- 1Department of Chemistry, Government College University Faisalabad, Faisalabad, Pakistan
- 2Department of Pharmaceutics, Government College University, Faisalabad, Pakistan
- 3Department of Basic Sciences, Department of Histology, Embriology and Cytophysiology, Medical University of Lublin, Lublin, Poland
- 4Faculty of Health Sciences Collegium Medicum, The Mazovian Academy in Plock, Płock, Poland
2,3-Dihydrobenzofurans are noteworthy scaffolds in organic and medicinal chemistry, constituting the structural framework of many of the varied medicinally active organic compounds. Moreover, a diverse variety of biologically potent natural products also contain this heterocyclic nucleus. Reflecting on the wide biological substantiality of dihydrobenzofurans, several innovative and facile synthetic developments are evolving to achieve these heterocycles. This review summarizes the transition-metal-free, efficient, and novel synthetic pathways toward constructing the dihydrobenzofuran nucleus established after 2020.
1 Introduction
Five-membered heterocycles and their derivatives exhibit a remarkable role in the pharmaceutical industry (Delost et al., 2018; Irfan et al., 2023a; Irfan et al., 2023b). 2,3-Dihydrobenzofurans are composed of a benzene ring attached to a dihydrofuran skeleton (oxygen involving a five-membered ring), which can easily be obtained as a result of hydrogenation of benzofurans (benzene attached to a furan ring). The 2,3-dihydrobenzofurans were formerly called “coumarane.” Dihydrobenzofurans are generally neutral or weakly basic. A diverse range of medicinally active organic compounds are known to include the dihydrobenzofuran ring and its derivatives in their structural framework, thereby emphasizing the biological potential of this moiety (Khanam, 2015; Naik et al., 2015; Sachdeva et al., 2022; Farooq et al., 2019). The general structure of dihydrobenzofurans has been presented in Figure 1 (Chen et al., 2019).
Various naturally occurring organic compounds are also composed of 2,3-dihydrobenzofuran ring skeletons which play a significant role in medicinal chemistry (Chen et al., 2019; Macías et al., 1999; Pieters et al., 1999). Some of the significant biologically active natural products constituting dihydrobenzofuran core in their skeletal framework are (+)-lithospermic acid (Varadaraju and Hwu, 2012) 2, (−)-linderol A (Mimaki et al., 1995; Yamashita et al., 2003) 3, bisabosqual A (Minagawa et al., 2001; Snider and Lobera, 2004; am Ende et al., 2013) 6, and (+)-decursivine (Sun et al., 2011) 4, which are used against human immunodeficiency virus, inhibit the melanin’s biosynthesis, and treat fungal diseases and malarial infection, respectively. (+)-Conocarpan (Chen and Weisel, 2013; Zheng et al., 2003) 5 is another naturally occurring dihydrobenzofuran constituting organic compound known for its broad pharmaceutical applications, including anti-trypanosomal, insecticidal, and anti-fungal action (Figure 2). This unparalleled medicinal effectivity has resulted in several endeavors regarding the procurement of dihydrobenzofuran-based compounds.
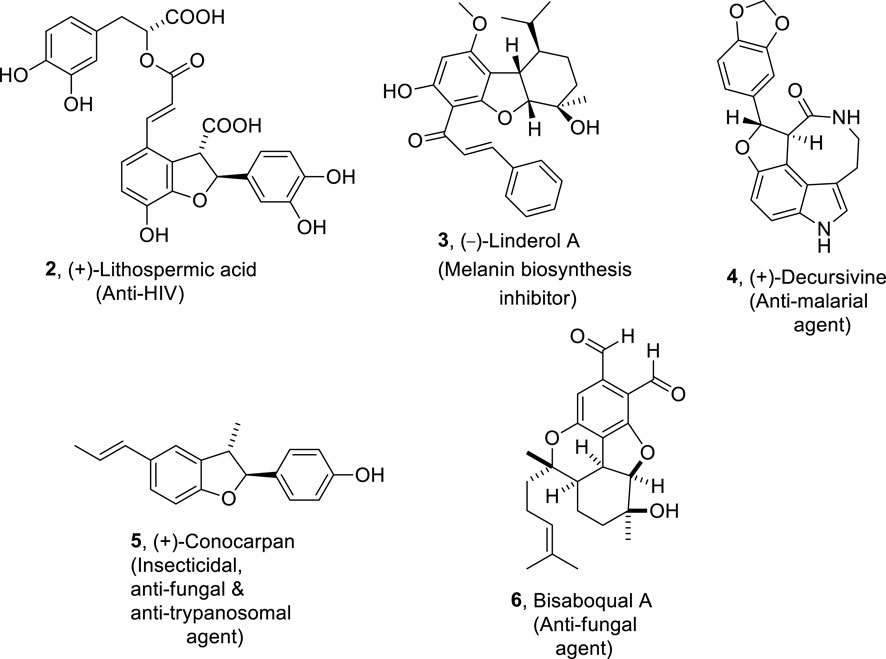
Figure 2. Structures of the dihydrobenzofuran ring constituting biologically active natural products.
The initial work on the synthesis of dihydrobenzofuran was reported in 1892. Since then, several methodologies have been presented concerning the construction of the dihydrobenzofuran skeleton. However, the synthetic routes developed in the early twentieth century were not found to be satisfactory in terms of yield and productivity. The developed protocols suffered from certain challenges, including steps involving the utilization of toxic and costly metal catalysts, harsh reaction conditions, and restricted substrate scope (Li et al., 2023). Most of the developed methods involve the use of pre-functionalized radicals as starting materials (Chen et al., 2023). Moreover, in a few instances, reactivity was observed to be diminished due to steric crowding (Lu et al., 2021). Over the years, researchers have proposed numerous novel, efficient, and high-yielding alternative synthetic pathways to achieve dihydrobenzofurans (Alexander, 1892; Rao et al., 2003).
Transition metal-catalyzed reactions have gained considerable significance owing to their employment in achieving several organic compounds (Pannilawithana et al., 2021; Bai et al., 2018). Among diversely reported efficient strategies for synthesizing dihydrobenzofurans and their derivatives, transition metal catalysis has also taken a significant locale in this domain. Various transition metal catalysts, including ruthenium (Sau et al., 2023), iridium (Kusaka et al., 2022), rhodium (Jiang et al., 2022), gold (Morita et al., 2023), nickel (Jin et al., 2022), palladium (Sun et al., 2023; Ding et al., 2023; Kang et al., 2022), platinum (Yang et al., 2023), vanadium (Wang et al., 2023), silver (Guo et al., 2023), tungsten (Gowda et al., 2023), and copper (Zhu et al., 2023; Rao and Islam, 2021), have been exploited to introduce the efficient catalytic systems for the construction of dihydrobenzofuran nucleus by treating different substrates. Individual and dual metal catalysis approaches have been demonstrated to afford high-yielding dihydrobenzofurans in a feasible manner (Huang et al., 2022; Zhao et al., 2023).
Apart from widely employed transition metal catalysis, several transition metal-free approaches regarding the synthesis of dihydrobenzofurans and their derivatives have been accomplished in recent years, which involved the utilization of visible light-promoted preparation (Caiuby et al., 2018), electrocatalysis (Kärkäs, 2018), and Bronsted (Suneja and Schneider, 2018) and Lewis (Sharma et al., 2017) acid-mediated synthetic routes to forge the dihydrobenzofuran nucleus. With respect to the increasing concerns regarding ever-increasing environmental pollution, catalyst-free synthesis has also gained definite significance as per green chemistry criteria (Chen et al., 2018).
Several reviews have been published highlighting the significance of dihydrobenzofurans and their derivatives. Bertolini and Pineschi (2009) reported a review on the development of benzofuran synthesis. In 2011, Sheppard provided an overview of newly developed synthetic pathways to achieve dihydrobenzofurans (Sheppard, 2011). In 2019, Chen et al. covered the synthesis of natural products involving dihydrobenzofuran rings in their structural formula (Chen et al., 2019). Afterward, in early 2020, a review covering the synthetic protocols developed in 2012–2019 was put forward by Laurita et al. (2020). Similarly, in 2022, a comprehensive review was published by Dapkekar et al. (2022), which focused on the newly developed synthetic routes to furnish the 2,3-dihydrobenzofurans and 1,3-dihydroisobenzofurans along with their applications. Recently, transition metal-catalyzed protocols to access dihydrobenzofuran nuclei have been reviewed by our group (Ashraf et al., 2024). Currently, developments are in progress regarding the construction of the dihydrobenzofuran skeleton. The focus of this review is to summarize the transition metal-free novel synthetic protocols toward the construction of dihydrobenzofurans developed during 2021–2023.
2 Review of the literature
2.1 Bronsted acid-induced synthesis of dihydrobenzofurans
Since the beginning of the twenty-first century, Bronsted acids have been widely utilized as efficacious catalysts for the formation of C–C bonds as they activate the electrophilic centers for facile nucleophilic addition reactions (Yamamoto and Ishihara, 2008; Jacob et al., 2017). Yang et al. (2021) reported the Bronsted acid- [i.e., polyphosphoric acid (PPA)]-mediated synthesis of 2,3-dihydrobenzofuran derivatives 8. In their novel approach, ortho-allyl/prenyl phenols 7 were transformed into desired cyclic heterocycles 8 via the activation of phenolic oxygen by PPA in dimethylformamide. The proposed mechanism involved the generation of phosphorylated adduct A by treating substituted phenols 7 with PPA at increased temperature. The disruption of electron stability in A gave rise to intermediate B, which subsequently led to the synthesis of dihydrobenzofurans 8 via nucleophilic annulation. The target molecules were obtained in moderate to excellent yields (52%–90%) by using the above-mentioned strategy (Scheme 1).
In the same year, another phosphoric acid (PA)-catalyzed synthesis of dihydrobenzofurans was documented by Zhang et al. (2021) by employing a [3 + 2] annulation reaction, which is widely carried out to construct sophisticated heterocyclic functionalities. They designed a facile methodology for the enantioselective preparation of 2,3-dihydrobenzofuran derivatives 11 via stereoselective [3 + 2] annulation. In their innovative methodology, substituted quinone monoimines 9 were reacted with 3-hydroxymaleimides 10 in the presence of asymmetric phosphoric acid catalyst (PA) in dichloroethane (DCE) to achieve moderate to excellent yields (62%–99%) of dihydrobenzofurans with remarkable enantioselectivities (49%–99% ee). The plausible reaction mechanism commenced with the activation of both reactants by PA, followed by the attack of 3-hydroxymaleimides 10 on quinone monoimines 9 to generate intermediate A, which further underwent 1,4-addition and aromatization to afford intermediate B. Upon intramolecular cyclization, the intermediate B furnished the desired succinimide fused 2,3-dihydrobenzofurans 11 (Scheme 1) (Zhang et al., 2021).
In order to overcome microbial diseases, synthetic researchers have carried out several synthetic efforts to afford efficient antimicrobial agents (Breijyeh and Karaman, 2023; Zahoor et al., 2017). Yan et al. (2022) demonstrated another productive enantioselective route to synthesize dihydrobenzofurans 14 via a chiral phosphoric acid-catalyzed [3 + 2] annulation reaction between quinone imines 12 and 4-aminoisoxazoles 13 by utilizing dichloromethane as solvent. The reaction mechanism was believed to involve a 1,4-addition reaction followed by an aromatization reaction to yield intermediate B. The intermediate B was then subjected to cyclization to afford target isoxazoline-based-dihydrobenzofurans 14 in efficient yields. The resulting dihydrobenzofurans 14 were then found to be effective against disease-causing fungi in plants (Scheme 2).
Li et al. (2023) also discussed the Bronsted acid (p-toluene sulfonic acid)-mediated [3 + 2] cycloaddition reaction involving the synthesis of dihydrobenzofurans. The newly developed efficient synthetic protocol involved the treatment of substituted styrylnaphthols 16 and a variety of substituted allylic alcohols 15 via toluene sulfonic acid-promoted series of cyclization reactions in dichloromethane to result in a library of dihydronaphthofurans 17 in moderate to excellent yields (48%–99%). The developed methodology involved the TsOH-promoted [3 + 2] cycloaddition reaction to generate intermediate A, which was subjected to an intramolecular addition reaction with a subsequent 1,2-hydride shift to form intermediate C. The resulting intermediate C then afforded the target molecules 17 in efficient yields with confined diastereoselective ratios (more than 20:1) on the subsequent addition of a nucleophile along with the removal of a proton. (Scheme 2).
Nakamura et al. (2022) established the chalcone rearrangement strategy by treating chalcone 18 utilizing p-toluene sulfonic acid in acetonitrile to synthesize 2,3-dihydrobenzofuran 19 in efficient yields (Scheme 3). The dihydrobenzofuran derivatives obtained via mentioned protocol were then transformed into two different types of benzofuran derivatives by employing varied reaction conditions. Slightly acidic or basic conditions gave 3-acylbenzofurans, while conversion using p-toluene sulfonic acid resulted in the synthesis of 3-formylbenzofuran heterocycles.
In organic synthesis, various types of condensation reactions are applied to furnish several heterocycles. Grishin et al. (2022) proposed a novel and facile synthetic protocol for the synthesis of benzofuran derivatives via a Bronsted-acid-promoted condensation reaction between phenols 20 and nitrovinyl-substituted indoles 21. The reaction was carried out in the presence of MsOH in 4 mL at 40°C to obtain high yields of benzofuran derivatives 22 (Scheme 3).
Bronsted acid-catalyzed [4 + 1] annulation reactions have also been applied to furnish heterocyclic scaffolds (Das et al., 2023). Lu et al. (2021) afforded the synthesis of 2,3-dihydrobenzofuran derivatives 25 (comprising of a quaternary C center) via a metal-free, TfOH-catalyzed protocol. In their developed synthetic route, p-quinone methides 23 were reacted with α-aryl diazoacetates 24 via [4 + 1] annulation. The suggested mechanistic insights revealed that the oxonium ion intermediate A was formed from 23 after interaction with TfOH followed by the generation of phenol-substituted intermediate C by undergoing a Michael addition with diazoester B. This intermediate C then underwent nucleophilic substitution reaction to form the desired products 25 in moderate to high yield (40%–86%) with significant diastereoselectivity (3:0:1 dr) (Scheme 4).
Corey–Chaykovsky reactions are carried out to achieve the synthesis of cyclopropanes, aziridine, and oxiranes by treating sulfur ylides with alkenes, imines, and carbonyl compounds, respectively (Heravi et al., 2016). Fadeev et al. (2022) accomplished a concise and efficient methodology for the preparation of a library of 2,3-dihydrobenzofuran scaffolds 27 by utilizing an extended Corey–Chaykovsky reaction. In their optimized strategy, 2-hydroxychalcones 26 were used as starting materials, which underwent further transformations in the presence of a Brønsted acid (Amberlyst 15) and Corey ylide (Me3SOI) to form 2,3-dihydrobenzofurans 27 in low to high yields (22%–75%). The recommended mechanistic details inferred that 2-hydroxychalcone 26 reacted with Me3SOI to form a reactive cyclopropane intermediate A, which was further treated with an alkali solution, followed by subsequent dehydration to give intermediate B. The intermediate B upon intramolecular oxa-Michael reaction resulted in 2,3-dihydrobenzofurans 27 (Supplementary Scheme 4).
2.2 Bronsted–Lewis acid-induced synthesis of dihydrobenzofurans
Chen et al. (2023) reported a combination of Lewis and Bronsted acid (boron trifluoride diethyletherate and propionic acid)-catalyzed synthesis of 3-enamide-substituted dihydrobenzofurans 30 via the reaction of 1,6-enynes 28 and nitriles 29 in DCE under a nitrogen atmosphere. This synthetic approach has a diverse substrate scope with the chemoselective unistep construction of C–C and C–N bonds to facilitate the moderate to high yields of target molecules, that is, 49%–82%. As per the documented mechanistic details, the Bronsted acid interacted with 1,6-enyne 28 to generate an intermediate A, which underwent an electrophilic addition reaction with propionic acid to afford the oxonium intermediate B. In the next step, intermediate B underwent cyclization and reacted with nitrile 29 to give another intermediate C. Upon hydrolysis of intermediate C, desired 2,3 disubstituted dihydrobenzofurans 30 were attained (Scheme 5).
2.3 Photocatalytic synthesis of dihydrobenzofurans
Visible light-catalyzed reactions are widely utilized to carry out profitable organic syntheses (Vellakkaran and Hong, 2021; Mueller et al., 2015; Chen et al., 2012). Shen et al. (2022) designed a visible light-mediated multicomponent cascade protocol for the synthesis of difluoroamidosulfonylated dihydrobenzofurans 34 via condensation of N-allylbromodifluoroacetamides 31, terminal alkynes 33, and DABCO. (SO2)2 32. The conditions employed for this tandem reaction incorporated NaHCO3 (as the base) and DMA (as the solvent) to obtain good yields of dihydrobenzofurans. In their synthetic practice, a plausible mechanism involved the combination of allylbromodifluoroacetamides 31 and DABCO. (SO2)2 32 followed by visible light irradiation to generate radical intermediate A. The intermediate A then swiftly underwent 5-exo trig radical-mediated cyclization, proceeded by entangling sulfur dioxide to acquire the difluoroamidosulfonyl radical B. The intermediate B was then made to react with terminal alkynes 33 followed by 1,5-hydrogen atom transfer (HAT), which caused the emergence of another radical intermediate C. Later, the intermediate C encountered additional 5-exo trig radical annulation in sequence with the addition of hydrogen atom to secure dihydrobenzofurans 34 in efficient yields (Scheme 6).
Another synthetic approach involving the visible light-mediated synthesis of dihydrobenzofurans was presented by Li et al. (2022). In this approach, substituted alkynyl ethers 33 were made to react with a diverse variety of sulfonyl chlorides 35 in the presence of photocatalyst and 2-MeTHF solvent without any additive to accomplish sulfonyl-substituted dihydrobenzofuran derivatives 36 in a 56%–93% yield range. The reaction mechanism was assumed to involve the excitation of alkynyl ethers 33 by visible light, followed by quenching with sulfonyl chloride 35 to give radical intermediate A, which upon intramolecular 1,5-hydrogen atom transfer gave rise to radical intermediate B. The radical intermediate B was then believed to undergo 5-exo trig (radical) cyclization to develop intermediate C, which led to the procurement of dihydrobenzofuran derivatives 36 after the abstraction of proton (Scheme 7).
In 2023, Corti et al. (2023) developed a facile and efficient photo-induced method for the synthesis of sulfonated 2,3-dihydrobenzofuran derivatives 38. In their metal-free strategy, 2-allyphenol derivatives 7 were made to react with α-iodo sulfones/alkyl iodide 37 in the presence of 1,1,3,3-tetramethylguanidine (TMG used as a base) and 1,2-dichlorobenzene (as a solvent) within a short duration (i.e., 35 min). This methodology covered a broad substrate scope with low to high yield (29%–69%) of target molecules. The synthesis commenced from the generation of phenolate anions B from 2-allyphenol derivatives 7 that stimulated the α-iodo sulfones/alkyl iodide 37 to form radical precursor A, which further reacted with the alkene part of the B to proceed via the tandem atom transfer radical addition (ATRA) cycle. The ATRA cycle generated intermediate D through the halogen atom transfer of in situ intermediate C, which, upon the subsequent nucleophilic substitution reaction, synthesized the desired 2,3-dihydrobenzofuran derivative 38 (Scheme 8).
Maejima et al. (2023) envisioned a facile, one-pot, photocatalytic methodology for the synthesis of regioselective C3-substituted dihydrobenzofuran derivatives 41. In this regard, p-benzoquinones 40 were reacted with substituted alkenes 39 in the presence of catalytic amounts of Lewis acid (LA) B(C6F5)3 and Lewis base P (o-tol)3. The mechanistic investigations revealed the initial excitation and intersystem crossing of p-benzoquinones to generate intermediate A, followed by radical addition to alkenes, thereby leading to intermediate B. The Paterno–Buchi reaction [2 + 2 cycloaddition] thus resulted in the formation of oxetane intermediate C, which, upon coordination with a Lewis acid, generated oxetane-Lewis acid intermediate D followed by the dienone-phenol rearrangement to form the C3-substituted dihydrobenzofuran derivatives 41. High yields (94%) were achieved when the reaction was carried out in the presence of an LED source (450 nm) in chloroform (solvent) (Scheme 9).
Being an integral structural constituent of various natural products, substituted dihydrobenzofurans have gained tremendous weight in organic synthesis. In this perspective, Jabeen et al. (2023) reported a methodology illustrating the role of a type II sensitized photooxidation and aprotic solvent toward the synthesis of 2,3-dihydrobenzofurans 43. In this regard, o-prenyl phenol 42 was oxidized in the presence of type II oxygen species (1O2) and benzene (aprotic solvent) to synthesize 2,3-dihydrobenzofurans 43. The aprotic solvent (benzene) was observed to increase the quenching rate by about ∼10-fold as compared to a protic solvent (methanol), thereby enhancing the reactivity of 1O2, which resulted in the formation of intermediate A. On decomposition, this intermediate A furnished the desired 2-(prop-1-en-2-yl)-2,3-dihydrobenzofurans 43 (up to 20%) and H2O2 (Scheme 10).
Covalent organic frameworks (COFs) are important structural units that can be employed as convenient heterogeneous photocatalysts for many organic conversions, such as coupling reactions and olefin isomerization (Yu et al., 2021; Guo and Jiang, 2020; Ma et al., 2018). Parvatkar et al. (2023) proposed a photo-induced, metal-free [3 + 2] cycloaddition involving protocol for the efficient synthesis of dihydrobenzofuran derivatives. For this purpose, they utilized a previously synthesized heterogenous photo-active catalyst, that is, Hex-Aza-COF, and exploited it for the construction of dihydrobenzofuran motifs 47 and 48. The reaction of substituted phenols 44 with olefins 45 and 46 via the oxidative cycloaddition in the presence of photocatalyst Hex-Aza-COF-3 white LEDs and (NH4)2S2O8 (oxidant) in acetonitrile (solvent) furnished the 2,3-dihydrobenzofuran 47 (up to 95% yield) and dihydro-6H-benzofuro [3,2-c]chromene 48 (up to 94% yield) (Scheme 11). The proposed mechanism for this reaction involved the light irradiation of the photocatalyst to obtain e−-h+ separation of charges, which further resulted in the oxidation of phenol to generate intermediate A. The intermediate A was then subjected to the hydrogen atom transfer (HAT) process to result in intermediate B, which furnished intermediate C upon a [3 + 2] cycloaddition reaction with substituted olefins 45, which finally led to the desired dihydrobenzofurans via aromatization (Figure 3).
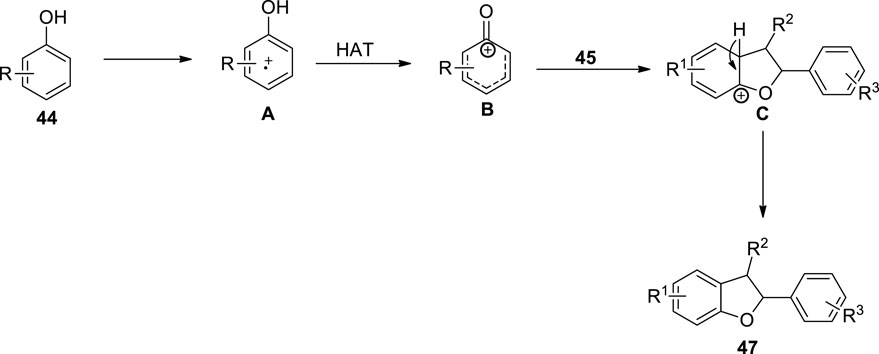
Figure 3. Proposed mechanism for the synthesis of dihydrobenzofuran 47 by a photocatalytic reaction.
Organic chemists are continuously progressing toward the total synthesis of natural products by using several synthetic routes (Faiz et al., 2017; Toure and Hall, 2009; Munawar et al., 2022). The dihydrobenzofuran derivatives synthesized using the above protocol were also applied as a precursor for the synthesis of naturally occurring compounds, namely, (±)-conocarpan 5 and (±)-pterocarpin 49 (Figure 4).
O'Callaghan et al. (2023) reported a metal-free protocol for the synthesis of dihydrobenzofuran derivatives 52 and 52′ via C-H insertion, which was achieved by flow photolysis of aryldiazoacetate 50. For this purpose, a photochemical chamber was utilized in which different wavelength filters (365–450 nm of LEDs or 250–390 nm of mercury lamp) with a pressure of 8 bar (generated via back pressure regulator) were employed in tert-butyl methyl ether (TBME) to achieve low to moderate yields (up to 50%) of desired products 52 and 52′ (substituted dihydrobenzofuran). Moreover, in the presence of a suitable photosensitizer 51 (i.e., 4,4′-dimethoxybenzophenone), enhanced diastereoselective products (i.e., trans isomer) were observed (Scheme 12).
Zhou et al. (2021) put forward the visible light-mediated, base-catalyzed protocol for the synthesis of 2,3-dihydrobenzofurans 55 by making use of an O-H insertion reaction and cyclization. In their synthetic approach, diazo compounds 53 and substituted para-quinones 54 under the irradiation of blue LED and Cs2CO3 afforded the 2,3-disubstituted dihydrobenzofurans 55. The proposed mechanism suggested that the diazo compounds 53 acted as a source of free carbene species A, which reacted with p-quinone 54 via O-H insertion to generate intermediate B. The intermediate B swiftly went through base-mediated deprotonation to furnish intermediate C. Finally, cyclization of C, followed by hydrogenation, generated the desired dihydrobenzofuran scaffolds 55 (Scheme 13).
2.4 Base-induced synthesis of dihydrobenzofurans
Among surplus synthetic pathways to achieve dihydrobenzofurans, base-promoted approaches have gained noteworthy significance (Medishetti et al., 2021). Wang et al. (2023) established the total synthesis of dihydrobenzofuran, which constitutes the anti-platelet and vasodilator drug Beraprost. They utilized the innovative protocol to access the dihydrobenzofuran core by treating enol ether 57 (accessed by subjecting enal lactone 56 to several steps) with substituted thiophene-1,1-dioxide 59 by carrying out an inverse-electron demand Diels–Alder reaction (IEDDA) followed by cheletropic elimination of sulfur dioxide, removal of the halogen, and aromatization in 2,6-lutidine (base) to afford the dihydrobenzofuran core. The dihydrobenzofuran core 60 was achieved in 49% yield with 4:4:1 rr (regioisomeric ratio). The dihydrobenzofuran core-substituted compound 60 was then reacted further over several steps to yield a biologically active drug 61 (Scheme 14).
Cycloaddition reactions are widely utilized to construct diversely functionalized heterocycles, owing to their efficient high atom economic and high-yielding nature (Meloche and Ashfeld, 2017; Kobayashi and Jørgensen, 2002). In 2022, Yuan et al. (2022) developed a synthetic protocol for synthesizing 2,3-dihydrobenzofurans 64. In their developed pathway, N-vinyl oxindole nitrones 62 were reacted with o-silylphenyl triflates 63 in the presence of CsF (base) to furnish desired polycyclic 2,3-dihydrobenzofurans 64 in low to high yields (29%–92%) with a notable E/Z ratio (20:1). The advocated mechanism inferred the employment of o-silylphenyl triflates 63 as aryne precursors, which underwent [3 + 2] cycloaddition with N-vinyl oxindole nitrones 62 to generate a cycloadduct A, which was further transformed into intermediate B via [3,3] rearrangement. Next, this intermediate was converted into desired 2,3-dihydrobenzofurans 64 by undergoing a retro-Mannich reaction and intramolecular cyclization (Scheme 15).
In 2023, Guan et al. (2023) performed the very first [4 + 1] cycloaddition reaction to attain adjustable diastereoselective synthesis of dihydrobenzofurans 66. They treated azoalkenes 65 (utilized as synthon of one carbon) with para quinone methides 23 via a diastereodivergent cycloaddition reaction. The cesium carbonate (base)-catalyzed [4 + 1] cycloaddition reaction between the given substrates resulted in less crowded thermodynamic target molecules 66 (in a 58%–85% yield range). However, when the reaction was performed by utilizing phosphoric acid (a Bronsted acid) as a catalyst, H-bonding linkages gave rise to kinetic products dominantly (in the 70%–93% yield range). Their synthetic protocol involving [4 + 1] cycloaddition proceeded via oxa-1,6-addition and 1,4-addition operations (Scheme 16).
Fang et al. (2022) developed a green and effective base-mediated procedure to access 3-amino-2,3-dihydrobenzofurans via [4 + 1] cyclization of trimethylsulfoxonium iodide 68 and substituted 2-hydroxylimides 67 in moderate to excellent yields (50–94%). In the presence of a base (NaH), sulfur ylide B attacked anionic 2-hydroxylimide A, affording an intermediate C. The subsequent cyclization of the intermediate C gave rise to the aziridine formation D, followed by the intramolecular cyclization to acquire the desired 3-amino-2,3-dihydrobenzofurans 69 in DMSO (Scheme 17).
Epoxide ring opening reactions are of significant importance in the synthesis of diverse organic molecules (Faiz and Zahoor, 2016; Ahmad et al., 2018). Li et al.(2023) reported the synthesis of dihydrobenzofuran spirooxindole scaffolds 73 through a solvent-free grinding protocol. To accomplish this, they treated α-chlorooxindoles 70 with salicylaldehyde 71 in the presence of KOH via [4 + 1] cyclization to afford the desired product 73 in good to excellent yields (49%–92%) with notable diastereoselectivities (up to 98:2 dr). As per the proposed mechanistic details, enolate carbanion emerged by treating chlorooxindoles 70 with a Bronsted base (KOH), which then underwent Darzen-type epoxidation with salicylaldehyde 71 to generate two isomers, that is, syn 72 and the most probably anti isomer 72′ (more stable). Next, a base-mediated intramolecular furan ring-closing and epoxide ring-opening reaction of 72′ occurred, thereby leading to the formation of syn-2,3-dihydrobenzofuranspirooxindoles 73 (Scheme 18).
Nair et al. (2023) envisioned a base-mediated protocol for synthesizing isobenzofuranone-spiro-linked benzofuranone 76. In their synthetic approach, formyl triflates 74 were made to react with sulfonylphthalide 75 via a Hauser–Kraus reaction ([4 + 4] annulation reaction) in the presence of Cs2CO3 (base) in THF to synthesize the desired dihydrobenzofuranones 76 in low to high yields (32%–88%). According to the plausible mechanism investigation, the phthalide anion generated from 75 attacked the carbonyl center of 77, followed by the desulfonation to generate intermediate B, which, upon enolization and triflate capture, afforded an oxonium intermediate C. In the next step, the oxonium anion attacked the lactone carbon to form an eight-membered ring via the Dieckmann process, which underwent a ring-opening reaction with carbonate, followed by the elimination of CO2 to form the benzil intermediate D. Finally, dehydration of D leads to the formation of the benzofuranone scaffold 76 (Scheme 19).
Wang et al. (2022) disclosed a base that is a cesium carbonate-mediated reaction of β-nitro-ortho-hydroxystyrenes (EWG = NO2)/ortho-hydroxychalcones (EWG = COAr) 77 with Morita−Baylis−Hillman (MBH) maleimides 78 and nitriles 81 of isatin via cascade annulation to access dihydrobenzofuran-spirooxindole motifs 79, 80, and 82, respectively. The reaction was carried out in MeCN (as a solvent) for approximately 2 h to obtain a maximum yield of 89%. In this domino reaction, phenolates of hydroxychalcones/hydroxystyrenes 77 attacked at the α-position of MBH maleimides/nitriles 78 or 81 to result in the formation of an intermediate, which underwent a double Michael addition reaction to give final products 79, 80, and 82, respectively (Scheme 20) (Figure 5).
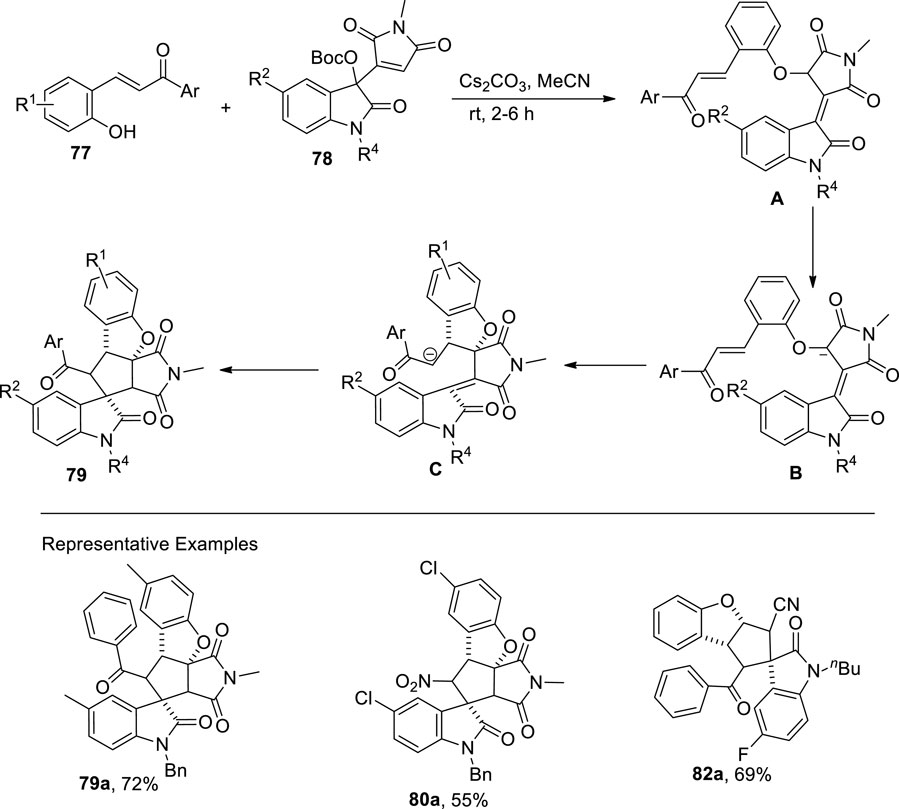
Figure 5. Proposed mechanism for the synthesis of the dihydrobenzofuran derivative 79 using a base-induced reaction.
Chen et al. (2021) proposed an efficient, metal-free cascade approach for constructing dihydrobenzofuran skeletons. In their novel approach, an ortho-allyloxy benzenediazonium salt reacted with thiophenols in the presence of DABCO (1,4-diazabicyclo [2.2.2]octane), which was used as an additive and an organic base, to afford desired heterocyclic products in moderate to high yields (63%–83%). The DABCO-catalyzed thiolate anion interacted with diazonium salt to generate diazo sulfide B, followed by thermal homolysis to give sulfenyl radical C and intermediate D. The intermediate D then proceeded through intramolecular 5-exo cyclization between the aromatic radical and the double bond, followed by reaction with sulfenyl radical C to furnish 2,3-disubstituted dihydrobenzofuran derivatives (Supplementary Scheme 1).
2.5 Catalyst-free synthesis of dihydrobenzofurans
Catalyst-free synthesis is a proficient and green approach that has been extensively applied in organic synthesis to avoid the use of expensive catalysts (Gawande et al., 2013). Das et al. (2022) subjected allyl-linked diazonium salts with substituted vinyl boronic acid and DABSO (1,4-diazabicyclo [2.2.2]octane bis(sulfur dioxide) adduct) under catalyst-free conditions utilizing KHCO3 in an optimized ratio of dimethylformamide and acetone (9:1) to afford sulfonylated dihydrobenzofuran derivatives in efficient yields (70%–80%) (Supplementary Scheme 2). Another example of catalyst-free synthesis was reported by Rao et al. (2023). They initially treated substituted salicylaldehydes with chloroacetonitrile in the presence of sodium carbonate and NaI in dimethyl formamide to obtain benzaldehyde derivatives. These derivatives were then reacted with substituted anilines in a catalyst-free approach to afford remarkable yields (80%–90%) of benzofuran derivatives (Supplementary Scheme 3).
Bisag et al. (2021) experimented with another catalyst-free protocol for the synthesis of 2,3-dihydrobenzofuran scaffolds. In their synthetic approach, substituted salicylaldehydes were reacted with sulfoxonium ylide in non-catalyst conditions to afford dihydrobenzofurans in high yields (80%–89%) in CH2Cl2. Sulfur ylide attacked the carbonyl carbon of substituted salicylaldehydes, ensuing a proton transfer reaction that resulted in the emergence of intermediate B. The intermediate B, upon dehydration, generated intermediate C. The desired products were finally obtained after a [4 + 2] cyclization reaction between ylide and intermediate C (Supplementary Scheme 4).
Another catalyst-free synthesis of substituted dihydrobenzofurans was developed by Zhou et al. (2022) recently via a [3 + 2] cyclization of unsaturated imines and iodine-substituted phenols in the presence of base (Supplementary Scheme 5). The reaction mechanism was assumed to move forward with the formation of intermediate A, which underwent C-I bond breakage followed by tautomerization and an addition reaction with iodine radical to give diradical intermediate D. The intermediate D then resulted in the synthesis of target molecules as a result of subsequent cyclization and hydrolysis. (Supplementary Scheme 5). Considering the deadly effects of cancer, researchers are continuously working to develop novel anti-cancer agents (Emami and Dadashpour, 2015; Irfan et al., 2022). The dihydrobenzofuran derivatives obtained via the above-mentioned protocol were utilized to synthesize anti-cancer agents and α-glucosidase inhibitors (Supplementary Figure 1).
2.6 Iodine-induced synthesis of dihydrobenzofurans
Iodine-mediated synthesis of organic molecules has earned significant weight owing to its economical nature and correspondence with green chemistry criteria (Samanta and Mondal, 2021; Banerjee et al., 2006). Cheng et al. (2023) envisioned an efficient, iodine-catalyzed protocol for generating 2,3-dihydrobenzofuran units. In their established protocol, chalcones and isobutyraldehyde were reacted in the presence of I2 (catalyst) to procure the desired dihydrobenzofuran motifs in good yield (67%). This synthetic pathway proceeds at ambient reaction conditions and covers a diverse range of functional groups (Supplementary Scheme 6).
Chen et al. (2023) disclosed a highly efficacious and metal-free transformation for synthesizing 3-aryl-2,3-dihydrobenzofurans. In their established pathway, ortho-hydroxystilbenes reacted with alcohol via cyclization and the aryl migration (CAM) process in the presence of an iodine oxidant in ethanol. o-Hydroxystilbenes were activated and converted to an iodonium ion intermediate A in the presence of an oxidant (I2 or ICI), followed by the subsequent removal of HI and aryl migration in the acidic medium to generate intermediate D. The intermediate D was then subjected to alcohol to synthesize target molecules in low to excellent yields (19%–92%) (Supplementary Scheme 7).
Azevedo et al. (2023) developed an eco-friendly approach involving solvent-free synthesis of chalcogenyl-based dihydrobenzofuran derivatives. In their green methodology, substituted allylphenols were treated with diaryl diselenides under metal-free, microwave-irradiated neat conditions, utilizing catalytic iodine with DMSO as an oxidant. The resulting dihydrobenzofuran derivatives were then evaluated for MAO-B inhibitory potential. The MAO-B inhibitory activity of synthesized dihydrobenzofurans gave indication about their utilization against Alzheimer’s and Parkinson’s diseases (Supplementary Scheme 8). The suggested mechanism inferred the reaction of diselenides with iodine using microwave irradiation to form electrophilic species A. In the next step, A coordinated with allylphenols to create the chalcogeniranium intermediate B, which opened via phenoxide ion to result in the required dihydrobenzofurans (Supplementary Figure 2).
2.7 Electrocatalytic synthesis of dihydrobenzofurans
Electrocatalytic reactions have been observed to be feasible and environmentally benign synthetic pathways to accomplish the synthesis of several organic compounds (Hammerich and Speiser, 2015; Nikishin et al., 1988). Elinson et al. (2021) proposed the synthesis of benzofuran derivatives by employing an electrolysis-mediated one-pot reaction of N,N-dimethylbarbiturate cyclic diketone and substituted aryl aldehydes. The reaction mechanism was thought to involve deprotonation, Knoevenagel condensation, Michael addition, bromination, and cyclization to yield dihydrobenzofurans. Another electrolysis-mediated facile approach leading to the synthesis of benzofuran heterocycles, including methanol and sodium bromide in reaction media, was presented by Ryzhkov et al. (2021). This novel methodology involving the cyclization of benzylidenebarbiturates and cyclic diketones in selected optimal conditions gave high yields of target molecules (72%–85%). As per the mechanistic details, a dienone anion (obtained by reaction with methoxide ion) was added to a cyclic diketone to give intermediate A, which was then subjected to treatment with bromine followed by exposure to methoxide ion, thereby resulting in intermediate C. The generated intermediate then underwent cyclization to afford corresponding dihydrobenzofuran derivatives (Supplementary Scheme 9; Figure 3).
2.8 Organocatalyzed synthesis of dihydrobenzofurans
Owing to their significant correspondence with green chemistry criteria, organocatalytic reactions have also flourished as efficient catalytic systems in organic transformations (Raj and Singh, 2009; Moyano and Rios, 2011). Xiang et al. (2021) proposed an efficient, organocatalyzed protocol for the synthesis of asymmetric dihydrobenzofurans via a Michael addition/hemiketalization reaction. In their novel methodology, substituted quinones were reacted with hydroxymaleimides in the presence of a chiral organocatalyst via a Michael addition followed by aromatization and hemiketalization to synthesize target molecules in moderate to excellent yields (68%–97%) with high enantiomeric (63:37-95:5 er) and diastereomeric ratios (>99:1 dr) (Supplementary Scheme 10).
Quinine-derived bifunctional squaramides were used by Susam et al. (2022) as active organocatalysts for the efficient synthesis of stereoselective dihydrobenzofurans and dihydronaphthofuran derivatives. In their synthetic approach, they used domino-type Friedel–Crafts/SN2 reactions to achieve the desired products in low to excellent yields (33%–95%) with remarkable enantioselectivity values (>99% ee). (Z)-α-Bromonitroalkenes were made to react with β-naphthols, α-naphthols, and substituted phenols in the presence of DABCO (used as a base) to achieve dihydronaphthofurans and dihydrobenzofurans, respectively (Supplementary Scheme 11).
2.9 Synthesis of dihydrobenzofurans by utilizing miscellaneous strategies
Asymmetric synthesis has been crucial in organic synthesis as various advanced and biologically important materials have chiral functionality (Taggi et al., 2003; Tsuji et al., 2018; Zbieg et al., 2012). N-Phosphinyl and N-phosphonyl imine acted as group-assisted purification (GAP) reagents for asymmetric synthesis (An et al., 2015). These GAP reagents have been used to prepare crystalline and solid products instead of oily and semi-solid products to avoid impurities. Rouh et al. (2022) reported the asymmetric synthesis of functionalized dihydrobenzofuran derivatives via an aggregate-based system. In their novel methodology, GAP reagents, that is, N-phosphonyl imines, were used to achieve the desired products in good to high yields (59%–70%) with excellent purity and diastereoselectivity values (77:23 S/R). Salicyl N-phosphonyl imines and dialkyl bromomalonates were reacted in different cosolvent ratios (THF:EtOH) and K3PO4 to furnish dihdrobenzofuran derivatives. When the ratios of these cosolvents were changed, the diastereomeric ratios of the products also changed. It was observed that with the increased amounts of ethanol in the THF:EtOH aggregate system, a high yield of the (R)- isomer was obtained over the (S)-isomer, and there was a reversal of results when iPr was used instead of EtOH. The formation of these aggregates of asymmetric starting material was measured by aggregation-induced polarization (an analytical technique) (Supplementary Scheme 12).
Scherübl et al. (2021) described the TEMPO (2,2,6,6-tetramethyl piperidine 1-oxyl)-promoted reaction of m-allyloxy aryl triflates (benzyne precursors) to synthesize dihydrobenzofuran derivatives by using cesium fluoride and 18-crwon-6-ether in n-hexane. TEMPO (acting as a stable radical) was added to o-substituted benzynes, thereby acting as a radical acceptor to generate aryl radicals. The generated radicals underwent 5-exo/6-endo cyclization with subsequent TEMPO-trapping to afford desired cyclic products in low to moderate yields (30%–62%) (Supplementary Scheme 13).
Roldan et al. (2023) demonstrated the influence of persistent radicals to avail the dihydrobenzofuran derivatives. For this purpose, they reacted persistent quinone methide radicals with phenols to afford transient phenoxy radicals. The transient and persistent radicals then underwent a cross-coupling reaction to result in pendant phenols and quinone methides, whose cyclization then furnished the synthesis of trans-2,3-diaryldihydrobenzofurans. The mechanism of this reaction was supposed to move forward by C–C bond formation, tautomerization, and cyclization to yield target molecules in efficient yields. This radical involving methodology provides an efficient pathway toward synthesizing naturally occurring stilbenoid, that is, resveratrol (Supplementary Scheme 14).
A metal-free synthetic route toward the procurement of dihydrobenzofuran derivatives was reported by Liu et al. (2022). They treated alkenyl-substituted phenols with sodium halides using K2S2O8 (as a promoter) in acetonitrile to obtain dihydrobenzofurans (Supplementary Scheme 15).
Another stereoselective approach to furnish dihydrobenzofurans was put forward by Kumari et al. (2023). In their stereoselective synthetic pathway, α-bromoketones were treated with pyridine-substituted Merrifield resin (promoter) in acetonitrile to generate pyridinium ylide A. The pyridinium ylides were then subjected to react with substituted o-hydroxychalcones via a [4 + 1] cyclization reaction using triethylamine as a base. As a result, chiral dihydrobenzofurans were attained in good to excellent yields (70%–92%). The formal [4 + 1] cyclization reaction was proposed to proceed via the conjugate addition of pyridinium ylide to substituted hydroxy chalcones followed by the attack of the nucleophilic oxygen to construct a carbon-oxygen bond, thus eliminating pyridine to yield the target molecules (Supplementary Scheme 16).
Röser et al. (2022) proposed a protocol for the synthesis of dihydrobenzofuran derivatives from β-ketolactone via the decarboxylation-cycloetherification method. The synthesis was accomplished by using a catalytic amount of quaternary ammonium iodides along with H2O2 (oxidant) in CH2Cl2 (solvent). This synthetic methodology covers a broad spectrum of substrates with good to excellent yields (57%–93%) under ambient reaction conditions. Cycloetherification is done in situ by the formation of ammonium hypoiodite from quaternary ammonium iodides. This synthesis was carried out by using β-ketolactone, affording a phenolic intermediate A that underwent oxidative cycloetherification to generate an intermediate B, which, upon further cyclization, resulted in the desired dihydrobenzofurans (Supplementary Scheme 17).
Lacuna et al. (2022) reported the utilization of the Pauson–Khand reaction by carrying out the cyclization of alkynylphenyl vinyl ethers. This less-time-consuming synthetic protocol resulted in efficient synthesis of numerous benzofuran derivatives in isolated yields (Supplementary Scheme 18). Kamitanaka et al. (2021) established the tetrabutylammonium triflate-catalyzed preparation of 2-oxygenated dihydrobenzofuran derivatives via [3 + 2] coupling. In their synthetic approach, quinone monoacetals (1.0 equiv.) reacted with vinyl ethers (2.0 equiv.) in HFIP utilizing nBu4NOTf (0.2 equiv.) to furnish dihydrobenzofurans in moderate to excellent yield range (45%–99%). Quinone monoacetals interacted with HFIP to form a charged intermediate A, followed by alkoxy group elimination and reaction with vinyl ethers at the α-carbon of the carbonyl group to generate intermediate B. This intermediate B then underwent aromatization to afford 2,3-dihydrobenzofurans (Supplementary Scheme 19).
Hellwig et al. (2021) used a versatile approach for the synthesis of 2,3-dihydrobenzofuran selenides. In their synthetic methodology, diorganyl dichalcogenides and 2-allylphenols were reacted in the presence of potassium peroxymonosulfate (Oxone®) as an effective oxidizing agent in MeCN. The proposed mechanistic insights unveiled that the alkyl diselenides were made to interact with Oxone® to form electrophilic selenium species A, which further reacted with 2-allylphenols to generate seleniranium intermediate B. The intermediate B then underwent intramolecular oxyselenocyclization and deprotonation to afford the desired 2,3-dihydrobenzofuran with a moderate to high yield range (45%–85%) (Supplementary Scheme 20).
3 Conclusion
In summary, we have outlined novel transition metal-free synthetic strategies with mechanistic insights to achieve the construction of the dihydrobenzofuran nucleus, reported within the last 3 years (2021–2023). With respect to this, Bronsted acid-mediated, Lewis acid-induced, photocatalyzed, base-catalyzed, iodine-promoted, electrocatalytic, and organocatalyzed synthetic transformations have been discussed. In addition to the utilization of earlier mentioned proficient catalysts, catalyst-free synthesis of dihydrobenzofurans has huge implications. These methodical approaches involve various annulation, insertion, and cycloaddition reactions, that is, [3 + 2] annulation, [4 + 1] annulation, [4 + 4] annulation, [4 + 1] cycloaddition, C-H insertion, 5-exo-trig cyclization, and condensation reactions to carry out the synthesis of these biologically active heterocyclic scaffolds. We envisage that our review will urge the researchers to escalate these developments to acquire dihydrobenzofuran derivatives, thereby gauging their role in medicinal chemistry.
Author contributions
AqM: data curation, investigation, and writing–original draft. MI: data curation, resources, and writing–review and editing. AH: data curation, formal analysis, and writing–review and editing. AsM: formal analysis, writing–review and editing, and resources. SK: data curation, resources, and writing–review and editing. AFZ: conceptualization, resources, supervision, and writing–review and editing. BP: data curation, formal analysis, resources, and writing–review and editing. AI: data curation, methodology, resources, and writing–review and editing. KK-M: funding acquisition, resources, software, and writing–review and editing. MG: formal analysis, funding acquisition, investigation, and writing–review and editing. MM: funding acquisition, project administration, resources, and writing–review and editing.
Funding
The author(s) declare that financial support was received for the research, authorship, and/or publication of this article. The research was partly financed by research projects: Collegium Medicum, the Mazovian Academy in Plock, and the Medical University of Lublin (DS 730).
Conflict of interest
The authors declare that the research was conducted in the absence of any commercial or financial relationships that could be construed as a potential conflict of interest.
Publisher’s note
All claims expressed in this article are solely those of the authors and do not necessarily represent those of their affiliated organizations, or those of the publisher, the editors, and the reviewers. Any product that may be evaluated in this article, or claim that may be made by its manufacturer, is not guaranteed or endorsed by the publisher.
Supplementary material
The Supplementary Material for this article can be found online at: https://www.frontiersin.org/articles/10.3389/fchem.2024.1470861/full#supplementary-material
References
Ahmad, S., Zahoor, A. F., Naqvi, S. A. R., and Akash, M. (2018). Recent trends in ring opening of epoxides with sulfur nucleophiles. Mol. Div. 22, 191–205. doi:10.1007/s11030-017-9796-x
Alexander, H. (1892). Reduction des Cumarons. Ber. Dtsch. Chem. 25, 2409–2411. doi:10.1002/cber.18920250236
am Ende, C. W., Zhou, Z., and Parker, K. A. (2013). Total synthesis of (±)-Bisabosqual A. J. Am. Chem. Soc. 135, 582–585. doi:10.1021/ja3108577
An, G., Seifert, C., and Li, G. (2015). N-Phosphonyl/phosphinyl imines and group-assisted purification (GAP) chemistry/technology. Org. Biomol. Chem. 13, 1600–1617. doi:10.1039/c4ob02254h
Ashraf, R., Zahoor, A. F., Ali, K. G., Nazeer, U., Saif, M. J., Mansha, A., et al. (2024). Development of novel transition metal-catalyzed synthetic approaches for the synthesis of a dihydrobenzofuran nucleus: a review. RSC Adv. 14, 14539–14581. doi:10.1039/d4ra01830c
Azevedo, A. R., Cordeiro, P., Strelow, D. N., de Andrade, K. N., Neto, M. R., Fiorot, R. G., et al. (2023). Green approach for the synthesis of chalcogenyl- 2,3-dihydrobenzofuran derivatives through allyl-phenols/naphthols and their potential as MAO-B inhibitors. Chem. Asian J. 18, e202300586. doi:10.1002/asia.202300586
Bai, Y., Chen, J., and Zimmerman, S. C. (2018). Designed transition metal catalysts for intracellular organic synthesis. Chem. Soc. Rev. 47, 1811–1821. doi:10.1039/c7cs00447h
Banerjee, A. K., Vera, W., Mora, H., Laya, M. S., Bedoya, L., and Cabrera, E. V. (2006). Iodine in organic synthesis. Available at: https://nopr.niscpr.res.in/handle/123456789/4831.
Bertolini, F., and Pineschi, M. (2009). Recent progress in the synthesis of 2,3-dihydrobenzofurans. Org. Prep. Proced. Int. 41, 385–418. doi:10.1080/00304940903240836
Bisag, G. D., Ruggieri, S., Fochi, M., and Bernardi, L. (2021). Catalyst- and substrate-dependent chemodivergent reactivity of stabilised sulfur ylides with salicylaldehydes. L. Adv. Synth. Catal. 363, 3053–3059. doi:10.1002/adsc.202100124
Breijyeh, Z., and Karaman, R. (2023). Design and synthesis of novel antimicrobial agents. Antibiotics 12, 628. doi:10.3390/antibiotics12030628
Caiuby, C. A. D., Ali, A., Santana, V. T., Lucas, F. W. D. S., Santos, M. S., Corrêa, A. G., et al. (2018). Intramolecular radical cyclization approach to access highly substituted indolines and 2,3-dihydrobenzofurans under visible-light. RSC Adv. 8, 12879–12886. doi:10.1039/c8ra01787e
Chen, C. Y., and Weisel, M. (2013). Concise asymmetric synthesis of (+)-Conocarpan and obtusafuran. Synlett 24, 189–192. doi:10.1055/s-0032-1317704
Chen, M., Huang, Z. T., and Zheng, Q. Y. (2012). Visible light-induced 3-sulfenylation of N-methylindoles with arylsulfonyl chlorides. Chem. Commun. 48, 11686–11688. doi:10.1039/c2cc36866h
Chen, T., Zheng, R., and Yu, J. (2021). An efficient approach to 3-thioether-functionalized 2,3-dihydrobenzofurans via a metal-free intramolecular radical cyclization/thiolation cascade reaction. Synth. Commun. 51, 2204–2212. doi:10.1080/00397911.2021.1927098
Chen, X. M., Xie, K. X., Yue, D. F., Zhang, X. M., Xu, X. Y., and Yuan, W. C. (2018). Catalyst-free synthesis of 2,3-dihydrobenzofurans through [4+1] cycloaddition of ortho -hydroxyphenylsubstituted para-quinone methides and sulfur ylides. Tetrahedron 74, 600–605. doi:10.1016/j.tet.2017.12.038
Chen, Y., Fan, Y., Li, Y., and Yao, C. (2023b). Iodonium ion-induced cyclization and aryl migration of ortho-hydroxystilbenes for the synthesis of 3-Aryl-2,3-dihydrobenzofuran. J. Org. Chem. 88, 11460–11472. doi:10.1021/acs.joc.3c00440
Chen, Z., Huang, W., Su, Y., Jiang, H., and Wu, W. (2023a). Lewis/Brønsted acid-mediated cyclization/amidation of 1,6-enynes with nitriles: access to 3-enamide substituted dihydrobenzofurans. Chem. Commun. 59, 4523–4526. doi:10.1039/d3cc00787a
Chen, Z., Pitchakuntla, M., and Jia, Y. (2019). Synthetic approaches to natural products containing 2,3-dihydrobenzofuran skeleton. Nat. Prod. Rep. 36, 666–690. doi:10.1039/c8np00072g
Cheng, D., Meng, X., Li, D., Jie, S., Liu, Y., and Jiao, X. (2023). Iodine-catalyzed simple and efficient synthesis of 1, 3, 5-triarylbenzenes and 2, 3-dihydrobenzofuran derivatives under mild reaction conditions. Synlett. 34, 1925–1929. doi:10.1055/s-0041-1738442
Corti, V., Dosso, J., Prato, M., and Filippini, G. (2023). Photoinduced cascade reactions of 2-allylphenol derivatives toward the production of 2,3-dihydrobenzofurans. J. Org. Chem. 88, 6008–6016. doi:10.1021/acs.joc.3c00347
Dapkekar, A. B., Sreenivasulu, C., Ravi Kishore, D., and Satyanarayana, G. (2022). Recent advances towards the synthesis of dihydrobenzofurans and dihydroisobenzofurans. Asian J. Org. Chem. 11, e202200012. doi:10.1002/ajoc.202200012
Das, A., Ajarul, S., Debnath, S., Hota, P., and Maiti, D. K. (2023). Bro̷nsted acid-catalyzed [5+1] and [4+1] annulation of cyclic anhydrides with o-alkynylanilines to construct fused-N-heterocycles. J. Org. Chem. 88, 15073–15084. doi:10.1021/acs.joc.3c01525
Das, P., Das, S., and Jana, R. (2022). Aryldiazonium salts and DABSO: a versatile combination for three-component sulfonylative cross-coupling reactions. Chem. Asian J. 17, e202200085. doi:10.1002/asia.202200085
Delost, M. D., Smith, D. T., Anderson, B. J., and Njardarson, J. T. (2018). From oxiranes to oligomers: architectures of U.S. FDA approved pharmaceuticals containing oxygen heterocycles. J. Med. Chem. 61, 10996–11020. doi:10.1021/acs.jmedchem.8b00876
Ding, M., Ou, P., Li, X., Yu, Y., Niu, M., Yang, Y., et al. (2023). Alkyne insertion enabled vinyl to acyl 1,5-palladium migration: rapid access to substituted 5-membered-dihydrobenzofurans and indolines. Angew. Chem. Int. Ed. 62, e202300703. doi:10.1002/anie.202300703
Elinson, M. N., Ryzhkova, Y. E., Vereshchagin, A. N., Ryzhkov, F. V., and Egorov, M. P. (2021). Electrocatalytic multicomponent one-pot approach to tetrahydro-2′H,H-spiro[benzofuran-2,5′-pyrimidine] scaffold. J. Heterocycl. Chem. 58, 1484–1495. doi:10.1002/jhet.4274
Emami, S., and Dadashpour, S. (2015). Current developments of coumarin-based anti-cancer agents in medicinal chemistry. Eur. J. Med. Chem. 102, 611–630. doi:10.1016/j.ejmech.2015.08.033
Fadeev, A. A., Makarov, A. S., Ivanova, O. A., Uchuskin, M. G., and Trushkov, I. V. (2022). Extended Corey–Chaykovsky reactions: transformation of 2-hydroxychalcones to benzannulated 2,8-dioxabicyclo[3.2.1]octanes and 2,3-dihydrobenzofurans. Org. Chem. Front. 9, 737–744. doi:10.1039/d1qo01646f
Faiz, S., Yousaf, M., Zahoor, A. F., Naqvi, S. A. R., Irfan, A., and Zaman, G. (2017). Synthetic strategies toward the synthesis of polyphenolic natural products: pauciflorol F and isopaucifloral F: a review. Synth. Commun. 47, 1121–1135. doi:10.1080/00397911.2017.1303510
Faiz, S., and Zahoor, A. F. (2016). Ring opening of epoxides with C-nucleophiles. Mol. Div. 20, 969–987. doi:10.1007/s11030-016-9686-7
Fang, Z., Zhang, Y., Guo, Y., Jin, Q., Zhu, H., Xiu, H., et al. (2022). The [4+1] cyclization reaction of 2-hydroxylimides and trimethylsulfoxonium iodide for the synthesis of 3-amino-2,3-dihydrobenzofurans. New J. Chem. 46, 18124–18127. doi:10.1039/d2nj03131k
Farooq, U., Naz, S., Shams, A., Raza, Y., Ahmed, A., Rashid, U., et al. (2019). Isolation of dihydrobenzofuran derivatives from ethnomedicinal species Polygonum barbatum as anticancer compounds. Biol. Res. 52, 1–12. doi:10.1186/s40659-018-0209-0
Gawande, M. B., Bonifácio, V. D., Luque, R., Branco, P. S., and Varma, R. S. (2013). Benign by design: catalyst-free in-water, on-water green chemical methodologies in organic synthesis. Chem. Soc. Rev. 42, 5522–5551. doi:10.1039/c3cs60025d
Gowda, P. S., Sharada, D. S., and Satyanarayana, G. (2023). A TBADT photocatalyst-enabled radical-induced cyclization pathway to access functionalized dihydrobenzofurans. Chem. Commun. 59, 9094–9097. doi:10.1039/d3cc02340k
Grishin, I. Y., Arutiunov, N. A., Aksenov, D. A., Aksenov, N. A., Aksenov, A. V., Gasanova, A. Z., et al. (2022). Improved method for preparation of 3-(1H-Indol-3-yl)benzofuran-2(3H)-ones. C. Mol. 27, 1092. doi:10.3390/molecules27061902
Guan, C. Y., Han, T. J., Jia, S. K., Hua, Y. Z., and Mei, G. J. (2023). Diastereodivergent formal [4 + 1] cycloaddition of azoalkenes as one-carbon synthons. Green Synth. Catal. 4, 258–262. doi:10.1016/j.gresc.2022.05.003
Guo, J., and Jiang, D. (2020). Covalent organic frameworks for heterogeneous catalysis: principle, current status, and challenges. ACS Cent. Sci. 6, 869–879. doi:10.1021/acscentsci.0c00463
Guo, L., Chen, G., Li, H., Tung, C. H., and Wang, Y. (2023). Visible-light-driven [3 + 2] cyclization of phenols with indoles and olefins using recyclable Ag3PO4 nanoparticles. Green Chem. 25, 7102–7108. doi:10.1039/d3gc02418k
Hammerich, O., and Speiser, B. (2015). 2 Techniques for Studies. Organic Electrochemistry: Revised and Expanded (Boca Raton, FL: CRC Press), 97.
Hellwig, P. S., Barcellos, A. M., Furst, C. G., Alberto, E. E., and Perin, G. (2021). Oxyselenocyclization of 2-allylphenols for the synthesis of 2,3-dihydrobenzofuran selenides. ChemistrySelect 6, 13884–13889. doi:10.1002/slct.202104072
Heravi, M., Asadi, M., Nazari, S., and Lashkariani, N. M. (2016). Boshra. Curr. Org. Synth. 13, 308–333. doi:10.2174/1570179412666150710182304
Huang, W., Wang, H., Liu, B., Shen, R., and Zhu, S. (2022). Synthesis of 1,1,4,5-tetrasubstituted phthalans via Pd-catalyzed three-component reactions of haloarenes, alkynes, and protic nucleophiles. Org. Lett. 24, 8651–8656. doi:10.1021/acs.orglett.2c03460
Irfan, A., Faisal, S., Ahmad, S., Al-Hussain, S. A., Javed, S., Zahoor, A. F., et al. (2023b). Structure-based virtual screening of furan-1,3,4-oxadiazole tethered N-phenylacetamide derivatives as novel class of hTYR and hTYRP1 inhibitors. Pharmaceuticals 16, 344. doi:10.3390/ph16030344
Irfan, A., Faisal, S., Zahoor, A. F., Noreen, R., Al-Hussain, S. A., Tuzun, B., et al. (2023a). In silico development of novel benzofuran-1,3,4-oxadiazoles as lead inhibitors of M. tuberculosis polyketide synthase 13. Pharmaceuticals 16, 829. doi:10.3390/ph16060829
Irfan, A., Faiz, S., Rasul, A., Zafar, R., Zahoor, A. F., KotwicaMojzych, K., et al. (2022). Exploring the synergistic anticancer potential of benzofuran–oxadiazoles and triazoles: improved ultrasound- and microwave-assisted synthesis, molecular docking, hemolytic, thrombolytic and anticancer evaluation of furan-based molecules. Molecules 27, 1023. doi:10.3390/molecules27031023
Jabeen, S., Ghosh, G., Lapoot, L., Durantini, A. M., and Greer, A. (2023). Sensitized photooxidation of ortho-prenyl phenol: biomimetic dihydrobenzofuran synthesis and total 1 quenching†. J. Photochem. Photobiol. 99, 637–641. doi:10.1111/php.13689
Jacob, A., Roy, T., Kaicharla, T., and Biju, A. T. (2017). Metal-free, brønsted acid-catalyzed formal [3+2] annulation of quinone monoacetals with 2-naphthols. J. Org. Chem. 82, 11269–11274. doi:10.1021/acs.joc.7b02033
Jiang, J., Liu, J., Yang, Z., Zheng, L., and Liu, Z. Q. (2022). Three-component synthesis of benzofuran-3(2H)-ones with tetrasubstituted carbon stereocenters via Rh(III)-Catalyzed C−H/C−C bond activation and cascade annulation. Adv. Synth. Catal. 364, 2540–2545. doi:10.1002/adsc.202200396
Jin, Y., Fan, P., and Wang, C. (2022). Nickel-catalyzed reductive asymmetric aryl-acylation and aryl-carbamoylation of unactivated alkenes. CCS Chem. 4, 1510–1518. doi:10.31635/ccschem.021.202101040
Kamitanaka, T., Tsunoda, Y., Fujita, Y., Dohi, T., and Kita, Y. (2021). [3 + 2] coupling of quinone monoacetals with vinyl ethers effected by tetrabutylammonium triflate: regiocontrolled synthesis of 2-oxygenated dihydrobenzofurans. Org. Lett. 23, 9025–9029. doi:10.1021/acs.orglett.1c02792
Kang, Y., Lu, J. L., Zhang, Z., Liang, Y. K., Ma, A. J., and Peng, J. B. (2022). Palladium-catalyzed intramolecular heck/aminocarbonylation of alkene-tethered iodobenzenes with nitro compounds: synthesis of carbamoyl-substituted benzoheterocycles. J. Org. Chem. 88, 5097–5107. doi:10.1021/acs.joc.2c01253
Kärkäs, M. D. (2018). Electrochemical strategies for C–H functionalization and C–N bond formation. Chem. Soc. Rev. 47, 5786–5865. doi:10.1039/c7cs00619e
Khanam, H. (2015). Bioactive Benzofuran derivatives: a review. Eur. J. Med. Chem. 97, 483–504. doi:10.1016/j.ejmech.2014.11.039
Kobayashi, S., and Jørgensen, K. A. (2002). Cycloaddition reactions in organic synthesis. John Wiley & Sons.
Kumari, A., Jain, A., Shukla, K., Patra, R., and Rana, N. K. (2023). A reusable polymer anchored pyridine mediated formal [4 + 1] annulation reaction for the diastereoselective synthesis of 2,3-dihydrobenzofurans. Org. Biomol. Chem. 21, 5542–5546. doi:10.1039/d3ob00804e
Kusaka, S., Ohmura, T., and Suginome, M. (2022). Iridium-catalyzed enantioselective intramolecular cross-dehydrogenative coupling of alkyl aryl ethers giving enantioenriched 2,3-dihydrobenzofurans. Chem. Lett. 51, 601–604. doi:10.1246/cl.220129
Lacuna, J. G.-, Alonso, M., Domínguez, G., and Castells, J. P. (2022). Study of the Pauson–Khand reaction in flow over alkynylphenyl vinyl ethers: towards the synthesis of tricyclic multisubstituted benzofurans. RSC Adv. 12, 7313–7317. doi:10.1039/d2ra01062c
Laurita, T., D’Orsi, R., Chiummiento, L., Funicello, M., and Lupattelli, P. (2020). Recent advances in synthetic strategies to 2,3-dihydrobenzofurans. Synthesis 52, 1451–1477. doi:10.1055/s-0039-1690820
Li, H. P., Wu, X. L., Zhan, G., Fu, X. J., Chen, J. H., He, X. H., et al. (2023a). Construction of cyclopenta[b]dihydronaphthofurans via TsOH-catalyzed consecutive biscyclization of dithioallylic alcohols and 1-styrylnaphthols. Chem. Commun. 59, 2275–2278. doi:10.1039/d2cc06324g
Li, L., Li, J. Z., Sun, Y. B., Luo, C. M., Qiu, H., Tang, K., et al. (2022). Visible-light-catalyzed tandem radical addition/1,5-hydrogen atom transfer/cyclization of 2-alkynylarylethers with sulfonyl chlorides. Org. Lett. 24, 4704–4709. doi:10.1021/acs.orglett.2c01977
Li, Y., Wang, F., Ning, R., Kong, D., and Wu, M. (2023b). Formal [4+1] annulations of salicylaldehydes with 3-chlorooxindoles for diastereoselective construction of dihydrobenzofuranspirooxindoles by solvent-free grinding. J. Heterocycl. Chem. 60, 1809–1814. doi:10.1002/jhet.4674
Liu, R., Wang, Y., Hu, Z., Wu, J., Gong, C., Jiang, Z., et al. (2022). K2S2O8-Promoted haloamination of non-functionalized olefins for the synthesis of 2-halomethyl indolines and 2-halomethyl dihydrobenzofuran. ChemistrySelect 7, e202201932. doi:10.1002/slct.202201932
Lu, Z., Zhang, Q., Ke, M., Hu, S., Xiao, X., and Chen, F. (2021). TfOH-catalyzed [4 + 1] annulation of p-quinone methides with α-aryl diazoacetates: straightforward access to highly functionalized 2,3-dihydrobenzofurans. J. Org. Chem. 86, 7625–7635. doi:10.1021/acs.joc.1c00672
Ma, D., Wang, Y., Liu, A., Li, S., Lu, C., and Chen, C. (2018). Covalent organic frameworks: promising materials as heterogeneous catalysts for C-C bond formations. Catalysts 8, 404. doi:10.3390/catal8090404
Macías, F. A., Varela, R. M., Torres, A., and Molinillo, J. M. (1999). New bioactive plant heliannuols from cultivar sunflower leaves. J. Nat. Prod. 62, 1636–1639. doi:10.1021/np990249y
Maejima, S., Yamaguchi, E., and Itoh, A. (2023). Visible-light-induced regioselective functionalization of α-olefin: development of one-pot photo-synthesis of C3-substituted dihydrobenzofurans. Org. Lett. 25, 1856–1861. doi:10.1021/acs.orglett.3c00335
Medishetti, N., Ittamalla, C., Kale, A., Nanubolu, J. B., and Atmakur, K. (2021). Base-promoted synthetic transformation of 4H-chromenes into dihydrobenzofurans and bispyrazolospirocyclopropanes via intramolecular cyclization. ChemistrySelect 6, 9867–9872. doi:10.1002/slct.202102655
Meloche, J. L., and Ashfeld, B. L. (2017). A rhodium(II)-Catalyzed formal [4+1]-Cycloaddition toward spirooxindole pyrrolone construction employing vinyl isocyanates as 1,4dipoles. Angew. Chem. Int. Ed. 56, 6604–6608. doi:10.1002/anie.201701147
Mimaki, Y., Kameyama, A., Sashida, Y., Miyata, Y., and Fuji, A. (1995). A novel hexahydrodibenzofuran derivative with potent inhibitory activity on melanin biosynthesis of cultured B-16 melanoma cells from Lindera umbellata bark. Chem. Pharm. Bull. 43, 893–895. doi:10.1248/cpb.43.893
Minagawa, K., Kouzuki, S., Nomura, K., Yamaguchi, T., Kawamura, Y., Matsushima, K., et al. (2001). Bisabosquals, novel squalene synthase inhibitors. I. Taxonomy, fermentation, isolation and biological activities. J. Antibiot. 54, 890–895. doi:10.7164/antibiotics.54.890
Morita, N., Chiaki, H., Tanaka, K., Hashimoto, Y., Tamura, O., and Krause, N. (2023). Sustainable chemical synthesis of 2,3-dihydrobenzofurans/1,2,3-trisubstituted indanes in water by using a permethylated β-cyclodextrin-tagged N-heterocyclic carbene–gold catalyst. Synlett 34, 1425–1432. doi:10.1055/a-2016-6577
Moyano, A., and Rios, R. (2011). Asymmetric organocatalytic cyclization and cycloaddition reactions. Chem. Rev. 111, 4703–4832. doi:10.1021/cr100348t
Mueller, J. O., Schmidt, F. G., Blinco, J. P., and Barner-Kowollik, C. (2015). Visible-light-induced click chemistry. Angew. Chem. Int. Ed. 54, 10284–10288. doi:10.1002/anie.201504716
Munawar, S., Zahoor, A. F., Ali, S., Javed, S., Irfan, M., Irfan, A., et al. (2022). Mitsunobu reaction: a powerful tool for the synthesis of natural products: a review. Molecules 27, 6953. doi:10.3390/molecules27206953
Naik, R., Harmalkar, D. S., Xu, X., Jang, K., and Lee, K. (2015). Bioactive benzofuran derivatives: moracins A–Z in medicinal chemistry. Eur. J. Med. Chem. 90, 379–393. doi:10.1016/j.ejmech.2014.11.047
Nair, D., Basu, P., Pati, S., Baseshankar, K., Sankara, C. S., and Namboothiri, I. N. (2023). Synthesis of spirolactones and functionalized benzofurans via addition of 3-sulfonylphthalides to 2-formylaryl triflates and conversion to benzofuroisocoumarins. J. Org. Chem. 88, 4519–4527. doi:10.1021/acs.joc.2c03097
Nakamura, A., Imamiya, A., Ikegami, Y., Rao, F., Yuguchi, H., Miki, Y., et al. (2022). Selective synthesis of 3-formylbenzofuran and 3-acylbenzofuran using a chalcone rearrangement strategy. RSC Adv. 12, 30426–30431. doi:10.1039/d2ra06080a
Nikishin, G. I., Elinson, M. N., and Makhova, I. V. (1988). Electrocatalytic haloform reaction: transformation of methyl ketones into methyl esters. Angew. Chem. Int. Ed. Eng. 27, 1716–1717. doi:10.1002/anie.198817161
O'Callaghan, K. S., Lynch, D., Baumann, M., Collins, S. G., and Maguire, A. R. (2023). Flow photolysis of aryldiazoacetates leading to dihydrobenzofurans via intramolecular C–H insertion. Org. Biomol. Chem. 21, 4770–4780. doi:10.1039/D3OB00541K
Pannilawithana, N., Pudasaini, B., Baik, M. H., and Yi, C. S. (2021). Experimental and Computational studies on the ruthenium-catalyzed dehydrative C–H coupling of phenols with aldehydes for the synthesis of 2-alkylphenol, benzofuran, and xanthene derivatives. J. Am. Chem. Soc. 143, 13428–13440. doi:10.1021/jacs.1c06887
Parvatkar, P. T., Kandambeth, S., Shaikh, A. C., Nadinov, I., Yin, J., Kale, V. S., et al. (2023). A tailored COF for visible-light photosynthesis of 2,3-dihydrobenzofurans. J. Am. Chem. Soc. 145, 5074–5082. doi:10.1021/jacs.2c10471
Pieters, L., Van Dyck, S., Gao, M., Bai, R., Hamel, E., Vlietinck, A., et al. (1999). Synthesis and biological evaluation of dihydrobenzofuran lignans and related compounds as potential antitumor agents that inhibit tubulin polymerization. J. Med. Chem. 42, 5475–5481. doi:10.1021/jm990251m
Raj, M., and Singh, V. K. (2009). Organocatalytic reactions in water. Chem. Commun. 44, 6687–6703. doi:10.1039/b910861k
Rao, M., Yang, M., Kuehner, D., Grosso, J., and Deshpande, R. P. (2003). A practical pilot-scale synthesis of 4-Vinyl-2,3-dihydrobenzofuran using imidate ester chemistry and phase-transfer catalysis. Org. Proce. Res. Dev. 7, 547–550. doi:10.1021/op030201y
Rao, M. L., and Islam, S. S. (2021). Copper-catalyzed synthesis of 1-(2-benzofuryl)-N-heteroarenes from o-hydroxy-gem-(dibromovinyl)benzenes and N-heteroarenes. Org. Biomol. Chem. 19, 9076–9080. doi:10.1039/d1ob01765a
Rao, P. N., Reddy, B. S., Sudhakar, K., Kumar, K. N., and Kumar, U. S. (2023). A concise and metal catalyst free synthesis of 2,3-disubstituted dihydrobenzofuran-3-amines. Tetrahedron Lett. 119, 154431. doi:10.1016/j.tetlet.2023.154431
Roldan, B., Hammerstad, T., Galliher, M., Keylor, M., Pratt, D., and Stephenson, C. (2023). Leveraging the persistent radical effect in the synthesis of trans-2,3-diaryl-dihydrobenzofurans. Available at: https://chemrxiv.org/engage/chemrxiv/article-details/6448315ee4bbbe4bbf3eb18a.
Röser, K., Scheucher, A., Mairhofer, C., Bechmann, M., and Waser, M. (2022). Oxidative decarboxylative ammonium hypoiodite-catalysed dihydrobenzofuran synthesis. Org. Biomol. Chem. 20, 3273–3276. doi:10.1039/d2ob00463a
Rouh, H., Tang, Y., Xu, T., Yuan, Q., Zhang, S., Wang, J. Y., et al. (2022). Aggregation-induced synthesis (AIS): asymmetric synthesis via chiral aggregates. Research. doi:10.34133/2022/9865108
Ryzhkov, F. V., Elinson, M. N., Ryzhkova, Y. E., Vereshchagin, A. N., Fakhrutdinov, A. N., and Egorov, M. P. (2021). Electrocatalytic cascade approach to the synthesis of dihydro-2'H,3H-spiro[1-benzofuran-2,5'-pyrimidines]. Chem. Heterocycl. Compd. 57, 672–678. doi:10.1007/s10593-021-02966-8
Sachdeva, H., Khaturia, S., Saquib, M., Khatik, N., Khandelwal, A. R., Meena, R., et al. (2022). Oxygen- and sulphur-containing heterocyclic compounds as potential anticancer agents. Appl. Biochem. Biotechnol. 194, 6438–6467. doi:10.1007/s12010-022-04099-w
Samanta, S., and Mondal, S. (2021). Iodine-catalyzed or-mediated reactions in aqueous medium. Asian J. Org. Chem. 10, 2503–2520. doi:10.1002/ajoc.202100424
Sau, S., Mukherjee, K., Kondalarao, K., Gandon, V., and Sahoo, A. K. (2023). Probing chiral sulfoximine auxiliaries in Ru(II)-Catalyzed one-pot asymmetric C–H hydroarylation and annulations with alkynes. Org. Lett. 25, 7667–7672. doi:10.1021/acs.orglett.3c02969
Scherübl, M., Daniliuc, C. G., and Studer, A. (2021). Arynes as radical acceptors: TEMPO-mediated cascades comprising addition, cyclization, and trapping. Angew. Chem. Int. Ed. 60, 711–715. doi:10.1002/anie.202012654
Sharma, S., Parumala, S. K. R., and Peddinti, R. K. (2017). Lewis acid mediated [3+2] coupling of masked benzoquinones with styrenes: facile synthesis of 2,3-dihydrobenzofurans. Synlett 28, 239–244. doi:10.1055/s-0036-1588622
Shen, Q., Zheng, X., Li, L., Zhong, T., Yin, C., and Yu, C. (2022). Photoinduced three-component difluoroamidosulfonylation/bicyclization: a route to dihydrobenzofuran derivatives. Org. Lett. 24, 2556–2561. doi:10.1021/acs.orglett.2c00761
Sheppard, T. D. (2011). Strategies for the synthesis of 2,3-dihydrobenzofurans. J. Chem. Res. 35, 377–385. doi:10.3184/174751911x13096980701749
Snider, B. B., and Lobera, M. (2004). Synthesis of the tetracyclic core of the bisabosquals. Tetrahedron Lett. 45, 5015–5018. doi:10.1016/j.tetlet.2004.05.005
Sun, D., Zhao, Q., and Li, C. (2011). Total synthesis of (+)-Decursivine. Org. Lett. 13, 5302–5305. doi:10.1021/ol2021669
Sun, H., He, H., Ni, S. F., and Guo, W. (2023). Asymmetric (4+1) annulations by cascade allylation and transient σ-alkyl-Pd(II) initiated allylic Csp3−H activation. Angew. Chem. Inter. Ed. 62, e202315438. doi:10.1002/anie.202315438
Suneja, A., and Schneider, C. (2018). Phosphoric acid catalyzed [4 + 1]-cycloannulation reaction of ortho-quinone methides and diazoketones: catalytic, enantioselective access toward cis-2,3-dihydrobenzofurans. Org. Lett. 20, 7576–7580. doi:10.1021/acs.orglett.8b03311
Susam, Z. D., Özcan, B. D., Kurtkaya, E., Yildirim, E., and Tanyeli, C. (2022). Organocatalytic enantioselective synthesis of dihydronaphthofurans and dihydrobenzofurans: reaction development and insights into stereoselectivity. Org. Biomol. Chem. 20, 8725–8740. doi:10.1039/d2ob01571d
Taggi, A. E., Hafez, A. M., and Lectka, T. (2003). α-Imino Esters: versatile substrates for the catalytic, asymmetric synthesis of α- and β-amino acids and β-lactams. Acc. Chem. Res. 36, 10–19. doi:10.1021/ar020137p
Toure, B. B., and Hall, D. G. (2009). Natural product synthesis using multicomponent reaction strategies. Chem. Rev. 109, 4439–4486. doi:10.1021/cr800296p
Tsuji, N., Kennemur, J. L., Buyck, T., Lee, S., Prevost, S., Kaib, P. S. J., et al. (2018). Activation of olefins via asymmetric Brønsted acid catalysis. Science 359, 1501–1505. doi:10.1126/science.aaq0445
Varadaraju, T. G., and Hwu, J. R. (2012). Synthesis of anti-HIV lithospermic acid by two diverse strategies. Org. Biomol. Chem. 10, 5456–5465. doi:10.1039/c2ob25575h
Vellakkaran, M., and Hong, S. (2021). Visible-light-induced reactions driven by photochemical activity of quinolinone and coumarin scaffolds. Asian J. Org. Chem. 10, 1012–1023. doi:10.1002/ajoc.202100162
Wang, D., Sun, J., Han, Y., Sun, Q., and Yan, C. G. (2022). An access to highly functionalized dihydrobenzofuran spirooxindole scaffolds. Org. Lett. 24, 7790–7795. doi:10.1021/acs.orglett.2c03123
Wang, H., Zhou, Y., Xie, Y., Liu, Y., Li, Y., Zhang, H., et al. (2023a). Synthesis of functionalized 3-aryl-3H-benzofuranone derivatives from aryl acetate via [3 + 2] annulation of 1,4-dihydroxy-2-naphthoic acid ester. Org. Biomol. Chem. 21, 1821–1826. doi:10.1039/d2ob02319a
Wang, Z. S., Bennett, S. H., Kicin, B., Jing, C., Pradeilles, J. A., Thai, K., et al. (2023b). De novo synthesis of dihydrobenzofurans and indolines and its application to a modular, asymmetric synthesis of beraprost. J. Am. Chem. Soc. 145, 14124–14132. doi:10.1021/jacs.3c04582
Xiang, M., Li, C. Y., Zhang, J., Zou, Y., Huang, Z. C., Li, W.-S., et al. (2021). Organocatalyst-promoted diastereoselective and enantioselective Michael addition/hemiketalization reaction between hydroxymaleimide and quinone. Asian J. Org. Chem. 10, 1713–1717. doi:10.1002/ajoc.202100291
Yamamoto, H., and Ishihara, K. (2008). Acid catalysis in modern organic synthesis, Vol. 1 (Weinheim: Wiley VCH), 35–62.
Yamashita, M., Ohta, N., Shimizu, T., Matsumoto, K., Matsuura, Y., Kawasaki, I., et al. (2003). First total synthesis of (±)-Linderol A, a tricyclic hexahydrodibenzofuran constituent of Lindera umbellata bark, with potent inhibitory activity on melanin biosynthesis of cultured B-16 melanoma cells. J. Org. Chem. 68, 1216–1224. doi:10.1021/jo020619e
Yan, Y., Li, M., Liu, M., Huang, M., Cao, L., Li, W., et al. (2022). Sc(OTf)3-catalyzed dearomative [3+2] annulation of 5-aminoisoxazoles with quinone imine ketals or quinone monoacetals. Eur. J. Org. Chem. 18, e202200067. doi:10.1002/ejoc.202200067
Yang, Q., Wang, Y., Yang, J., Wu, Y., Li, L. Q., Chen, F.-J., et al. (2021). Activation of phenolic oxygen atom using polyphosphoric acid: synthesis of carbonyl-containing dihydrobenzofurans/dihydrobenzopyrans. Synth. Commun. 51, 1723–1730. doi:10.1080/00397911.2021.1902537
Yang, Y. H., Tan, Y. F., Zhao, Y. N., He, Y. H., and Guan, Z. (2023). Electrosynthesis of 5-aminocoumaran derivatives from olefins and aminophenols. Org. Lett. 25, 8205–8209. doi:10.1021/acs.orglett.3c03026
Yu, S.-C., Cheng, L., and Liu, L. (2021). Asymmetric organocatalysis with chiral covalent organic frameworks. Org. Mater. 3, 245–253. doi:10.1055/a-1400-5581
Yuan, H., Lu, D. L., Liang, C., and Mo, D. L. (2022). Synthesis of spirooxindole-benzo[d]oxazoles and dihydrobenzofurans through cycloaddition and rearrangement of N-vinyl nitrones and arynes. Adv. Synth. Catal. 364, 1409–1414. doi:10.1002/adsc.202101372
Zahoor, A. F., Yousaf, M., Siddique, R., Ahmad, S., Naqvi, S. A. R., and Rizvi, S. M. A. (2017). Synthetic strategies toward the synthesis of enoxacin-levofloxacin-and gatifloxacin-based compounds: a review. Synth. Commun. 47, 1021–1039. doi:10.1080/00397911.2017.1300921
Zbieg, J. R., Yamaguchi, E., McInturff, E. L., and Krische, M. J. (2012). Enantioselective C-H crotylation of primary alcohols via hydrohydroxyalkylation of butadiene. Science 336, 324–327. doi:10.1126/science.1219274
Zhang, J., Liu, M., Huang, M., Liu, H., Yan, Y., and Zhang, X. (2021). Enantioselective [3 + 2] annulation of 3-hydroxymaleimides with quinone monoimines. Org. Chem. Fron. 8, 2268–2273. doi:10.1039/d1qo00128k
Zhao, C., Ge, Z., Hu, J., Tian, H., and Wang, X. (2023). Enantioselective reductive aryl-benzylation of alkenes by a nickel-titanium bimetallic system. Cell Rep. 4, 101474. doi:10.1016/j.xcrp.2023.101474
Zheng, S. L., Yu, W. Y., Xu, M. X., and Che, C. M. (2003). First synthesis of naturally occurring (±)-epi-conocarpan. Tetrahedron Lett. 44, 1445–1447. doi:10.1016/s0040-4039(02)02860-5
Zhou, B., Yuan, Z., Yu, J., and Luan, X. (2022). Dearomatization/deiodination of o-iodophenolic compounds with α,β-unsaturated imines for accessing benzofuran derivatives. Org. Lett. 24, 837–841. doi:10.1021/acs.orglett.1c04065
Zhou, S., Cai, B., Hu, C., Cheng, X., Li, L., and Xuan, J. (2021). Visible light and base promoted O-H insertion/cyclization of para-quinone methides with aryl diazoacetates: an approach to 2,3-dihydrobenzofuran derivatives. Chin. Chem. Lett. 32, 2577–2581. doi:10.1016/j.cclet.2021.03.010
Zhu, J.-X., Tian, J.-M., Chen, Y.-Y., Hu, X.-J., Han, X., Chen, W., et al. (2023). Enantioselective synthesis of 2,3,3a,8a-Tetrahydrofuro[2,3-b]benzofuran scaffolds enabled by Cu(II)/SPDO-Catalyzed [3+2] cycloaddition of 2,3-dihydrofuran and quinone esters. J. Org. Chem. 88, 14670–14675. doi:10.1021/acs.joc.3c01681
Keywords: dihydrobenzofurans, transition metal-free, photocatalytic, organocatalyzed, catalyst free
Citation: Mushtaq A, Irfan M, Haq Au, Mansha A, Khan SG, Zahoor AF, Parveen B, Irfan A, Kotwica-Mojzych K, Glowacka M and Mojzych M (2024) Novel transition metal-free synthetic protocols toward the construction of 2,3-dihydrobenzofurans: a recent update. Front. Chem. 12:1470861. doi: 10.3389/fchem.2024.1470861
Received: 26 July 2024; Accepted: 31 October 2024;
Published: 13 December 2024.
Edited by:
Pezhman Shiri, Chinese Academy of Sciences (CAS), ChinaReviewed by:
Sagnik Sengupta, University of Texas Southwestern Medical Center, United StatesBing Yu, Zhengzhou University, China
Jayachandran Jayakumar, National Tsing Hua University, Taiwan
Copyright © 2024 Mushtaq, Irfan, Haq, Mansha, Khan, Zahoor, Parveen, Irfan, Kotwica-Mojzych, Glowacka and Mojzych. This is an open-access article distributed under the terms of the Creative Commons Attribution License (CC BY). The use, distribution or reproduction in other forums is permitted, provided the original author(s) and the copyright owner(s) are credited and that the original publication in this journal is cited, in accordance with accepted academic practice. No use, distribution or reproduction is permitted which does not comply with these terms.
*Correspondence: Ameer Fawad Zahoor, ZmF3YWQuemFob29yQGdjdWYuZWR1LnBr; Mariusz Mojzych, bW1vanp5Y2hAeWFob28uY29t