- Faculty of Metallurgical and Energy Engineering, Kunming University of Science and Technology, Kunming, China
High-performance wide-bandgap (WBG) perovskite solar cells are used as top cells in perovskite/silicon or perovskite/perovskite tandem solar cells, which possess the potential to overcome the Shockley-Queisser limitation of single-junction perovskite solar cells (PSCs). However, WBG perovskites still suffer from severe nonradiative recombination and large open-circuit voltage (Voc) losses, which restrict the improvement of PSC performance. Herein, we introduce 3,3′-diethyl-oxacarbo-cyanine iodide (DiOC2(3)) and multifunctional groups (C=N, C=C, C-O-C, C-N) into perovskite precursor solutions to simultaneously passivate deep level defects and reduce recombination centers. The multifunctional groups in DiOC2(3) coordinate with free Pb2+ at symmetric sites, passivating Pb vacancy defects, effectively suppressing nonradiative recombination, and maintaining considerable stability. The results reveal that the power conversion efficiency (PCE) of the 1.68 eV WBG perovskite solar cell with an inverted structure increases from 18.51% to 21.50%, and the Voc loss is only 0.487 V. The unpackaged device maintains 95% of its initial PCE after 500 h, in an N2 environment at 25°C.
Introduction
Perovskite solar cells (PSCs) are one of the most promising next-generation photovoltaic technologies due to their high light absorption coefficient, tunable bandgap width, excellent low-light performance, long carrier mobility, and flexible preparation (Kim et al., 2016; Bai et al., 2019; Min et al., 2021). In just over a decade, the laboratory’s power conversion efficiency (PCE) surged from 3.8% to 26.1% (Kojima et al., 2009; Best, 2024), which is almost comparable to that of crystalline silicon solar cells. The PSCs face huge challenges in moving toward industrialization due to limitations in the Shockley-Queisser (SQ) limit and poor stability (Leijtens et al., 2018; Kim et al., 2021). Tandem solar cells can efficiently harness photon energy to overcome the SQ limit. This entails appropriate wide-bandgap (WBG) perovskites (bandgap (E.g.,) > 1.65 eV) being used as the top cell, and narrow-bandgap (NBG) perovskites (Jiang et al., 2022; Wang et al., 2022; Chen et al., 2023) or silicon (Si) (Lamanna et al., 2020; Luo et al., 2023) (E.g., ≈ 1.12 eV) being used as the bottom cell. The use of bromide, instead of partial iodide, to adjust the bandgap of WBG perovskite materials has garnered interest (Eperon et al., 2014; Saliba et al., 2018). However, WBG PSCs under continuous illumination would cause problems such as photo-induced halide segregation. Halides in perovskites demonstrate nonuniform distribution in the vertical direction; bromine and iodide distribute at the top and bottom of the perovskites (Hoke et al., 2015; Zheng et al., 2022), creating nonradiative recombination centers, resulting in a much greater open circuit voltage (Voc) loss than expected. Therefore, it is imperative to address the problem limiting the performance of WBG mixed halide PSCs to achieve low-cost and high-performance tandem solar cells (Li et al., 2023).
So far, WBG perovskites always suffer from high Voc losses (Wang et al., 2024), which hinders improving the PCE. There are two main reasons for the considerable loss of Voc: i) Deep-level defects in perovskite films become recombination centers, thus affecting the lifetime of nonequilibrium minority carriers, causing irreversible damage to the solar cells, and significantly affecting the photovoltaic performance of PSCs (Shen et al., 2024). ii) The energy level mismatch between the perovskite layer and the electron transport layer (ETL) causes electrons to cross higher potential barriers during transmission. Some carriers that cannot be crossed not only fail to produce radiative recombination but also deteriorate device performance (Gan et al., 2023). To improve the efficiency of WBG PSCs, reduce recombination, and enhance carrier extraction and mobility, different new self-assembled monolayer small molecules or additive engineering are used to suppress nonradiative recombination, adjust energy level arrangement, achieve efficient carrier extraction and transmission, and improve performance. For example, Zhao et al. designed a novel self-assembled monolayer (4- (7H dibenzo [c, g] carbazol-7-yl) phosphonic acid (4PADCB)) as a hole transport layer for PSCs by constructing a new 7H dibenzcarbazole (DCB) end group, promoting the growth of high-quality WBG perovskites, and suppressing nonradiative recombination at the interface, thereby achieving efficient hole extraction (He et al., 2023). In addition, Fang et al. added potassium antimony tartrate (APTA) to the precursor of WBG perovskite as a multifunctional additive, which regulates the dynamic growth of perovskite crystals, reduces the binding energy of lead, and improves the energy level arrangement between the absorption and electron transport layers of perovskites, effectively accelerating the extraction of charge carriers and reducing the Voc loss (Hu et al., 2023). Although introducing new self-assembled molecules can improve interface contact and reduce Voc losses, the defects of lead iodide vacancies and halogen vacancies still exist. Vacancy defects can generate deep trap energy levels, inducing nonradiative recombination, leading to carrier recombination, and causing unnecessary performance defects. Therefore, understanding the types of defects present in perovskites and leveraging the synergistic passivation effect of various functional groups can effectively reduce the trap density of states, suppress ion migration, enhance carrier mobility, and significantly improve device performance and stability.
In this study, we present a doping strategy to modify perovskite thin films by introducing 3,3′-diethyl-oxacarbo-cyanine iodide (DiOC2(3)) into a perovskite precursor solution. DiOC2(3) is composed of two benzene rings, carbon double bonds (C=C), carbon-nitrogen double bonds (C=N), and carbon-nitrogen single bonds (C-N) and ether bonds (C-O-C), exhibiting a symmetrical structure with multiple functional groups. Consequently, DiOC2(3) with these electron-rich groups theoretically serves as a Lewis acid and base to bind to uncoordinated Pb2+, while C=N and C-N, as symmetric structures, can simultaneously anchor free Pb2+. Furthermore, the unsaturated C=C groups would modify the energy level structure of the perovskite, reducing the potential barrier during electron and hole transport, and establishing a P-N heterojunction. This effectively enhances the carrier transfer rate, benefiting from the improvement of the built-in electric field. A more stable band structure and lower defect density significantly improve the performance of PSCs. The findings indicate that the device modified with DiOC2(3) achieved a higher power conversion efficiency (PCE) of 21.50%, compared to the control device with 18.51% PCE. The short-circuit current density (Jsc) also notably increased from 20.18 mA cm-2–22.59 mA cm-2, the Voc loss has been only 0.487 V, and the modified device is exhibiting enhanced humidity stability. Specifically, the unpackaged device maintains 95% of its initial photoelectric conversion efficiency after 500 h, in an N2 environment at 25°C.
Results and discussions
As shown in Figure 1A, the molecular structure of DiOC2(3) displayed a centrally symmetric arrangement with the C=C bond at its core, flanked by C=N and C-N bonds on the left and right, respectively. Figure 1B shows the device structure diagram. DiOC2(3) is introduced proportionally into the perovskite precursor solution, followed by spin-coating onto the hole transport layer (HTL) and a subsequent annealing process at 100°C to facilitate crystallization. Furthermore, we fabricate and test a series of PSCs and perovskite films treated with different volume ratios of DiOC2(3) solution. The J-V curves of the devices are shown in Supplementary Figure S1a and the steady state photoluminescence (PL) spectra of the control film and DiOC2(3)-modified film are shown in Supplementary Figure S1b, Supporting Information. The PCE of the DiOC2(3)-modified device demonstrated a trend of first increasing and then decreasing with the increase of volume ratio in comparison with the control device, when the volume ratio of perovskite: DiOC2(3) is 150: 1, PSCs exhibit the best performance. In addition, the steady state PL spectra of the perovskite film show the same trend, and the PL intensity of modified perovskite film (perovskite: DiOC2(3) = 150: 1) is three times higher than that of the control film. Therefore, we conduct the systematic studies under the optimal volume ratio conditions. Ultraviolet-visible (UV-vis) absorption spectroscopy is performed to investigate the effect of DiOC2(3) on the optical properties of perovskite films. As shown in Figure 1C, the absorption spectra of DiOC2(3)-modified and control films are consistent with an absorption intensity of 500–800 nm, and the corresponding Tauc plots indicate the identical bandgap of 1.68 eV (Supplementary Figure S2, Supporting Information), indicating that DiOC2(3) does not change the bandgap of the perovskite film. Notably, within the 300–500 nm wavelength range, the absorption intensity increases following DiOC2(3) modification, suggesting that DiOC2(3) possesses additional UV spectrum absorption capabilities and effectively harnesses solar spectral energy (Dai et al., 2024). We investigate the carrier transport dynamics of DiOC2(3) on perovskite films by testing the steady-state PL and time-resolved photoluminescence (TRPL) of perovskite films. As shown in Figure 1D, the PL spectrum of the DiOC2(3)-modified perovskite film is stronger than that of the control, indicating a decrease in the defect density and an inhibition of nonradiative recombination after DiOC2(3) modification. The characteristic PL peaks of the DiOC2(3)-modified perovskite films and control film are both at 738 nm, indicating that the bandgap of the perovskite film is not changing, which is consistent with the UV-vis spectroscopy results (Guo et al., 2023; Zhang et al., 2023). Additionally, Figure 1E illustrates the TRPL outcomes for both DiOC2(3)-modified perovskite films and the control film, revealing that the perovskite films treated with DiOC2(3) demonstrate a slower decay and longer carrier lifetime. The corresponding double exponential fitting results are presented in Table 1. The fitting data is calculated according to the following Equation 1:
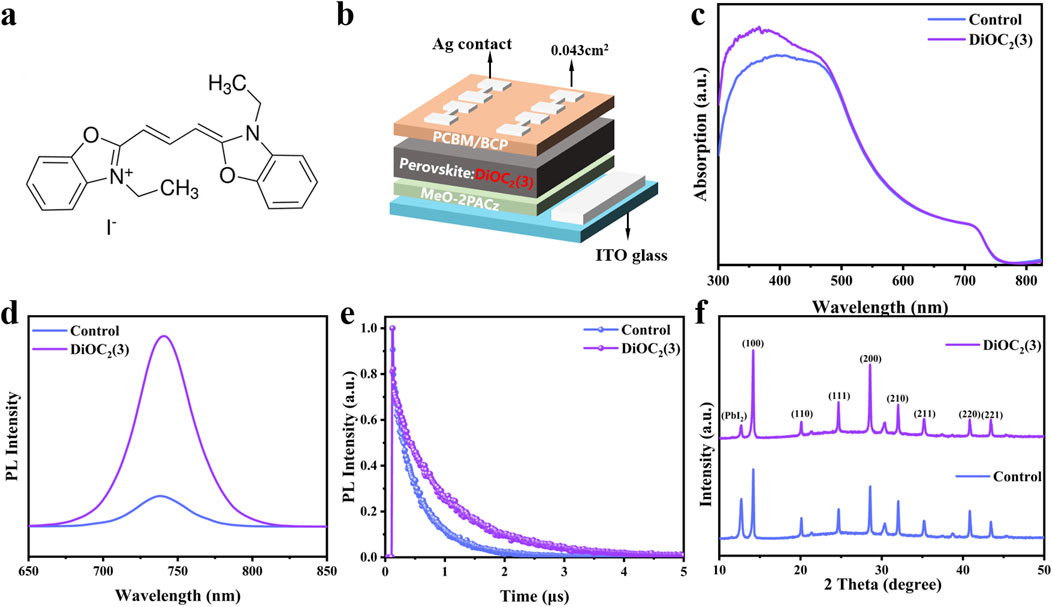
Figure 1. (A) Molecular structure of DiOC2(3). (B) Schematic diagram of the device structure with the DiOC2(3) introduced proportionally into the perovskite precursor solution. (C) The UV-vis absorption spectra of perovskite films without and with DiOC2(3) modification. (D) PL spectra and (E) TRPL spectra of perovskite films without and with DiOC2(3) modification. (F) XRD patterns of the perovskite films without and with DiOC2(3) modification.
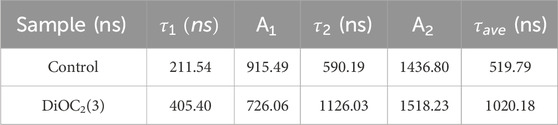
Table 1. Fitting parameters of the bi-exponential decay function in TRPL spectra of 1.68 eV perovskite films without and with DiOC2(3) modification.
Where average lifetime
To further investigate the effect of DiOC2(3) on the crystallization kinetics of perovskites, X-ray diffraction patterns (XRD) of perovskite films are tested. Figure 1F shows the XRD patterns of DiOC2(3)-modified perovskite films and control films. The peak of PbI2 in the perovskite film modified with DiOC2(3) is significantly reduced. The peaks of (100) and (200) crystal planes are stronger, as shown in Supplementary Figure S3, Supporting Information, and the corresponding half peak width (FWHM) is reduced, indicating that DiOC2(3) coordinated with free Pb2+ through multi-site anchoring groups, forming C=N. Pb and C-N. Pb covalent bonds, thus reducing the precipitation of PbI2 (Cao et al., 2022; Du et al., 2022). Moreover, I− ions occupy halogen vacancies, thus impeding ion migration and enhancing the crystallinity of the perovskite (Dong et al., 2023). A better crystal orientation increases the grain size, and the grain boundary resistance experienced by charge carriers during transport decreases, thereby improving the performance of perovskite thin films (Kim et al., 2019).
We investigate the effect of DiOC2(3) on the morphology of perovskite films by scanning electron microscopy (SEM) (Figures 2A,B) and atomic force microscopy (AFM) (Figure 2C). The results show that the grain size of DiOC2(3)-modified perovskites is increased from 160 nm to 220 nm (Supplementary Figure S4, Supporting Information), and the surface morphology becomes smoother than that of control perovskite thin films, with significantly reduced gaps and uniform grain morphology, which illustrates the increase of Jsc. In the vertical direction, as shown in Figure 2B, the grain boundaries between the grains serve as defect states, less grain boundaries in DiOC2(3)-modified perovskite implies suppressed defect states (Chen et al., 2023). Moreover, the AFM results in Figure 2C demonstrate that the root-mean-square (RMS) roughness of the surface of the DiOC2(3)-modified perovskites film is decreased from 23.5 nm to 19.0 nm. A smoother surface is conducive to the deposition of electron transport layers on the surface of the perovskite layer, resulting in tighter contact between layers and more effective electron extraction processes (Li et al., 2023). Figure 2D shows the contact angle between the DiOC2(3)-modified and control surfaces. The results show that the contact angle of the DiOC2(3)-modified surface is increased, indicating a significant improvement in the hydrophobicity of the perovskite surface (Liu et al., 2024). This is due to the ether bond (C-O-C) in the DiOC2(3) molecule. The difference in electronegativity between carbon and oxygen atoms makes it difficult for the ether molecule to dissolve in water, thereby improving its stability (Chen et al., 2022).
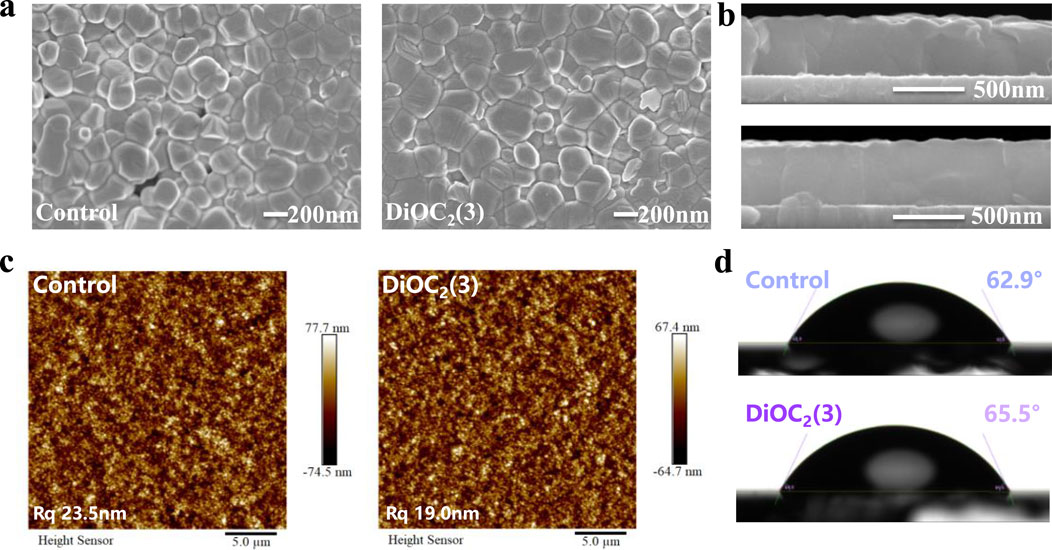
Figure 2. (A) Top-view and (B) Cross-sectional scanning electron microscopy (SEM) images of perovskite films without and with DiOC2(3) modification. (C) AFM images of perovskite films without and with DiOC2(3) modification. (D) Water contact angles of perovskite films without and with DiOC2(3) modification.
To verify the interaction between multifunctional groups in the DiOC2(3) molecule and perovskite, the chemical state of the surface elements of perovskites is studied through X-ray photoelectron spectrometry (XPS). Figure 3A shows the resolution spectra of Pb 4f and O 1s. The Pb 4f peak of the perovskite film modified with DiOC2(3) shifts towards the direction with lower binding energy, but the shift amplitude is relatively small, indicating that the C=N and C-N groups of DiOC2(3) interact synergistically at symmetric sites, The C=N bond acts as a Lewis base to coordinate with Pb2+, as a donor for electronic units and increasing the electron cloud density around the atom (Ren et al., 2022). The C-N bond acts as a Lewis acid to coordinate with Pb2+, reducing the electron cloud density around the atom. However, owing to the stronger interaction of the C=N bond, Pb 4f shifts slightly towards a lower binding energy (Zhang et al., 2018). Furthermore, the C=N as an electron-rich unit, could play a Lewis acid-base role, causing the electron-rich environment of Pb2+ through electron donating, thereby stabilizing the structure. The peak of O 1s in Figure 3B shifts from 530.96 to 531.20 eV, indicating a strong interaction between ether bonds and the perovskite, filling the FA vacancy by binding with A-site cations. As shown in Supplementary Figure S5, Supporting Information, the peak of C 1s shifts from 284.80 to 284.93 eV, indicating that the C=C bond serves as the central site group interacting with perovskite (Jayanthi et al., 2022).
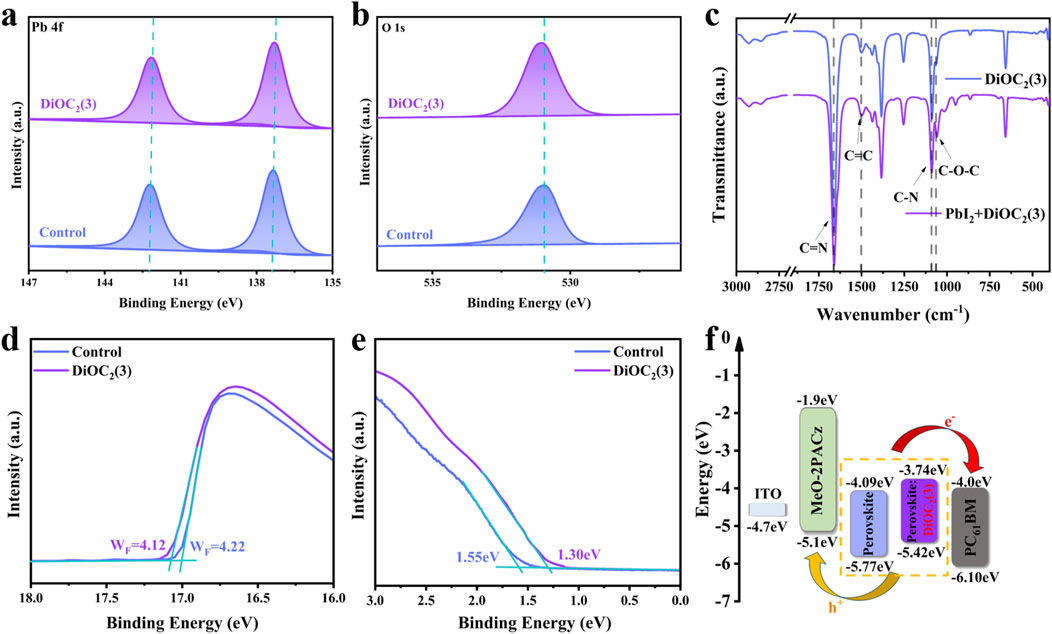
Figure 3. X-ray photoelectron spectrometry (XPS) spectra of (A) Pb 4f and (B) O 1s in the perovskite films without and with DiOC2(3) modification. The FTIR spectra of pure DiOC2(3) and DiOC2(3)/PbI2 are shown in panels (C). UPS data of perovskite films without and with DiOC2(3). (D) Helium Iα (hν = 21.2 eV) spectra of secondary electron cut-off and (E) UPS spectra of the valence band (VB) region. (F) Schematic illustration of energy level alignment of this work.
Fourier transform infrared spectroscopy (FTIR) analysis is performed on the pure solution of DiOC2(3) and the mixed solution of DiOC2(3) and PbI2, as shown in Figure 3C. The stretching vibration peaks of C=N and C-N groups appeared at 2,500–1,600 cm-1 and 1,250–1,000 cm-1, respectively, This proves that DiOC2(3) can interact well with Pb2+, as a Lewis acid and base to effectively passivate Pb vacancies (Cao et al., 2022). In addition, C=C and C-O-C stretching vibration peaks appeared at 1,600–1,300 cm-1 and 1,100–1,000 cm-1 (An et al., 2023; Zhang et al., 2023), indicating that the C=C bond is serving as the central site group. The C-O-C bond, as a symmetric site group, effectively stabilizes the perovskite lattice, forming a low-defect, high-crystallinity perovskite film, which is conducive to the transport of charge carriers (Shao et al., 2024). Additionally, the work function (WF) and valence band maximum (VBM) is measured through UV photoelectron spectroscopy (UPS) to further demonstrate the improvement of the carrier transport rate, as shown in Figures 3D–F. The WF and VBM are calculated from the measured secondary electron cut-off edge and Fermi edge, respectively. Based on the VBM and bandgap (1.68 eV) of the perovskite, the minimum conduction band (CBM) of the perovskite film is determined, as shown in Figure 3D. The WF modified with DiOC2(3) decreased from 4.22 to 4.12 eV, whereas the VBM is located at 1.30 and 1.55 eV, respectively (Figure 3E), with an upward shift in energy levels, which matches the energy levels of ETL and HTL. During electron and hole transports, the potential barrier is lowered, thereby improving the transfer rate, reducing nonradiative recombination, and decreasing the density of defect states (Stolterfoht et al., 2019; Azmi et al., 2022).
Based on this, we prepared PSCs based on ITO/MeO-2PACz/Perovskite: DiOC2(3)/PCBM/BCP/Ag, as shown in Figure 1B. Figure 4A shows the current density (J) - voltage (V) curve of the PSCs before and after DiOC2(3) modification and Table 2 shows the numerical comparison of each parameter. The control PSCs have a short-circuit current density (Jsc) of 20.18 mA cm-2, an open circuit voltage (Voc) of 1.170 V, and a fill factor (FF) of 0.78 and a PCE of 18.51%,. After modification with DiOC2(3), the PCE of the device reaches 21.50%, Jsc is 22.59 mA cm-2, Voc is 1.193 V (Voc loss is only 0.487 V), and FF is 0.80. As shown in Supplementary Figure S6, Supporting Information, the target device showed a higher PCE of 21.49 (21.47) % with a VOC of 1.193 (1.189) V, a JSC of 22.58 (22.55) mA cm-2, and a fill factor (FF) of 79.79 (80.13) % under reverse (forward) scan. The results show that the target PSCs display negligible hysteresis. The improvement in the device performance also proved the reduction in surface defects in DiOC2(3)-modified perovskite films. The external quantum efficiency (EQE) is further conducted (Supplementary Figure S7a, Supporting Information), and the results show that the DiOC2(3)-modified perovskite device has a higher EQE in the visible light range. The integrated Jsc of control and DiOC2(3)-modified PSCs are 20.52 and 22.36 mA cm-2. The steady-state output (SPO) efficiencies of the corresponding control and target devices at the maximum power points were 18.4% and 21.3% (Supplementary Figure S7b), respectively, which were also in line with the values determined from the J–V curves.
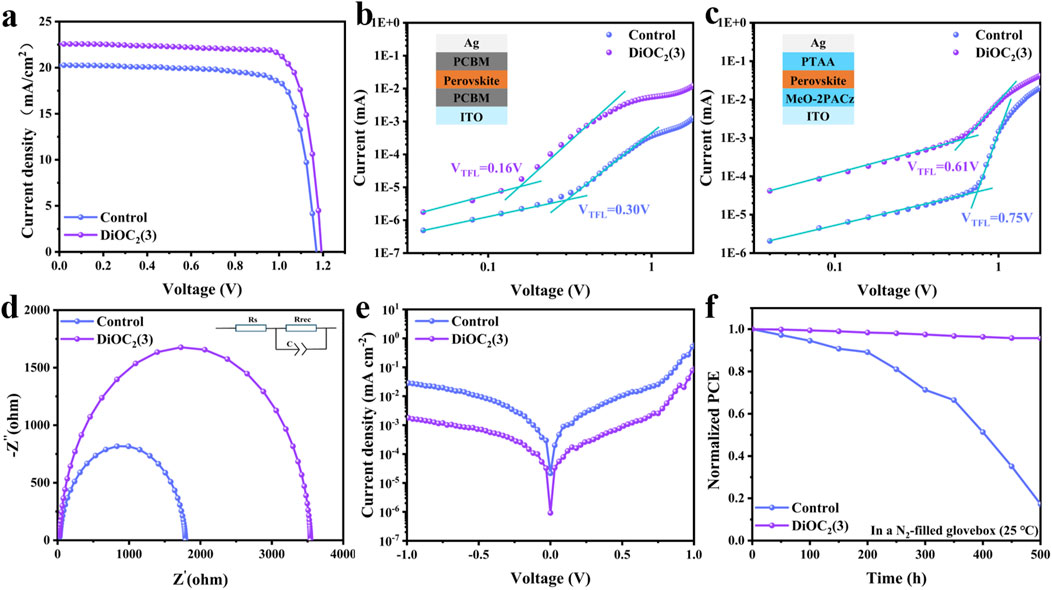
Figure 4. (A) J-V curves of the control DiOC2(3)-modified devices measured at 100 mW cm-2 irradiation. SCLC curves of the electron-only device based on (B) and the hole-only device based on (C) without and with DiOC2(3) modification perovskite films. The inset shows the device structure. (D) Nyquist plots of the two devices at a bias of 1 V in the dark. (E) Dark J-V curves of perovskite films without and with DiOC2(3) modification. (F) Long-term stability of the unencapsulated devices under an N2 atmosphere with a temperature of 25°C.
To further investigate the passivation effect of DiOC2(3) on perovskites, we fabricate pure electron and hole devices to study the trap-state density of states using the space-charge-limited current (SCLC) method (Figures 4B,C). The density of trap-states can be calculated by formula 2:
where Ntrap is the density of trap-states, εr is the vacuum permittivity, ε0 is the relative dielectric constant for the perovskite film, e is the electron charge, L is the thickness of the perovskite layer (≈450 nm), and VTFL is the trap-filled limit voltage (Zheng et al., 2020). Figure 4B shows the electron defect density of states, where VTFL decreased from 0.30 V to 0.16 V, and the corresponding defect density of states decreased from 1.95 × 1016 cm-3 to 1.04 × 1016 cm-3. Figure 4C shows the density of states for hole defects, where VTFL decreased from 0.75 V to 0.61 V, and the corresponding density of states for defects decreased from 4.88 × 1016 cm-3 to 3.97 × 1016 cm-3. The results show that the density of states for both electron and hole defects decreases, thereby increasing the mobility of electrons and holes. It is assumed that the DiOC2(3) modification interacts with uncoordinated Pb2+, occupying Pb and I vacancies. The electron impedance spectroscopy (EIS) analysis is conducted under dark conditions as shown in Figure 4D. The perovskite modified with DiOC2(3) exhibits a decreased in Rs and increased in Rrec, further indicating the suppression of nonradiative recombination, (Zhu et al., 2022; Zhao et al., 2023). The reverse saturation current density (Jo) is related to the defect state density and the smaller the value of Jo, the lower the defect state density as shown in Figure 4E (Song et al., 2021; Wu et al., 2021). This further confirms that DiOC2(3) has a significant passivation effect on defects under the synergistic effect of multiple sites of C=N, C-N, C=C, and C-O-C bonds. Finally, we compared the humidity stability of PSCs before and after DiOC2(3) modification in a nitrogen glove box environment (25°C). As shown in Figure 4F, the DiOC2(3)-modified device maintains 95% of its initial efficiency after 500 h, whereas the control device drops down 50% of its initial efficiency after 400 h. Noteworthy that the light stability and thermal stability are shown in Supplementary Figure S8, Supporting Information. It is observed that the control devices exhibit rapid degradation after working for 200 h, while the DiOC2(3)-modified devices show excellent light stability and thermal stability. These results indicate that PSCs with DiOC2(3)-modified film possess better stability.
Conclusion
In this study, the DiOC2(3) with a central symmetric structure and additional absorption in the ultraviolet band, is incorporated into the WBG perovskite precursor solution to passivate the. Pb vacancies and ion vacancy defects. The synergistic passivation effect of multiple functional group sites. could inhibit ion migration while reducing the density of defect states, improving the extraction and transport capacity of charge carriers, and achieving a lower Voc loss. The difference in electronegativity between carbon and oxygen atoms in ether bonds strongly improves the stability of the perovskite thin films. Therefore, the WBG PSCs modified with DiOC2(3) exhibit a PCE of 21.50%, with a Voc loss of 0.487 V. It is worth noting that the unpackaged device maintains 95% of its initial PCE in a N2 environment (25°C) for 500 h. Our study provides a simple and effective method for preparing high-performance, low Voc-loss, and stable PSCs.
Experimental section
Materials: N, N-dimethylformamide (DMF, 99.8%), dimethyl sulfoxide (DMSO, 99.9%) were purchased from Sigma-Aldrich. Lead iodide (PbI2, 99.999%), lead bromide (PbBr2, 99.99%), Cesium iodide (CsI, 99.99%), Methylammonium bromide (MABr, 99.99%), holeblocking material 2,9-dimethyl-4,7-diphenyl-1,10-phenanthroline (BCP, 99.5%) were purchased from Xi’an Yuri Solar Co., Ltd. Formamidinium iodide (H2N = CHNH2I; FAI), [6,6]-phenyl-C61-butyric acid methyl ester (PCBM, 99.9%) was purchased from Advanced Election Technology Co., Ltd. Indium Tin Oxide (ITO coated glass, square resistance 15 Ω) is purchased from Advanced Election Technology Co., Acid (Meo-2PACz) is purchased from TCI. 3,3′-diethyl-oxacarbo-cyanine iodide (DiOC2(3), 99.7%) was purchased from Sigma-Aldrich. Other materials were purchased from Alfa Aesar. All salts and solvents were used as received without any further purification.
Wide-bandgap perovskite precursor preparation: A 1.50 M Cs0.05(FA0.77MA0.23)0.95Pb(I0.77Br0.23)3 wide-bandgap perovskite precursor was prepared by adding 19.48 mg of CsI, 188.69 mg of FAI, 36.70 mg of MABr, 532.47 mg of PbI2, 126.62 mg of PbBr2 and with 2% PbX2 excess, respectively(X = I or Br) in an alloyed solvent of DMF and DMSO with a volume ratio of 4:1. Then, the solutions were stirred 60 min at 60°C temperature in a N2 glovebox. For the perovskite with DiOC2(3) additive, DiOC2(3) was added at volume ratio 150:1 (perovskite: DiOC2(3)). Before preparing perovskite films, the precursor solutions were filtered through a 0.45 mm polytetrafluoroethylene (PTFE) filter.
Fabrication of single junction wide-bandgap perovskite device: The ITO glass substrates were sequentially cleaned with cleanser, isopropanol, deionized water, and ethyl alcohol in a sonication bath for 15 min. Then we treated the dried substrates by UV Ozone for 30 min before use. For using MeO-2PACz as a hole transportation layer, the solution in ethanol with concentration of 1.0 mg/mL was spin-coated at 5,000 rpm for 30s and annealed at 100°C for 10 min. For the WBG perovskites, 50 μL perovskite solution was uniformly dropped on the HTL by spin-coated at 1,100 rpm for 2 s, and 6,000 rpm for 35 s; In the last 10 s of spin coating, 150 μL of CB was dropped on the spinning substrates. Then, the perovskite film was immediately placed on a hot plate and annealed at 100°C for 30 min. Then, PCBM (20 mg/mL in chlorobenzene) and BCP (1 mg/ml in saturated ethanol solution) were spin-coated with spinning speeds of 1,500 rpm for 50 s and 6,000 rpm for 25 s, respectively. Lastly, 120 nm Ag was evaporated under high vacuum (<8 × 10−4 Pa) on the substrates to form electrodes.
Device characterization: Fourier transform infrared spectroscopy (FTIR) measurements were performed on a Nicolet iS50 Infrared Fourier transform microscope by Thermo-Fisher Scientific (America). J−V curves were measured using a solar simulator equipped with a 150 W xenon lamp and a Keithley 2,420 source meter. X-ray diffractometer (XRD, D8 Advance, Bruker). Atomic force microscopy (AFM) was conducted on a Bruker Dimension Icon (United States). Scanning electron microscopy (SEM) images were collected by employing a field-emission scanning electron microscope (Tescan MIRA LMS, Czech Republic). Ultraviolet−visible (UV−vis) absorption spectra were measured with a UV−vis spectrometer (UV 2600 Shimadzu, Japan). The steady-state photoluminescence (PL) and time-resolved photoluminescence (TRPL) spectra were measured using a DeltaFlex fluorescence spectrometer (HORIBA). X-ray photoelectron spectroscopy (XPS) spectra were measured using an XPS/UPS system (Thermo Scientific, Escalab 250Xi).
Data availability statement
The original contributions presented in the study are included in the article/Supplementary Material, further inquiries can be directed to the corresponding authors.
Author contributions
XY: Writing–original draft, Data curation, Formal Analysis, Software, Writing–review and editing. HH: Writing–review and editing. YH: Writing–review and editing. HW: Writing–review and editing. XiZ: Writing–review and editing. SL: Writing–review and editing. TZ: Writing–review and editing.
Funding
The author(s) declare that financial support was received for the research, authorship, and/or publication of this article. The authors acknowledge the National Natural Science Foundation of China (Grant no. 62204103), the Yunnan Fundamental Research Projects (Grant no. 202101BE070001-007), Yunnan Major Scientific and Technological Projects (Grant no. 202202AG050017-1) for financial support, and acknowledgments Financial support for this work from the Major Science and Technology Projects in Yunnan Province (No.202202AB080010, 202202AG050012); Yunnan Fundamental Research Projects (grant NO. 202201AW070014) and the Program for Innovative Research Team in University of Ministry of Education of China (No. IRT_17R48).
Conflict of interest
The authors declare that the research was conducted in the absence of any commercial or financial relationships that could be construed as a potential conflict of interest.
Publisher’s note
All claims expressed in this article are solely those of the authors and do not necessarily represent those of their affiliated organizations, or those of the publisher, the editors and the reviewers. Any product that may be evaluated in this article, or claim that may be made by its manufacturer, is not guaranteed or endorsed by the publisher.
Supplementary material
The Supplementary Material for this article can be found online at: https://www.frontiersin.org/articles/10.3389/fchem.2024.1441057/full#supplementary-material
References
An, Z., Chen, S., Lu, T., Zhao, P., Yang, X., Li, Y., et al. (2023). Interfacial modification via aniline molecules with multiple active sites for performance enhancement of n-i-p perovskite solar cells. J. Mater. Chem. C 11 (37), 12750–12758. doi:10.1039/d3tc02144k
Azmi, R., Ugur, E., Seitkhan, A., Aljamaan, F., Subbiah, A. S., Liu, J., et al. (2022). Damp heat-stable perovskite solar cells with tailored-dimensionality 2D/3D heterojunctions. Science 376 (6588), 73–77. doi:10.1126/science.abm5784
Bai, S., Da, P. M., Li, C., Wang, Z. P., Yuan, Z. C., Fu, F., et al. (2019). Planar perovskite solar cells with long-term stability using ionic liquid additives. Nature 571 (7764), 245–250. doi:10.1038/s41586-019-1357-2
Best (2024). NREL. Best research-cell efficiency chart. Available at: www.nrel.gov/best-research-cell-efficiencies.
Cao, Q., Li, Y., Zhang, Y., Zhao, J., Wang, T., Yang, B., et al. (2022). N-type conductive small molecule assisted 23.5% efficient inverted perovskite solar cells. Adv. Energy Mater. 12 (34). doi:10.1002/aenm.202201435
Chen, H., Maxwell, A., Li, C., Teale, S., Chen, B., Zhu, T., et al. (2023). Regulating surface potential maximizes voltage in all-perovskite tandems. Nature 613 (7945), 676–681. doi:10.1038/s41586-022-05541-z
Chen, H., Teale, S., Chen, B., Hou, Y., Grater, L., Zhu, T., et al. (2022). Quantum-size-tuned heterostructures enable efficient and stable inverted perovskite solar cells. Nat. Photonics 16 (5), 352–358. doi:10.1038/s41566-022-00985-1
Chen, X., Geng, C., Yu, X., Feng, Y., Liang, C., Peng, Y., et al. (2023). Additive engineering in spray enables efficient methylammonium-free wide-bandgap perovskite solar cells. Mater. Today Energy 34, 101316. doi:10.1016/j.mtener.2023.101316
Chen, X., Liu, J., Wu, J., Li, G., Chen, Q., Cai, F., et al. (2022). Polyethylene glycol dodecyl ether as interfacial modifier for improving efficiency of perovskite solar cells. Energy Technol. 10 (11). doi:10.1002/ente.202200761
Dai, Y., Ge, X., Shi, B., Wang, P., Zhao, Y., and Zhang, X. (2024). Enhancing ultraviolet stability and performance of wide bandgap perovskite solar cells through ultraviolet light-absorbing passivator. Small Methods, e2301793. doi:10.1002/smtd.202301793
Dong, J., Peng, Y., Long, J., Zhang, Y., Wang, Z., Park, S., et al. (2023). An all-stretchable, ultraviolet protective, and electromagnetic-interference-free E-textile. Adv. Funct. Mater. 33 (45). doi:10.1002/adfm.202308426
Du, Y., Tian, Q., Chang, X., Fang, J., Gu, X., He, X., et al. (2022). Ionic liquid treatment for highest-efficiency ambient printed stable all-inorganic CsPbI3 perovskite solar cells. Adv. Mater. 34 (10), e2106750. doi:10.1002/adma.202106750
Eperon, G. E., Stranks, S. D., Menelaou, C., Johnston, M. B., Herz, L. M., and Snaith, H. J. (2014). Formamidinium lead trihalide: a broadly tunable perovskite for efficient planar heterojunction solar cells. Energy Environ. Sci. 7 (3), 982–988. doi:10.1039/c3ee43822h
Gan, Y., Hao, X., Li, W., Zhang, J., and Wu, L. (2023). Additive combining passivator for inverted wide-bandgap perovskite solar cells with 22% efficiency and reduced voltage loss. Sol. Rrl 7 (24). doi:10.1002/solr.202300519
Guan, H., Zhou, S., Fu, S., Pu, D., Chen, X., Ge, Y., et al. (2024). Regulating crystal orientation via ligand anchoring enables efficient wide-bandgap perovskite solar cells and tandems. Adv. Mater. 36 (1), e2307987. doi:10.1002/adma.202307987
Guo, H., Fang, Y., Lei, Y., Wu, J., Li, M., Li, X., et al. (2023). Mitigating ion migration with an ultrathin self-assembled ionic insulating layer affords efficient and stable wide-bandgap inverted perovskite solar cells. Small 19 (38), e2302021. doi:10.1002/smll.202302021
He, R., Wang, W., Yi, Z., Lang, F., Chen, C., Luo, J., et al. (2023). Improving interface quality for 1-cm2 all-perovskite tandem solar cells. Nature 618 (7963), 80–86. doi:10.1038/s41586-023-05992-y
Hoke, E. T., Slotcavage, D. J., Dohner, E. R., Bowring, A. R., Karunadasa, H. I., and McGehee, M. D. (2015). Reversible photo-induced trap formation in mixed-halide hybrid perovskites for photovoltaics. Chem. Sci. 6 (1), 613–617. doi:10.1039/C4SC03141E
Hu, X., Li, J., Wang, C., Cui, H., Liu, Y., Zhou, S., et al. (2023). Antimony potassium tartrate stabilizes wide-bandgap perovskites for inverted 4-T all-perovskite tandem solar cells with efficiencies over 26%. Nano-Micro Lett. 15 (1), 103. doi:10.1007/s40820-023-01078-6
Jayanthi, K., Spanopoulos, I., Zibouche, N., Voskanyan, A. A., Vasileiadou, E. S., Islam, M. S., et al. (2022). Entropy stabilization effects and ion migration in 3D “hollow” halide perovskites. J. Am. Chem. Soc. 144 (18), 8223–8230. doi:10.1021/jacs.2c01383
Jiang, Q., Tong, J., Scheidt, R. A., Wang, X., Louks, A. E., Xian, Y., et al. (2022). Compositional texture engineering for highly stable wide-bandgap perovskite solar cells. Science 378 (6626), 1295–1300. doi:10.1126/science.adf0194
Kim, D. H., Muzzillo, C. P., Tong, J., Palmstrom, A. F., Larson, B. W., Choi, C., et al. (2019). Bimolecular additives improve wide-band-gap perovskites for efficient tandem solar cells with CIGS. Joule 3 (7), 1734–1745. doi:10.1016/j.joule.2019.04.012
Kim, H., Lim, K. G., and Lee, T. W. (2016). Planar heterojunction organometal halide perovskite solar cells: roles of interfacial layers. Energy Environ. Sci. 9 (1), 12–30. doi:10.1039/c5ee02194d
Kim, S., Walsh, A., Rho, S., and Yoon, S. (2021). Measurements of acoustic radiation force of ultrahigh frequency ultrasonic transducers using model-based approach. Appl. Phys. Lett. 118 (24), 184102. doi:10.1063/5.0044512
Kojima, A., Teshima, K., Shirai, Y., and Miyasaka, T. (2009). Organometal halide perovskites as visible-light sensitizers for photovoltaic cells. J. Am. Chem. Soc. 131 (17), 6050–6051. doi:10.1021/ja809598r
Lamanna, E., Matteocci, F., Calabro, E., Serenelli, L., Salza, E., Martini, L., et al. (2020). Mechanically stacked, two-terminal graphene-based perovskite/silicon tandem solar cell with efficiency over 26%. Joule 4 (4), 865–881. doi:10.1016/j.joule.2020.01.015
Leijtens, T., Bush, K. A., Prasanna, R., and McGehee, M. D. (2018). Opportunities and challenges for tandem solar cells using metal halide perovskite semiconductors. Nat. Energy 3 (10), 828–838. doi:10.1038/s41560-018-0190-4
Li, S., Zheng, Z., Ju, J., Cheng, S., Chen, F., Xue, Z., et al. (2023). A generic strategy to stabilize wide bandgap perovskites for efficient tandem solar cells. Adv. Mater. 36, e2307701. doi:10.1002/adma.202307701
Li, T., Xu, J., Lin, R., Teale, S., Li, H., Liu, Z., et al. (2023). Inorganic wide-bandgap perovskite subcells with dipole bridge for all-perovskite tandems. Nat. Energy 8 (6), 610–620. doi:10.1038/s41560-023-01250-7
Liu, L., Farhadi, B., Li, J., Liu, S., Lu, L., Wang, H., et al. (2024). Hydrophobic hydrogen-bonded polymer network for efficient and stable perovskite/Si tandem solar cells. Angew. Chemie-International Ed. 63 (8), e202317972. doi:10.1002/anie.202317972
Luo, X., Luo, H., Li, H., Xia, R., Zheng, X., Huang, Z., et al. (2023). Efficient perovskite/silicon tandem solar cells on industrially compatible textured silicon. Adv. Mater. 35 (9), e2207883. doi:10.1002/adma.202207883
Min, H., Lee, D., Kim, J., Kim, G., Lee, K. S., Kim, J., et al. (2021). Perovskite solar cells with atomically coherent interlayers on SnO2 electrodes. Nature 598 (7881), 444–450. doi:10.1038/s41586-021-03964-8
Ren, Y., He, L., Zhang, B., Li, T., Yuan, Y., Zhang, J., et al. (2022). A trifluorothymine interlayer reduces the degradation of perovskite and controls the cracks of hole transport layers. J. Mater. Chem. A 10 (30), 16080–16086. doi:10.1039/d2ta03601k
Saliba, M., Correa-Baena, J.-P., Gratzel, M., Hagfeldt, A., and Abate, A. (2018). Perovskite solar cells: from the atomic level to film quality and device performance. Angew. Chemie-International Ed. 57 (10), 2554–2569. doi:10.1002/anie.201703226
Shao, W., Wang, H., Fu, S., Ge, Y., Guan, H., Wang, C., et al. (2024). Tailoring perovskite surface potential and chelation advances efficient solar cells. Adv. Mater. 36, e2310080. doi:10.1002/adma.202310080
Shen, J., Li, N., Wang, Y., Ge, X., Tao, J., Yin, S., et al. (2024). Delaying crystallization and anchoring the grain boundaries defects via π-π stacked molecules for efficient and stable wide-bandgap perovskite solar cells. Chem. Eng. J. 489, 151459. doi:10.1016/j.cej.2024.151459
Song, J., Hu, Q., Zhang, Q., Xiong, S., Zhao, Z., Ali, J., et al. (2021). Manipulating the crystallization kinetics by additive engineering toward high-efficient photovoltaic performance. Adv. Funct. Mater. 31 (14). doi:10.1002/adfm.202009103
Stolterfoht, M., Caprioglio, P., Wolff, C. M., Marquez, J. A., Nordmann, J., Zhang, S., et al. (2019). The impact of energy alignment and interfacial recombination on the internal and external open-circuit voltage of perovskite solar cells. Energy Environ. Sci. 12 (9), 2778–2788. doi:10.1039/c9ee02020a
Wang, C., Zhao, Y., Ma, T., An, Y., He, R., Zhu, J., et al. (2022). A universal close-space annealing strategy towards high-quality perovskite absorbers enabling efficient all-perovskite tandem solar cells. Nat. Energy 7 (8), 744–753. doi:10.1038/s41560-022-01076-9
Wang, R., Zhu, J., You, J., Huang, H., Yang, Y., Chen, R., et al. (2024). Custom-tailored solvent engineering for efficient wide-bandgap perovskite solar cells with a wide processing window and low VOC losses. Energy Environ. Sci. 17 (7), 2662–2669. doi:10.1039/d4ee00330f
Wu, W., Xu, H., Liu, G., Zheng, H., and Pan, X. (2021). Introduction of 4-hydroxybenzaldehyde as interface modifier with multidimensional defects passivation effect for high-performance perovskite solar cells. Appl. Surf. Sci. 570, 151259. doi:10.1016/j.apsusc.2021.151259
Zhang, F., Bi, D., Pellet, N., Xiao, C., Li, Z., Berry, J. J., et al. (2018). Suppressing defects through the synergistic effect of a Lewis base and a Lewis acid for highly efficient and stable perovskite solar cells. Energy Environ. Sci. 11 (12), 3480–3490. doi:10.1039/c8ee02252f
Zhang, J., Niu, X., Peng, C., Jiang, H., Yu, L., Zhou, H., et al. (2023). Inhibiting ion migration through chemical polymerization and chemical chelation toward stable perovskite solar cells. Angew. Chemie-International Ed. 62 (50), e202314106. doi:10.1002/anie.202314106
Zhang, K., Feng, Y., Tang, H., Zhang, X., Li, T., Hu, D., et al. (2023). Stable and efficient inverted perovskite solar cells enabled by structural design of Lewis base molecules. Acs Appl. Energy Mater. 6 (18), 9276–9286. doi:10.1021/acsaem.3c01126
Zhao, Y., Wang, C., Ma, T., Zhou, L., Wu, Z., Wang, H., et al. (2023). Reduced 0.418 V VOC-deficit of 1.73 eV wide-bandgap perovskite solar cells assisted by dual chlorides for efficient all-perovskite tandems. Energy Environ. Sci. 16 (5), 2080–2089. doi:10.1039/d2ee04087e
Zheng, H., Wu, W., Xu, H., Zheng, F., Liu, G., Pan, X., et al. (2020). Self-additive low-dimensional ruddlesden-popper perovskite by the incorporation of Glycine hydrochloride for high-performance and stable solar cells. Adv. Funct. Mater. 30 (15). doi:10.1002/adfm.202000034
Zheng, Y., Wu, X., Liang, J., Zhang, Z., Jiang, J., Wang, J., et al. (2022). Downward homogenized crystallization for inverted wide-bandgap mixed-halide perovskite solar cells with 21% efficiency and suppressed photo-induced halide segregation. Adv. Funct. Mater. 32 (29). doi:10.1002/adfm.202200431
Keywords: wide band gap perovskite, open-circuit voltage losses, iodide, defects, high performance
Citation: Yu X, He H, Hui Y, Wang H, Zhu X, Li S and Zhu T (2024) Additive engineering for efficient wide-bandgap perovskite solar cells with low open-circuit voltage losses. Front. Chem. 12:1441057. doi: 10.3389/fchem.2024.1441057
Received: 30 May 2024; Accepted: 22 August 2024;
Published: 02 September 2024.
Edited by:
Yang Wang, Fujian Normal University, ChinaReviewed by:
Xiaohui Liu, Ningbo University, ChinaJunsheng Luo, University of Electronic Science and Technology of China, China
Copyright © 2024 Yu, He, Hui, Wang, Zhu, Li and Zhu. This is an open-access article distributed under the terms of the Creative Commons Attribution License (CC BY). The use, distribution or reproduction in other forums is permitted, provided the original author(s) and the copyright owner(s) are credited and that the original publication in this journal is cited, in accordance with accepted academic practice. No use, distribution or reproduction is permitted which does not comply with these terms.
*Correspondence: Tao Zhu, emh1dGFvMzMwNkAxNjMuY29t; Shaoyuan Li, bHN5NDE1ODA4NTUwQDE2My5jb20=