochondrial dysfunction and neuroinflammation (20). Several studies have correlated DMN dysfunction with conditions such as dementia, schizophrenia, and autism (21). Specifically, functional MRI studies have identified aberrant DMN connectivity in both children and adults with ASD (22).
Transcranial photobiomodulation (tPBM) therapy, a non-invasive and painless approach that leverages visible red light (600–700 nm) and/or invisible near-infrared light (NIR, 800–1,200 nm) to treat neurological disorders. tPBM has been shown to effectively restore or enhance mitochondrial function (23–25), reduce inflammation and oxidative damage (26), and potentially modulate default mode network (DMN) connectivity (27, 28). tPBM is being trialed as a therapeutic intervention for ASD with encouraging results. Ceranoglu (29) demonstrated significant reduction of symptoms in adults with high-functioning ASD (Social Responsiveness Scale, 2nd Edition) after 8 weeks of tPBM stimulation (pulsed, 850 nm) of the forebrain. Pallanti (30) reported a small study in which tPBM successfully reduced symptoms of ASD. Fradkin et al. (31) demonstrated significant reduction of symptoms of ASD in 2- to 6-year-old autistic children (CARS-2, 2nd Edition or “CARS-2”) after 8 weeks of tPBM stimulation with the investigational medical device (40 hz, 850 nm) of targeted brain regions, which included some cortical nodes of the DMN as well as some areas in pre-frontal cortex and the temporal lobe.
In this study, we administered the tPBM treatment using the investigational medical device and assessed the generalizability of tPBM as a therapeutic intervention for children aged 2–7 with ASD. We used CARS-2 to score the severity of ASD symptoms pre- post-intervention and collected EEG data of known neural signatures after each tPBM session using a high-resolution EEG device, specifically designed for young children (Ant-Neuro Eego Sports 32). We focused on clinical results, without seeking validation of the mechanism of action of PBM therapy in ASD.
Materials and methods
Study design
This was an 8-week open-label exploratory study designed to validate the safety and efficacy of the tPBM device designed for pediatric autistic patients.
The study protocol was approved by WCG institutional review board (IRB, 1280247) and is registered at ClinicalTrials.gov (Identifier: NCT04660552).
Participants
Two- to seven-year-olds, inclusive of both genders and all ethnicities, with a previous diagnosis of ASD and CARS-2 scores above 28, were eligible to participate in the study. The diagnosis had to be given by a licensed professional (e.g., a developmental pediatrician) in accordance with the Diagnostic and Statistical Manual of Mental Disorders, fifth edition (32). These evaluations typically involved a battery of tests, including CARS-2, Autism Diagnostic Observation Schedule (ADOS), Temperament and Atypical Behavior Scale (TABS), and Autism Diagnostic Interview-Revised (ADI-R).
Detailed inclusion and exclusion criteria are presented in Table 1.
Recruitment was conducted through Applied Behavioral Analysis agencies and specialized schools, specific to the ASD population.
Informed consent was obtained for each treatment session.
Setting
Treatments were applied and clinical data collected in an IRB approved location.
Investigational product and treatment procedure
The device under investigation was: a wireless, light-weight device specifically designed for young children with ASD to remain mobile (see Figure 1). The device is Bluetooth controlled and provides transcranial delivery of pulsed NIR light (40 Hz, 850 nm), via 6 LEDs (150 mW maximum optical power, each) to targeted brain areas (which included amongst others, cortical nodes of DMN, Broca and Wernicke areas, occipital lobe and prefrontal cortex).
Following a two-week titration protocol (described below), each participant underwent treatment twice a week for 8 weeks. Each treatment session was followed by 15 min of EEG data collection from participants that tolerated wearing the EEG cap.
Titration protocol: Each participant started with a 2 min tPBM treatment. Participants who tolerated the treatment well received incrementally larger doses (2 min) in each of 5 subsequent sessions, for a maximum dose of 12 min.
Measures
Demographics
Age, gender, race, and ethnicity were collected by staff before intervention.
Primary outcome: CARS-2: childhood autism rating scale
To evaluate the effect of tPBM on ASD symptoms, pre- and post-intervention assessments of CARS-2 were conducted on all participants by the last author, a licensed psychologist experienced in the use of CARS-2 in clinical practice. To minimize the potential bias present in open-label studies, the evaluator did not see the pre-treatment CARS-2 scores during the post-treatment CARS-2 evaluations.
CARS-2 is a validated clinical rating scale designed for use by trained clinicians to assess ASD based on direct observation of the child (33). It comprises 15 items, each corresponding to core domains affected by ASD. Total scores on the scale range from 15 to 60. Interpretations of scores are categorized as follows: scores below 30 indicate the non-autistic range; scores from 30 to 36.5 indicate mild to moderate autism; scores from 37 to 60 indicate severe autism.
To evaluate the impact of tPBM on brain electrophysiology, trained staff members proficient in operating the EEG devices conducted EEG data collection sessions lasting 15 min after each tPBM session. EEG data was collected utilizing the eego™ sports 32, a dry-electrode, 32-channel EEG device specifically engineered for use in young children (ANT Neuro GmbH, Germany). Data collection was limited to children comfortable with wearing the EEG cap.
EEG is used for brain electrophysiology, providing valuable insights into the neural dynamics associated with ASD, aiding diagnostic information, and informing early intervention strategies (34–37).
Analysis
Primary outcome: CARS-2
Pre- and post-intervention CARS-2 scores were analyzed and compared using a paired samples t-test. Changes in mean CARS-2 scores were considered significant at p < 0.05.
Secondary outcome: EEG
EEG data was preprocessed using the EEGLAB software (38). Noisy channels identified upon initial visual inspection were removed from the data and interpolated using a spherical spline interpolation, using the average signal of the surrounding channels to reconstruct the data in the removed channel. The data were detrended, and a low-pass filter at 50 Hz was applied to remove high-frequency noise. Ocular correction was performed by removing segments of data containing eye blinks and lateral eye movements. The data were then re-referenced to the average signal of all channels. Event-related spectral perturbation (ERSP) power in delta (1–4 Hz), theta (4–7 Hz), alpha (8–12 Hz), beta (15–30 Hz), and gamma (30–50 Hz) frequency bands were resolved using a fast Fourier transform (FFT).
Here we interrogated the recorded ERSP power by: (1) using a correlation-based analysis involving scaled time as in (31) using a traditional pre-post intervention analysis. For the first approach, we evaluated global power by collapsing the ERSP power signal across all electrodes. To assess changes in spectral power over scaled time, Pearson correlations were performed to test the relationship between spectral power in each band collected at each treatment session for each participant. Given that participants had different number of treatment sessions, to evaluate change in this signal, only participants who had at least 3 treatment sessions were included in the analysis. For the second approach, we directly investigated spectral power pre- and post-treatment using each individual's first and final treatment sessions by paired t-test analysis. Given the small sample size of this study, each test functioned following a bootstrapping procedure where 1,000 samples were taken to achieve 95% confidence interval threshold. Results were significant at p < 0.05.
Results
Study participants (sample characteristics)
Thirty-one children aged 2–7 years were screened to ensure they met all inclusion and none of the exclusion criteria. Most participants received their diagnosis during the 24 months evaluation period provided through the New York State Department of Health Early Intervention program.
Twenty-five children were initially enrolled. Three dropped out due to travel limitations, leaving twenty-two participants who completed the study.
The mean age of the participants was 4.9 years old, with a standard deviation (SD) of 1.46 years. Four (4) were 7 years old. Nineteen were males. 86.36% of the participants were White, 4.55% were Black, 4.55% were Central Asian, and 4.55% were South Asians.
Baseline demographics data are presented in Table 2.
Clinical results
Treatment procedure and dosing
All twenty-two participants that completed the study tolerated the treatment well and received up-to 12 min treatments twice a week for 8 weeks. Four participants experienced headaches. Seventeen experienced hyperactivity at least once during the study, according to their parents. Fifteen participants tolerated at least three 15 min EEG recording sessions.
Primary outcome: CARS-2
After the intervention, a significant reduction in CARS-2 scores was observed, with a mean decrease of 7 points (t = 10.23, p < .0001). The pre-intervention CARS-2 scores had a mean of 36.5 (SD = 5.6, n = 22), while post-intervention scores averaged 29.7 (SD = 5.5).
Detailed CARS-2 scores for each participant, along with the overall mean (M) and standard deviation (SD), are presented in Table 3. Participant's pre- post-intervention score changes by CARS-2 sub-scale are tabulated in Table 4.
Although the very small sub-group sample sizes make conclusions speculative, a post-hoc analysis of the data revealed no significant differences in the reduction of mean CARS-2 scores between participants aged 2–6, participants aged 7.
The observed 7-point change in mean CARS-2 scores before and after the intervention aligns with the results from the (31), where the difference in mean CARS-2 score change between the two groups was 7.23 (95% CI: 2.357–12.107, p = 0.011).
The CARS-2 scores result for the (31) study are reproduced in Table 5.
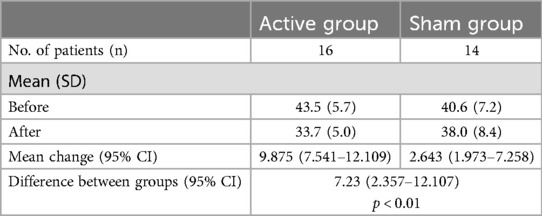
Table 5. CARS-2 scores, mean (SD) (31),.
Secondary outcome: EEG
In our initial ERSP analysis, we found a notable negative correlation between the number of treatment sessions and delta ERSP power (r(192) = −0.18, p = 0.013; see Figure 2A). This suggests that delta power decreased as the number of treatment sessions increased. Additionally, we observed significant positive correlations between treatment sessions and both gamma and beta power (gamma: r(192) = 0.19, p = 0.007, see Figure 2B; beta: r(192) = 0.15, p = 0.04, see Figure 2C), indicating that these neural signals strengthened over time. No significant correlations were found in the other frequency bands (theta: r(192) = 0.03, p = 0.72; alpha: r(192) = 0.07, p = 0.34).
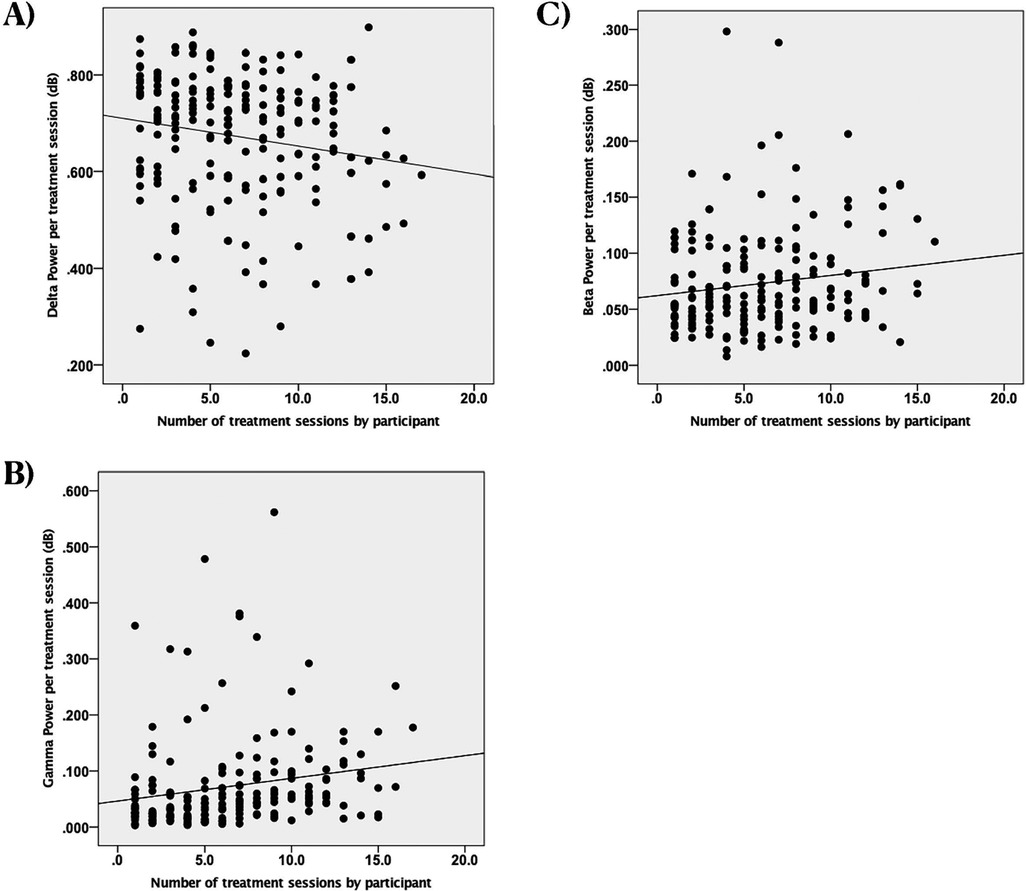
Figure 2. Scatterplots of spectral power in different frequency bands vs. the number of treatment sessions with EEG recordings by participant, dB, decibels (unitless measure of power amplitude). (A) Change in delta power. Each dot represents an individual observation. The line is the regression line. X axis: scaled time. Y axis: power of delta. (B) Change in gamma power. Each dot represents an individual observation. The line is the regression line. X axis: scaled time. Y axis: power of gamma. (C) Change in beta power. Each dot represents an individual observation. The line is the regression line. X axis: scaled time. Y axis: power of beta.
We also identified significant negative correlations between improvements in CARS-2 scores (a measure of symptom severity) and changes in both delta power (r(15) = −0.59, p = 0.020; see Figure 3A) and beta power (r(15) = −0.54, p = 0.037; see Figure 3B). However, no significant associations were found between CARS-2 scores and changes in gamma or theta power (gamma: r(15) = −0.43, p = 0.11; theta: r(15) = 0.11, p = 0.70).
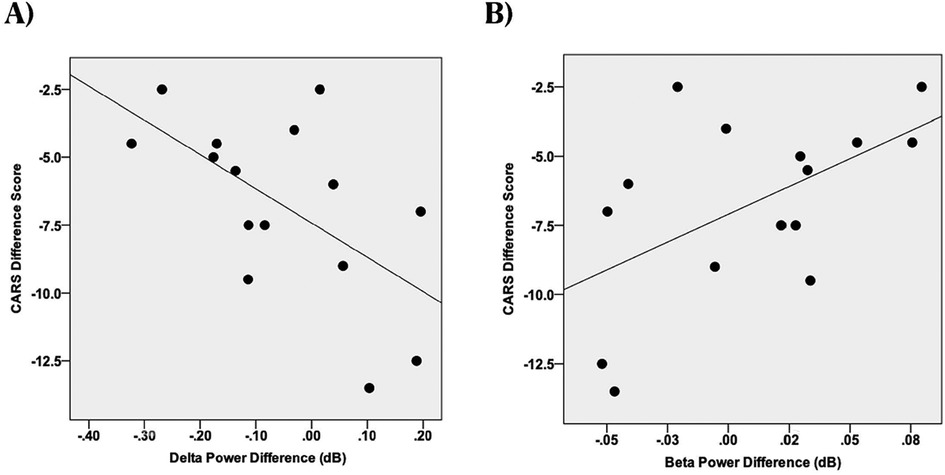
Figure 3. Scatterplots of change in spectral power (last session minus first session) in different frequency bands vs. the Childhood Autism Rating Scales (CARS-2), dB, decibels (unitless measure of power amplitude). (A) CARS-2, EEG delta power correlation. (B) CARS-2, EEG beta power correlation.
Our second ERSP analysis, which directly compared ERSP power between the first and final treatment sessions, showed a significant pre- post-treatment increase in gamma power (t(14) = 2.30, p = 0.047). There were no significant differences in any of the other frequency bands.
Discussion
The present open-label one arm study investigated the effect of tPBM (delivered by the investigational medical device) on the symptoms of ASD and brain electrophysiology.
We evaluated changes in ASD symptoms using the CARS-2, comparing scores before and after the tPBM intervention. To assess brain activity, we analyzed EEG data collected after each tPBM session using Ant-Neuro EEG cap. After the eight-week tPBM treatment, we observed a significant reduction in ASD symptoms and notable changes in brain activity. These results closely mirrored those of the (31), which were achieved using the same investigational medical device.
The observed 7-point improvement in mean CARS-2 scores suggests clinically significant changes as (39) established in their seminal work, that the change of 4.5 points is clinically significant. Specific CARS-2 sub-scale changes (Table 4) indicate meaningful progress in several core areas affected by autism, such as communication, social interaction, and sociability. However, assessing a clinically significant difference in ASD symptoms using CARS-2 is challenging. Many notable improvements, such as holding hands while crossing the street or using the bathroom independently, were not captured by CARS-2 due to its lack of sensitivity to these changes, despite the significant enhancement they brought to family quality of life. Future research should incorporate additional scales, such as the Aberrant Behavior Checklist (40) and the Social Responsiveness Scale (41).
The study replicated the decline in delta power and its correlation with improved CARS-2 scores previously reported in findings from (31). We replicated the significant reduction in delta power over time which correlated with post-treatment decline in CARS-2. The current study also found significant correlations between EEG gamma and beta power with the duration of the intervention, as well as changes in gamma power before and after treatment. Higher power of Gamma waves is associated with improved memory and cognition (42–44).
The observed changes in brain activity were associated with improvements in ASD symptoms and shifts toward more typical brainwave patterns.
Although speculative, the observed changes in delta brainwave power may indicate reduction of neuroinflammation, which is known to affect ASD symptoms (13, 14, 45). Frohlich et al. (45) argued that delta waves in wakeful states are often associated with various neurological conditions including TBI, chronic hemorrhage, microglial activation, and inflammation. Furthermore, the presence of delta waves in the wakeful state was associated with the locations of future seizures (46). If this is the case, these findings align with prior research on non-pharmacological treatments that have shown similar brain activity changes correlate with improved performance on untrained tasks (18, 47, 48). On a related note, research by (46) suggests that increased delta power during wakefulness may signal a heightened risk for seizures, while Weiss (49) found a link between delta waves and epileptiform activity in adults. These findings imply that tPBM could be a promising treatment option for individuals with ASD who also experience seizures, highlighting the need for more research.
These findings should be interpreted with caution. Studies comparing EEG patterns in children with ASD to neurotypical children have produced mixed results (50–52), and we only observed changes in gamma power after the intervention. Despite this, the results suggest that EEG pattern changes could be a valuable tool for assessing treatment effectiveness, personalizing therapy, and guiding future research.
Limitations
Several limitations should be acknowledged when interpreting these findings. First, the sample's heterogeneity, limited sample size, lack of a direct control group, and relatively wide age range could confound the results with natural developmental changes. Second, some children were unable to tolerate the EEG cap after each tPBM session. Third, although the CARS-2 is an FDA-required outcome measure for ASD trials, it may not optimally capture the full range of symptom improvements, especially those that substantially impact family life but are not well-reflected in the scale. Additionally, the study did not include follow-up assessments (CARS-2 or EEG) months later to determine the persistence or transience of the treatment effects.
Given these limitations, the results should be interpreted with caution. They should be considered only within the specific population and conditions under which the study was conducted. Broad generalizations or extrapolations beyond this context may lead to inaccurate conclusions or misinterpretations.
Conclusions
This open-label study adds significant support to using tPBM as a safe and effective intervention for reducing the core symptoms of ASD. Nevertheless, larger-scale studies with more rigorous designs are necessary to validate and extend these results. Future research should specifically examine the impact of light-dosing variables—such as pulsing frequency—on treatment outcomes; assess long-term efficacy and potential side effects; investigate drug interactions; and analyze changes in specific symptoms by evaluating each CARS-2 subscale individually. Additionally, studies should evaluate the effects on both the child's and parents' quality of life. It is also important for future research to consider factors like gender, functional impairment, verbal status, and concomitant therapies, as well as the high comorbidity of ASD with other neurological and psychiatric disorders—including attention deficit hyperactivity disorder (ADHD), obsessive-compulsive disorder (OCD), anxiety, and cognitive disabilities. Moreover, given that a significant percentage of children diagnosed with ASD develop seizures later in life, further studies employing EEG are warranted.
Data availability statement
The raw data supporting the conclusions of this article will be made available by the authors, without undue reservation.
Ethics statement
The studies involving humans were approved by WCG North America Institutional Review Board. 212 Carnegie Center, Suite 301, Princeton, NJ 08540, USA. The studies were conducted in accordance with the local legislation and institutional requirements. Written informed consent for participation in this study was provided by the participants' legal guardians/next of kin. Written informed consent was obtained from the minor(s)' legal guardian/next of kin for the publication of any potentially identifiable images or data included in this article.
Author contributions
YF: Investigation, Writing – review & editing. JA: Data curation, Formal Analysis, Writing – review & editing. AS: Data curation, Formal Analysis, Writing – review & editing. LD: Data curation, Formal Analysis, Writing – original draft, Writing – review & editing. ES: Conceptualization, Investigation, Methodology, Supervision, Writing – original draft, Writing – review & editing.
Funding
The author(s) declare financial support was received for the research, authorship, and/or publication of this article. This study received funding from The National Science Foundation, SBIR grant #2136474, partially funded the study and was not involved in the study design, collection, analysis, interpretation of data, the writing of this article, or the decision to submit it for publication.
Acknowledgments
We are thankful to Michael Hamblin for his conceptual contribution to the design of the investigational medical device. We are thankful to Robert Naviaux for his comments on the early versions of this manuscript and for his conceptual guidance. We are thankful to our research assistants: Liza Logounova and Maya Rozenblat and Sara Segal for their assistance with data collection and in running the study. We are thankful to Liza Logounova and Katya Sverdlov for coordinating the study.
Conflict of interest
The authors declare that JelikaLite had the following involvement with the study: JelikaLite developed the investigational treatment device used in this study. ES is a co-Founder of JelikaLite. ES was involved in study design, CARS-2 scores collections and analysis, manuscript writing and the decision to submit to Frontiers in Neurology. LT is a JelikaLite stock option holder. LT was involved in manuscript writing and decision to submit to Frontiers in Neurology. JA and AS were paid consultants on this project.
The remaining authors declare that the research was conducted in the absence of any commercial or financial relationships that could be construed as a potential conflict of interest.
Publisher's note
All claims expressed in this article are solely those of the authors and do not necessarily represent those of their affiliated organizations, or those of the publisher, the editors and the reviewers. Any product that may be evaluated in this article, or claim that may be made by its manufacturer, is not guaranteed or endorsed by the publisher.
References
1. Karst JS, Van Hecke AV. Parent and family impact of autism spectrum disorders: a review and proposed model for intervention evaluation. Clin Child Fam Psychol Rev. (2012) 15:247–77. doi: 10.1007/s10567-012-0119-6
2. Cakir J, Frye RE, Walker SJ. The lifetime social cost of autism: 1990–2029. Res Autism Spectr Disord. (2020) 72:101502. doi: 10.1016/j.rasd.2019.101502
3. Hansen SN, Schendel DE, Francis RW, Windham GC, Bresnahan M, Levine SZ, et al. Recurrence risk of autism in siblings and cousins: a multinational, population-based study. J Am Acad Child Adolesc Psychiatry. (2019) 58(9):866–75. doi: 10.1016/j.jaac.2018.11.017
4. Sandin S, Lichtenstein P, Kuja-Halkola R, Larsson H, Hultman CM, Reichenberg A. The familial risk of autism. JAMA. (2014) 311(17):1770–7. doi: 10.1001/jama.2014.4144
5. Frye RE. Mitochondrial dysfunction in autism spectrum disorder: unique abnormalities and targeted treatments. Semin Pediatr Neurol. (2020) 35:100829. doi: 10.1016/j.spen.2020.100829
6. Rossignol DA, Genuis SJ, Frye RE. Environmental toxicants and autism spectrum disorders: a systematic review. Transl Psychiatry. (2014) 4(2):e360. doi: 10.1038/tp.2014.4
7. Awadh SM, Yaseen ZM, Al-Suwaiyan MS. The role of environmental trace element toxicants on autism: a medical biogeochemistry perspective. Ecotoxicol Environ Saf. (2023) 251:114561. doi: 10.1016/j.ecoenv.2023.114561
8. Bastaki KN, Alwan S, Zahir FR. Maternal prenatal exposures in pregnancy and autism spectrum disorder: an insight into the epigenetics of drugs and diet as key environmental influences. Adv Neurobiol. (2020) 24:143–62. doi: 10.1007/978-3-030-30402-7_5
9. Rodriguez JI, Kern JK. Evidence of microglial activation in autism and its possible role in brain underconnectivity. Neuron Glia Biol. (2011) 7(2–4):205–13. doi: 10.1017/S1740925X12000142
10. Takano T. Role of microglia in autism: recent advances. Dev Neurosci. (2015) 37(3):195–202. doi: 10.1159/000398791
11. Kim JW, Hong JY, Bae SM. Microglia and autism spectrum disorder: overview of current evidence and novel immunomodulatory treatment options. Clin Psychopharmacol Neurosci. (2018) 16(3):246–52. doi: 10.9758/cpn.2018.16.3.246
12. Farhana A, Khan YS. Mitochondrial dysfunction: a key player in the pathogenesis of autism Spectrum disorders and Alzheimer’s disease. In Autism Spectrum Disorder and Alzheimer’s Disease: Advances in Research. Singapore: Springer Nature Singapore. (2022). p. 21–42.
13. Giulivi C, Zhang YF, Omanska-Klusek A, Ross-Inta C, Wong S, Hertz-Picciotto I, et al. Mitochondrial dysfunction in autism. JAMA. (2010) 304(21):2389–96. doi: 10.1001/jama.2010.1706
14. Frye RE, Rossignol DA. Mitochondrial dysfunction can connect the diverse medical symptoms associated with autism spectrum disorders. Pediatr Res. (2011) 69(5 Part 2):41R–7R. doi: 10.1203/PDR.0b013e318212f16b
15. Just MA, Keller TA, Malave VL, Kana RK, Varma S. Autism as a neural systems disorder: a theory of frontal-posterior underconnectivity. Neurosci Biobehav Rev. (2012) 36(4):1292–313. doi: 10.1016/j.neubiorev.2012.02.007
16. Long Z, Duan X, Mantini D, Chen H. Alteration of functional connectivity in autism spectrum disorder: effect of age and anatomical distance. Sci Rep. (2016) 6(1):1–8. doi: 10.1038/srep26527
17. O’Reilly C, Lewis JD, Elsabbagh M. Is functional brain connectivity atypical in autism? A systematic review of EEG and MEG studies. PLoS One. (2017) 12(5):e0175870. doi: 10.1371/journal.pone.0175870.
18. Benkarim O, Paquola C, Park BY, Hong SJ, Royer J, Vos de Wael R, et al. Connectivity alterations in autism reflect functional idiosyncrasy. Commun Biol. (2021) 4(1):1–15. doi: 10.1038/s42003-021-02572-6
19. Lin M, Liu N, Qin Z, Wang Y. Mitochondrial-derived damage-associated molecular patterns amplify neuroinflammation in neurodegenerative diseases. Acta Pharmacol Sin. (2022) 43(10):2439–47. doi: 10.1038/s41401-022-00879-6
20. Nair A, Jolliffe M, Lograsso YSS, Bearden CE. A review of default mode network connectivity and its association with social cognition in adolescents with autism spectrum disorder and early-onset psychosis. Front Psychiatry. (2020) 11:614. doi: 10.3389/fpsyt.2020.00614
21. Broyd SJ, Demanuele C, Debener S, Helps SK, James CJ, Sonuga-Barke EJ. Default-mode brain dysfunction in mental disorders: a systematic review. Neurosci Biobehav Rev. (2009) 33(3):279–96. doi: 10.1016/j.neubiorev.2008.09.002
22. Padmanabhan A, Lynch CJ, Schaer M, Menon V. The default mode network in autism. Biol Psychiatry Cogn Neurosci Neuroimaging. (2017) 2(6):476–86. doi: 10.1016/j.bpsc.2017.04.004
23. Hamblin MR. Shining light on the head: photobiomodulation for brain disorders. BBA Clin. (2016) 6:113–24. doi: 10.1016/j.bbacli.2016.09.002
24. Chung H, Dai T, Sharma SK, Huang YY, Carroll JD, Hamblin MR. The nuts and bolts of low-level laser (light) therapy. Ann Biomed Eng. (2012) 40(2):516–33. doi: 10.1007/s10439-011-0454-7
25. Huang YY, Sharma SK, Carroll J, Hamblin MR. Biphasic dose response in low level light therapy. Dose Response. (2011) 9(4):602–18. doi: 10.2203/dose-response.11-009.Hamblin
26. Hamblin MR. Mechanisms and applications of the anti-inflammatory effects of photobiomodulation. AIMS Biophys. (2017) 4(3):337–61. doi: 10.3934/biophy.2017.3.337
27. Zomorrodi R, Loheswaran G, Pushparaj A, Lim L. Pulsed near infrared transcranial and intranasal photobiomodulation significantly modulates neural oscillations: a pilot exploratory study. Sci Rep. (2019) 9(1):1–11. doi: 10.1038/s41598-019-42693-x
28. Cassano P, Petrie SR, Hamblin MR, Henderson TA, Iosifescu DV, Lavretsky H. Review of transcranial photobiomodulation for major depressive disorder: targeting brain metabolism, inflammation, oxidative stress, and neurogenesis. Photomed Laser Surg. (2016) 34(10):610–26. doi: 10.1117/1.NPh.3.3.031404
29. Ceranoglu TA. Effects of transcranial photobiomodulation on adults with autism spectrum disorder: a pilot study. J Autism Dev Disord. (2020) 50(4):1182–97. doi: 10.1007/s10803-019-04348-8
30. Pallanti S. Transcranial photobiomodulation in autism spectrum disorders: a review. J Psychiatr Res. (2016) 80:47–54. doi: 10.3390/children9050755
31. Fradkin Y. A randomized sham-controlled clinical trial evaluating the safety and efficacy of transcranial photobiomodulation to improve symptoms of autism spectrum disorder and brain electrophysiology in children aged 2–6 years. Front Neurol. (2024) 15:1221193. doi: 10.3389/fneur.2024.1221193
32. American Psychiatric Association. Diagnostic and Statistical Manual of Mental Disorders: DSM-5. 5th ed. Washington, DC: American Psychiatric Association (2013).
33. Vaughan CA. Test review: E. Schopler, M. E. Van bourgondien, G. J. Wellman, & S. R. Love childhood autism rating scale. Los Angeles, CA: western psychological services, 2010. J Psychoeduc Assess. (2011) 29(5):489–93. doi: 10.1177/0734282911400873
34. Gurau O, Bosl WJ, Newton CR. How useful is electroencephalography in the diagnosis of autism spectrum disorders and the delineation of subtypes: a systematic review. Front Psychiatry. (2017) 8:230005. doi: 10.3389/fpsyt.2017.00121
35. Boutros NN, Lajiness-O’Neill R, Zillgitt A, Richard AE, Bowyer SM. EEG changes associated with autistic spectrum disorders. Neuropsychiatr Electrophysiol. (2015) 1(1):1–20. doi: 10.1186/s40810-014-0001-5
36. Bo J, Acluche F, Lasutschinkow PC, Augustiniak A, Ditchfield N, Lajiness-O’Neill R. Motor networks in children with autism spectrum disorder: a systematic review on EEG studies. Exp Brain Res. (2022) 240(12):3073–87. doi: 10.1007/s00221-022-06483-8
37. Alhassan S, Soudani A, Almusallam M. Energy-efficient EEG-based scheme for autism spectrum disorder detection using wearable sensors. Sensors. (2023) 23(4):2228. doi: 10.3390/s23042228
38. Delorme A, Makeig S. EEGLAB: an open source toolbox for analysis of single-trial EEG dynamics including independent component analysis. J Neurosci Methods. (2004) 134(1):9–21. doi: 10.1016/j.jneumeth.2003.10.009
39. Jurek L, Baltazar M, Gulati S, Novakovic N, Núñez M, Oakley J, et al. Correction to: response (minimum clinically relevant change) in ASD symptoms after an intervention according to CARS-2: consensus from an expert elicitation procedure. Eur Child Adolesc Psychiatry. (2023) 32:367. doi: 10.1007/s00787-021-01864-w
40. Aman MG, Singh NN. Aberrant Behavior Checklist Manual. East Aurora, NY: Slosson Publications (1986).
41. Constantino JN, Gruber CP. Social Responsiveness Scale, Second Edition (SRS-2). Torrance, CA: Western Psychological Services (2012).
42. Miller EK, Lundqvist M, Bastos AM. Working memory 2.0. Neuron. (2018) 100:463–75. doi: 10.1016/j.neuron.2018.09.023
43. Griffiths BJ, Parish G, Roux F, Michelmann S, van der Plas M, Kolibius LD, et al. Directional coupling of slow and fast hippocampal gamma with neocortical alpha/beta oscillations in human episodic memory. Proc Natl Acad Sci U S A. (2019) 116:21834–42. doi: 10.1073/pnas.1914180116
44. Christoffersen GRJ, Schachtman TR. Electrophysiological CNS-processes related to associative learning in humans,. Behav Brain Res. (2016) 296:211–32. doi: 10.1016/j.bbr.2015.09.011
45. Frohlich J, Toker D, Monti MM. Consciousness among delta waves: a paradox? Brain. (2021) 144(8):2257–77. doi: 10.1093/brain/awab095
46. Sachdev RN, Gaspard N, Gerrard JL, Hirsch LJ, Spencer DD, Zaveri HP. Delta rhythm in wakefulness: evidence from intracranial recordings in human beings. J Neurophysiol. (2015) 114(2):1248–54. doi: 10.1152/jn.00249.2015
47. Anguera JA, Volponi JJ, Simon AJ, Gallen CL, Rolle CE, Anguera-Singla R, et al. Integrated cognitive and physical fitness training enhances attention abilities in older adults. NPJ Aging. (2022) 8(1):12. doi: 10.1038/s41514-022-00093-y
48. Eells JT, Henry MM, Summerfelt P, Wong-Riley MTT, Buchmann EV, Kane M, et al. Therapeutic photobiomodulation for methanol-induced retinal toxicity. Proc Natl Acad Sci USA. (2003) 100(6):3439–44. doi: 10.1073/pnas.0534746100
49. Weiss SA, Sheybani L, Seenarine N, Fried I, Wu C, Sharan A, et al. Delta oscillation coupled propagating fast ripples precede epileptiform discharges in patients with focal epilepsy. Neurobiol Dis. (2022) 175:105928. doi: 10.1016/j.nbd.2022.105928
50. Wang J, Barstein J, Ethridge LE, Mosconi MW, Takarae Y, Sweeney JA. Resting state EEG abnormalities in autism spectrum disorders. J Neurodev Disord. (2013) 5:1–14. doi: 10.1186/1866-1955-5-24
51. Tierney AL, Gabard-Durnam L, Vogel-Farley V, Tager-Flusberg H, Nelson CA. Developmental trajectories of resting EEG power: an endophenotype of autism spectrum disorder. PLoS One. (2012) 7(6):e39127. doi: 10.1371/journal.pone.0039127
Keywords: ASD, autism spectrum disorder, photobiomodulation, transcranial photobiomodulation, EEG, delta power, pediatric neurology
Citation: Fradkin Y, Anguera JA, Simon AJ, De Taboada L and Steingold E (2025) Transcranial photobiomodulation for reducing symptoms of autism spectrum disorder and modulating brain electrophysiology in children aged 2–7: an open label study. Front. Child Adolesc. Psychiatry 4:1477839. doi: 10.3389/frcha.2025.1477839
Received: 8 August 2024; Accepted: 3 January 2025;
Published: 29 January 2025.
Edited by:
Noemi Mazzoni, University of Bologna, ItalyReviewed by:
John Mitrofanis, Université Grenoble Alpes, FranceTolga Ceranoglu, Massachusetts General Hospital and Harvard Medical School, United States
Copyright: © 2025 Fradkin, Anguera, Simon, De Taboada and Steingold. This is an open-access article distributed under the terms of the Creative Commons Attribution License (CC BY). The use, distribution or reproduction in other forums is permitted, provided the original author(s) and the copyright owner(s) are credited and that the original publication in this journal is cited, in accordance with accepted academic practice. No use, distribution or reproduction is permitted which does not comply with these terms.
*Correspondence: Yuli Fradkin, eWYxNTVAcndqbXMucnV0Z2Vycy5lZHU=