- International Centre for Quantum and Molecular Structures, Department of Physics, College of Sciences, Shanghai University, Shanghai, China
The James Webb Space Telescope (JWST) opened a new era for the identification of molecular systems in the interstellar medium (ISM) by vibrational features. One group of molecules of increasing interest is cyano-derivatives of aromatic organic molecules, which have already been identified in the ISM on the basis of the analysis of rotational signatures, and so, are plausible candidates for the detection by the JWST. Benzonitrile considered in this work represents a suitable example for the validation of a computational strategy, which can be further applied for different, larger, and not-yet observed molecules. For this purpose, anharmonic simulations of infrared (IR) spectra have been compared with recent FTIR experimental studies. The anharmonic computations using the generalized second-order vibrational perturbation theory (GVPT2) in conjunction with a hybrid force field combining the harmonic part of revDSD-PBEP86-D3/jun-cc-pVTZ with anharmonic corrections from B3LYP-D3/SNSD show very good agreement with those in the experiment, with a mean error of
1 Introduction
Despite being initially considered too harsh and diluted for molecule creation and survival, it is now recognized that several chemical processes can happen in astronomic environments leading to more than 310 molecules already detected in the interstellar medium (ISM) or circumstellar shells (Endres et al., 2016). Several of these molecules have been detected in the last decade, in particular, a number of complex organic species (COMs), defined as astrophysically relevant organic molecules consisting of six or more atoms. Some COMs that contain C, H, N, O, and possibly also S atoms can be claimed as prebiotic (Fulvio et al., 2021). To date, more than 30 prebiotic molecules have been detected in Taurus molecular cloud 1 (TMC-1), a dust-enshrouded gaseous cloud located 400 light-years from the Sun in the Taurus constellation. Such progress and fast increase in new detections has become possible due to advances in instrumentation, in particular the sub-millimeter and radio domains, allowing the analysis of the lowest rotational lines where radiation can pass through dust-enshrouded clouds (Guélin and Cernicharo, 2022).
However, rotational transitions are not always suitable and accessible for a study, for instance, for exoplanet atmospheres or dense dark regions, for which vibrational spectroscopies are often the methods of choice. The increasing importance of vibrational transitions in astrochemical context is clearly represented by the James Webb Space Telescope (JWST), which, with the infrared (IR) (Frost et al., 2022) observations obtained by the mid-infrared (MIR) and near-IR (NIR) instruments integrated within the Integrated Science Instrument Module (ISIM, Near-Infrared Camera [NIRCam], Near-Infrared Spectrograph [NIRSpec], Mid-Infrared Instrument [MIRI], and Jet Propulsion Laboratory [JPL]), already provides superlative sensitivity, spectral resolution, and wavelength coverage compared to previous space telescopes, such as Herschel, that observe in the visible and ultraviolet spectra (see Figure 1). These emerging experimental data already provide new information about the major ices in molecular cloud cores just prior to their collapse to form protostars (McClure et al., 2023). Currently, the Mars 2020 Perseverance rover also searches for signs of organic matter, in the contexts of the emergence of life, as well as its consideration as a habitable planet, performing, among others, NIR studies using SuperCam (Perez et al., 2017; Eigenbrode et al., 2018; Lasne, 2021; Sharma et al., 2023; McIntosh et al., 2024). These missions yield a huge amount of new significant results, which need to be analyzed, also highlighting the urgent need for accurate reference data in the MIR-to-NIR (0.6–28
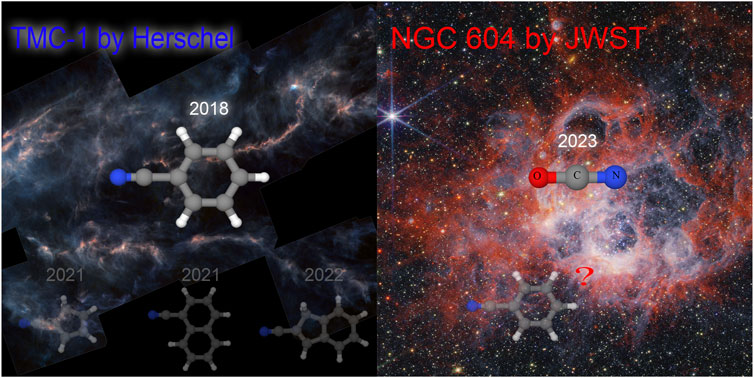
Figure 1. Cyano-derivatives in the ISM and the year of detection by rotational (left panel) or vibrational (right panel) features. Left panel: astro- COMs and Herschel’s view of TMC-1 (Herschel Space Observatory, 2017) (credit: ESA/Herschel/NASA/JPL-Caltech TMC-1). Right panel:
Spectroscopic techniques are the key for the analysis of astronomical observations and the detection of molecules in the interstellar medium and other astrochemical environments, such as atmospheres or soils of exoplanets or planetary moons (Loru et al., 2022; Lemmens et al., 2022; Mackie et al., 2022; Peeters et al., 2021; K. Lemmens et al., 2023; Petrignani and Candian, 2022; Puzzarini et al., 2023). However, the investigation of the chemical composition of “astrochemical samples” is complicated due to the concomitant presence of many, possibly unknown species. The detection of molecules is based on the comparison of spectra from spectroscopic observations in space with reference experiments from the laboratory (Puzzarini et al., 2023). Unfortunately, the latter might be limited, incomplete, or difficult to obtain under appropriate conditions (Barone et al., 2015c; Fortenberry, 2024b). The optimal strategy is represented by the combination of experiments with the theoretical approaches (Barone et al., 2021; Puzzarini, 2022), supporting and/or complementing laboratory studies (Biczysko et al., 2018a; Barone and Puzzarini, 2023; Fortenberry, 2024a). An increasing role of computational spectroscopy is related to its increasing accuracy (Yang et al., 2021; Barone and Puzzarini, 2023), as well as the possibility to match different, often extreme environmental conditions possible in space (Barone et al., 2015c; Biczysko et al., 2018b; Zapata Trujillo et al., 2023; Fortenberry, 2024b).
Among all the molecules detected to date in the ISM, over 30% bear a nitrogen atom, which usually bonds to carbon, in a large fraction (over 80% of species) by the triple C
In this work, we focus on the first observed cyano-astroCOM, i.e., benzonitrile (McGuire et al., 2018). The experimental microwave spectrum of benzonitrile was first studied simultaneously by Erlandsson (1954) and Lide (1954). Bak et al. conducted comprehensive analyses of benzonitrile utilizing infrared and centimeter-wave techniques, also including feasible mono-substituted isotopologs (Bak and Nielsen, 1960; Bak et al., 1962). This led to the first derivation of a substitution structure,
This work focuses on the IR spectra in the MIR-NIR region, first comparing the simulated infrared spectra with the available experimental counterparts and then providing the prediction of not yet available spectral data, which can support either laboratory or astrochemical studies.
2 Computational details
In order to simulate the spectroscopic parameters of benzonitrile in its electronic ground state
Computations of the third- and fourth-order derivatives of the potential energy surface have been performed at the B3LYP level by the numerical differentiation (Barone, 2005; Bloino, 2015) of analytic second-order derivatives, while the cubic electric dipole moment surfaces have been obtained through numerical differentiations of the dipole moment derivatives. The revDSD equilibrium and harmonic computations have been combined with B3LYP anharmonic computations to create a hybrid model used in spectroscopic simulations. The consistency of these two sets of data has been checked automatically, as implemented in GAUSSIAN 16. In other words, the overlap between two sets of normal modes (two different levels of theory) is defined using the linear transformation, as proposed by Duschinsky (1937):
where
This cost-effective (Xu et al., 2024) hybrid revDSD/B3LYP scheme has been further used to compute spectroscopic parameters using the second-order vibrational perturbation theory (VPT2) (Nielsen, 1951; Mills, 1972). The ground vibrational state rotational constants have been obtained from the revDSD equilibrium structure by adding vibrational corrections computed at the revDSD/B3LYP level, which also provided data allowing the determination of the quartic and sextic centrifugal-distortion constants (Puzzarini et al., 2010; Puzzarini, 2013). For the vibrational spectra, it is also necessary to account for the possible presence of anharmonic resonances (Amos et al., 1991; Martin et al., 1995; Barone, 2005; Vázquez and Stanton, 2007; Rosnik and Polik, 2014; Barone et al., 2014; Bloino et al., 2015; Bloino, 2015; Krasnoshchekov et al., 2015; Franke et al., 2021; Mendolicchio et al., 2021; Franke et al., 2021) by the generalized VPT2 (GVPT2) model (Bloino and Barone, 2012; Bloino et al., 2015), where nearly resonant contributions are removed from the perturbative treatment (leading to the deperturbed model, DVPT2) and treated in a second step variationally. Resonance definition and general recommendations on the applied computational procedures are described in detail in the tutorial review by Bloino et al. (2016). It should be noted that, although improved criteria to define automatic resonances have been proposed recently, they would have negligible impacts on the energies (Yang and Bloino, 2022). Overall, the GVPT2 scheme employed in this work has been successfully applied to medium-sized or larger biomolecules with up to 100 atoms (Fornaro et al., 2015; Fusè et al., 2019; Yatsyna et al., 2019; Green and Improta, 2020; Yang et al., 2021; Sheng et al., 2021), also in the astrochemical context (Zhao et al., 2021; McIntosh et al., 2024; Alberini et al., 2024), so it is a valuable tool to be employed also for significantly larger cyano-astroCOMs.
3 Results and discussion
3.1 Equilibrium structure and rotational parameters
Selected equilibrium structural parameters of benzonitrile calculated at the revDSD/junTZ level are shown in Figure 2, while Table 1 compares all equilibrium parameters with the semi-experimental equilibrium structure
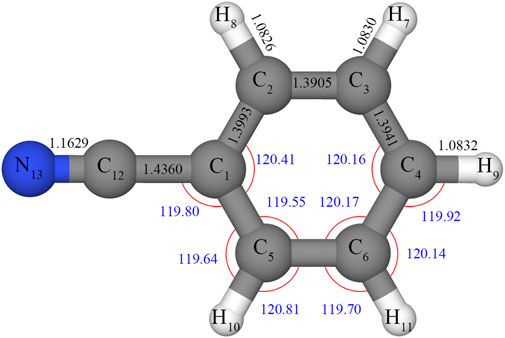
Figure 2. Structural parameter of benzonitrile as computed at the rDSD/junTZ level, with bond lengths (black) in Å and angles (blue) in degrees.
The final validation is provided by the direct comparison with the experiment, i.e., spectroscopic constants from Watson’s asymmetric rotor Hamiltonian (A-reduction,
3.2 Vibrational properties and IR spectra
Table 3 compares harmonic vibrational wavenumbers and IR intensities with those computed at the CCSD(T)/ANO1 level, showing very good agreement with the average error of approximately 6
Table 4 lists all fundamental anharmonic wavenumbers and IR intensities of benzonitrile computed at the rDSD/junTZ//B3PLYP/SNSD GVPT2 level, while selected overtones and combination bands are given in Table 5. The accuracy of the simulated IR spectra of benzonitrile in the 500
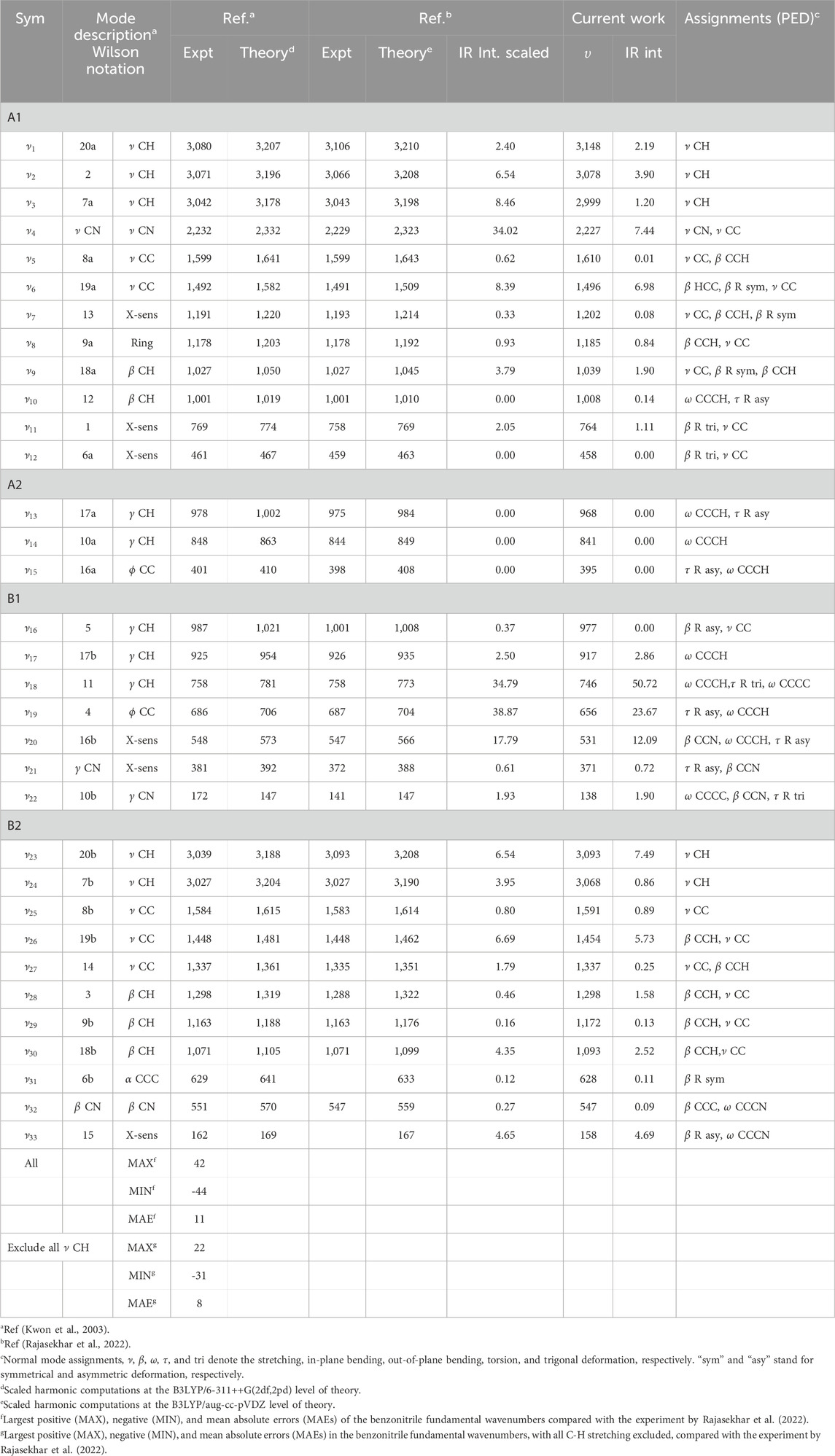
Table 4. Fundamental wavenumbers
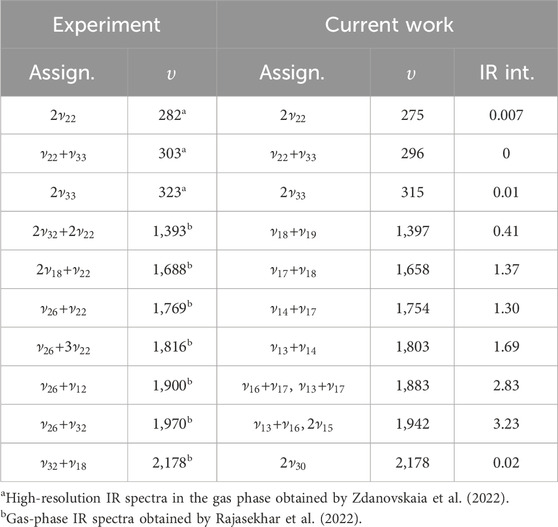
Table 5. Non-fundamental band wavenumbers
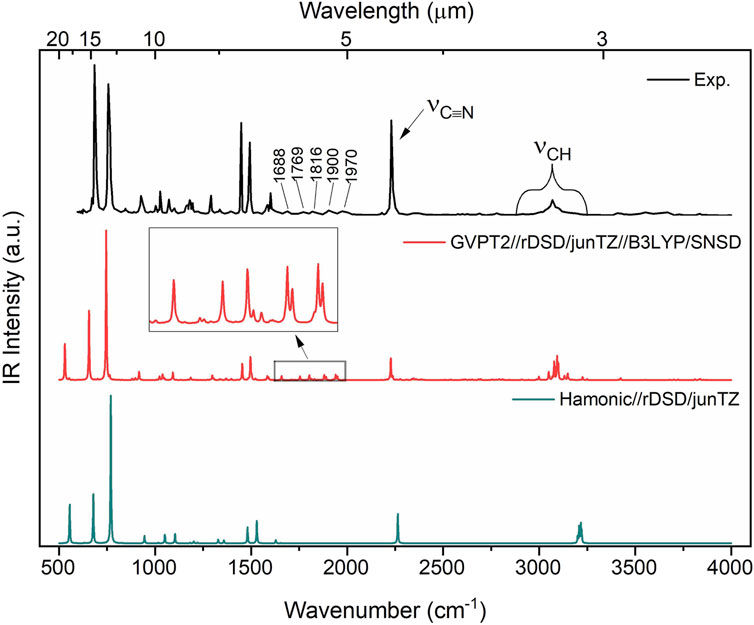
Figure 3. IR spectrum of benzonitrile in the range 500–4,000
A direct comparison between the spectra given in Figure 3 highlights that the GVPT2 computation not only correctly predicts fundamental bands but also a pattern of five distinct non-fundamental bands in the
Overall, the good accuracy of our simulations, for both fundamental and non-fundamental transitions, allows us to predict the spectra in the NIR region, which is shown in Figures 4A and B for
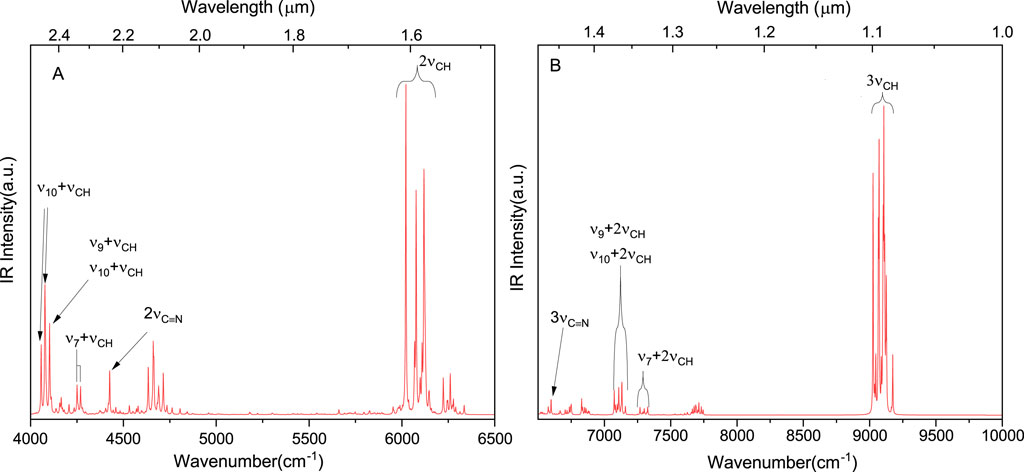
Figure 4. NIR spectra simulated at the GVPT2//rDSD/junTZ//B3LYP/SNSD level. The spectra were broadened by Lorentzian functions with HWHM of 2
4 Conclusion and astrochemical implications
Good accuracy of simulated MIR spectra, confirmed by a comparison with available experimental results, allowed us to provide predictions regarding the “missing” data on relevance for the astrochemical observations, in particular, concerning the NIR region.
The availability of NIR references is important for the interpretation of data collected during the Mars 2020 (Williford et al., 2018) space mission by instruments such as SuperCam, for incoming ExoMars 2022 (ESZ-Roscosmos) (Vago et al., 2017), as well as for the JWST observations by the NIRSpec (McClure et al., 2023). The advantage of NIR is its lower spectrum congestion than MIR, which is also clearly visible in Figures 3, 4. However, these reference NIR data are often very scarce and were not available even for benzonitrile prior to this work. It has been already highlighted that anharmonic computations provide significant support in the analysis of experimental results and identification of plausible molecules by NIR features (Fornaro et al., 2020; Alberini et al., 2024).
Computational spectroscopy can also support the identification of B
Extensive laboratory experimental investigation of the photoabsorption spectra of benzonitrile recorded using synchrotron radiation in
The most relevant are those based on aromatic systems, such as 1- and 2-cyanocyclopentadiene (McCarthy et al., 2021; Lee et al., 2021), and 1- and 2-cyanonaphthalene (McGuire et al., 2021), which have been already discovered in TMC-1. However, it can be expected that similar accuracy can also be obtained for aliphatic systems, for which several conformers can be present (Barone et al., 2013; 2015b). This situation was highlighted by the recent discovery of five cyano-derivatives of propene (
Data availability statement
The original contributions presented in the study are included in the article/Supplementary Material; further inquiries can be directed to the corresponding author.
Author contributions
YX: formal analysis, investigation, validation, writing–original draft, writing–review and editing, data curation, and visualization. MB: formal analysis, investigation, validation, writing–original draft, writing–review and editing, conceptualization, funding acquisition, methodology, project administration, resources, and supervision.
Funding
The author(s) declare that financial support was received for the research, authorship, and/or publication of this article. This work was performed within COST Action CA21101 “Confined molecular systems: from a new generation of materials to the stars” (COSY) supported by COST (European Cooperation in Science and Technology). This work was supported by the National Natural Science Foundation of China (Grant No. 31870738).
Acknowledgments
MB acknowledges support from the COST Action “COSY,” in particular, discussions with Action Chair Prof. María Pilar de Lara-Castells, Action Vice-Chair Prof. Cristina Puzzarini, and WG5 “Confined Systems in Astrochemistry” Leader Prof. Lauri Halonen. MB and XY thank Prof. Julien Bloino for fruitful discussions on anharmonic computations.
Conflict of interest
The authors declare that the research was conducted in the absence of any commercial or financial relationships that could be construed as a potential conflict of interest.
The author(s) declared that they were an editorial board member of Frontiers, at the time of submission. This had no impact on the peer review process and the final decision.
Publisher’s note
All claims expressed in this article are solely those of the authors and do not necessarily represent those of their affiliated organizations, or those of the publisher, the editors, and the reviewers. Any product that may be evaluated in this article, or claim that may be made by its manufacturer, is not guaranteed or endorsed by the publisher.
Supplementary material
The Supplementary Material for this article can be found online at: https://www.frontiersin.org/articles/10.3389/fchem.2024.1439194/full#supplementary-material
References
Alberini, A., Fornaro, T., García-Florentino, C., Biczysko, M., Poblacion, I., Aramendia, J., et al. (2024). Investigating the stability of aromatic carboxylic acids in hydrated magnesium sulfate under uv irradiation to assist detection of organics on mars. Sci. Rep. 14, 15945. doi:10.1038/s41598-024-66669-8
Amos, R. D., Handy, N. C., Green, W. H., Jayatilaka, D., Willetts, A., and Palmieri, P. (1991). Anharmonic vibrational properties of ch2f2: a comparison of theory and experiment. J. Chem. Phys. 95, 8323–8336. doi:10.1063/1.461259
Arumainayagam, C. R., Herbst, E., Heays, A. N., Mullikin, E., Farrah, M., and Mavros, M. G. (2021). “Extraterrestrial photochemistry: principles and applications,” in Prebiotic photochemistry: from urey–miller-like experiments to recent findings. Editors F. Saija, and G. Cassone (Cambridge, UK: The Royal Society of Chemistry), 9–36. doi:10.1039/9781839164354-00009
Bak, B., Christensen, D., Dixon, W. B., Hansen-Nygaard, L., and Rastrup-Andersen, J. (1962). Benzene ring distortion by one substituent. Microwave determination of the complete structure of benzonitrile. J. Chem. Phys. 37, 2027–2031. doi:10.1063/1.1733422
Bak, Bø., and Nielsen, J. T. (1960). Infrared absorption spectra of benzonitrile and its monodeuterated derivatives: tentative assignment of fundamentals. Z. Elektrochem. Ber. Bunsenges. Phys. Chem. 64, 560–562. doi:10.1002/bbpc.196000003
Barone, V. (2005). Anharmonic vibrational properties by a fully automated second-order perturbative approach. J. Chem. Phys. 122, 014108. doi:10.1063/1.1824881
Barone, V., Alessandrini, S., Biczysko, M., Cheeseman, J. R., Clary, D. C., McCoy, A. B., et al. (2021). Computational molecular spectroscopy. Nat. Rev. Methods Prim. 1, 38–27. doi:10.1038/s43586-021-00034-1
Barone, V., Biczysko, M., and Bloino, J. (2014). Fully anharmonic ir and Raman spectra of medium-size molecular systems: accuracy and interpretation. Phys. Chem. Chem. Phys. 16, 1759–1787. doi:10.1039/C3CP53413H
Barone, V., Biczysko, M., Bloino, J., Cimino, P., Penocchio, E., and Puzzarini, C. (2015a). CC/DFT route toward accurate structures and spectroscopic features for observed and elusive conformers of flexible molecules: pyruvic acid as a case study. J. Chem. Theory Comput. 11, 4342–4363. doi:10.1021/acs.jctc.5b00580
Barone, V., Biczysko, M., Bloino, J., Cimino, P., Penocchio, E., and Puzzarini, C. (2015b). Cc/dft route toward accurate structures and spectroscopic features for observed and elusive conformers of flexible molecules: pyruvic acid as a case study. J. Chem. Theory Comput. 11, 4342–4363. doi:10.1021/acs.jctc.5b00580
Barone, V., Biczysko, M., Bloino, J., and Puzzarini, C. (2013). Glycine conformers: a never-ending story? Phys. Chem. Chem. Phys. 15, 1358–1363. doi:10.1039/C2CP43884D
Barone, V., Biczysko, M., and Puzzarini, C. (2015c). Quantum chemistry meets spectroscopy for astrochemistry: increasing complexity toward prebiotic molecules. Acc. Chem. Res. 48, 1413–1422. doi:10.1021/ar5003285
Barone, V., Ceselin, G., Fusè, M., and Tasinato, N. (2020). Accuracy meets interpretability for computational spectroscopy by means of hybrid and double-hybrid functionals. Front. Chem. 8, 584203. doi:10.3389/fchem.2020.584203
Barone, V., and Lazzari, F. (2023). Hunting for complex organic molecules in the interstellar medium: the role of accurate low-cost theoretical geometries and rotational constants. J. Phys. Chem. A 127, 10517–10527. doi:10.1021/acs.jpca.3c06649
Barone, V., and Puzzarini, C. (2023). Gas-phase computational spectroscopy: the challenge of the molecular bricks of life. Annu. Rev. Phys. Chem. 74, 29–52. doi:10.1146/annurev-physchem-082720-103845
Becke, A. D. (1993). Density-functional thermochemistry. iii. the role of exact exchange. J. Chem. Phys. 98, 5648–5652. doi:10.1063/1.464913
Biczysko, M., Bloino, J., and Puzzarini, C. (2018a). Computational challenges in astrochemistry. WIREs Comput. Mol. Sci. 8, e1349. doi:10.1002/wcms.1349
Biczysko, M., Krupa, J., and Wierzejewska, M. (2018b). Theoretical studies of atmospheric molecular complexes interacting with NIR to UV light. Faraday Discuss. 212, 421–441. doi:10.1039/C8FD00094H
Bloino, J. (2015). A vpt2 route to near-infrared spectroscopy: the role of mechanical and electrical anharmonicity. J. Phys. Chem. A 119, 5269–5287. doi:10.1021/jp509985u
Bloino, J., Baiardi, A., and Biczysko, M. (2016). Aiming at an accurate prediction of vibrational and electronic spectra for medium-to-large molecules: an overview. Int. J. Quantum Chem. 116, 1543–1574. doi:10.1002/qua.25188
Bloino, J., and Barone, V. (2012). A second-order perturbation theory route to vibrational averages and transition properties of molecules: general formulation and application to infrared and vibrational circular dichroism spectroscopies. J. Chem. Phys. 136, 124108. doi:10.1063/1.3695210
Bloino, J., Biczysko, M., and Barone, V. (2015). Anharmonic effects on vibrational spectra intensities: infrared, Raman, vibrational circular dichroism and Raman optical activity. J. Phys. Chem. A 119, 11862–11874. doi:10.1021/acs.jpca.5b10067
Burova, T. G., and Anashkin, A. A. (2007). Quantum-mechanical calculation of the intensity distribution in the resonance Raman spectrum of benzonitrile. Opt. Spectrosc. 102, 825–828. doi:10.1134/S0030400X07060045
Casado, J., Nygaard, L., and Sørensen, G. O. (1971). Microwave spectra of isotopic benzonitriles. Refined molecular structure of benzonitrile. J. Molec. Struct. 8, 211–224. doi:10.1016/0022-2860(71)80056-X
Cernicharo, J., Fuentetaja, R., Cabezas, C., Agúndez, M., Marcelino, N., Tercero, B., et al. (2022). Discovery of five cyano derivatives of propene with the QUIJOTE line survey. Astron. Astrophys. 663, L5. doi:10.1051/0004-6361/202244255
Ceselin, G., Barone, V., and Tasinato, N. (2021). Accurate biomolecular structures by the nano-LEGO approach: pick the bricks and build your geometry. J. Chem. Theory Comput. 17, 7290–7311. doi:10.1021/acs.jctc.1c00788
Charmet, A. P., Stoppa, P., Tasinato, N., Giorgianni, S., Barone, V., Biczysko, M., et al. (2013). An integrated experimental and quantum-chemical investigation on the vibrational spectra of chlorofluoromethane. J. Chem. Phys. 139, 164302. doi:10.1063/1.4825380
COSY COST Action CA21101 (2024). COSY Confined molecular systems: from a new generation of materials to the stars CA21101 COST Action.
Császár, A. G., and Fogarasi, G. (1989). Scaled quantum mechanical (SQM) force field and theoretical vibrational spectrum for benzonitrile. Spectrochim. Acta A 45, 845–854. doi:10.1016/0584-8539(89)80222-3
[Dataset] Herschel Space Observatory (2017). Herschel’s view of the Taurus molecular cloud annotated. Available at: https://www.herschel.caltech.edu/image/nhsc2017-003a.
Duschinsky, F. (1937). The importance of the electron spectrum in multi atomic molecules. concerning the franck-condon principle. Acta Physicochim. URSS 7, 551.
Eigenbrode, J. L., Summons, R. E., Steele, A., Freissinet, C., Millan, M., Navarro-González, R., et al. (2018). Organic matter preserved in 3-billion-year-old mudstones at Gale crater, Mars. Science 360, 1096–1101. doi:10.1126/science.aas9185
Endres, C. P., Schlemmer, S., Schilke, P., Stutzki, J., and Müller, H. S. P. (2016). The cologne database for molecular spectroscopy, CDMS, in the virtual atomic and molecular data centre, VAMDC. J. Mol. Spectrosc. 327, 95–104. doi:10.1016/j.jms.2016.03.005
Erlandsson, G. (1954). Microwave spectrum of benzonitrile. J. Chem. Phys. 22, 1152. doi:10.1063/1.1740313
Esposito, V. J., Fortenberry, R. C., Boersma, C., Maragkoudakis, A., and Allamandola, L. J. (2024). CN stretches around 4.4 microns dominate the IR absorption spectra of cyano-polycyclic aromatic hydrocarbons. Mon. Not. R. Astron. Soc. Lett. 531, L87–L93. doi:10.1093/mnrasl/slae037
Fornaro, T., Brucato, J. R., Poggiali, G., Corazzi, M. A., Biczysko, M., Jaber, M., et al. (2020). UV irradiation and near infrared characterization of laboratory mars soil analog samples. Front. Astron. Space Sci. 7. doi:10.3389/fspas.2020.539289
Fornaro, T., Carnimeo, I., and Biczysko, M. (2015). Toward feasible and comprehensive computational protocol for simulation of the spectroscopic properties of large molecular systems: the anharmonic infrared spectrum of uracil in the solid state by the reduced dimensionality/hybrid vpt2 approach. J. Phys. Chem. A 119, 5313–5326. doi:10.1021/jp510101y
Fortenberry, R. C. (2024a). Quantum chemistry and astrochemistry: a match made in the heavens. J. Phys. Chem. A 128, 1555–1565. doi:10.1021/acs.jpca.3c07601
Fortenberry, R. C. (2024b). A vision for the future of astrochemistry in the interstellar medium by 2050. ACS Phys. Chem. Au 4, 31–39. doi:10.1021/acsphyschemau.3c00043
Franke, P. R., Stanton, J. F., and Douberly, G. E. (2021). How to VPT2: accurate and intuitive simulations of CH stretching infrared spectra using VPT2+K with large effective Hamiltonian resonance treatments. J. Phys. Chem. A 125, 1301–1324. doi:10.1021/acs.jpca.0c09526
Frisch, M. J., Trucks, G. W., Schlegel, H. B., Scuseria, G. E., Robb, M. A., Cheeseman, J. R., et al. (2016). Gaussian 16 rev. C.01. Wallingford CT: Gaussian, Inc.
Frost, A. J., Lau, R. M., Burtscher, L., Packham, C., Tasker, E. J., Rees, G. A., et al. (2022). IR 2022: an infrared-bright future for ground-based IR observatories in the era of JWST. Nat. Astron. 6, 772–773. doi:10.1038/s41550-022-01733-9
Fulvio, D., Potapov, A., He, J., and Henning, T. (2021). Astrochemical pathways to complex organic and prebiotic molecules: experimental perspectives for in situ solid-state studies. Life 11, 568. doi:10.3390/life11060568
Fusè, M., Mazzeo, G., Longhi, G., Abbate, S., Masi, M., Evidente, A., et al. (2019). Unbiased determination of absolute configurations by vis-à-vis comparison of experimental and simulated spectra: the challenging case of diplopyrone. J. Phys. Chem. B 123, 9230–9237. PMID: 31580674. doi:10.1021/acs.jpcb.9b08375
Green, J. A., and Improta, R. (2020). Vibrations of the guanine–cytosine pair in chloroform: an anharmonic computational study. Phys. Chem. Chem. Phys. 22, 5509–5522. doi:10.1039/C9CP06373K
Green, J. H. S., and Harrison, D. J. (1976). Vibrational spectra of benzene derivatives—XVII. Benzonitrile and substituted benzonitriles. Spectrochim. Acta A 32, 1279–1286. doi:10.1016/0584-8539(76)80166-3
Grimme, S. (2011). Density functional theory with london dispersion corrections. WIREs Comput. Mol. Sci. 1, 211–228. doi:10.1002/wcms.30
Grimme, S., Antony, J., Ehrlich, S., and Krieg, H. (2010). A consistent and accurate ab initio parametrization of density functional dispersion correction (dft-d) for the 94 elements h-pu. J. Chem. Phys. 132, 154104. doi:10.1063/1.3382344
Grimme, S., Ehrlich, S., and Goerigk, L. (2011). Effect of the damping function in dispersion corrected density functional theory. J. Comput. Chem. 32, 1456–1465. doi:10.1002/jcc.21759
Guélin, M., and Cernicharo, J. (2022). Organic molecules in interstellar space: latest advances. Front. Astron. Space Sci. 9. doi:10.3389/fspas.2022.787567
Krasnoshchekov, S. V., Vogt, N., and Stepanov, N. F. (2015). Ab initio anharmonic analysis of vibrational spectra of uracil using the numerical-analytic implementation of operator Van Vleck perturbation theory. J. Phys. Chem. A 119, 6723–6737. doi:10.1021/acs.jpca.5b03241
Kwon, C. H., Kim, H. L., and Kim, M. S. (2003). Vibrational analysis of vacuum ultraviolet mass-analyzed threshold ionization spectra of phenylacetylene and benzonitrile. J. Phys. Chem. A 107, 10969–10975. doi:10.1021/jp036391w
Lasne, J. (2021). Heterogeneous physical chemistry in the atmospheres of earth, mars, and venus: perspectives for rocky exoplanets. ACS Earth Space Chem. 5, 149–162. doi:10.1021/acsearthspacechem.0c00126
Lee, K. L. K., Changala, P. B., Loomis, R. A., Burkhardt, A. M., Xue, C., Cordiner, M. A., et al. (2021). Interstellar detection of 2-cyanocyclopentadiene, C5H5CN, a second five-membered ring toward TMC-1. Astrophys. J. Lett. 910, L2. doi:10.3847/2041-8213/abe764
Lemmens, A. K., Rap, D. B., Brünken, S., Buma, W. J., and Rijs, A. M. (2022). Polycyclic aromatic hydrocarbon growth in a benzene discharge explored by IR-UV action spectroscopy. Phys. Chem. Chem. Phys. 24, 14816–14824. doi:10.1039/D2CP01631A
Lemmens, K., Mackie, C., Candian, A., Lee, J., Tielens, M., Anouk, M. R., et al. (2023). Size distribution of polycyclic aromatic hydrocarbons in space: an old new light on the 11.2/3.3 m intensity ratio. Faraday Discuss. 245, 380–390. doi:10.1039/D2FD00180B
Lide, D. R. (1954). Microwave spectrum and structure of benzonitrile. J. Chem. Phys. 22, 1577–1578. doi:10.1063/1.1740461
Loru, D., Steber, A. L., Thunnissen, J. M. M., Rap, D. B., Lemmens, A. K., Rijs, A. M., et al. (2022). New potential candidates for astronomical searches discovered in the electrical discharge of the PAH naphthalene and acetonitrile. J. Mol. Spectrosc. 386, 111629. doi:10.1016/j.jms.2022.111629
Mackie, C. J., Candian, A., Lee, T. J., and Tielens, A. G. G. M. (2022). Anharmonicity and the IR emission spectrum of neutral interstellar PAH molecules. J. Phys. Chem. A 126, 3198–3209. doi:10.1021/acs.jpca.2c01849
Martin, J. M. L., Lee, T. J., Taylor, P. M., and François, J.-P. (1995). The anharmonic force field of ethylene, c2h4, by means of accurate ab initio calculations. J. Chem. Phys. 103, 2589–2602. doi:10.1063/1.469681
Martin-Drumel, M.-A., Spaniol, J.-T., Hölzel, H., Agúndez, M., Cernicharo, J., Moth-Poulsen, K., et al. (2023). Searches for bridged bicyclic molecules in space—norbornadiene and its cyano derivatives. Faraday Discuss. 245, 284–297. doi:10.1039/D3FD00016H
McCarthy, M. C., Lee, K. L. K., Loomis, R. A., Burkhardt, A. M., Shingledecker, C. N., Charnley, S. B., et al. (2021). Interstellar detection of the highly polar five-membered ring cyanocyclopentadiene. Nat. Astron. 5, 176–180. doi:10.1038/s41550-020-01213-y
McClure, M. K., Rocha, W. R. M., Pontoppidan, K. M., Crouzet, N., Chu, L. E. U., Dartois, E., et al. (2023). An Ice Age JWST inventory of dense molecular cloud ices. Nat. Astron. 7, 431–443. doi:10.1038/s41550-022-01875-w
McGuire, B. A. (2022). 2021 census of interstellar, circumstellar, extragalactic, protoplanetary disk, and exoplanetary molecules. Astrophys. J. Suppl. Ser. 259, 30. doi:10.3847/1538-4365/ac2a48
McGuire, B. A., Burkhardt, A. M., Kalenskii, S., Shingledecker, C. N., Remijan, A. J., Herbst, E., et al. (2018). Detection of the aromatic molecule benzonitrile (c-C6H5CN) in the interstellar medium. Science 359, 202–205. doi:10.1126/science.aao4890
McGuire, B. A., Loomis, R. A., Burkhardt, A. M., Lee, K. L. K., Shingledecker, C. N., Charnley, S. B., et al. (2021). Detection of two interstellar polycyclic aromatic hydrocarbons via spectral matched filtering. Science 371, 1265–1269. doi:10.1126/science.abb7535
McIntosh, O., García-Florentino, C., Fornaro, T., Marabello, D., Alberini, A., Siljeström, S., et al. (2024). Undecanoic acid and L-phenylalanine in vermiculite: detection, characterization, and UV degradation studies for biosignature identification on mars. Astrobiology 24, 518–537. doi:10.1089/ast.2023.0088
Mehta, N., Santra, G., and Martin, J. M. (2023). Is explicitly correlated double-hybrid density functional theory advantageous for vibrational frequencies? Can. J. Chem. 101, 656–663. doi:10.1139/cjc-2022-0277
Mendolicchio, M., Bloino, J., and Barone, V. (2021). General perturb-then-diagonalize model for the vibrational frequencies and intensities of molecules belonging to abelian and non-abelian symmetry groups. J. Chem. Theory Comput. 17, 4332–4358. doi:10.1021/acs.jctc.1c00240
Mills, I. M. (1972). Molecular spectroscopy: modern research (academic press, New York), chap. Vibration-rotation structure in asymmetric- and symmetric-top molecules, 115–140.
Najibi, A., and Goerigk, L. (2018). The nonlocal kernel in van der waals density functionals as an additive correction: An extensive analysis with special emphasis on the B97M-v and B97M-V approaches. J. Chem. Theory Comput. 14, 5725–5738. doi:10.1021/acs.jctc.8b00842
Nazari, P., van Gelder, M. L., van Dishoeck, E. F., Tabone, B., van ’t Hoff, M. L. R., Ligterink, N. F. W., et al. (2021). Complex organic molecules in low-mass protostars on Solar System scales - II. Nitrogen-bearing species. Astron. Astrophys. 650, A150. doi:10.1051/0004-6361/202039996
Nielsen, H. H. (1951). The vibration-rotation energies of molecules. Rev. Mod. Phys. 23, 90–136. doi:10.1103/RevModPhys.23.90
Öberg, K. I. (2016). Photochemistry and astrochemistry: photochemical pathways to interstellar complex organic molecules. Chem. Rev. 116, 9631–9663. doi:10.1021/acs.chemrev.5b00694
Palmer, M. H., Ridley, T., Hoffmann, S. V., Jones, N. C., Coreno, M., de Simone, M., et al. (2015a). Interpretation of the vacuum ultraviolet photoabsorption spectrum of iodobenzene by ab initio computations. J. Chem. Phys. 142, 134302. doi:10.1063/1.4916121
Palmer, M. H., Ridley, T., Hoffmann, S. V., Jones, N. C., Coreno, M., de Simone, M., et al. (2015b). The ionic states of iodobenzene studied by photoionization and ab initio configuration interaction and DFT computations. J. Chem. Phys. 142, 134301. doi:10.1063/1.4916120
Papajak, E., Zheng, J., Xu, X., Leverentz, H. R., and Truhlar, D. G. (2011). Perspectives on basis sets beautiful: seasonal plantings of diffuse basis functions. J. Chem. Theory Comput. 7, 3027–3034. doi:10.1021/ct200106a
Peeters, E., Mackie, C., Candian, A., and Tielens, A. G. G. M. (2021). A spectroscopic view on cosmic PAH emission. Acc. Chem. Res. 54, 1921–1933. doi:10.1021/acs.accounts.0c00747
Perez, R., Parès, L. P., Newell, R., Robinson, S., Bernardi, P., Réess, J.-M., et al. (2017). The supercam instrument on the NASA Mars 2020 mission: optical design and performance. nternational Conf. Space Opt. — ICSO 2016 (SPIE) 10562, 744–752. doi:10.1117/12.2296230
Petrignani, A., and Candian, A. (2022). “Chapter 3 - astrochemistry: ingredients of life in space,” in New Frontiers in astrobiology. Editors R. Thombre, and P. Vaishampayan (Elsevier), 49–66. doi:10.1016/B978-0-12-824162-2.00007-5
Piccardo, M., Penocchio, E., Puzzarini, C., Biczysko, M., and Barone, V. (2015). Semi-experimental equilibrium structure determinations by employing b3lyp/snsd anharmonic force fields: validation and application to semirigid organic molecules. J. Phys. Chem. A 119, 2058–2082. doi:10.1021/jp511432m
Pietropolli Charmet, A., Ceselin, G., Stoppa, P., and Tasinato, N. (2022). The spectroscopic characterization of halogenated pollutants through the interplay between theory and experiment: application to R1122. Molecules 27, 748. doi:10.3390/molecules27030748
Pulay, P., Meyer, W., and Boggs, J. E. (1978). Cubic force constants and equilibrium geometry of methane from Hartree–Fock and correlated wavefunctions. J. Chem. Phys. 68, 5077–5085. doi:10.1063/1.435626
Puzzarini, C. (2013). Rotational spectroscopy meets theory. Phys. Chem. Chem. Phys. 15, 6595–6607. doi:10.1039/C3CP44301A
Puzzarini, C. (2022). Gas-phase chemistry in the interstellar medium: the role of laboratory astrochemistry. Front. Astron. Space Sci. 8. doi:10.3389/fspas.2021.811342
Puzzarini, C., Alessandrini, S., Bizzocchi, L., Melosso, M., and Rivilla, V. M. (2023). From the laboratory to the interstellar medium: a strategy to search for exotic molecules in space. Front. Astron. Space Sci. 10. doi:10.3389/fspas.2023.1211784
Puzzarini, C., Stanton, J. F., and Gauss, J. (2010). Quantum-chemical calculation of spectroscopic parameters for rotational spectroscopy. Int. Rev. Phys. Chem. 29, 273–367. doi:10.1080/01442351003643401
Rajasekhar, B. N., Dharmarpu, V., Das, A. K., Shastri, A., Veeraiah, A., and Krishnakumar, S. (2022). A spectroscopic study of benzonitrile. J. Quant. Spectrosc. Radiat. Transf. 283, 108159. doi:10.1016/j.jqsrt.2022.108159
Rosnik, A. M., and Polik, W. F. (2014). Vpt2+k spectroscopic constants and matrix elements of the transformed vibrational Hamiltonian of a polyatomic molecule with resonances using Van Vleck perturbation theory. Mol. Phys. 112, 261–300. doi:10.1080/00268976.2013.808386
Rudolph, H. D., Demaison, J., and Császár, A. G. (2013). Accurate determination of the deformation of the benzene ring upon substitution: equilibrium structures of benzonitrile and phenylacetylene. J. Phys. Chem. A 117, 12969–12982. doi:10.1021/jp408208s
Santra, G., Sylvetsky, N., and Martin, J. M. L. (2019). Minimally empirical double-hybrid functionals trained against the GMTKN55 database: revDSD-PBEP86-D4, revDOD-PBE-D4, and DOD-SCAN-D4. J. Phys. Chem. A 123, 5129–5143. doi:10.1021/acs.jpca.9b03157
Sharma, S., Roppel, R. D., Murphy, A. E., Beegle, L. W., Bhartia, R., Steele, A., et al. (2023). Diverse organic-mineral associations in Jezero crater, Mars. Nature 619, 724–732. doi:10.1038/s41586-023-06143-z
Sheng, M., Silvestrini, F., Biczysko, M., and Puzzarini, C. (2021). Structural and vibrational properties of amino acids from composite schemes and double-hybrid dft: hydrogen bonding in serine as a test case. J. Phys. Chem. A 125, 9099–9114. doi:10.1021/acs.jpca.1c06993
Sita, M. L., Changala, P. B., Xue, C., Burkhardt, A. M., Shingledecker, C. N., Lee, K. L. K., et al. (2022). Discovery of interstellar 2-cyanoindene (2-C9H7CN) in GOTHAM observations of TMC-1. Astrophys. J. Lett. 938, L12. doi:10.3847/2041-8213/ac92f4
Tasinato, N., Pietropolli Charmet, A., Ceselin, G., Salta, Z., and Stoppa, P. (2022). In vitro and in silico vibrational–rotational spectroscopic characterization of the next-generation refrigerant HFO-1123. J. Phys. Chem. A 126, 5328–5342. doi:10.1021/acs.jpca.2c04680
Vago, J. L., Westall, F., Coates, A. J., Jaumann, R., Korablev, O., Ciarletti, V., et al. (2017). Habitability on early mars and the search for biosignatures with the ExoMars rover. Astrobiology 17, 471–510. doi:10.1089/ast.2016.1533
Vázquez, J., and Stanton, J. F. (2007). Treatment of fermi resonance effects on transition moments in vibrational perturbation theory. Mol. Phys. 105, 101–109. doi:10.1080/00268970601135784
Webb Space Telescope (2024). NGC 604 (NIRCam image). Available at: https://webbtelescope.org/contents/media/images/2024/110/01HQNV4GP6PR6E7ZSJXRRBQQDS.
Williford, K. H., Farley, K. A., Stack, K. M., Allwood, A. C., Beaty, D., Beegle, L. W., et al. (2018). “Chapter 11 - the NASA mars 2020 rover mission and the search for extraterrestrial life,” in From habitability to life on Mars. Editors N. A. Cabrol, and E. A. Grin (Elsevier), 275–308. doi:10.1016/B978-0-12-809935-3.00010-4
Xu, R., Jiang, Z., Yang, Q., Bloino, J., and Biczysko, M. (2024). Harmonic and anharmonic vibrational computations for biomolecular building blocks: benchmarking DFT and basis sets by theoretical and experimental IR spectrum of glycine conformers. J. Comput. Chem. 45, 1846–1869. doi:10.1002/jcc.27377
Yamamoto, R., Ishikawa, S., Ebata, T., and Mikami, N. (2000). Vibrational spectra and relaxation of benzonitrile and its clusters using time-resolved stimulated Raman–UV double resonance spectroscopy. J. Raman Spectrosc. 31, 295–304. doi:10.1002/(sici)1097-4555(200004)31:4<295:aid-jrs542>3.0.co;2-y
Yang, Q., and Bloino, J. (2022). An effective and automated processing of resonances in vibrational perturbation theory applied to spectroscopy. J. Phys. Chem. A 126, 9276–9302. doi:10.1021/acs.jpca.2c06460
Yang, Q., Mendolicchio, M., Barone, V., and Bloino, J. (2021). Accuracy and reliability in the simulation of vibrational spectra: a comprehensive benchmark of energies and intensities issuing from generalized vibrational perturbation theory to second order (GVPT2). Front. Astron. Space Sci. 8. doi:10.3389/fspas.2021.665232
Yatsyna, V., Mallat, R., Gorn, T., Schmitt, M., Feifel, R., Rijs, A. M., et al. (2019). Competition between folded and extended structures of alanylalanine (ala-ala) in a molecular beam. Phys. Chem. Chem. Phys. 21, 14126–14132. doi:10.1039/C9CP00140A
Zapata Trujillo, J. C., Pettyjohn, M. M., and McKemmish, L. K. (2023). High-throughput quantum chemistry: empowering the search for molecular candidates behind unknown spectral signatures in exoplanetary atmospheres. Mon. Not. R. Astron. Soc. 524, 361–376. doi:10.1093/mnras/stad1717
Zdanovskaia, M. A., Martin-Drumel, M.-A., Kisiel, Z., Pirali, O., Esselman, B. J., Woods, R. C., et al. (2022). The eight lowest-energy vibrational states of benzonitrile: analysis of Coriolis and Darling-Dennison couplings by millimeter-wave and far-infrared spectroscopy. J. Mol. Spectrosc. 383, 111568. doi:10.1016/j.jms.2021.111568
Keywords: vibrational spectra, density functional theory, second-order vibrational perturbation theory, near-infrared, James Webb Space Telescope
Citation: Xu Y and Biczysko M (2024) Toward the identification of cyano-astroCOMs via vibrational features: benzonitrile as a test case. Front. Chem. 12:1439194. doi: 10.3389/fchem.2024.1439194
Received: 27 May 2024; Accepted: 12 August 2024;
Published: 03 September 2024.
Edited by:
Jean-Claude Guillemin, UMR6226 Institut des Sciences Chimiques de Rennes (ISCR), FranceReviewed by:
Barbara Michela Giuliano, Max Planck Institute for Extraterrestrial Physics, GermanyNicola Tasinato, Normal School of Pisa, Italy
Copyright © 2024 Xu and Biczysko. This is an open-access article distributed under the terms of the Creative Commons Attribution License (CC BY). The use, distribution or reproduction in other forums is permitted, provided the original author(s) and the copyright owner(s) are credited and that the original publication in this journal is cited, in accordance with accepted academic practice. No use, distribution or reproduction is permitted which does not comply with these terms.
*Correspondence: Malgorzata Biczysko, bWFsZ28uYmljenlza29AZ21haWwuY29t