- 1Department of Materials Science and Engineering, Yonsei University, Seoul, Republic of Korea
- 2Department of Chemical and Biomolecular Engineering, Yonsei University, Seoul, Republic of Korea
- 3Materials Science and Chemical Engineering Center, Institute for Advanced Engineering, Yongin-si, Republic of Korea
- 4Yonsei-KIST Convergence Research Institute, Seoul, Republic of Korea
Room temperature gas sensing is crucial for practical devices used in indoor environments. Among various materials, metal oxides are commonly used for gas sensing, but their strong insulating properties limit their effectiveness at room temperature. To address this issue, many studies have explored diverse methods such as nanoparticle decoration or conductive support, etc. Here, we report the emergence of gas-sensing functionality at room temperature with improved CO gas selectivity on SnO2 nanoparticles through sequential steps by using amorphous carbon (a-C) support and PtOx decoration. The SnO2 decorated on amorphous carbon shows enhanced gas adsorption compared to inactive gas sensing on SnO2 decorated carbon support. The higher Vo site of SnO2 on a-C induces gas adsorption sites, which are related to the higher sp2 bonding caused by the large density of C defects. The ambiguous gas selectivity of SnO2/a-C is tailored by PtOx decoration, which exhibits six values of sensing responses (Rg/Ra or Ra/Rg) under CO gas at room temperature with higher selectivity. Compared to PtOx/a-C, which shows no response, the enhanced CO gas sensing functionality is attributed to the CO adsorption site on PtOx-decorated SnO2 particles. This report not only demonstrates the applicability of CO gas sensing at room temperature but also suggests a strategy for using SnO2 and carbon compositions in gas sensing devices.
1 Introduction
The increasing presence of air pollution gases, originating from not only the exhaust systems of combustion engines in heavy industries and vehicles but also from emissions in public places and residential buildings, has raised public awareness of biological health risks (Wang et al., 2018; Hou et al., 2012; Utriainen et al., 2003; Jia et al., 2014; Devaraj et al., 2022; Yamazoe, 2005). In response to this phenomenon, many studies have successfully developed gas sensing devices capable of detecting gases at sensitivities under a few parts per million (ppm) using metal oxide (MO) semiconductors. Consequently, the research trend in advanced gas-sensing materials has focused on improving sensitivity and selectivity, as measured by significant electrical resistance changes before and after analyte gas exposure on the MO surface (Dey, 2018; Franco et al., 2022; Eranna et al., 2024; Simon et al., 2001; Ji et al., 2019; Zakrzewska, 2001; Singh et al., 2021; Chen et al., 2013; Choi et al., 2021; Kim M. Y. et al., 2023; Kim et al., 2023b). This is achieved through various methods such as doping, nano-structuring, and creating heterojunctions. While these developed materials exhibit higher gas sensing performance at high temperatures and require significant electric power, there is a growing demand for room-temperature operational chemiresistor gas sensors. These sensors can be applied to smart mobile devices or IoT applications that monitor toxic gases in indoor environments with low electric power and integrated systems. Despite the increasing market demand for gas sensors that operate at room temperature, metal oxides face challenges due to their large band gaps and the interference caused by the preferred adsorption of hydroxyl groups over target gases on the MO surface (Tang et al., 2022; Srinivasan et al., 2019).
Among the diverse metal oxide (MO) candidates, SnO2 is one of the most widely used materials for gas sensing applications. SnO2 is an n-type semiconductor with a wide band gap of more than 3.0 eV, commonly used in gas sensors and catalytic activities due to its unique physical and chemical properties (Sun et al., 2022; Chen and Lou, 2013; Li et al., 2020; Masuda, 2022). Therefore, SnO2 has been investigated in various forms, such as hydrothermal, sol-gel, electrospinning, and polyol techniques, all of which have demonstrated promising performance (Chen and Lou, 2013; Masuda, 2022; Liu et al., 2023; Tonezzer et al., 2019; Mei et al., 2014; Yin et al., 2019). However, high-temperature operational SnO2-based gas sensors face challenges due to the strong insulating properties resulting from the large band gap and the adsorption of -OH groups from unavoidable moisture in the air, which interferes with the adsorption of analyte gases on the SnO2 surface (Tang et al., 2022; Srinivasan et al., 2019; Shah et al., 2022; Duoc et al., 2021). Thus, developing room-temperature operational SnO2 gas sensors has become a significant goal. To overcome these limitations, many studies have attempted to mix SnO2 with higher conductivity elements or employ nano-structuring techniques. Nevertheless, the consistently low response at room temperature makes it difficult to determine gas selectivity for these modified materials, leading to a trial-and-error approach in the development of room-temperature operational gas sensing materials.
In this report, we sequentially modify SnO2 to function as a room-temperature gas-sensing material using amorphous carbon (a-C) and PtOx. We enhance gas adsorption on SnO2 by using a-C support with a higher concentration of carbon defects, which shows no response in SnO2/C samples. Subsequently, PtOx-decorated SnO2/a-C demonstrates a CO gas selectivity with six of response, which is higher than the response for other gases. This improvement is due to CO adsorption on PtOx-coated SnO2 nanoparticles and external charge carrier conduction from PtOx/SnO2 to a-C, as opposed to the lack of gas response from PtOx nanoparticles on a-C. These results suggest a method for modifying MO particles for room-temperature gas sensing using a sequential strategy.
2 Materials and methods
2.1 Material synthesis
SnO2/a-C. The 34 mmol of D-glucose was dissolved in 170 mL of deionized water and stirred for 30 min. The mixed solution was placed in Teflon inner container and performed hydrothermal synthesis at 200°C for 12 h. After performing centrifuge from hydrothermally synthesized solution were dried in the vacuum oven overnight. The dried powder was conducted in a tube furnace filled with Ar atmosphere at 500°C to obtain the a-C. SnO2 decoration was again performed via hydrothermal synthesis with SnCl4⋅5H2O and a-C. The solution of SnCl4⋅5H2O and a-C in deionized water was stirred for 30 min and hydrothermal synthesis was performed at 200°C for 12 h and sequentially dried the centrifuged powder in the vacuum oven overnight. The obtained powder was calcined under an Ar atmosphere at 500°C for 1 h.
SnO2/C. SnO2 decoration on carbon was performed via hydrothermal synthesis using SnCl4⋅5H2O with nano-sized carbon (Sigma-Aldrich, nano powder carbon <100 nm) under 200°C for 12 h. The solution of SnCl4⋅5H2O and carbon nanoparticles in deionized water was stirred for 30 min, and hydrothermal synthesis was performed under 200°C for 12 h. The resulting powder was calcined under an Ar atmosphere at 500°C for 1 h.
PtOx/SnO2/a-C and PtOx/a-C. PtOx decoration on the SnO2/a-C or a-C particles was carried out using microwave synthesis for 30 s with H2PtCl6∙H2O (Sigma-Aldrich, 99.9% trace metals basis) dissolved in deionized water. After drying the synthesized powder overnight in the oven, we obtained the PtOx/SnO2/a-C and PtOx/a-C powder.
2.2 Material characterization
Morphological measurements were conducted by scanning electron microscopy (SEM, JEOL-7800F, JEOL Ltd.) and transmission electron microscopy (TEM, JEM-F200, JEOL Ltd.). The crystal structure characterization was performed using X-ray diffraction (XRD, Smart Lab, Rigaku) with Cu Kα radiation. Chemical bonding states were analyzed via X-ray photoelectron spectroscopy (XPS, K-alpha, Thermo Fisher Scientific Co.) Raman spectroscopy (LabRam Aramis, Horiba Jovin Yvon) was utilized to confirm the carbon vibration mode. The water contact angles for a-C and carbon were measured by Attension Theta Lite (Biolin Scientific).
2.3 Evaluate the gas-sensing performance
A 2-probe electrode configuration was employed for the gas sensing analysis. SnO2/a-C, SnO2/a-C, PtOx/SnO2/a-C, and PtOx/a-C properties were uniformly dispersed in ethanol at identical concentrations and subsequently drop-cast onto gold electrodes positioned on an alumina substrate. The gas-sensing performance of the fabricated sensors was evaluated within a custom-built chamber equipped with mass flow controllers, maintaining a fixed flow rate of 500 standard cubic centimeters per minute using air as the carrier gas. The sensors were exposed to target gas concentrations ranging up to 20 ppm for 500 s, followed by a recovery period in air for 1,000 s at a controlled temperature of 30°C. The resistance values in air (Ra) and upon exposure to the target gases (Rg) were recorded, and the sensor response (Rg/Ra) or Ra/Rg) was determined by calculating the ratio of resistance values in ambient air to those under gas exposure conditions Gas sensing measurements were performed for various gases, including Com HCHO, acetone, p-Xylene, Benzene, NO2, and SO2.
3 Results
3.1 Emergence of room temperature gas sensing on SnO2 using amorphous carbon
The emergence of gas adsorption sites on SnO2 nanoparticles depends on the bonding characteristics of the carbon supports. As shown in the SEM results in Figure 1A, we used various nano-sized carbon and a-C particles for support. The intensity ratio between sp3 carbon bonding at the D site and sp2 carbon bonding at the G site, noted as the ID/IG ratio in the Raman spectra (Figure 1B), is 0.77 for a-C, indicating a higher G band (sp2 peak of carbon) bonding in a-C. This implies a higher C vacancy site than in the carbon particles (ID/IG = 1.08) and suggests a higher -OH group concentration than in the carbon particles. Consequently, the favorable sp2 bonding character in a-C results in lower water contact angles compared to the carbon particle surface, as shown in Figure 1C.
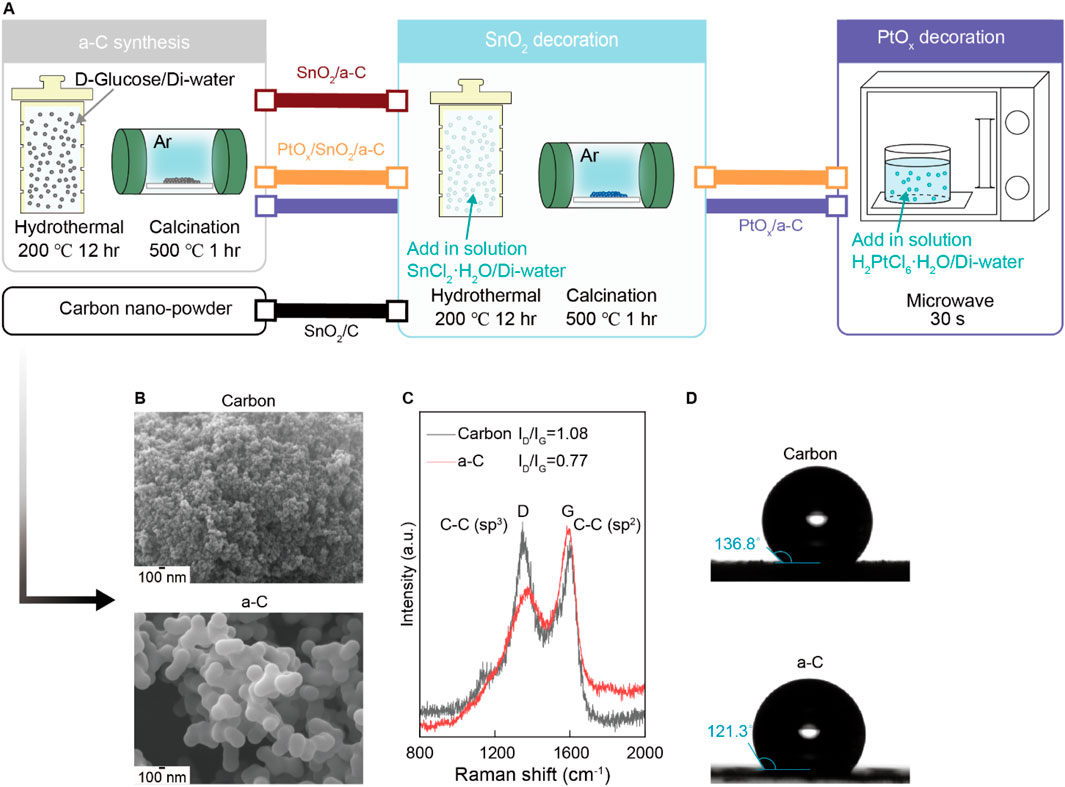
Figure 1. (A) Schematic illustration synthesis process of all samples. (B) SEM results of Carbon and a-C particles. (C) Raman results from Carbon and a-C particles. (D) Water contact angle difference of hydrophobic Carbon and a-C particles.
SnO2 nano-particles decorated on carbon supports (SnO2/C) and a-C (SnO2/a-C) are shown in the HR-TEM and EDS results (Figures 2A, B). Approximately 10 nm-sized SnO2 particles are evenly distributed on all Carbon supports. The X-ray diffraction (XRD) results in Figure 2C show the SnO2 single-phase peaks in all samples. However, the unobservable carbon peaks in the XRD results suggest lower crystallinity or an amorphous phase of the carbon supports. The XPS results for SnO2/C and SnO2/a-C (Figures 2D–F) demonstrate different chemical bonding characteristics. The carbon XPS results exhibit a larger asymmetric peak in SnO2/a-C binding energy. The remaining XPS intensity at higher binding energy than 284.8 eV indicates the existence of C-O single bonding or C=O double bonding, suggesting stronger bonding between a-C and Oxygen in SnO2 particles than in the Carbon supports (Kalish et al., 1999; Chokradjaroen et al., 2021). The Sn 3d and O 1s results display 496.4 eV for Sn 3d3/2, 487.9 eV for Sn 3d5/2, and 531.8 eV for O 1s in SnO2/a-C, whereas SnO2/C exhibits 496.3 eV for Sn 3d3/2, 487.8 eV for Sn 3d5/2, and 531.7 eV for O 1s. The higher binding energies of the peak positions indicate that the Sn on decorated SnO2 nanoparticles maintains the preferred +4 charge state on both the C and a-C supports, consistent with the single-phase SnO2 XRD pattern. Despite similar maximum peak positions for all samples, the higher Oxygen vacancy (VO) region compared to the symmetric shape of the O 1s peak is observed in the SnO2/C sample.
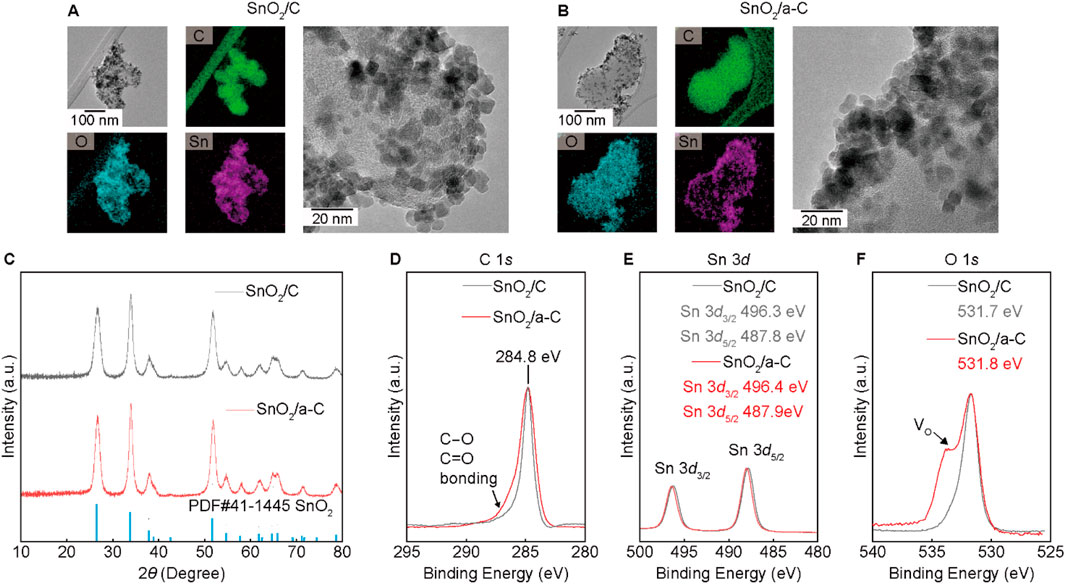
Figure 2. SnO2 on C or a-C particles. (A, B) TEM/EDS results of SnO2/C (A) and SnO2/a-C (B). (C) XRD results of SnO2/C and SnO2/a-C. (D–F) XPS results with composed elements such as C 1s (D), Sn 3d (E), and O 1s (F).
The higher VO density in SnO2/a-C provides more activated gas adsorption sites compared to SnO2/C samples. The chemiresistive gas sensing functionality, shown in Figure 3, measured the electrical resistance differences before and after exposure to 10 ppm analyte gases (CO, HCHO, acetone, p-Xylene, NO2, and SO2) for SnO2/C and SnO2/a-C at room temperature. Unlike SnO2/C, which shows no significant electrical resistance difference under any of the analyte gases, SnO2/a-C exhibits a response unit (Ra/Rg, where Ra is the resistance in air and Rg is the resistance under analyte gas) of less than five for all gases. However, SnO2/a-C shows ambiguous gas selectivity, with a response of 4.5 for CO gas, which is higher compared to less than three for other gases.
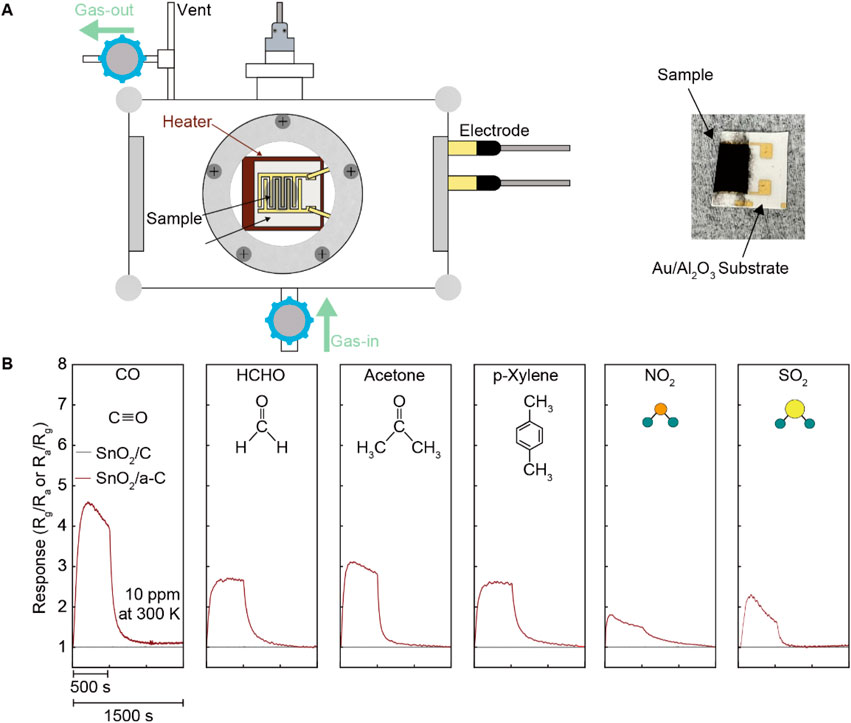
Figure 3. Emerged gas sensing functionality on SnO2/a-C than the SnO2/C at room temperature. (A) Schematically illustrated gas sensing measurement system and photo of SnO2/a-C coated Au/Al2O3 substrate. (B) Gas sensing is performed by resistance difference before/after diverse gases exposure such as CO, HCHO, acetone, p-Xylene, NO2, and SO.
3.2 Gas selectivity by PtOx decoration on the SnO2/a-C
The ambiguous gas selectivity of SnO2/a-C particles is modulated by decorating them with PtOx nanoparticles using hydrothermal synthesis methods. The PtOx nanoparticles are evenly distributed on the SnO2/a-C powder, as observed in the HR-TEM/EDS measurements in Figure 4A. The XRD results in Figure 4B show that the few wt% of PtOx nanoparticles are decorated while maintaining the crystal structure of SnO2/a-C powder. The Raman spectra of PtOx/SnO2/a-C in Figure 4C show a higher sp2 vibration peak (G) compared to the sp3 vibration mode peak (D), indicating a higher carbon vacancy on the a-C support. The XPS results for PtOx/SnO2/a-C particles in Figures 4D–G show measurements for Pt 4f, C 1s, Sn 3d, and O 1s, respectively. Compared to SnO2/a-C, the PtOx/SnO2/a-C samples exhibit a higher binding energy region on a-C, indicating C-O and C=O bonding sites between SnO2 and a-C. The Sn and O measurements show peaks at 495.5 eV for Sn 3d3/2, 487.1 eV for Sn 3d5/2, and 531.1 eV for O 1s, respectively. The similar peak positions of the Sn 3d peaks indicate a consistent charge state of Sn in SnO2/a-C samples, remaining in the 4+ valence state. The lower VO region in the O 1s XPS results after PtOx decoration on SnO2/a-C suggests complex O bonding with Sn and Pt. The peaks at 73.2 eV for Pt 4f7/2 and 76.5 eV for Pt 4f5/2 indicate the fully ionized state of Pt cations as PtOx on the SnO2 and a-C support. Furthermore, the oxidation state of Pt in PtOx/SnO2/a-C is similar to that in PtOx/a-C, independent of the matrix materials. However, the 533.3 eV O 1s peak, attributed to covalent bonding on the carbon tape, indicates a lower concentration of PtOx nanoparticles, suggesting that they are physically decorated on the surface without strong chemical bonding with SnO2 or carbon. The gas selectivity is primarily generated by the PtOx particles when they are decorated on SnO2 nanoparticles.
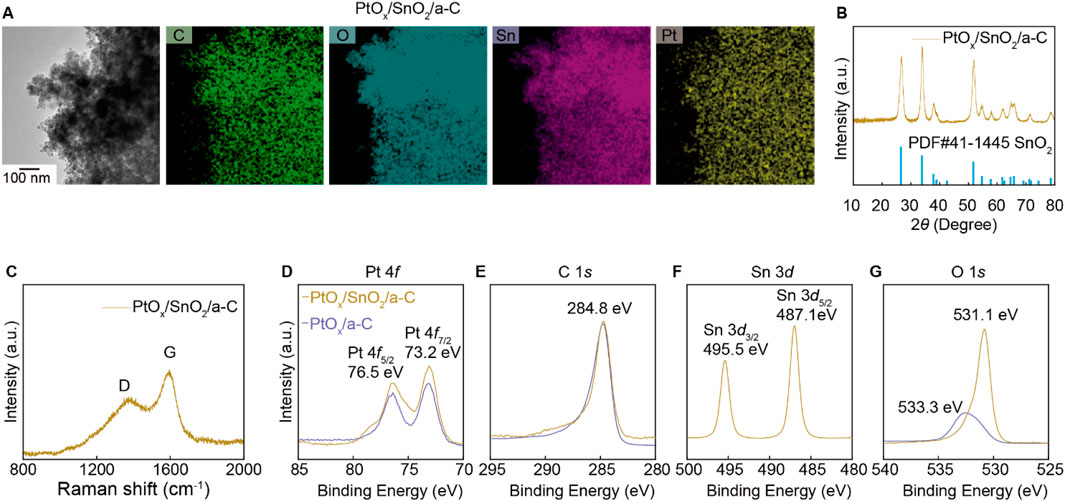
Figure 4. Structure analysis of PtOx decorated on SnO2/a-C particles. (A) TEM/EDS mapping results. (B) XRD results. (C) Raman results. (D–G) XPS results of PtOx/SnO2/a-C and PtOx/a-C with composed elements such as Pt 4f (D), C 1s (E), Sn 3d (F), and O 1s (G).
To further investigate the role of PtOx, we conducted gas-sensing measurements under the same conditions (room temperature, 10 ppm concentration of CO, HCHO, acetone, p-Xylene, NO2, and SO2). We also synthesized PtOx/a-C particles and evaluated their gas-sensing performance. The results, shown in Figure 5A, reveal a gas-sensing response of nearly seven under CO gas. Additionally, the CO gas sensing response of PtOx/SnO2/a-C remained consistent 1 month after synthesis, demonstrating higher selectivity for CO compared to other gases (HCHO, acetone, p-Xylene, NO2, and SO2). In contrast, PtOx/a-C powder showed no gas-sensing functionality for all of the tested gases. Comparing the gas selectivity for SnO2/C, SnO2/a-C, and PtOx/SnO2/a-C, as plotted in Figure 5B, demonstrates that the decorated PtOx particles impart CO gas selectivity to SnO2/a-C, which has an activated gas adsorption site. The response and recovery times (tRes. and tRec.) of SnO2/a-C and PtOx/SnO2/a-C under 10 ppm of CO gas are shown in the inset. The PtOx/SnO2/a-C sample displays a response time of 231 s and a recovery time of 101 s, while the SnO2/a-C sample exhibits a response time of 125 s and a recovery time of 149 s, respectively. A detailed analysis reveals that PtOx/SnO2/a-C demonstrates a faster tRes. than SnO2/a-C up to 70% of the maximum response, with the tRes. of PtOx/SnO2/a-C surpassing that of SnO2/a-C as it approaches the maximum gas response.
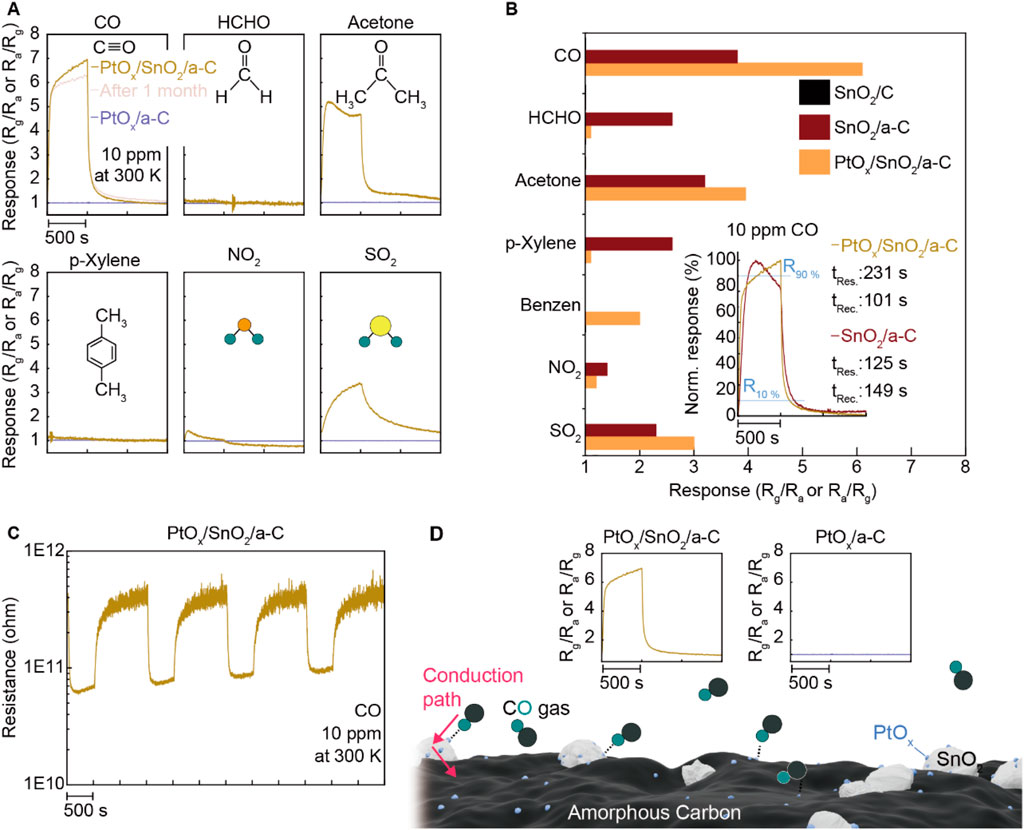
Figure 5. Specified gas selectivity on PtOx/SnO2/a-C. (A) Gas sensing response of PtOx/SnO2/a-C and PtOx/C under diverse gases such as CO, HCHO, acetone, p-Xylene, NO2, and SO2. (B) Comparison of PtOx/SnO2/a-C gas selectivity to SnO2/C and SnO2/a-C. The inset plot is the tRes. and tRec. of PtOx/SnO2/a-C and SnO2/a-C under CO gas. (C) The resistance-time plot while operating the gas sensing test. (D) Schematic illustrated the emergence of higher CO analyte gas adsorption site on PtOx/SnO2/a-C by comparing with none of the gas sensing responses on PtOx/C.
The reversible gas-sensing functionality of PtOx/SnO2/a-C during long-term gas-sensing measurements, shown in Figure 5C, demonstrates that PtOx/SnO2/a-C is an n-type gas-sensing device and exhibits a stable resistance range of 1011∼1012 Ω. The higher CO gas selectivity of PtOx/SnO2/a-C, compared to the lack of gas response on PtOx/a-C, demonstrates that PtOx on SnO2 is the primary gas adsorption site, and PtOx-.SnO2-a-C provides a sequential external carrier conduction route, as described in Figure 5D.
4 Conclusion
Both a-C and PtOx provide additional options for enabling gas sensing at room temperature on the SnO2 semiconductor. Specifically, a-C activates gas sensing functionality with higher gas adsorption at room temperature due to a higher concentration of carbon vacancies, while PtOx on SnO2 induces higher CO gas selectivity. These results suggest that this strategy for developing gas sensing materials can be applied not only to SnO2 but also to other conventional metal oxides with large band gaps, enabling their operation at room temperature.
Data availability statement
The original contributions presented in the study are included in the article/Supplementary Material, further inquiries can be directed to the corresponding authors.
Author contributions
YK: Formal Analysis, Investigation, Methodology, Writing–original draft. SL: Conceptualization, Data curation, Methodology, Writing–original draft, Writing–review and editing. YJ: Formal Analysis, Investigation, Methodology, Writing–original draft. JL: Formal Analysis, Investigation, Writing–original draft. DK: Formal Analysis, Investigation, Writing–original draft. BL: Formal Analysis, Investigation, Writing–original draft. CJ: Conceptualization, Writing–original draft, Writing–review and editing. KL: Conceptualization, Writing–original draft, Writing–review and editing.
Funding
The author(s) declare that financial support was received for the research, authorship, and/or publication of this article. This research was supported by Basic Science Research Program through the National Research Foundation of Korea (NRF) funded by the Ministry of Education (RS-2023-00243422). Kyu Hyoung Lee was supported by Basic Science Research Program through the National Research Foundation of Korea (NRF) funded by the Ministry of Education (NRF-2019R1A6A1A11055660), the Korea government (MSIT). (2022R1A2C2005210) and supported by the Technology Innovation Program (“20013621”, Center for Super Critical Material Industrial Technology) funded by the Ministry of Trade, Industry & Energy (MOTIE, South Korea). Yong Hwan Kim was supported by a grant of the Korea Health Technology R&D Project through the Korea Health Industry Development Institute (KHIDI), funded by the Ministry of Health & Welfare, Republic of Korea (HI19C1234). This study was supported by Samsung Electronics Co., Ltd. (IO201216–08204-01).
Conflict of interest
The authors declare that the research was conducted in the absence of any commercial or financial relationships that could be construed as a potential conflict of interest.
Publisher’s note
All claims expressed in this article are solely those of the authors and do not necessarily represent those of their affiliated organizations, or those of the publisher, the editors and the reviewers. Any product that may be evaluated in this article, or claim that may be made by its manufacturer, is not guaranteed or endorsed by the publisher.
References
Chen, J. S., and Lou, X. W. (2013). SnO2-Based nanomaterials: synthesis and application in lithium-ion batteries. Small 9, 1877–1893. doi:10.1002/smll.201202601
Chen, X., Wong, C. K. Y., Yuan, C. A., and Zhang, G. (2013). Nanowire-based gas sensors. Sens. Actuators B Chem. 177, 178–195. doi:10.1016/j.snb.2012.10.134
Choi, M. S., Kim, M. Y., Mirzaei, A., Kim, H.-S., Kim, S.-I., Baek, S.-H., et al. (2021). Selective, sensitive, and stable NO2 gas sensor based on porous ZnO nanosheets. Appl. Surf. Sci. 568, 150910. doi:10.1016/j.apsusc.2021.150910
Chokradjaroen, C., Watanabe, H., Ishii, T., and Ishizaki, T. (2021). Simultaneous synthesis of graphite-like and amorphous carbon materials via solution plasma and their evaluation as additive materials for cathode in Li–O2 battery. Sci. Rep. 11, 6261. doi:10.1038/s41598-021-85392-2
Devaraj, M., Rajendran, S., Hoang, T. K. A., and Moscoso, M. S.- (2022). A review on MXene and its nanocomposites for the detection of toxic inorganic gases. Chemosphere 302, 134933. doi:10.1016/j.chemosphere.2022.134933
Dey, A. (2018). Semiconductor metal oxide gas sensors: a review. Mater. Sci. Eng. B 229, 206–217. doi:10.1016/j.mseb.2017.12.036
Duoc, V. T., Hung, C. M., Nguyen, H., Duy, N. V., Hieu, N. V., and Hoa, N. D. (2021). Room temperature highly toxic NO2 gas sensors based on rootstock/scion nanowires of SnO2/ZnO, ZnO/SnO2, SnO2/SnO2 and, ZnO/ZnO. Sens. Actuators B Chem. 348, 130652. doi:10.1016/j.snb.2021.130652
Eranna, G., Joshi, B. C., Runthala, D. P., and Gupta, R. P. (2024). Oxide materials for development of integrated gas sensors—a comprehensive review. Crit. Rev. Solid State Mater. Sci. 29, 111–188. doi:10.1080/10408430490888977
Franco, M. A., Conti, P. P., Andre, R. S., and Correa, D. S. (2022). A review on chemiresistive ZnO gas sensors. Sens. Actuators Rep. 4, 100100. doi:10.1016/j.snr.2022.100100
Hou, C., Li, J., Huo, D., Luo, X., Dong, J., Yang, M., et al. (2012). A portable embedded toxic gas detection device based on a cross-responsive sensor array. Sens. Actuators B Chem. 161 (1), 244–250. doi:10.1016/j.snb.2011.10.026
Ji, H., Zeng, W., and Li, Y. (2019). Gas sensing mechanisms of metal oxide semiconductors: a focus review. Nanoscale 11, 22664–22684. doi:10.1039/C9NR07699A
Jia, Q., Ji, H., Zhang, Y., Chen, Y. Y., Sun, X., and Jin, Z. (2014). Rapid and selective detection of acetone using hierarchical ZnO gas sensor for hazardous odor markers application. J. Hazard. Mater. 276, 262–270. doi:10.1016/j.jhazmat.2014.05.044
Kalish, R., Reznik, A., Nugent, K. W., and Prawer, S. (1999). The nature of damage in ion-implanted and annealed diamond. Nucl. Instrum. Meth. B 148, 626–633. doi:10.1016/S0168-583X(98)00857-X
Kim, M. Y., Hwang, J. Y., Mirzaei, A., Choi, S.-W., Kim, S.-I., Kim, H.-S., et al. (2023b). NO2 gas sensing properties of Ag-functionalized porous ZnO sheets. Adsorpt. Sci. Technol. 2023. doi:10.1155/2023/9021169
Kim, M. Y., Lee, S. Y., Kim, J., Park, C. O., Shi, W., Mina, H., et al. (2023a). Generation of nanogaps on porous ZnO sheets via Li-ion implantation: NO2 gas sensing with ultrafast recovery time. Sens. Actuators B Chem. 379, 133283. doi:10.1016/j.snb.2022.133283
Li, B., Zhou, Q., Peng, S., and Liao, Y. (2020). Recent advances of SnO2 -based sensors for detecting volatile organic compounds. Front. Chem. 8, 321. doi:10.3389/fchem.2020.00321
Liu, P., Wang, J., Jin, H., Ge, M., Zhang, F., Wang, C., et al. (2023). SnO2 mesoporous for highly nanoparticle-based gas sensor sensitive and low concentration formaldehyde detection. RSC Adv. 13, 2256–2264. doi:10.1039/d2ra06745e
Masuda, Y. (2022). Recent advances in SnO2 nanostructure based gas sensors. Sens. Actuators B Chem. 364, 131876. doi:10.1016/j.snb.2022.131876
Mei, L., Chen, Y., and Ma, J. (2014). Gas sensing of SnO2 nanocrystals revisited: developing ultra-sensitive sensors for detecting the H2S leakage of biogas. Sci. Rep. 4, 6028. doi:10.1038/srep06028
Shah, V., Bhaliya, J., Patel, G. M., and Joshi, P. (2022). Room-temperature chemiresistive gas sensing of SnO2 nanowires: a review. J. Inorg. Organomet. Polym. Mater. 32, 741–772. doi:10.1007/s10904-021-02198-5
Simon, I., Bârsan, N., Bauer, M., and Weimar, U. (2001). Micromachined metal oxide gas sensors: opportunities to improve sensor performance. Sens. Actuators B Chem. 73 (1), 1–26. doi:10.1016/S0925-4005(00)00639-0
Singh, A. A., Sikarwar, S., Verma, A., and Yadav, B. C. (2021). The recent development of metal oxide heterostructures based gas sensor, their future opportunities and challenges: a review. Sens. Actuators A Phys. 332 (1), 113127. doi:10.1016/j.sna.2021.113127
Srinivasan, P., Ezhilan, M., Kulandaisamy, A. J., Babu, K. J., and Rayappan, J. B. B. (2019). Room temperature chemiresistive gas sensors: challenges and strategies—a mini review. J. Mater. Sci. Mater. Electron. 30, 15825–15847. doi:10.1007/s10854-019-02025-1
Sun, C., Yang, J., Xu, M., Cui, Y., Ren, W., Zhang, J., et al. (2022). Recent intensification strategies of SnO2-based photocatalysts: a review. J. Chem. Eng. 427, 131564. doi:10.1016/j.cej.2021.131564
Tang, Y., Zhao, Y., and Liu, H. (2022). Room-temperature semiconductor gas sensors: challenges and opportunities. ACS Sens. 7, 3582–3597. doi:10.1021/acssensors.2c01142
Tonezzer, M., Izidorod, S. C., Moraes, J. P. A., and Dang, L. T. T. (2019). Improved gas selectivity based on carbon modified SnO2 nanowires. Front. Mater. 6, 277. doi:10.3389/fmats.2019.00277
Utriainen, M., Kärpänoja, E., and Paakkanen, H. (2003). Combining miniaturized ion mobility spectrometer and metal oxide gas sensor for the fast detection of toxic chemical vapors. Sens. Actuators B Chem. 93 (1–3), 17–24. doi:10.1016/S0925-4005(03)00337-X
Wang, H., Lustig, W. P., and Li, J. (2018). Sensing and capture of toxic and hazardous gases and vapors by metal–organic frameworks. Chem. Soc. Rev. 47, 4729–4756. doi:10.1039/C7CS00885F
Yamazoe, N. (2005). Toward innovations of gas sensor technology. Sens. Actuators B Chem. 108 (1–2), 2–14. doi:10.1016/j.snb.2004.12.075
Yin, X. T., Zhou, W. D., Li, J., Wang, Q., Wu, F. Y., Dastan, D., et al. (2019). A highly sensitivity and selectivity Pt-SnO2 nanoparticles for sensing applications at extremely low level hydrogen gas detection. J. Alloys Compd. 805, 229–236. doi:10.1016/j.jallcom.2019.07.081
Keywords: SnO2, gas sensor, CO gases, room temperature, nanocomposites
Citation: Kim YH, Lee SY, Ji Y, Lee JH, Kim DW, Lee B, Jin C and Lee KH (2024) Stepwise emergence of CO gas sensing response and selectivity on SnO2 using C supports and PtOx decoration. Front. Chem. 12:1469520. doi: 10.3389/fchem.2024.1469520
Received: 24 July 2024; Accepted: 13 September 2024;
Published: 03 October 2024.
Edited by:
Xiaopeng Han, Tianjin University, ChinaReviewed by:
Diego González-Flores, University of Costa Rica, Costa RicaFeng Hu, Nanjing University of Aeronautics and Astronautics, China
Copyright © 2024 Kim, Lee, Ji, Lee, Kim, Lee, Jin and Lee. This is an open-access article distributed under the terms of the Creative Commons Attribution License (CC BY). The use, distribution or reproduction in other forums is permitted, provided the original author(s) and the copyright owner(s) are credited and that the original publication in this journal is cited, in accordance with accepted academic practice. No use, distribution or reproduction is permitted which does not comply with these terms.
*Correspondence: Changhyun Jin, ejgwMTUwMjZAeW9uc2VpLmFjLmty; Kyu Hyoung Lee, a2hsZWUyMDE4QHlvbnNlaS5hYy5rcg==
†These authors have contributed equally to this work contributed to this work