- 1Pacific Northwest National Laboratory, Richland, WA, United States
- 2University of Alabama, Department of Chemical & Biological Engineering, Tuscaloosa, AL, United States
- 3Washington State University, Department of Chemical Engineering and Bioengineering, Pullman, WA, United States
Cryogenic time-of-flight secondary ion mass spectrometry (cryo ToF-SIMS) has emerged as a powerful tool for investigating molecular interactions, speciation, and dynamics in materials for CO2 capture. In this study, we apply cryo ToF-SIMS to probe interactions between CO2, water, and PEEK-ionene membranes—a promising material for direct CO2 capture due to its selectivity, durability, and efficiency. Despite this potential, the mechanisms governing CO2 diffusion and the influence of water vapor on CO2 behavior remain unclear. To address this, we loaded PEEK-ionene membranes with 13CO2 and D2O and employed cryo ToF-SIMS to visualize the 3D distribution of CO2 and water within the membrane. While prior studies suggest that 13CO2 is absorbed under ambient conditions, our cryo ToF-SIMS analysis revealed no enhancement of the 13C/12C ratio, suggesting weak CO2-membrane interactions. As a result, CO2 vaporizes even at low temperatures (−140°C) under vacuum conditions. In contrast, D2O displayed a relatively homogeneous distribution in the membrane, suggesting stronger water-membrane interactions via hydrogen bonding (18–20 kJ/mol). Interestingly, CO2 was not detected in D2O-loaded membranes, indicating minimal interference from water vapor on CO2 diffusion. As a comparison, the cryo ToF-SIMS data show that 13CO2 can readily react with a basic Na2CO3 aqueous solution to form NaH13CO3. These findings demonstrate cryo ToF-SIMS as a critical technique for understanding gas-water-membrane interactions, offering insights for membrane functionalization to improve CO2 capture efficiency.
1 Introduction
Climate change and global warming have led to an urgent need for technologies capable of capturing and storing CO2 from industrial processes and the atmosphere. Among the various approaches developed to address this challenge, membrane-based separation has emerged as a promising solution due to its potential for high selectivity, efficiency, and adaptability to various operational environments (Kammakakam et al., 2022; Jansen and Drioli, 2009).
Poly (ether ether ketone) (PEEK) is a semicrystalline thermoplastic known for its exceptional thermal stability, mechanical properties, and chemical resistance, making it a prime candidate for demanding applications. While pristine PEEK exhibits low gas permeability and is not considered a viable polymer for gas separation membranes, development of PEEK-ionene membranes offers opportunities to increase permeability while maintaining a robust polymer structure. PEEK-ionene materials integrate cationic groups directly within their backbones, combining the robustness of PEEK with the selective permeability offered by ionenes. The incorporation of ionic liquids (ILs) further enhances the membranes’ CO2 selectivity, making them particularly interesting for gas separation applications (Kammakakam et al., 2022; O’Harra et al., 2022).
Understanding the interactions among CO2, PEEK-ionene membranes, and water is essential for improving membrane-based CO2 capture technologies. However, it has long been challenging to track small molecules, such as CO2 and H2O, in organic matrixes at micron or submicron scales. For example, Nuclear Magnetic Resonance (NMR) is a powerful tool which has been extensively used in CO2 capture studies for quite a few years (Heldebrant et al., 2008; Heldebrant et al., 2017; Leclaire et al., 2024). It can not only quantitatively monitor CO2 in bulk samples, but also identify reactants and products to elucidate reaction mechanisms during the CO2 capture process based on chemical shift (Leclaire et al., 2024). However, the spatial resolution of NMR imaging is a limitation, and NMR 3D imaging at micron to submicron scales has been less reported. Transmission electron microscopy (TEM) and scanning electron microscope (SEM) are popularly used imaging tools, and they can provide atomic-level spatial resolution (Winey et al., 2014; Franken et al., 2020; Kourkoutis et al., 2012). However, electron beam damage of organic species and lack of molecular recognition capability make them hardly used to image small molecules in an organic matrix.
To address these challenges, cryo-secondary ion mass spectrometry (cryo-SIMS) is emerging as an effective tool to study the speciation, movement, and exchange of small molecules like CO2 and H2O within materials. A combination of isotopic labeling and cryo-SIMS provides a unique method to track small molecules in organic matrixes at nanoscale (Hoppe et al., 2013; Gao et al., 2016). SIMS provides 3D nanoscale imaging of elemental, isotopic and molecular species with excellent sensitivity (Williams, 1985; Benninghoven, 1994; Lombardo et al., 2023). The depth resolution of SIMS depth profiling can go down to a few nanometer (nm) in organic matrices and such a capability allows quantitative determination of polymer self-diffusion with a combination of isotopic labeling (Zheng et al., 1997; Zheng et al., 1995). At the same time, SIMS can provide sub-micron lateral resolution isotopic imaging, and such a capability has been used to resolve important scientific questions (Dekas et al., 2009; Pareek et al., 2020; Pett-Ridge and Weber, 2022; Li et al., 2023). More interestingly, ToF (time-of-flight)-SIMS can detect molecular ion and characteristic fragment ions, providing direct molecular evidence to elucidate reaction mechanisms. For example, in situ 3D ToF-SIMS imaging of isotopic labeled amino acids in single cells can directly visualize the de novo purine biosynthesis process, confirming a long-existing hypothesis that metabolons should be biosynthetic “hotspots” (Pareek et al., 2020).
SIMS operates under high vacuum conditions, making sample preparation crucial, particularly for samples with volatile components (Benninghoven, 1994; Gao et al., 2016). For example, most fresh biological samples contain a high-water content, which poses a challenge for SIMS analysis under ambient conditions. The high vapor pressure of water can interfere with the vacuum environment required for reliable SIMS measurements, leading to signal instability and reduced sensitivity. It is well-known that the vapor pressure is temperature-dependent, and low temperature can greatly reduce vapor pressure. Therefore, cryo-sample preparation has been extensively used in SIMS imaging of biological samples. Studies have shown that cooling biological samples to −110°C can reduce the vapor pressure of water to the 10–8 mbar range, enabling standard SIMS analysis (Guo et al., 2020; Cannon et al., 2000).
It should be noted that vapor pressure is closely related to intermolecular interactions. Strong interactions, such metallic bonds and ionic bonds (in metal and metal oxide samples), lead to very low vapor pressure, and such samples can be directly analyzed using SIMS. However, weak intermolecular interactions, such as London dispersion forces, facilitate easier vaporization resulting in high vapor pressure, especially for small gas molecules (e.g., CO2, O2, N2, etc.). Although water molecules are also small, they are unique due to the presence of hydrogen bonds, which have an energy of approximately 18–20 kJ/mol. As a result, water exhibits a relatively low vapor pressure compared to CO2 and O2. Therefore, temperature during SIMS analysis can be used as an indicator of the strength of intermolecular interactions. For example, water can be retained on the sample surface at −110°C during SIMS analysis. If CO2 cannot be retained under the same conditions, it suggests that the CO2-sample (polymer + IL) interaction is weaker than hydrogen bonds in water.
In this work, ToF-SIMS 3D imaging was used to investigate PEEK-ionene membranes purged with 13CO2 and heavy water deuterium oxide (D2O). By using isotopically labeled 13CO2 and D2O, we track the interactions of 13CO2 and D2O within the membrane, offering insights into gas sorption and diffusion mechanisms. Additionally, by exploring the effect of water on CO2 capture, the study seeks to address potential challenges posed by water vapor in gas separation processes.
2 Materials and methods
2.1 Materials and preparation
The lecture bottle containing 13CO2 (99 atom% 13C) was purchased from Cambridge Isotope Laboratories, Inc. Sodium bicarbonate (NaHCO3) was purchased from Sigma-Aldrich (Catalog Number S5761). Sodium carbonate (Na2CO3) was obtained from Sigma-Aldrich (Catalog Number 451614). Deuterium oxide (D2O) was acquired from Sigma-Aldrich (Catalog Number 151882, 99.9 atom% D).
In this study, it is crucial to cool the PEEK-ionene sample after loading 13CO2. To achieve this, a specially designed sample block was developed to facilitate the pre-cooling before SIMS analysis (Figure 1a). The sample block has an effective area of 8 mm × 16 mm. A 0.1 mm thick molybdenum (Mo) plate, designed with a central hole of 5.0 mm in diameter for sample exposure, is positioned on top of the block. The plate is securely fastened to the copper (Cu) block using two small screw holes, pressing the sample firmly onto the Cu block. This ensures effective thermal contact, allowing efficient cooling to the sample when the sample block is mounted on the heating/cooling sample stage of the SIMS instrument (Supplementary Figure S1). The PEEK-ionene sample was cut into 0.5 cm × 1 cm piece and clamped onto the sample block below the Mo plate (Figure 1a). The sample block was then inserted into a gas loading unit and sealed before purging with 300 psig 13CO2 (Figure 1b). After purging, the entire gas loading unit was immersed in a liquid nitrogen (N2)-filled cryogenic container (Figure 1c) for fast freezing. Once the boiling and bubbling of liquid N2 subsided, the membraned-loaded sample block was taken out from the gas loading unit inside the liquid N2, covered with a small Cu piece (to avoid any ice condensation during transfer, as shown in Supplementary Figure S1), and then quickly transferred to the pre-cooled cooling/heating sample stage (−140 to −150°C) inside the ToF-SIMS loadlock (Figure 1d). The loadlock pumping process can be started immediately. After the loadlock was pumped down to 1 × 10−6 mbar and maintained at the desired temperature (−140 to −150°C), the cooling/heating stage was transferred to the pre-cooled main analysis chamber for ToF-SIMS analysis (Figures 1e, f). The sample temperature during ToF-SIMS analysis was approximately −140°C. It is important to note that the anti-ice condensation Cu piece must be removed by rotating the heating/cooling sample stage, thereby exposing the sample surface before transferring the sample stage into the analysis chamber.
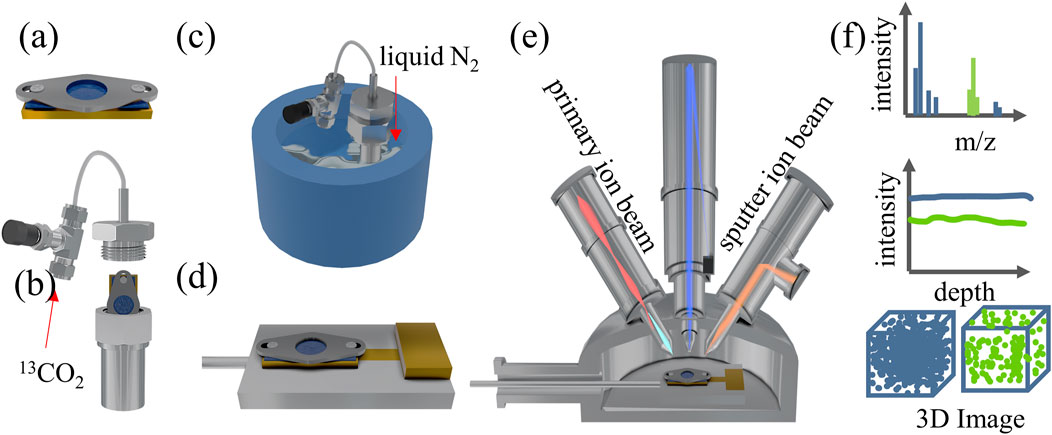
Figure 1. Schematic of cryo ToF-SIMS workflow including the (a) sample mounting, (b) gas loading, (c) flash freezing, (d) mounting the sample on a pre-cooled heating/cooling sample holder, (e) cryo ToF-SIMS analysis, and (f) the results of SIMS spectra, depth profiles, 3D images.
0.1M Na2CO3 and 0.1M NaHCO3 were prepared separately using Milli-Q deionized water (18.2 MΩ.cm at 25°C). 2 mL Na2CO3 was added into a gas loading apparatus and purged with 300 psig 13CO2. The solution was left to equilibrate for 2 days. 20 μL of 13CO2 – reacted Na2CO3 was taken from the gas loading apparatus and dispensed onto a cleaned silicon (Si) chip which was mounted on the customized sample holder (Figure 1a). The silicon chip was quickly placed into the liquid N2-filled cryogenic container for flash freezing. Additionally, 20 µL of 0.1M NaHCO3 and 20 µL of 0.1M Na2CO3 were separately deposited onto two different Si chips directly before flashing freezing. The remaining steps of the procedure follow those illustrated in Figures 1d–f.
The imidazolium-functionalized PEEK–ionene with bistriflimide ([Tf2N]) anions were synthesized and the method was reported previously (Ravula et al., 2024; O’Harra et al., 2022). In this study, the membrane p ([K (EEK)2 (2mIm)4(C6)3][Tf2N]4 was added with stoichiometric equivalent amount of “free” [C4mIm][Tf2N], i.e., 1-butyl-3-methylimidazolium bistriflimide ionic liquid (IL).
2.2 Cryo ToF-SIMS analysis
In this study, isotope imaging and depth profiling were conducted using a ToF-SIMS 5 mass spectrometer manufactured by IONTOF GmbH, Münster, Germany. To eliminate any thin ice layers or other potential surface contaminants, an Ar cluster ion beam (10 keV, 4.0 nA) was employed to pre-sputter a selected sample surface area of 1,000 × 1,000 μm2 for 20 s.
The depth profiling measurements were carried out in the negative ion mode for most of the samples, and both negative and positive ion mode for selected samples. The charge compensation was done with a low energy electron flood gun. Depth profiling of the samples was performed in the non-interlaced mode (analysis 3.2 s, sputter 5.0 s, pulse 1.0 s). For PEEK-ionene membrane samples, a 2.0 keV Cs+ beam was used as the sputtering beam, which was scanned on a 500 μm2 × 500 μm2 area. Cs+ sputter beam is chosen because it enhances the negative ionization (Houssiau and Mine, 2010), and ions like C− and H− are of significant interest in this study. The instrument was equipped with a 25 keV Binq+ source (n = 1, 3, and 5, q = 1 and 2). In this study, we used Bi32+ beam, which results in a total acceleration voltage of 50 keV. The Bi32+ beam was scanned on a 100 μm2 × 100 μm2 area at the center of the Cs+ sputter area. The Bi32+ beam scanning was performed in random mode with 100 µs cycle time, 128 × 128 pixels, one shot/frame/pixel and two frames per step. The obtained mass resolution m/Δm was above 7,000 at peak m/z C2H− for all measurements. Internal mass calibration was performed using H−, C−, and C2− mass signals in negative ion mode.
After depth profiling, the sputter crater was measured by a Veeco Dektak 6M stylus profilometer. The obtained depth was used to determine the erosion rate (nm/s), which was applied to all measurements of the membrane samples for calibrating the depth profiles, assuming a constant erosion rate. Data analysis of the ToF-SIMS measurements was done using Surface Lab Software version 7.1 (IONTOF GmbH, Münster, Germany). For the Na2CO3, NaHCO3 and 13CO2-loaded Na2CO3 aqueous solution samples, the Ar cluster beam was used as a sputter ion beam, because molecular signals were desired.
In addition, a Keyence 3D Surface Profiler (VK-X3000) was applied to obtain the morphological variation of IL-loaded PEEK-ionene membrane before and after 13CO2 loading.
3 Results and discussion
Since C− signal is much more sensitive than C+ in SIMS analysis, the negative ion mode was prioritized for analysis and comparison. Figure 2 shows the negative ion spectra of a pristine PEEK-ionene membrane, as well as the spectra obtained after loading with 13CO2, D2O separately, and a combination of 13CO2 + D2O. Interestingly, no 13C enrichment was observed after loading with 13CO2 or 13CO2 + D2O. In fact, no significant difference was observed between the whole spectra of the pristine and the 13CO2 -loaded membranes in a m/z range from 1–800 (Supplementary Figure S2). We repeated measurement multiple times, and the results are consistent: no enrichment of 13C was observed. These results suggest that very little 13CO2 remains in the sample during the cryo ToF-SIMS analysis. In contrast, a clear increase in D− and OD− was observed in the spectra of the D2O-loaded and 13CO2 + D2O-loaded samples (Figure 2), suggesting that D2O remains in the sample during the cryo ToF-SIMS analysis. These results indicate that the CO2-PEEK-ionene interactions are relatively weak, at least weaker than H2O-H2O interaction in ice (18–20 kJ/mol (Wendler et al., 2010)). This is supported by previous reports showing that ice can be kept under ToF-SIMS analysis at around −110°C (Guo et al., 2020; Cannon et al., 2000), and D2O-related signals can be clearly seen during our testing. The choice to use D2O instead of H2O was primarily to eliminate potential interference from water condensation during the rapid freezing process. Since H2O is ubiquitous in the environment, using it could introduce uncertainty in our analysis due to unintended condensation. Additionally, the PEEK-ionene membrane itself contains hydrogen, which would further complicate species differentiation. Using D2O allows for clear identification of water-related signals without interference, ensuring more accurate assessment of water-membrane interactions.
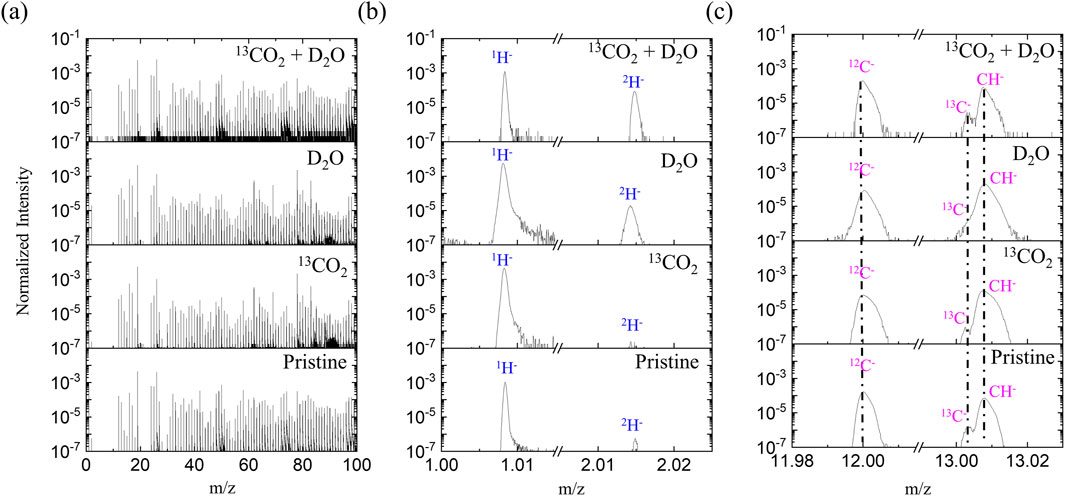
Figure 2. Negative ion ToF-SIMS spectra of a pristine PEEK-ionene sample, after 13CO2 loading, after D2O loading and after 13CO2 + D2O loading, with (a) m/z 0–100, (b) showing H- and 2H-, and (c) showing 12C-, 13C, and 12CH-.
It should be noted that NMR results indeed show that 13CO2 can go into the PEEK-ionene membrane, and the evidence is 13C NMR frequency shift if compared to pure 13CO2 gas (Walter et al., 2023; Chen et al., 2025). However, the 13C NMR frequency shift of 13CO2 molecules is small, only about 0.5–4 ppm, indicating some weak interactions between CO2 and PEEK-ionene, qualitatively consistent with our cryo ToF-SIMS result. It is important to mention that a chemical interaction can lead to a large 13C NMR frequency shift. For example, N-(2-ethoxyethyl)-3-morpholinopropan-1-amine (EEMPA) can react with CO2 to form chemically bound carbamate, where about 35 ppm 13C NMR frequency shift can be observed (from about 125 ppm (13CO2 gas) to about 160 ppm (carbamate)) (Walter et al., 2023; Chen et al., 2025).
Based on the above results, it is evident that CO2 molecules do not engage in any chemical interactions with the PEEK-ionene membrane, only exhibiting van der Waals forces between them. These interactions appear to induce a structural response in the membrane, as the initially flat PEEK-ionene membrane became notably brittle after CO2 loading. Figure 3 shows the representative surface optical images of PEEK-ionene membranes with and without 13CO2 loading, obtained using a Keyence 3D Surface Profiler. Figures 3a, c show the surface of the 13CO2-loaded membrane, characterized by more pronounced porous features, likely resulting from CO2 diffusion into the membrane. Figures 3b, d depict the pristine membrane, displaying a relatively smoother structure with fewer porous characteristics. The change in membrane morphology is expected, as some level of affinity between CO2 and the PEEK-ionene membrane is essential to facilitate CO2 diffusion into the membrane. Additionally, these interactions must remain weak, as stronger interactions would reduce the mobility of CO2 molecules, hindering their diffusion and passage through the PEEK-ionene membrane.
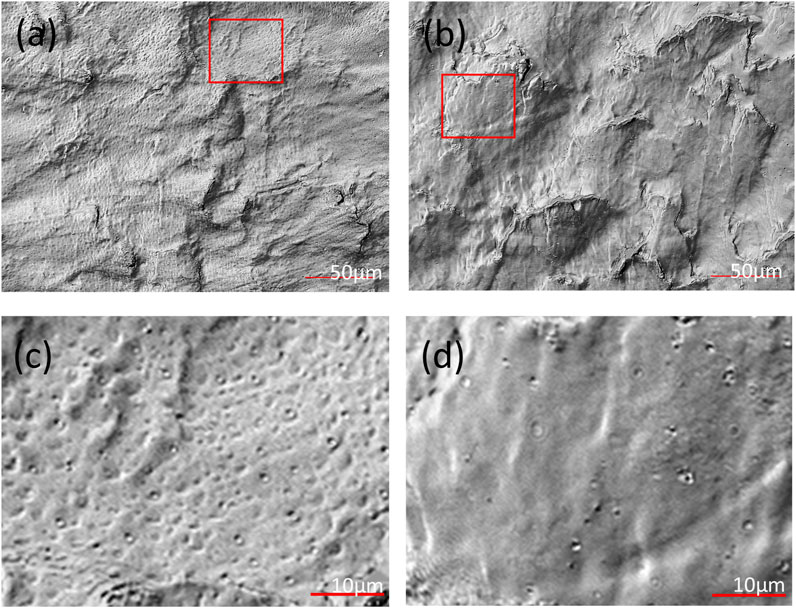
Figure 3. Optical images of (a, c) the 13CO2-loaded PEEK-ionene membrane and (b, d) the pristine membrane at different magnifications, with panels (c, d) showing zoomed-in views of the regions highlighted by the red boxes in (a, b), respectively, showing the porous structure observed in the PEEK- ionene membrane after CO2, loading.
The increase of the D− signal is expected in the D2O-loaded membrane, as D2O has similar properties with H2O, which can be immobilized as ice under high vacuum at −110°C. Since the sample temperature during our cryo ToF-SIMS analysis was approximately −140°C, the D2O remained in its ice form throughout the entire analysis. A comparison of the spectra of the pristine PEEK-ionene sample and the D2O-loaded sample shows that few new signals were observed except for D−, OD−, and (D2O)nOD− signals, indicating that water molecules did not react with any components in the PEEK-ionene membrane or IL, in alignment with our expectation (Supplementary Figure S3).
It should be noted that the D2O-related signals, such as D− and OD−, are relatively weak in the D2O-loaded sample, while they are much stronger in the 13CO2 + D2O-loaded sample. A possible explanation is that the presence of CO2 may promote the diffusion of D2O into the PEEK-ionene membrane, as CO2 can react with H2O to form H2CO3. This explanation is supported by an NMR observation that 13CO2-D2O interaction can be seen in PEEK-ionene membrane (Chen et al., 2025). However, the existence of D2O does not appear to facilitate the trapping of 13CO2 in the PEEK-ionene membrane, as no 13C enrichment was observed in the 13CO2 + D2O loaded membrane sample. This is understandable since H2CO3 is an unstable compound and readily dissociates to release CO2. These results suggest that the CO2-H2O interactions are weaker than the H2O-H2O interactions. Therefore, it is reasonable to expect that the presence of water vapor would have a limited effect on CO2 diffusion in the PEEK-ionene membrane, even with IL loading.
Figure 4 shows 3D images of the D2O related peaks (D−, OD−) and the peaks from PEEK-ionene (C6H− and m/z C2F6NO4S2−), obtained from the D2O+13CO2 loaded membrane. More molecular fragments and peak assignments of this type of PEEK-ionene have been published previously using static ToF-SIMS (Strange et al., 2024b; Strange et al., 2024a). The 3D images of D− and OD− show that D2O distribution is relatively even, indicating that water molecules can diffuse into the PEEK-ionene membrane. In addition, there are some D− enrichment locations, suggesting localized water accumulation, possibly in regions such as pores observed in Figure 3. This observation is interesting, because our previous NMR and computer simulation results do suggest some structure changes after CO2 loading (Walter et al., 2023; Chen et al., 2025). The 3D images show a stable distribution of D2O in the membrane up to a depth of 0.5 µm. Practically, ToF-SIMS molecular imaging analysis of an organic sample can provide a lateral resolution of about 0.2–0.3 µm and a depth resolution better than 5 nm if the imaging analysis mode is applied. In this study, we optimized for high mass resolution rather than imaging mode. Although the spatial resolutions cannot compete with TEM and SEM, ToF-SIMS imaging can provide a unique field-of-view to examine uniformity of the PEEK-ionene membrane. 3D images of the peaks D−, OD−, C6H− and C2F6NO4S2− observed from the pristine PEEK-ionene membrane and D2O loaded membrane are presented in Supplementary Figure S4 for comparison.
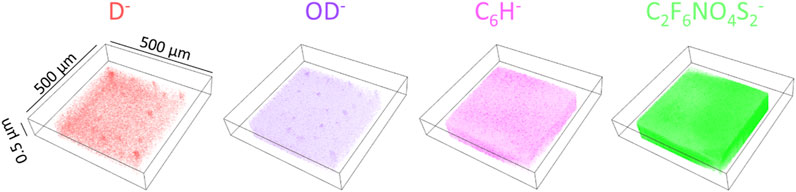
Figure 4. 3D images of the characteristic secondary ions on a 13CO2 + D2O loaded PEEK-ionene membrane.
To test if chemically bonded 13CO2 molecules can be observed during cryo ToF-SIMS testing, we introduced 13CO2 into a 0.1 M Na2CO3 aqueous solution for cryo ToF-SIMS analysis. A pristine 0.1 M Na2CO3 and a 0.1 M NaHCO3 aqueous solution samples (both at natural isotopic abundance) were also tested as references. Figure 5 shows the negative ion spectra of these three samples. A significant increase of 13C/12C ratio was observed in the spectrum of the 13CO2-loaded Na2CO3 sample, indicating a chemical reaction effectively captures CO2 in an aqueous system. The chemical reaction formula is represented in Equation 1.
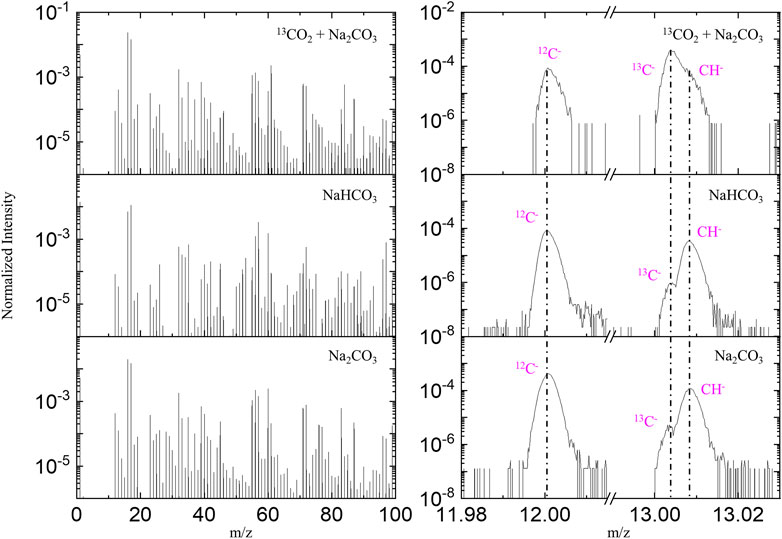
Figure 5. Negative ion TOF-SIMS spectra of a 0.1 M Na2CO3, a 13CO2 loaded 0.1 M Na2CO3, and a 0.1 M NaHCO3 aqueous solution, with left panel showing the spectral comparison of m/z 1–100, and right panel highlighting 12C-, 13C-, and 12CH-.
The NMR frequency shift of 13C in HCO3− is about 160–161 ppm (about 169–170 ppm in CO32−) (Pironti et al., 2017; Moore et al., 2015), much larger than the NMR frequency shift of 13C in 13CO2 gas (about 125–128 ppm) (Sin et al., 2019; Walter et al., 2023; Chen et al., 2025), indicating that a chemical interaction (normally, hundreds of kJ/mol) results in significant NMR frequency shift (30–40 ppm) compared to van der Waals interactions (normally a few to tens of kJ/mol).
ToF-SIMS analysis offers two distinct advantages: molecular recognition and isotopic identification capabilities. Negative ion ToF-SIMS spectra from a 0.1 M NaHCO3, a 0.1 M Na2CO3 and a 13CO2-loaded 0.1 M Na2CO3 aqueous solutions are shown in Supplementary Figure S5. Water clusters (H2O)nOH− (such as m/z 35, 53, 71, 89, 107) and NanOHn+1–clusters (such as m/z 57, 97) are clearly observed, indicating that an ice matrix was analyzed. Supplementary Figure S5 shows that after 13CO2 loading, a number of 12COx− related signals decreased while corresponding 13COx signals increased. For example, CO3− (m/z 60) signal is strong and HCO3− (m/z 61) is weak in the spectra of the NaHCO3 and Na2CO3 samples. As a comparison, CO3− (m/z 60) signal is weak but 13CO3− (m/z 61) is strong in the spectrum of 13CO2-loaded Na2CO3 sample. Similarly, Na2CO3OH− (m/z 123), Na3(CO3)2− (m/z 189) and (Na2CO3)2OH− (m/z 229) are strong in the spectra of the NaHCO3 and Na2CO3 samples, while the intensities of Na213CO3OH− (m/z 124), Na3(13CO3)2− (m/z 191), and (Na213CO3)2OH− (m/z 231) are prominent in the spectrum of 13CO2-loaded Na2CO3 sample.
This isotopic analysis reveals that the 13C/12C ratio in the Na2CO3 solution increases from a natural abundance of approximately 1.1% to 267.2% after loading with 13CO2 (Table 1). Although a 1:1 13C/12C ratio might be expected from the reaction Equation 1, the data show a more complete exchange of 12C by 13C. This observation can be explained by the reversible nature of the reaction, allowing the excess 13CO2 to continuously replace the original CO32− in the solution until the 13C/12C ratio equilibrates between the gas and liquid phases. Based on this 13C enrichment, we estimate that about 44.9% of the carbon atoms in the original Na2CO3 solution were replaced by 13CO2.
Figure 6 presents a schematic representation of the interaction mechanisms between CO2 and IL-loaded PEEK-ionene membranes, as evaluated using cryo ToF-SIMS. The figure highlights the distinction between strong and weak interactions. When strong interactions occur, such as chemical bonding, cryo ToF-SIMS can detect the relevant molecular signals, confirming the presence of chemical bonds. On the other hand, if only weak interactions, such as London dispersion forces, are present, cryo ToF-SIMS analysis confirms the absence of chemical bonding, as no significant molecular signals corresponding to strong interactions are observed. This method proves effective in determining whether CO2 is chemically bound to the membrane or merely interacting through weak van der Waals forces. This method can be broadly applied to assess various gas-material interactions. For instance, the hydrogen bond between water molecules (∼18–20 kJ/mol) serves as a reference point for evaluating interaction strengths. If an interaction is weaker than a hydrogen bond, small molecules can sublimate under high vacuum, even at low temperatures (−110 to −140°C). Stronger interactions, such as chemical bonds, can retain gas molecules in the matrix. The interaction strength can be further assessed by systematically increasing the temperature, providing a powerful tool to quantify gas-material interactions in various CO2 capture systems, including ionic liquid-loaded membranes like PEEK-ionene. This method serves as a screening tool to qualitatively evaluate gas-material interactions and determine whether chemical bonding or a reaction has occurred. For a more definitive assessment, techniques such as Infrared (IR) spectroscopy or calorimetry can be used. If multiple gases interact with the material and are expected to exhibit interactions weaker than hydrogen bonds, lowering the temperature may help distinguish their interactions with the membrane. A relevant cryogenic technique using liquid He is discussed in other group’s work (Suzuki and Iimura, 2024).
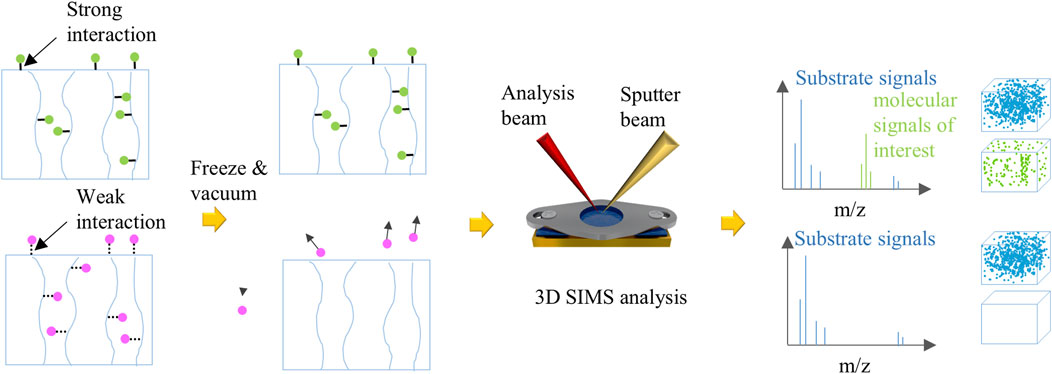
Figure 6. A schematic illustration of investigation of various interactions between gas molecules and samples using cryo ToF-SIMS. When gas molecules can form strong interaction with samples, e.g., equal to or stronger than hydrogen bond in ice, the gas molecules can stay in the sample during cryo ToF-SIMS testing. Two unique advantages are TOF-SIMS spectra can provide molecular information to distinguish the product, and 3D imaging can track gas molecules distribution in the sample. Also, combined with isotopic labeling, it is possible to quantify trace gas molecules in the samples. If only weak interactions exist between gas molecules and the samples, such as van der Waals forces, the gas molecules escape into vacuum during cryo TOF-SIMS testing, and little change can be observed before and after gas loading.
The results in this work hold significant potential for post-combustion CO2 capture research. For example, the interaction of SOx (sulfur oxides) and NOx (nitrogen oxides) with CO2 capture solvents like EEMPA (Zheng et al., 2020) can form heat-stable salts with SOx and NOx. This methodology can help further the understanding of SOx and NOx sorption in carbon capture media (Wang et al., 2021; Boumghar et al., 2020) as detecting and quantifying low concentrations of SOx and NOx is challenging for conventional experimental methodologies like NMR and IR. Given the excellent detection limits of ToF-SIMS (ppm to ppb level) (Lockyer et al., 2024; Benninghoven, 1994), we expect that combining isotopic labeling (e.g., 15NOx, S18O2) with cryo-ToF-SIMS will enable confident quantification of these gases in CO2 capture systems, providing critical insights into mechanisms of heat stable salt formation. Future studies will focus on this approach, offering a pathway to better understand the role of these flue gas components in solvent-based carbon capture.
Moreover, controlling the interaction strength between CO2 and PEEK-ionene membranes is crucial for optimizing CO2 permeability and diffusion. Since CO2 can react with H2O to form carbonic acid (H2CO3), the introduction of basic functional groups like -NH2 into the PEEK-ionene membrane could significantly influence CO2-membrane interactions. Basic groups can promote chemical reactions with CO2, leading to stronger and less reversible interactions. This would trap CO2 within the membrane, reducing its permeability and overall efficiency of gas separation. Therefore, our experimental observations suggest that avoiding basic conditions is essential to maintain optimal membrane performance.
4 Conclusion
In this study, 13CO2 and D2O was introduced to the IL-loaded PEEK-ionene membranes and cryo ToF-SIMS was utilized to examine interactions among CO2, water and the membrane. A special sample block was designed and fabricated that allows direct sample cooling under 13CO2 loading condition and facilitates subsequent sample transferring under low temperature with little ice condensation on the sample surface. While previous NMR data show that 13CO2 can be absorbed into the PEEK-ionene membrane under ambient condition, little enhancement of 13C/12C ratio (compared to natural abundance) was observed in our cryo ToF-SIMS data, suggesting that the CO2-membrane interactions are weak, leading to CO2 vaporization even at low temperatures (−140°C) under vacuum condition. In contrast, cryo ToF-SIMS detected D2O in the heavy water-loaded membrane, showing a relatively homogeneous 3D distribution within the membrane with some highlighted locations, possibly in the pores induced by CO2 loading. This implies that CO2-membrane interaction is weaker than the water-membrane interaction, as well as hydrogen bond in water (approximately 18–20 kJ/mol), which is consistent with our prior NMR and computer simulation results. Additionally, cryo ToF-SIMS did not detect 13CO2 in the membrane even with the co-existence of D2O, indicating weak CO2-D2O interactions, thereby suggesting minimal interference from water vapor on CO2 diffusion. As a comparison, our ToF-SIMS data show that 13CO2 can readily react with a basic Na2CO3 aqueous solution to form NaH13CO3, indicating a chemical interaction can effectively retain CO2 gas molecules under cryogenic conditions. This observation suggests the importance of controlling the conditions for CO2-membrane interactions. Specifically, it is recommended to avoid basic conditions when using the PEEK-ionene membrane for CO2 separation, as such conditions may lead to unwanted interactions, potentially compromising the membrane’s performance and the efficiency of CO2 separation. These observations suggest that our cryo ToF-SIMS method is a unique tool to examine interaction strength between various gas-material interactions. Also, the molecular recognition capability of ToF-SIMS can effectively elucidate the molecular structures of new chemical species after gas loading. Furthermore, a combination of isotopic labeling and cryo ToF-SIMS can be used to quantify impurities or poisonous gases in water-lean CO2 capture solvents. These advantages position cryo ToF-SIMS as a powerful analytical tool for advancing CO2 capture research and industrial application.
Data availability statement
The raw data supporting the conclusions of this article will be made available by the authors, without undue reservation.
Author contributions
JY: Formal Analysis, Investigation, Supervision, Writing–original draft, Writing–review and editing. JD: Data curation, Writing–review and editing. LS: Writing–review and editing. JB: Investigation, Supervision, Writing–review and editing. SR: Writing–review and editing. EW: Writing–review and editing. YC: Writing–review and editing. DH: Conceptualization, Funding acquisition, Investigation, Writing–review and editing. ZZ: Formal Analysis, Investigation, Methodology, Supervision, Writing–original draft, Writing–review and editing.
Funding
The author(s) declare that financial support was received for the research and/or publication of this article. This work was supported by the U.S. Department of Energy, Office of Science, Basic Energy Sciences, Chemical Sciences, Geosciences, and Biosciences Division, Materials Science and Separation Science programs, FWP 76830.
Acknowledgments
The SIMS characterizations were conducted in the William R. Wiley Environmental Molecular Sciences Laboratory (EMSL), a national scientific user facility sponsored by DOE’s Office of Biological and Environmental Research and located at PNNL.
Conflict of interest
The authors declare that the research was conducted in the absence of any commercial or financial relationships that could be construed as a potential conflict of interest.
The author(s) declared that they were an editorial board member of Frontiers, at the time of submission. This had no impact on the peer review process and the final decision.
Generative AI statement
The author(s) declare that no Generative AI was used in the creation of this manuscript.
Publisher’s note
All claims expressed in this article are solely those of the authors and do not necessarily represent those of their affiliated organizations, or those of the publisher, the editors and the reviewers. Any product that may be evaluated in this article, or claim that may be made by its manufacturer, is not guaranteed or endorsed by the publisher.
Supplementary material
The Supplementary Material for this article can be found online at: https://www.frontiersin.org/articles/10.3389/fchem.2025.1564084/full#supplementary-material
References
Benninghoven, A. (1994). Chemical analysis of inorganic and organic surfaces and thin films by static time-of-flight secondary ion mass spectrometry (TOF-SIMS). Angewandte Chemie Int. Ed. Engl. 33, 1023–1043. doi:10.1002/anie.199410231
Boumghar, S., Bedel, S., Sigot, L., and Vallières, C. (2020). Adsorption of CO2 in presence of NOx and SOx on activated carbon textile for CO2 capture in post-combustion conditions. Adsorption 26, 1173–1181. doi:10.1007/s10450-020-00207-6
Cannon, D. M., Pacholski, M. L., Winograd, N., and Ewing, A. G. (2000). Molecule specific imaging of freeze-fractured, frozen-hydrated model membrane systems using mass spectrometry. J. Am. Chem. Soc. 122, 603–610. doi:10.1021/ja992078p
Chen, Y., Nguyen, M.-T., Yao, J., Han, K. S., Ravula, S., Zhang, M., et al. (2025). CO2 in Ionene–Ionic liquid composite membranes. Adv. Sustain. Syst. doi:10.1002/adsu.202400802
Dekas, A. E., Poretsky, R. S., and Orphan, V. J. (2009). Deep-sea archaea fix and share nitrogen in methane-consuming microbial consortia. Science 326, 422–426. doi:10.1126/science.1178223
Franken, L. E., Grünewald, K., Boekema, E. J., and Stuart, M. C. A. (2020). A technical introduction to transmission electron microscopy for soft-matter: imaging, possibilities, choices, and technical developments. Small 16, 1906198. doi:10.1002/smll.201906198
Gao, D., Huang, X., and Tao, Y. (2016). A critical review of NanoSIMS in analysis of microbial metabolic activities at single-cell level. Crit. Rev. Biotechnol. 36, 884–890. doi:10.3109/07388551.2015.1057550
Guo, W., Kanski, M., Liu, W., Gołuński, M., Zhou, Y., Wang, Y., et al. (2020). Three-dimensional mass spectrometric imaging of biological structures using a vacuum-compatible microfluidic device. Anal. Chem. 92, 13785–13793. doi:10.1021/acs.analchem.0c02204
Heldebrant, D. J., Koech, P. K., Glezakou, V.-A., Rousseau, R., Malhotra, D., and Cantu, D. C. (2017). Water-lean solvents for post-combustion CO2 capture: fundamentals, uncertainties, opportunities, and outlook. Chem. Rev. 117, 9594–9624. doi:10.1021/acs.chemrev.6b00768
Heldebrant, D. J., Yonker, C. R., Jessop, P. G., and Phan, L. (2008). Organic liquid CO2 capture agents with high gravimetric CO2 capacity. Energy and Environ. Sci. 1, 487–493. doi:10.1039/b809533g
Hoppe, P., Cohen, S., and Meibom, A. (2013). NanoSIMS: technical aspects and applications in cosmochemistry and biological geochemistry. Geostand. Geoanalytical Res. 37, 111–154. doi:10.1111/j.1751-908x.2013.00239.x
Houssiau, L., and Mine, N. (2010). Molecular depth profiling of polymers with very low energy reactive ions. Surf. Interface Analysis 42, 1402–1408. doi:10.1002/sia.3159
Jansen, J. C., and Drioli, E. (2009). Poly(ether ether ketone) derivative membranes—a review of their preparation, properties and potential. Polym. Sci. Ser. A 51, 1355–1366. doi:10.1134/s0965545x09110200
Kammakakam, I., O’Harra, K. E., Bara, J. E., and Jackson, E. M. (2022). Spirobisindane-containing imidazolium polyimide ionene: structural design and gas separation performance of “Ionic PIMs”. Macromolecules 55, 4790–4802. doi:10.1021/acs.macromol.1c02317
Kourkoutis, L. F., Plitzko, J. M., and Baumeister, W. (2012). Electron microscopy of biological materials at the nanometer scale. Annu. Rev. Mater. Res. 42, 33–58. doi:10.1146/annurev-matsci-070511-155004
Leclaire, J., Heldebrant, D. J., Grubel, K., Septavaux, J., Hennebelle, M., Walter, E., et al. (2024). Tetrameric self-assembling of water-lean solvents enables carbamate anhydride-based CO2 capture chemistry. Nat. Chem. 16, 1160–1168. doi:10.1038/s41557-024-01495-z
Li, Q., Chang, J., Li, L., Lin, X., and Li, Y. (2023). Research progress of nano-scale secondary ion mass spectrometry (NanoSIMS) in soil science: evolution, applications, and challenges. Sci. Total Environ. 905, 167257. doi:10.1016/j.scitotenv.2023.167257
Lockyer, N. P., Aoyagi, S., Fletcher, J. S., Gilmore, I. S., VAN DER Heide, P. A. W., Moore, K. L., et al. (2024). Secondary ion mass spectrometry. Nat. Rev. Methods Prim. 4, 32. doi:10.1038/s43586-024-00311-9
Lombardo, T., Walther, F., Kern, C., Moryson, Y., Weintraut, T., Henss, A., et al. (2023). ToF-SIMS in battery research: advantages, limitations, and best practices. J. Vac. Sci. and Technol. A 41. doi:10.1116/6.0002850
Moore, J. K., Surface, J. A., Brenner, A., Skemer, P., Conradi, M. S., and Hayes, S. E. (2015). Quantitative identification of metastable magnesium carbonate minerals by solid-state 13C NMR spectroscopy. Environ. Sci. and Technol. 49, 657–664. doi:10.1021/es503390d
O’Harra, K., Kammakakam, I., Shinde, P., Giri, C., Tuan, Y., Jackson, E. M., et al. (2022). Poly(ether ether ketone) Ionenes: ultrahigh-performance polymers meet ionic liquids. ACS Appl. Polym. Mater. 4, 8365–8376. doi:10.1021/acsapm.2c01312
Pareek, V., Tian, H., Winograd, N., and Benkovic, S. J. (2020). Metabolomics and mass spectrometry imaging reveal channeled de novo purine synthesis in cells. Science 368, 283–290. doi:10.1126/science.aaz6465
Pett-Ridge, J., and Weber, P. K. (2022). “NanoSIP: NanoSIMS applications for microbial biology,” in Microbial systems biology: methods and protocols. Editor A. NAVID (New York, NY: Springer US).
Pironti, C., Cucciniello, R., Camin, F., Tonon, A., Motta, O., and Proto, A. (2017). Determination of the 13C/12C carbon isotope ratio in carbonates and bicarbonates by 13C NMR spectroscopy. Anal. Chem. 89, 11413–11418. doi:10.1021/acs.analchem.7b02473
Ravula, S., Chen, Y., Wise, K. W., Shinde, P. S., Walter, E. D., Karkamkar, A., et al. (2024). Precisely segmented PEEK–ionene + ionic liquid composite membranes for CO2 separation. J. Mater. Chem. A 12, 2184–2199. doi:10.1039/d3ta05223k
Sin, M., Kavoosi, N., Rauche, M., Pallmann, J., Paasch, S., Senkovska, I., et al. (2019). In situ 13C NMR spectroscopy study of CO2/CH4 mixture adsorption by metal–organic frameworks: does flexibility influence selectivity? Langmuir 35, 3162–3170. doi:10.1021/acs.langmuir.8b03554
Strange, L. E., Heldebrant, D. J., Ravula, S., Chen, P., Zhu, Z., Bara, J. E., et al. (2024b). In-house synthesized poly(ether ether ketone) ionenes. I. ToF-SIMS spectra in the positive ion mode. Surf. Sci. Spectra 31. doi:10.1116/6.0003132
Strange, L., Ravula, S., Zhu, Z., Bara, J. E., Chen, P., Heldebrant, D. J., et al. (2024a). In-house synthesized poly(ether ether ketone) ionenes. II. ToF-SIMS spectra in the negative ion mode. Surf. Sci. Spectra 31. doi:10.1116/6.0003133
Suzuki, T. T., and Iimura, S. (2024). Quench-condensed hydrogen films studied by cryogenic time-of-flight secondary ion mass spectrometry. Phys. Rev. B 110, 085426. doi:10.1103/physrevb.110.085426
Walter, E. D., Zhang, D., Chen, Y., Sung Han, K., Bazak, J. D., Burton, S., et al. (2023). Cover feature: enhancing CO2 transport across a peek-ionene membrane and water-lean solvent interface (chemsuschem 13/2023). ChemSusChem 16, e202300898. doi:10.1002/cssc.202300898
Wang, S., Xu, S., Gao, S., Xiao, P., Jiang, M., Zhao, H., et al. (2021). Simultaneous removal of SO2 and NOx from flue gas by low-temperature adsorption over activated carbon. Sci. Rep. 11, 11003. doi:10.1038/s41598-021-90532-9
Wendler, K., Thar, J., Zahn, S., and Kirchner, B. (2010). Estimating the hydrogen bond energy. J. Phys. Chem. A 114, 9529–9536. doi:10.1021/jp103470e
Williams, P. (1985). Secondary ion mass spectrometry. Annu. Rev. Mater. Res. 15, 517–548. doi:10.1146/annurev.matsci.15.1.517
Winey, M., Meehl, J. B., O'Toole, E. T., Thomas, H., and Giddings, J. (2014). Conventional transmission electron microscopy. Mol. Biol. Cell 25, 319–323. doi:10.1091/mbc.e12-12-0863
Zheng, R. F., Barpaga, D., Mathias, P. M., Malhotra, D., Koech, P. K., Jiang, Y., et al. (2020). A single-component water-lean post-combustion CO2 capture solvent with exceptionally low operational heat and total costs of capture – comprehensive experimental and theoretical evaluation. Energy and Environ. Sci. 13, 4106–4113. doi:10.1039/d0ee02585b
Zheng, X., Rafailovich, M. H., Sokolov, J., Strzhemechny, Y., Schwarz, S. A., Sauer, B. B., et al. (1997). Long-range effects on polymer diffusion induced by a bounding interface. Phys. Rev. Lett. 79, 241–244. doi:10.1103/physrevlett.79.241
Keywords: cryo ToF-SIMS, PEEK-ionene membrane, intermolecular interactions, isotopic labelling, gas separation, ionic liquid
Citation: Yao J, Dhas JA, Strange LE, Bara JE, Ravula S, Walter ED, Chen Y, Heldebrant DJ and Zhu Z (2025) Investigating intermolecular interactions among CO2, water and PEEK-ionene membrane using cryo ToF-SIMS and isotopic labeling. Front. Chem. 13:1564084. doi: 10.3389/fchem.2025.1564084
Received: 21 January 2025; Accepted: 24 February 2025;
Published: 14 March 2025.
Edited by:
Lu-Tao Weng, Hong Kong University of Science and Technology (Guangzhou), ChinaReviewed by:
Yao Zhao, Chinese Academy of Sciences (CAS), ChinaZhaoying Wang, Minzu University of China, China
Yanyan Zhang, Chinese Academy of Sciences (CAS), China
Copyright © 2025 Yao, Dhas, Strange, Bara, Ravula, Walter, Chen, Heldebrant and Zhu. This is an open-access article distributed under the terms of the Creative Commons Attribution License (CC BY). The use, distribution or reproduction in other forums is permitted, provided the original author(s) and the copyright owner(s) are credited and that the original publication in this journal is cited, in accordance with accepted academic practice. No use, distribution or reproduction is permitted which does not comply with these terms.
*Correspondence: Jennifer Yao, amVubmlmZXIueWFvQHBubmwuZ292; Zihua Zhu, emlodWEuemh1QHBubmwuZ292