- Laboratory of Biomaterials and Biomechanics, Beijing Key Laboratory of Tooth Regeneration and Function Reconstruction, Beijing Stomatological Hospital, Capital Medical University, Beijing, China
Introduction: Smart multifunctional surfaces targeting intricate biological events or versatile therapeutic strategies are imminent to achieve long-term transmucosal implant success.
Methods: This study used dopamine (DA), graphene oxide (GO), and type IV collagen (COL-IV) to construct multilayer nanofilms (DGCn) based on their universal adhesive and biomimetic properties to design a versatile and bioactive titanium implant. The characterization of DGCn on different titanium surfaces was performed, and its loading capacity, release profile, in situ gene delivery, and in vitro biological properties were preliminarily evaluated.
Results: Our results demonstrate that hydrogenated TiO2 nanotubes (H) provide a better platform for the DGCn coating than machined Ti and air-TiO2 nanotubes. The H-DGC10 displayed the most stable surface with excellent loading capacity, sustained-release profile, and in situ gene transfection efficiency; this could be due to the high specific surface area of H and GO, as well as the functional groups in H, DA, and GO. Moreover, the H-DGC10 exhibited good biocompatibility for human oral epithelial cells and promoted the expression of integrin β4 and laminin 332, both being hemidesmosome-related proteins.
Discussion: Our findings suggest that H-DGCn can be designed as a smart multifunctional interface for titanium implants to achieve long-term transmucosal implant success and aid in versatile therapeutic strategies.
1 Introduction
The long-term success of dental implants is not only limited to stable osseointegration but also to the sealing effect of transmucosal soft tissues. Firm soft-tissue integration can resist mechanical stress, microbial invasion, and marginal bone resorption (Saso and Lee, 2018; Ahamed et al., 2021; Kunrath and Gerhardt, 2023). However, the implant–soft-tissue interface can exhibit inferior sealing functionality compared with natural teeth, leading to various biological complications such as peri-implant mucositis and peri-implantitis (Iacov-Craitoiu and Craitoiu, 2020; Weinstein et al., 2020). Titanium—the preferred biomaterial for dental implants—has been widely investigated to facilitate osseointegration. Currently, well-modified titanium surfaces for soft-tissue sealing are at the forefront of implant research.
Nanostructured, biomimetic, and antibacterial surfaces have been demonstrated to modulate soft-tissue integration and reduce biofilm formation (Guo et al., 2021; Areid et al., 2024; Shrivas et al., 2024). However, most modified surfaces have been developed with a single function for a specific objective, and may not cope with intricate peri-implant microenvironments. In practice, versatile surfaces are required. Mathur et al. (Mathur et al., 2022) fabricated a gelatin electrospun mat scaffold embedded with silver nanoparticles on a titanium alloy surface to improve fibroblast adhesion, differentiation, and antimicrobial activity. Boda et al. (Boda and Aparicio, 2022) introduced adhesive peptides and anti-inflammatory biomolecules into a dual-function titanium coating to reduce the inflammatory response and improve soft-tissue adhesion. Another study fabricated multilayer alginate/chlorhexidine coatings on titanium surfaces and demonstrated that the modified coatings inhibited plaque biofilm formation and decreased inflammation in vivo (Wu et al., 2023). Importantly, the coatings led to the adhesion and proliferation of fibroblasts, even in a bacterial environment. However, these methods are often designed to load a specific substance for a specific biological effect, which is not conducive to their general application or popularization. Consequently, a smart multifunctional surface with bioactive, immunomodulatory, and antibacterial properties that targets complicated and diverse biological events and versatile therapeutic strategies (such as bioactive molecule/drug/gene delivery during soft-tissue integration), is urgently needed.
Dopamine (DA) is a biomimetic substance derived from mussel adhesive proteins that can produce polydopamine (PDA) with more functional groups via self-polymerization under alkaline conditions. PDA is widely used to construct multifunctional bioengineering materials owing to its excellent interfacial interactivity, bioactivity, and antioxidant capacity (Liu et al., 2016; Ball, 2018; Xie et al., 2021). Qin et al. (Qin et al., 2022) used PDA to immobilize BMP-2 gene encapsulated in aminated poly (lactic-co-glycolic acid) microspheres on polyetheretherketone. They verified that the PDA coating enhanced biological activity and that gene delivery effectively improved osteogenic differentiation. Another study developed a multifunctional nanosystem with macrophage cell membrane-camouflaged oseltamivir-PDA nanoparticles (Liu et al., 2023b). PDA nanoparticles have been shown to suppress inflammatory storms by removing reactive oxygen species, leading to controlled drug release. Based on these properties, DA was employed as a biomimetic substance and an adhesive interface between titanium surfaces and other layers in this study.
Graphene oxide (GO) has recently emerged as a promising material for biological applications. Sharma et al. (Sharma et al., 2022) coated a polylactic acid scaffold with PDA-reduced GO, and the designed coating exhibited antioxidant and antimicrobial properties, as well as pro-angiogenic and osteoinductive functionality. Kutwin et al. (Kutwin et al., 2021) used graphene-based complexes as miRNA vectors to support anticancer therapy. In another study, GO was coated on collagen membranes to enhance their biocompatibility (Radunovic et al., 2017), with the GO-modified membranes inducing stem cell differentiation and reducing inflammation. Additionally, GO possesses a high specific surface area and abundant active groups (such as hydroxyl, carboxyl, and epoxy groups), which induce biochemical and bioconjugation reactions (Tiwari et al., 2020). In this study, we sought to take advantage of DA and GO to develop a flexible and versatile titanium coating to achieve excellent multifunctional integration. Impressively, GO has been widely applied in the regeneration, antimicrobial, anti-inflammatory, and gene/drug delivery fields (Hoseini-Ghahfarokhi et al., 2020; Raslan et al., 2020; Ramirez and Osendi, 2022; Inchingolo et al., 2023). Recently, bioactive coatings incorporating GO have been constructed using the layer-by-layer (LBL) technology. A previous study employed LBL to fabricate a multilayer coating on magnesium alloy surface, utilizing chitosan-functionalized GO (GOCS) and heparin (Hep). This coating not only improved corrosion resistance but also enhanced biocompatibility with endothelial cells (Gao et al., 2020). In another study, a bioinspired PDA/GO/collagen coating was constructed using LBL, serving as a multifunctional carrier for bioactive components (Xu et al., 2021). Furthermore, You et al. (You et al., 2024) developedε-poly-L-lysine (PLL)/GO self-assembly multilayers, demonstrating that 20 layers of PLL/GO exhibited remarkable antibacterial properties without any biological toxicity.
Biomimetic natural extracellular matrix (ECM) components provide a comparable microenvironment for cell communication, mechanotransduction, structural integrity, and signal regulation in biomedical engineering (Tamayo-Angorrilla et al., 2022; Liu et al., 2023a; Zhu et al., 2023). Type Ⅳ collagen (COL-Ⅳ)—a pivotal component of the basement membrane (BM), a specialized thin matrix of ECM that is critical for peri-implant soft tissue sealing—can integrate with laminin polymeric networks for epithelial cell anchoring. Coelho-Sampaio et al. (Coelho-Sampaio et al., 2020) fabricated a flat BM-like network by assembling COL-Ⅳ with poly-laminin, which was shown to be conducive to the formation of stratified cell layers, organized F-actin, and tight junctions. Another study designed a dense COL-Ⅳ and/or laminin layer on type Ⅰ collagen film to mimic the Descemet’s membrane (Palchesko et al., 2016). Moreover, Zeng et al. (Zeng et al., 2020) developed a multilayer COL-Ⅳ/laminin nanofilm, and demonstrated that the mimetic BM improved cell adhesion and spreading, while inhibiting cell migration. Consequently, constructing a biomimetic BM structure is potentially beneficial for sealing transmucosal soft tissue.
In this study, we designed a biomimetic, flexible, and versatile system as a template to target diverse biological events and develop therapeutic strategies to promote peri-implant soft-tissue sealing. Accordingly, multilayer DA/GO/COL-IV nanofilms were coated onto different titanium surfaces using a LBL technique. The surface characteristics, loading/delivery capabilities, and inherent bioactivities of the modified surfaces were evaluated.
2 Materials and methods
2.1 Materials
Machined titanium specimens (99.99%) were purchased from Cuibolin Nonferrous Metal Industry Co., Ltd. (Beijing, China). Acetone, ethyl alcohol, ethylene glycol, and ammonium fluoride were purchased from Sinopharm Chemical Reagent Co., Ltd. (Shanghai, China). XFNANO (Nanjing, China) provided us with graphene oxide (GO) dispersion water (2 mg/mL, lateral size 50–200 nm). Dopamine (DA) hydrochloride, type Ⅳ collagen (COL-Ⅳ), bovine serum albumin (BSA), and QuantiPro BCA Assay Kit were purchased from Sigma-Aldrich (Merck, Darmstadt, Germany). Sirius Red Total Collagen Detection Kit was supplied by Chondrex, Inc. (Woodinville, WA, United States). Recombinant adenoviral vectors expressing mCherry (Ad-mCherry) were constructed by HANBIO (Shanghai, China). Human oral epithelial cells (HOECs) were purchased from Wuhan Pricella Biotechnology Co., Ltd. (Wuhan, China). Anti-adenovirus type 5 antibody, rabbit antibody targeting integrin β4, and DyLight 488-conjugated anti-rabbit IgG were supplied by Abcam (Cambridge, United Kingdom). Paraformaldehyde (4%), Triton X-100, goat serum, 3-(4, 5-Dimethyl-2-thiazolyl)-2, 5-diphenyl-2H-tetrazolium bromide (MTT), and Calcein/PI Live/Dead Assay Kit were purchased from Beyotime Biotechnology (Beijing, China). 4′,6-Diamidino-2-phenylindole (DAPI) was purchased from ZSGB-BIO (Beijing, China). Dulbecco’s Modified Eagle Medium (DMEM), fetal bovine serum (FBS), and penicillin/streptomycin were obtained from Gibco (Thermo Fisher Scientific Inc., United States). TRIzol Reagent kit was purchased from Invitrogen (Carlsbad, CA, United States). PrimeScript RT Reagent kit was purchased from TaKaRa (Shiga, Japan). RT-PCR reagent was obtained from CWBio (Beijing, China). The primer sequences for the target genes were constructed by Shenggong (Shanghai, China).
2.2 Pretreatment of titanium specimens
Machined titanium specimens (10 mm × 10 mm × 0.2 mm) were used in this study. Ultrasonic cleaning was performed by rinsing the specimens with acetone, ethyl alcohol, and distilled water for 5 min, respectively. Anodic oxide specimens were then prepared by means of an electrochemical method (at 50 V for 15 min) using Ti as the anode and ethylene glycol (0.5 wt% ammonium fluoride and 10 vol% deionized water) as the electrolyte. After annealing at 500°C for 2 h in air, the specimens were labeled as air-TiO2 nanotubes (A group). Subsequently, hydrogenated TiO2 nanotubes (H group) were prepared using a thermal hydrogenation technique under a hydrogen atmosphere (0.95 × 105 Pa, 500°C, and 4 h). Machined titanium (T) specimens were used as controls.
2.3 Preparation of multilayer DA/GO/COL-Ⅳ (DGC) nanofilms
The layer-by-layer (LBL) self-assembly method was employed to fabricate multilayer DGC nanofilms on the surfaces of the T, A, and H groups. First, the specimens were dipped in a DA/Tris solution (2.0 mg/mL, pH = 8.5) at 25°C. After 5 min, the specimens were washed thrice and immersed in a GO suspension (0.5 mg/mL) for another 5 min. The specimens were then assembled with COL-IV in a COL-Ⅳ/acetic acid buffer solution (50 μg/mL, pH = 4.5) for 5 min. Following each immersion, the specimens were cleaned three times with deionized water to remove unbound components. By repeating this process 5, 10, and 20 times, multilayer DGC nanofilms (DGCn, where n denotes the number of DGC layers) were fabricated on the surfaces of the T, A, and H groups (denoted as T-DGCn, A-DGCn, and H-DGCn, respectively). All specimens intended for in vitro experiments were disinfected using an ultraviolet device for 20 min on both sides.
2.4 Characterization of specimens
Scanning electron microscopy (SEM, SU8010, Hitachi, Ltd., Tokyo, Japan) was employed to observe the surface morphology of the specimens. To determine the thickness of the nanofilms, the specimens were embedded in resin, and cross-sectional slices were obtained for SEM analysis. The elemental distribution on the specimens was analyzed through energy dispersive spectroscopy (EDS) equipped with SU8010 SEM. The surface roughness was analyzed using atomic force microscopy (AFM; Dimension ICON, Bruker, Germany). The contact angles (CAs) of the specimens were measured using an optical system (OCA20; Data Physics Instruments, Esslingen, Germany). The bonding strengths of the nanofilms were assessed using a nanoscratch test (TI 980, Bruker). Surface elemental composition and chemical state analyses were performed using X-ray photoelectron spectroscopy (XPS; ESCALAB Xi+, Thermo Scientific, United States).
2.5 Evaluation of COL-Ⅳ encapsulation
To evaluate the encapsulation capacities of the T-DGCn, A-DGCn, and H-DGCn, quantitative amounts of COL-IV were evaluated. Briefly, the T-DGCn, A-DGCn, and H-DGCn specimens were placed in a 24-well plate, before being repeatedly scraped with a pipette tip in 0.05 M acetic acid (350 μL/well) to collect the encapsulated nanofilms. Subsequently, ultrasonic treatment was performed for 10 min. The collected samples were analyzed using the Sirius Red Total Collagen Detection Kit according to the manufacturer’s instructions. The optical density (OD) was measured at 520 nm using a microplate spectrophotometer (SpectraMax Paradigm, Molecular Devices, CA, United States). The amount of COL-IV encapsulated in the DGC multilayers was calculated using regression analysis based on the standard curve. The T-DGC10, A-DGC10, and H-DGC10 specimens were stained with Sirius Red and observed under a stereoscopic microscope (Leica, Hamburg, Germany). The optical images were captured using a digital camera.
2.6 Evaluation of protein release
To evaluate the release profile of the DGC nanofilms, BSA was used as a model bioactive compound loaded onto the A-DGC10 and H-DGC10. In brief, specimens were immersed in a BSA solution (1 mg/mL) for 5 min between GO and COL-Ⅳ assembly during fabrication of each DGC layer. After 10 repeated cycles, the A and H specimens loaded with BSA-encapsulated DGC multilayers were denoted as A-DGBC10 and H-DGBC10, respectively. The A-DGBC10 and H-DGBC10 specimens were then dipped into 350 μL phosphate buffered saline (PBS, pH = 7.4), followed by incubation at 37°C and 98% relative humidity. After 2, 4, 6, 8, 12, 24, 48, 72, 120, 168, 240, and 336 h of incubation, 100 μL PBS containing the released protein (COL-Ⅳ and BSA) was removed at each time point and 100 μL fresh PBS was added. The collected protein samples were quantitatively detected using a QuantiPro BCA Assay Kit according to manufacturer’s instructions. After 168 and 336 h of release, the A-DGBC10 and H-DGBC10 specimens were removed and washed three times with deionized water. The surface morphology was observed using SEM (Hitachi Ltd., Japan).
2.7 Transfection efficiency
To evaluate the capability of the DGC nanofilms as an in situ gene delivery system, the transfection efficiency of cells on different specimens was evaluated. Ad-mCherry was used as gene vector models. An anti-adenovirus-functionalized surface, as described previously (Lin et al., 2010) was used as the positive control. In brief, the H-DGC10 samples were incubated with anti-adenovirus type 5 antibody (Ab; 1:1,000) overnight at 4°C. After three washes with PBS, the samples were labeled as H-DGC10-Ab. Subsequently, the H, H-DGC10, and H-DGC10-Ab were incubated with Ad-mCherry (0.1, 0.5, 1.0, and 2.0 × 108 PFU/mL) in 24-well plates at 37°C for 4 h. Following three cycles of washing to remove the unbonded Ad-mCherry, HOECs were seeded on the specimens at a density of 1 × 105 cells/well. After being transfected for 3 days, the cells were fixed with 4% paraformaldehyde and stained with DAPI. The samples were observed under a fluorescence microscope (Olympus). The percentage of mCherry-positive cells in three randomly selected microscopic fields was calculated using ImageJ software.
2.8 Evaluation of biological effects on HOECs
2.8.1 Cell culture
The HOECs were cultured in a complete DMEM supplemented with 10% FBS and 1% penicillin/streptomycin at 37°C in a 5% CO2 atmosphere.
2.8.2 Cell viability and proliferation
The effects of the T, A, H, T-DGC10, A-DGC10, and H-DGC10 on cell viability were evaluated using live/dead cell staining and the MTT assay. The HOECs were seeded onto the specimens at a density of 3 × 105 cells/well in 24-well plates. After culturing for 24 h, the cells were stained using a Calcein/PI Live/Dead Assay Kit according to the manufacturer’s instructions. The samples were observed under a fluorescence microscope (BX51; Olympus, Tokyo, Japan), and representative images were captured. The quantitative living cell ratio (%) was analyzed using ImageJ software.
To assess cell proliferation on the T, A, H, T-DGC10, A-DGC10, and H-DGC10 surfaces, the HOECs were seeded in 24-well plates (3 × 105 cells/well). After incubation for 1, 3, and 5 days, the cell proliferation was evaluated using MTT. Briefly, at each time point, the medium was replaced by 350 µL complete medium containing 10% 5 mg/mL MTT solution in each well. The MTT was reduced to formazan pigment by living cells after being incubated at 37°C for 4 h. Subsequently, formazan pigment was dissolved using 350 µL dimethyl sulfoxide, and the solution was transferred to 96-well plates (100 µL/well). The OD values were measured using a microplate spectrophotometer (SpectraMax Paradigm, United States) at 570 nm.
2.8.3 Immunofluorescence
Integrin β4, a hemidesmosome-related protein, was determined using immunofluorescence assay. The HOECs were cultured on different surfaces at a density of 5 × 104 cells/well in 24-well plates for 48 h. After three washes with PBS, the cells were fixed with 4% paraformaldehyde at room temperature for 15 min, followed by permeabilization with 0.1% Triton X-100 for 10 min. Subsequently, the cells were blocked with 10% goat serum for 30 min and incubated with a specific primary rabbit antibody targeting integrin β4 (1:250 dilution) overnight at 4°C. After three washes, the cells were incubated with DyLight 488-conjugated anti-rabbit IgG (1:400 dilution) in the dark for 1 h at room temperature and with DAPI for 5 min. Fluorescent images were obtained using a fluorescence microscope equipped with a camera (Olympus, Tokyo, Japan).
2.8.4 Quantitative real-time polymerase chain reaction (RT-PCR)
RT-PCR was conducted to evaluate the relative gene expression levels in HOECs on different surfaces. HOECs were seeded on T, A, H, T-DGC10, A-DGC10, and H-DGC10 at a density of 5 × 105 cells/well in six-well plates. After 48 h of incubation, total RNA was extracted from the HOECs using a TRIzol Reagent kit. Subsequently, all samples underwent reverse transcription with a PrimeScript RT Reagent kit. The expression levels of integrin β4 (ITGB4) and laminin 332 (LAMA3) were then determined using RT-PCR reagent. To normalized the Ct values, GAPDH expression was used as an internal control. The relative gene expression was calculated using the 2(−ΔΔCt) method. The primer sequences for the target genes are listed in Table 1.
2.9 Statistical analysis
All quantitative values were indicated as means ± standard deviation. Statistical analyses were performed using SPSS 19.0 (International Business Machines Corporation, NY, United States) via one-way analysis of variance (ANOVA) or the Kolmogorov-Smirnov test. Statistically, p < 0.05 was considered significant.
3 Results
3.1 Characterization of specimens
The SEM topographies of the T-DGCn, A-DGCn, and H-DGCn [where n represents 0, 5, 10, or 20 layers of dopamine/graphene oxide/type Ⅳ collagen (DA/GO/COL-Ⅳ, DGC)] are shown in Figure 1A. It is evident that group T exhibits relatively smooth surfaces, whereas groups A and H exhibit uniform nanotubes with diameters of approximately 100 nm. TiO2 nanotubes (TNTs) with large diameters, such as 100 nm, have been reported to exhibit increased surface energy and hydrophilicity. This enhanced property allows for greater loading capacity of proteins/drugs, ultimately leading to improved drug elution performance (Peng L et al., 2009; Kulkarni et al., 2015; Martinez-Marquez et al., 2020; Lin et al., 2021). Therefore, in this study, TNTs with a diameter of 100 nm were used as the initial platform for coating DGC nanofilms. Following assembly with five DGC layers, nanoparticles are evident in the T group, whereas nanofilm structures are formed on the nanotubes in the A and H groups (the nanofilm on the H surface covering a larger area than that on the A surface). The nanotubes in groups A and H are completely and uniformly covered with 10 and 20 DGC nanofilm layers, respectively. More GO ruffles (arrowheads) are evident in the H-DGC10 than in the A-DGC10. However, uneven and porous defects (arrows) appear on the surfaces of A-DGC20 and H-DGC20, indicating instability of the nanofilms. Additionally, the thicknesses of the DGC10 nanofilms were analyzed via cross-sectional observation and EDS mapping (Figures 1B,C), with the quantitative values indicating the thicknesses of the A-DGC10 and H-DGC10 to be 177 and 219 nm, respectively (Figure 1D). Unfortunately, the thickness of the T-DGC10 could not be reliably obtained, which may have been due to the DA/GO/COL-Ⅳ failing to form nanofilms on the T surfaces.
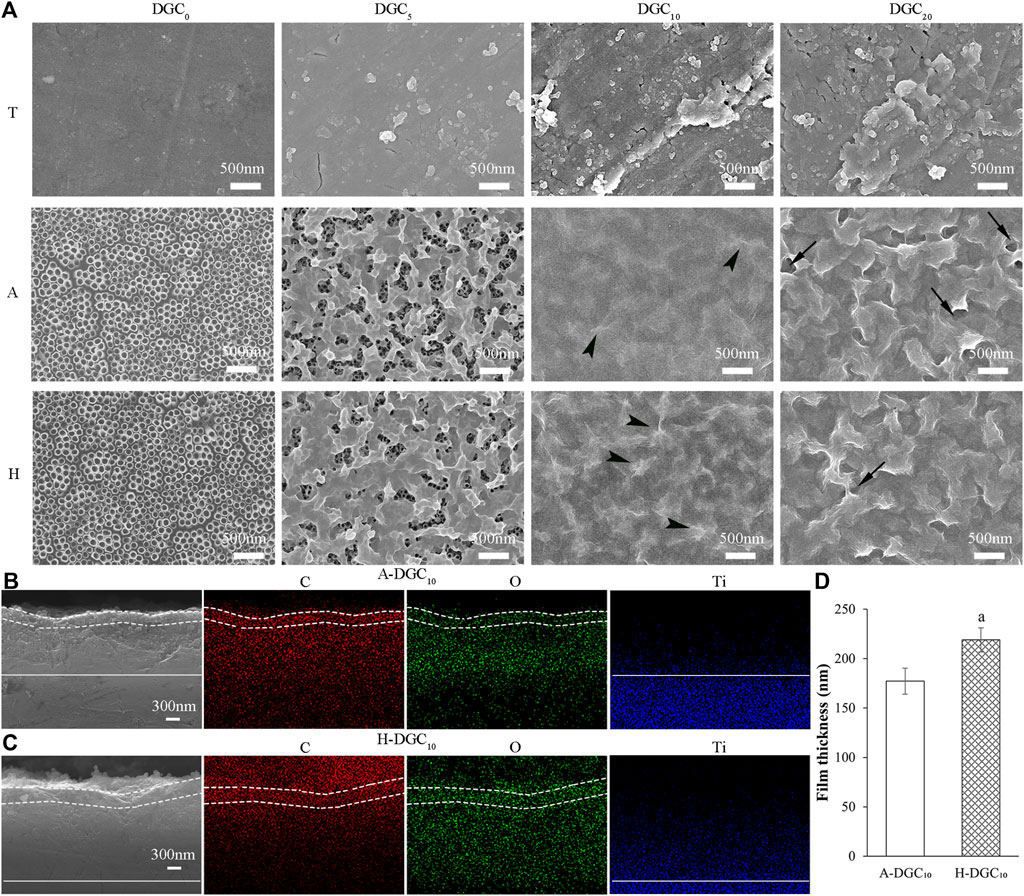
Figure 1. Surface topography and cross-sectional observation of different samples by SEM. (A) Surface topography of different samples (×30,000). Arrowheads: GO ruffles; arrows: uneven and porous defects. Cross-sectional observation and EDS mapping images of (B) the A-DGC10 group (×30,000) and (C) the H-DGC10 group (×30,000). The dashed line marks the DGC nanofilms on the surface of titanium dioxide nanotubes. The straight line marks the boundary between the titanium substrate and the nanotubes. (D) Thickness of nanofilms on the A-DGC10 and H-DGC10 determined from cross-sectional SEM and EDS mapping images. a, p < 0.05 vs the thickness of the A-DGC10 group.
Two-dimensional images of the surface topography obtained by AFM are shown in Figure 2, and the surface features of the different groups are consistent with those of the SEM observations. The surface roughness (Ra) of the different groups was analyzed using AFM. As shown in Figure 3A, the DGC coatings increase the Ra values of the T surfaces and decrease those of the A and H surfaces. The Ra values of the H-DGC10 and H-DGC20 are lower than that of group A. For DGC10 and DGC20 in all groups, increasing the number of layers of DGC has no effect on the surface roughness (p > 0.05).
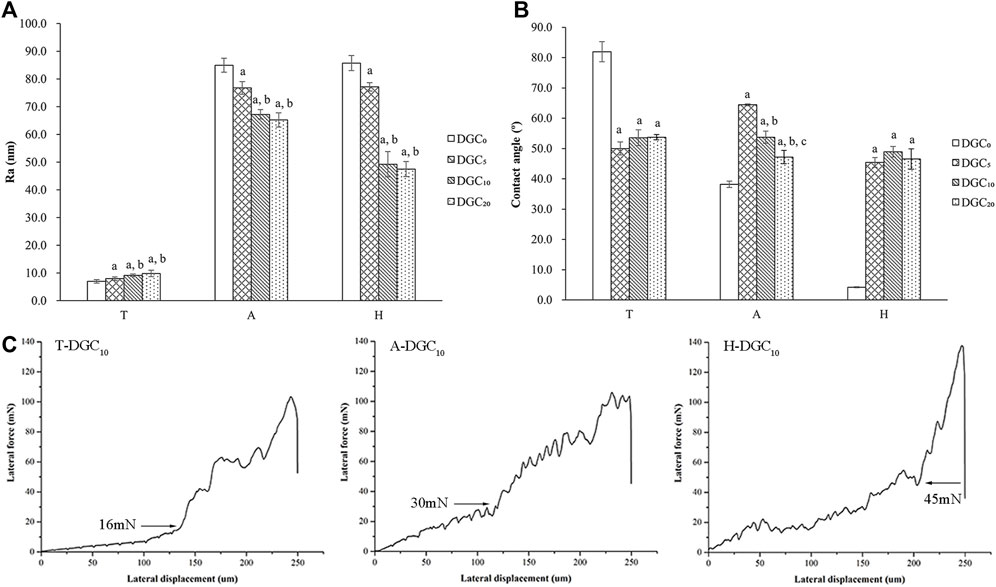
Figure 3. Surface physical characteristics of samples. (A) Surface roughness (Ra) of different surfaces determined by AFM. a, p < 0.05 vs the Ra value of the DGC0 group; b, p < 0.05 vs the Ra of the DGC5 group. (B) Water contact angles (CAs) of different surfaces. a, p < 0.05 vs the CA of the DGC0 group; b, p < 0.05 vs the CA of the DGC5 group; c, p < 0.05 vs the CA of the DGC10 group. (C) Nanoscratch test of the T-DGC10, A-DGC10, and H-DGC10 with critical points at 16, 30, and 45 mN, respectively.
Figure 3B shows the contact angle (CA) values of different samples. It is evident that the DGC coating decreases the CA values of the T surfaces and increases those of the A and H surfaces. The CA values of the T-DGCn and H-DGCn groups does not change significantly when the number of DGC nanofilms increases from 5 to 20 layers.
On behalf of DGCn on different titanium substrates, the microtribological properties of the T-DGC10, A-DGC10, and H-DGC10 were evaluated using a nanoscratch test. As shown in Figure 3C, the critical loads for the T-DGC10, A-DGC10, and H-DGC10 are approximately 16, 30, and 45 mN, respectively.
The XPS spectra of the A groups and H groups are shown in Figure 4. With the coating of DGC nanofilms, the Ti2p signal disappears, whereas the N1s signal derived from the DA and COL-Ⅳ appears along with enhanced C1s signal in the DGCn groups compared to the A or H group (Figures 4A,E). The XPS spectra of the A, A-DGC5, A-DGC10, and A-DGC20 groups reveal element signals that closely resemble those observed in the corresponding H groups. The high-resolution C1s spectra of the DGCn groups were resolved into five peaks assigned to C-C/C=C/CHx, C-N, C-O, C=O, and O-C=O, respectively (Figures 4B–D and Figure 4F–H). The percentage of C-N in the H-DGC5 group (5.1%) is lower than that in the H-DGC10 (7.3%) and H-DGC20 (8.0%). However, it is worth noting that the percentages of C-N in the A groups are comparatively lower than those in the corresponding H groups.
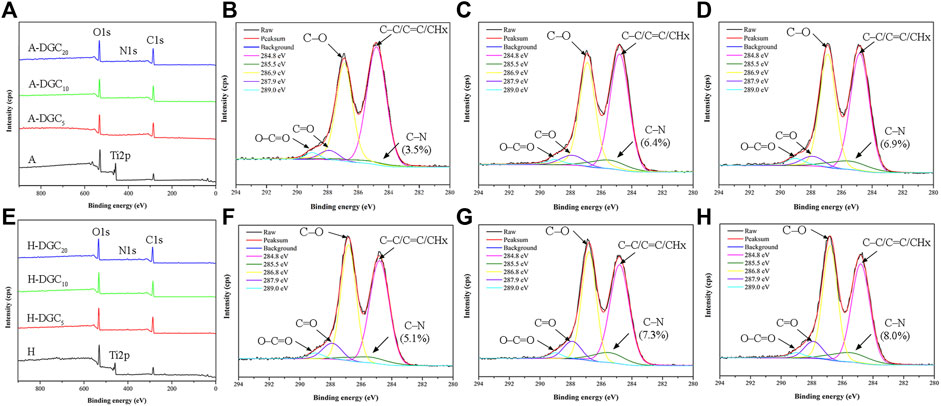
Figure 4. XPS results of samples. (A) XPS spectra of the A, A-DGC5, A-DGC10, and A-DGC20. High-resolution XPS spectra of C1s for the (B) A-DGC5, (C) A-DGC10, and (D) A-DGC20. (E) XPS spectra of the H, H-DGC5, H-DGC10, and H-DGC20. High-resolution XPS spectra of C1s for the (F) H-DGC5, (G) H-DGC10, and (H) H-DGC20.
3.2 Evaluation of COL-Ⅳ encapsulation
Quantitative results of the COL-IV encapsulation in the T-DGCn, A-DGCn, and H-DGCn are shown in Figure 5A. The COL-Ⅳ contents in DGC10 and DGC20 are similar to each other irrespective of the group (p > 0.05). When loading the same number of DGC layers, the COL-Ⅳ content in the T group is the least, followed by the A group, with the H group exhibiting the most COL-Ⅳ content. The inserts in Figure 5A show the surface COL-Ⅳ staining of the T-DGC10, A-DGC10, and H-DGC10, with the COL-Ⅳ being distributed more evenly and abundantly on the H-DGC10 surface.
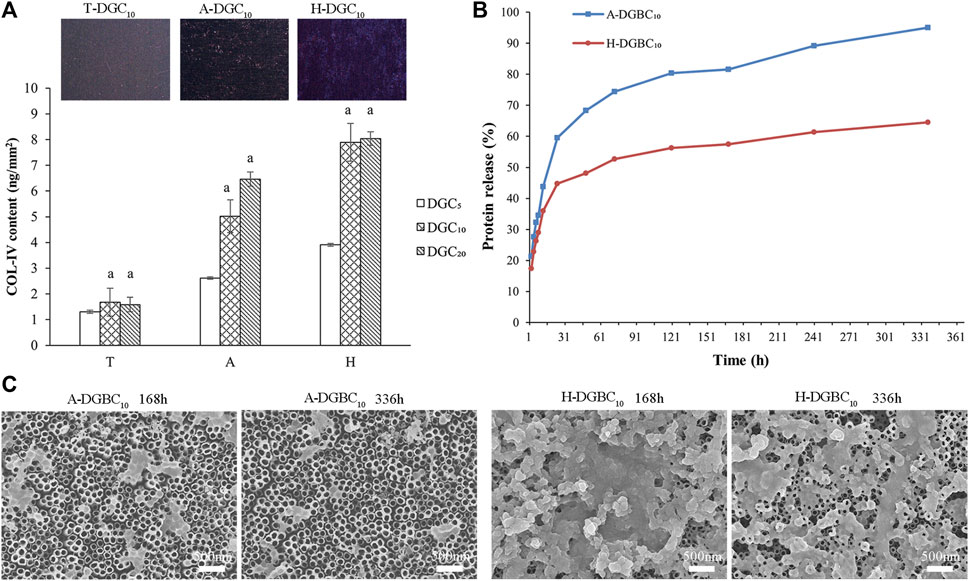
Figure 5. Protein content and protein release of samples. (A) COL-Ⅳ encapsulation in different groups. a, p < 0.05 vs the COL-Ⅳ content of the DGC5 group. The inserts show the surface COL-Ⅳ staining of the T-DGC10, A-DGC10, and H-DGC10. (B) Accumulated protein release behavior of the A-DGBC10 and H-DGBC10 surfaces after incubation in PBS at 37°C for 336 h. (C) Surface topographies of the A-DGBC10 and H-DGBC10 after 168 and 336 h of protein release by SEM (×30,000).
3.3 Evaluation of protein release
It can be difficult to measure the protein release in the T-DGC10 group because of the small amount of protein loading. Therefore, only the protein release profile of A-DGBC10 and H-DGBC10 are shown in Figure 5B. During the initial 24 h, proteins are rapidly released in both groups. In particular, the accumulated protein release in the A-DGBC10 group is up to approximately 60%, whereas it is at 45% in the H-DGBC10 group. Importantly, the protein release in the A-DGBC10 and H-DGBC10 groups is relatively slow and sustained until day 14, especially in the H-DGBC10 group. Within 336 h, the accumulated protein release from the A-DGBC10 and H-DGBC10 groups was 95% and 64%, respectively. The surface topographies of the A-DGBC10 and H-DGBC10 groups after 168 and 336 h of protein release are shown in Figure 5C. With the protein release, the nanofilms on the surfaces of the A-DGBC10 and H-DGBC10 groups disintegrate into nanoparticles. Moreover, the remaining DGBC components on the H-DGBC10 surfaces are greater than those on the A-DGBC10 surfaces. Some nanofilm structures remained on the surface of H-DGBC10 even after 336 h.
3.4 Evaluation of gene delivery
The transfection efficiency of the human oral epithelial cells (HOECs) was used to evaluate the capability of different groups as gene delivery systems. Representative fluorescence images of mCherry expression on different surfaces are shown in Figure 6A. Quantitative analysis showed that transfection efficiency in the HOECs was low in all groups at a titer of 0.1 × 108 PFU/mL (Figure 6B). Modification of the H surface with DGC nanofilms significantly increased the transfection efficiency of the HOECs when the titer of Ad-mCherry was no less than 0.5 × 108 PFU/mL (p < 0.05). The transfection efficiency of the HOECs on the H-DGC10 and H-DGC10-Ab was above 85% at titers of 1.0 and 2.0 × 108 PFU/mL without significant differences (p > 0.05).
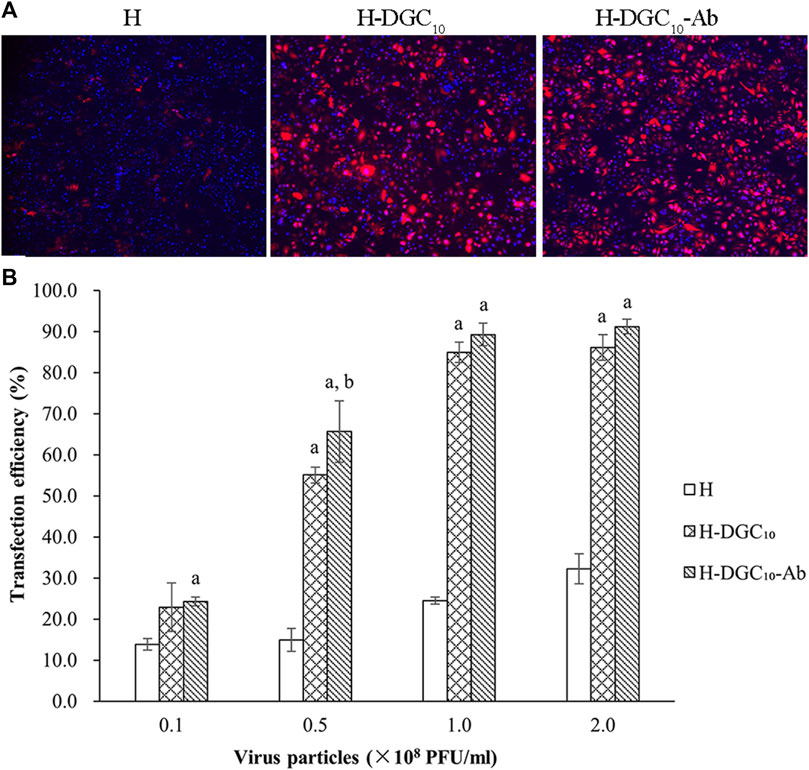
Figure 6. Transfection efficiency. (A) Representative fluorescent images of mCherry expression of cells on the H, H-DGC10, and H-DGC-Ab at a titer of 1.0 × 108 PFU/mL. Original magnification: ×4. (B) Transfection efficiency in the HOECs cultured on samples at different titers. a, p < 0.05 vs the transfection efficiency of the H group; b, p < 0.05 vs the transfection efficiency of the H-DGC10.
3.5 Evaluation of biological effects on HOECs
3.5.1 Cell viability and proliferation
Representative live/dead cell-staining images are shown in Figure 7A. It is evident that there are few dead cells in all groups, indicating the excellent cytocompatibility of all surfaces. The quantitative results showed that the living cell ratio of all groups was above 97% (Figure 7B). As shown in Figure 7C, the DGC nanofilms on the T, A, and H surfaces slightly increases cell proliferation, although the difference is not statistically significant (p > 0.05). Cell proliferation significantly improves in the H and H-DGC10 groups compared to the T group (p < 0.05).
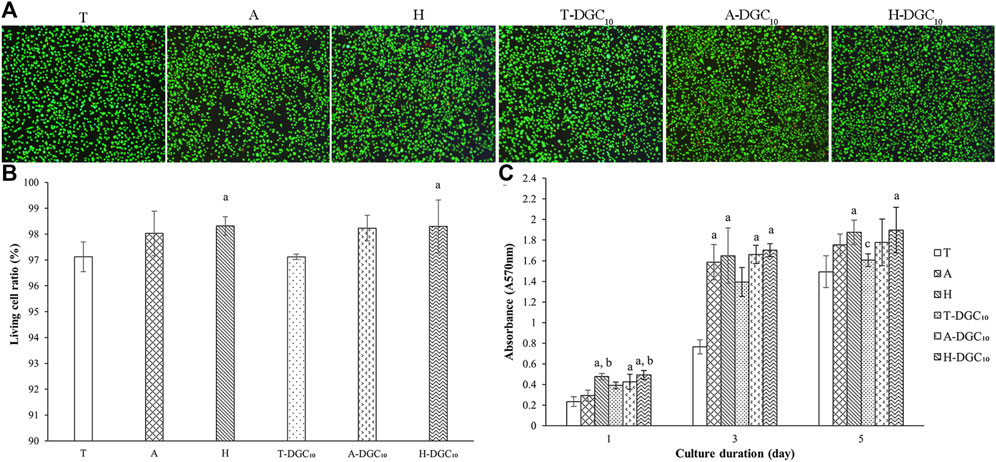
Figure 7. Cell viability and proliferation. (A) Representative live/dead staining images of the HOECs cultured on the surface of the T, A, H, T-DGC10, A-DGC10, and H-DGC10 for 24 h. (B) Living cell ratio of different samples determined from live/dead staining. a, p < 0.05 vs the living cell ratio of the T group. (C) Cell proliferation on different surfaces after culturing for 1, 3, and 5 days a, p < 0.05 vs the cell proliferation of the T group; b, p < 0.05 vs the cell proliferation of the A group; c, p < 0.05 vs the cell proliferation of the H group.
3.5.2 Immunofluorescence
The expression of integrin β4 in the HOECs cultured on different surfaces is displayed in Figure 8. It is evident that integrin β4 is diffusedly distributed around the nuclei of the HOECs on the T and A surfaces, while clustered integrin β4 immunoreactivity is evident at the periphery of the HOECs on the H and DGC10 surfaces, especially on the H-DGC10 surface.
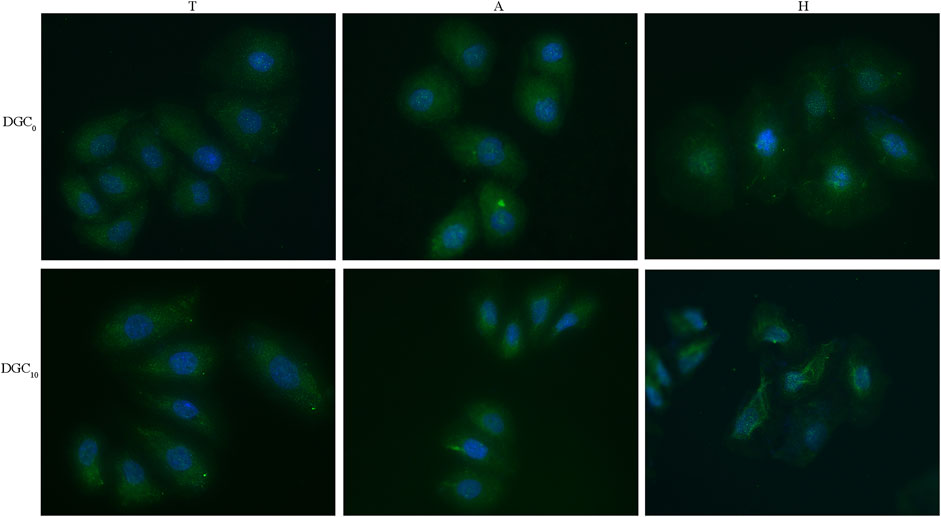
Figure 8. Immunofluorescence staining of integrin β4 in the HOECs cultured on different surfaces for 2 days. Original magnification: ×40. Green, integrin β4; blue, nuclei.
3.5.3 RT-PCR
As illustrated in Figure 9, HOECs on A-DGC10 and H-DGC10 exhibit significantly higher expression levels of ITGB4 and LAMA3 when compared to the initial uncoated platforms (p < 0.05). Additionally, H-DGC10 exhibites the highest ITGB4 expression among all other groups (p < 0.05), corroborating the immunofluorescence results.
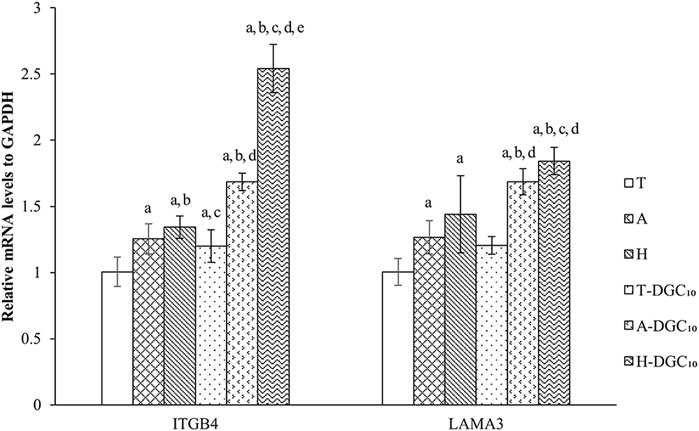
Figure 9. RT-PCR analysis of gene expression of HOECs cultured on the specimens for 48 h. a, p < 0.05 vs the relative gene expression of the T group; b, p < 0.05 vs the relative gene expression of the A group; c, p < 0.05 vs the relative gene expression of H group; d, p < 0.05 vs the relative gene expression of T-DGC10 group; e, p < 0.05 vs the relative gene expression of A-DGC10 group.
4 Discussion
This study investigated the multilayer dopamine/graphene oxide/type Ⅳ collagen (DA/GO/COL-Ⅳ, DGC) nanofilms coated on different titanium surfaces and demonstrated that ten layers of DGC nanofilms on hydrogenated TiO2 nanotubes significantly optimized the bioactivity and loading/delivery capability of biofunctional substances (such as proteins and gene vectors), indicating that the proposed system could be a promising template for multifunctional applications in the clinical implant field.
To choose the optimal initial platform, this study evaluated three different titanium surfaces for the DGCn coating. Our results demonstrate that the DGC nanofilms on the hydrogenated titanium dioxide nanotubes (TNTs, H-DGCn) displayed the most uniform and stable coating surface with the best loading capacity, followed by those on the A-DGCn and T-DGCn. A larger specific surface area was evident on the nanotubular surface of the H and A groups, providing more binding sites (Mendonca et al., 2008; Park et al., 2021) for DGC nanofilms. However, hydrogenated TNTs may generate more functional hydroxyl groups to react with DA, GO, and COL-IV. Previous studies have reported that the lattice defects (oxygen vacancies) present in hydrogenated TiO2 lead to a stable form of numerous hydroxyl groups on the surface (Chen et al., 2011; Lu et al., 2018), suggesting a much greater affinity for subsequent component binding. We assumed that the physicochemical characteristics of the DGC nanofilms were dependent on the specific surface area and density of the hydroxyl groups in the initial titanium platform.
We speculated that three types of components (DA, GO, and COL-Ⅳ) interlace on the substrate surface through covalent bonds, H-bonding, and electrostatic interaction to form DGC nanofilms during the layer-by-layer (LBL) process. First, the hydroxyl groups on different titanium surfaces interact with active catechol groups from the PDA (self-polymerization of DA under alkaline conditions) to form strong covalent attachments (Huang et al., 2016; Liu et al., 2016). More hydroxyl groups on the H surfaces may contribute to more covalent attachment, leading to an increased bonding strength of the H-DGC nanofilms (Figure 3C). Additionally, some of the hydroxyl groups may form hydrogen bonds with the amino, epoxy, and carboxyl groups from the PDA, GO, and COL-Ⅳ. Moreover, deprotonated hydroxyl groups could electrostatically interact with the protonated amino groups (-NH3+) of the PDA and COL-Ⅳ.
In addition to the interaction between the components and initial platforms, the interaction between each component in the LBL assembly process is crucial for DGCn formation and loading capacity. The oxidated catechol groups of the PDA could form covalent bonds (quinone/semiquinone) with the amine groups of COL-Ⅳ via similar Michael addition and Schiff reactions (Lee et al., 2007; Cai et al., 2019). Subsequently, intermolecular crosslinking reactions between the PDA polymers are triggered. Our high-resolution C1s spectra showed that the percentages of C-N in the DGC5 groups were less than those in the DGC10 and DGC20 groups. Importantly, the H-DGCn groups displayed higher percentages of C-N compared to the A-DGCn groups. This difference could potentially be attributed to the initial interactive coating on H surfaces, which forms active multilayers for more component interactions. Moreover, the functional hydroxyl and carboxyl groups of GO can form ester or hydrogen bonds (Tiwari et al., 2020) with PDA and COL-IV. The inherent negative surface charge of GO (Li et al., 2008) can interact with the COL-Ⅳ, which has a positive charge in acid solution. All these interactions among the platform, PDA, GO, and COL-Ⅳ determine the composition and structure of the DGC nanofilms. Additionally, the high specific surface area of the TNTs and GO may provide more binding sites for the DGC coating and bioactive agent loading.
Our SEM results indicated instability in the nanofilms of A-DGC20 and H-DGC20, which displayed uneven and porous defects. Additionally, the Ra values and contact angles of DGC20 were comparable to those of DGC10. Our high-resolution C1s spectra demonstrated that the percentages of C-N are similar in the DGC10 and DGC20 groups. Moreover, there was no significant difference in COL-Ⅳ contents between the DGC10 and DGC20 groups (p > 0.05). Based on these findings, we hypothesized that the interactions among the platforms, PDA, GO, and COL-Ⅳ might be approaching saturation once the DGC nanofilms was up to 10 layers. Therefore, we have chosen to focus our further investigations, including nanofilm thickness, protein release profiles, gene delivery, and biological evaluations, exclusively on DGC10.
To introduce bioactive agents, peptides, proteins, drugs, or therapeutic genes should be flexibly mobilized onto the titanium surface to achieve sustainable release. In this study, the BSA and adenoviral vectors were chosen as the two model bioactive agents to evaluate the delivery and sustained-release capability of the H-DGC10. BSA is a standard model protein for evaluating protein adhesion and drug delivery owing to its high availability and well-understood properties. Our results demonstrate that the sustained-release ability of the H-DGC10 was better than that of A-DGC10, suggesting excellent reactivity of the H-DGC10 for delivery applications. We hypothesized that the H-DGC10 possessed more residual hydroxyl, catechol, amino, or carboxyl groups to produce a large number of reactive sites and electrostatic interactions for substance loading and sustained release. The H group, characterized by its superhydrophilic surface and abundance of hydroxyl groups, might lead to a more interactive initial DA/GO/COL-Ⅳ layers on surfaces. These layers subsequently created additional binding sites for loading and controlled release of other components. As a result, H-DGC10 nanofilms achieved a superior sustained-release profile compared to A-DGC10. While DA and GO were incorporated into our multilayer nanofilms owing to their universal adhesive property suitable for numerous organic/inorganic surfaces, further investigations are required to understand the specific interactions between the nanofilms and different substances at different concentrations.
The timely establishment of peri-implant soft-tissue integration requires 7 days for initial epithelial attachment, and another 2–8 weeks for epithelial and connective tissue maturation (Hämmerle et al., 2014; Guo et al., 2021). Consequently, the release of bioactive agents during soft-tissue integration is crucial. Our results indicate that the drug release of the H-DGC10 group was sustained for 2 weeks, still retaining 40% unreleased; this supported the initial attachment of peri-implant soft tissue. In other words, the release profile of the H-DGC10 conforms to the soft-tissue healing process.
Viral vectors are widely used in gene therapy owing to their high transfection efficiency (Lee et al., 2017). However, unlike negatively charged nucleic acids, viral vectors can be difficult to immobilize on titanium surfaces for in situ gene delivery. Substrate-mediated gene delivery—a method to immobilize therapeutic genes directly on the substrate—has been reported to improve transfection efficiency by increasing the local gene concentration, leading to reduced gene doses and favorable biosecurity (Mantz et al., 2019; Laird et al., 2020; Puhl et al., 2023). However, therapeutic genes were more or less exposed to the process of loading or modification in most studies. Zhang et al. (Zhang et al., 2018) functionalized a titanium-coated surface with anti-adenovirus antibodies to immobilize adenovirus vectors. However, this functionalization method proved to be too limited for widespread application in other vectors. This study designed a more flexible coating for in situ gene delivery, and the H-DGC10 displayed excellent transduction efficiency of up to 90% with a low dose of vectors, thereby improving the biosafety. We inferred that the PDA and GO layers on H-DGC10 provided plenty of reactive sites for immobilizing gene vectors.
The junctional epithelium, which seals the peri-implant soft tissue, attaches to the transmucosal implant surfaces through cell-matrix structures comprising hemidesmosomes (HDs) and the basement membrane (BM) (Gould et al., 1984; Atsuta et al., 2019). Our results show that the thickness of the DGC nanofilms on the A-DGC10 and H-DGC10 was around 200 nm, which is similar to the bond width of the BM (60 nm–150 nm) (Stern, 1981; Atsuta et al., 2016). We hypothesized that DGC nanofilms play biomimetic and early adhesive roles in the initial epithelial sealing stage. When the DGC nanofilms disintegrates (Figure 5C), the cell-material interface is gradually constructed with a natural matrix secreted by the epithelial cells.
Our in vitro investigations show that the DGC nanofilms grown on different substrates exhibited good biocompatibility with the HOECs. Laminin 332, a pivotal BM protein, has been reported to interact with integrin α6β4 to modulate cell adhesion and HD formation (Larjava et al., 2011; Taniguchi et al., 2020; Te Molder et al., 2021). Mirjam et al. (Nievers et al., 2000) verified that integrin β4 could bind to HD1/plectin to form HD-like structures. In this study, the H-DGC10 significantly promoted the clustering of integrin β4 and the gene expression levels of integrin β4 and laminin 332, which could participate in HD formation. Additionally, the plentiful binding sites on the H-DGC10 surfaces could improve the adhesion of the HOECs, and the COL-Ⅳ molecules could interact with membrane receptors to facilitate the secretion of functional matrixes for the BM assembly and cell anchorage (Khoshnoodi et al., 2008; Takeuchi et al., 2010). We speculated that the superior performance of H-DGC10 could be attributed to the larger amount of active binding sites facilitating initial ECM protein adsorption, as well as a greater encapsulation of COL-Ⅳ with bioinspired properties when compared to other groups.
In this study, we investigated the characteristics of DGC nanofilms on different titanium surfaces, their potential application as in situ carrying, sustained releasing, and delivering agents, and their effects on epithelial cells. However, this study had a few limitations with respect to the detailed analysis of the interactions in the LBL process, comprehensive evaluation of the H-DGCn as a versatile carrier, and its unique biological properties. Therefore, follow-up studies should be conducted to thoroughly evaluate the effects of the H-DGCn on the controlled release profile in vitro and in vivo, as well as its biological effectiveness, including its immunomodulatory and antibacterial properties. Despite the limitations of this study, it remains particularly valuable for the long-term success of titanium implants, where versatile therapeutic strategies could be required in emergencies.
5 Conclusion
In this study, multilayer DGC nanofilms were fabricated on different Ti surfaces using the LBL technique. The hydrogenated TNTs proved to be the best platform for coating the DGC nanofilms, which achieved stability after the number of nanofilm layers increased to 10. The H-DGC10 displayed excellent loading capacity, sustained-release capability, and in situ gene delivery abilities. In vitro investigations demonstrated the biocompatibility of the H-DGC10 in epithelial cells. Consequently, a versatile and bioactive DGC multilayer coating could be developed on hydrogenated TNTs for expanded clinical applications.
Data availability statement
The original contributions presented in the study are included in the article/Supplementary Material, further inquiries can be directed to the corresponding author.
Ethics statement
Ethical approval was not required for the studies on humans in accordance with the local legislation and institutional requirements because only commercially available established cell lines were used.
Author contributions
CW: Conceptualization, Data curation, Funding acquisition, Investigation, Methodology, Project administration, Visualization, Writing–original draft, Writing–review and editing. RL: Methodology, Resources, Writing–review and editing. XC: Methodology, Writing–review and editing. YM: Methodology, Writing–review and editing. SC: Conceptualization, Funding acquisition, Project administration, Supervision, Writing–review and editing.
Funding
The author(s) declare that financial support was received for the research, authorship, and/or publication of this article. This work was supported by Beijing Stomatological Hospital, Capital Medical University Young Scientist Program [NO. YSP202108] and Beijing Hospitals Authority’s Ascent Plan [NO. DFL20221301].
Acknowledgments
We would like to thank Editage (www.editage.cn) for English language editing.
Conflict of interest
The authors declare that the research was conducted in the absence of any commercial or financial relationships that could be construed as a potential conflict of interest.
Publisher’s note
All claims expressed in this article are solely those of the authors and do not necessarily represent those of their affiliated organizations, or those of the publisher, the editors and the reviewers. Any product that may be evaluated in this article, or claim that may be made by its manufacturer, is not guaranteed or endorsed by the publisher.
References
Ahamed, A. S., Prakash, P. S. G., Crena, J., Victor, D. J., Subramanian, S., and Appukuttan, D. (2021). The influence of laser-microgrooved implant and abutment surfaces on mean crestal bone levels and peri-implant soft tissue healing: a 3-year longitudinal randomized controlled clinical trial. Int. J. Implant Dent. 7, 102. doi:10.1186/s40729-021-00382-3
Areid, N., Abushahba, F., Riivari, S., Närhi, T., and Casarin, R. (2024). Effect of TiO2 abutment coatings on peri-implant soft tissue behavior: a systematic review of in vivo studies. Int. J. Dent. 2024, 1–13. doi:10.1155/2024/9079673
Atsuta, I., Ayukawa, Y., Furuhashi, A., Narimatsu, I., Kondo, R., Oshiro, W., et al. (2019). Epithelial sealing effectiveness against titanium or zirconia implants surface. J. Biomed. Mater Res. A 107, 1379–1385. doi:10.1002/jbm.a.36651
Atsuta, I., Ayukawa, Y., Kondo, R., Oshiro, W., Matsuura, Y., Furuhashi, A., et al. (2016). Soft tissue sealing around dental implants based on histological interpretation. J. Prosthodont Res. 60, 3–11. doi:10.1016/j.jpor.2015.07.001
Ball, V. (2018). Polydopamine nanomaterials: recent advances in synthesis methods and applications. Front. Bioeng. Biotechnol. 6, 109. doi:10.3389/fbioe.2018.00109
Boda, S. K., and Aparicio, C. (2022). Dual keratinocyte-attachment and anti-inflammatory coatings for soft tissue sealing around transmucosal oral implants. Biomater. Sci. 10, 665–677. doi:10.1039/d1bm01649k
Cai, Z., Shi, J., Li, W., Wu, Y., Zhang, Y., Zhang, S., et al. (2019). Mussel-inspired pH-switched assembly of capsules with an ultrathin and robust nanoshell. ACS Appl. Mater Interfaces 11, 28228–28235. doi:10.1021/acsami.9b11445
Chen, X., Liu, L., Yu, P. Y., and Mao, S. S. (2011). Increasing solar absorption for photocatalysis with black hydrogenated titanium dioxide nanocrystals. Science 331, 746–750. doi:10.1126/science.1200448
Coelho-Sampaio, T., Tenchov, B., Nascimento, M. A., Hochman-Mendez, C., Morandi, V., Caarls, M. B., et al. (2020). Type IV collagen conforms to the organization of polylaminin adsorbed on planar substrata. Acta Biomater. 111, 242–253. doi:10.1016/j.actbio.2020.05.021
Gao, F., Hu, Y., Li, G., Liu, S., Quan, L., Yang, Z., et al. (2020). Layer-by-layer deposition of bioactive layers on magnesium alloy stent materials to improve corrosion resistance and biocompatibility. Bioact. Mater. 5, 611–623. doi:10.1016/j.bioactmat.2020.04.016
Gould, T. R., Westbury, L., and Brunette, D. M. (1984). Ultrastructural study of the attachment of human gingiva to titanium in vivo. J. Prosthet. Dent. 52, 418–420. doi:10.1016/0022-3913(84)90459-1
Guo, T., Gulati, K., Arora, H., Han, P., Fournier, B., and Ivanovski, S. (2021). Orchestrating soft tissue integration at the transmucosal region of titanium implants. Acta Biomater. 124, 33–49. doi:10.1016/j.actbio.2021.01.001
Hämmerle, C. H. F., and Giannobile, W. V.Working Group 1 of the European Workshop on Peri, and odontology (2014). Biology of soft tissue wound healing and regeneration--consensus report of Group 1 of the 10th European Workshop on Periodontology. J. Clin. Periodontol. 41 (Suppl. 15), 1–5. doi:10.1111/jcpe.12221
Hoseini-Ghahfarokhi, M., Mirkiani, S., Mozaffari, N., Abdolahi Sadatlu, M. A., Ghasemi, A., Abbaspour, S., et al. (2020). Applications of graphene and graphene oxide in smart drug/gene delivery: is the world still flat? Int. J. Nanomedicine 15, 9469–9496. doi:10.2147/ijn.S265876
Huang, S., Liang, N., Hu, Y., Zhou, X., and Abidi, N. (2016). Polydopamine-assisted surface modification for bone biosubstitutes. Biomed. Res. Int. 2016, 1–9. doi:10.1155/2016/2389895
Iacov-Craitoiu, M. M., and Craitoiu, M. (2020). Clinical, histopathological and immunohistochemical behavior of peri-implant soft tissue. Rom. J. Morphol. Embryol. 61, 121–128. doi:10.47162/RJME.61.1.13
Inchingolo, F., Inchingolo, A. M., Latini, G., Palmieri, G., Di Pede, C., Trilli, I., et al. (2023). Application of graphene oxide in oral surgery: a systematic review. Materials 16, 6293. doi:10.3390/ma16186293
Khoshnoodi, J., Pedchenko, V., and Hudson, B. G. (2008). Mammalian collagen IV. Microsc. Res. Tech. 71, 357–370. doi:10.1002/jemt.20564
Kulkarni, M., Flašker, A., Lokar, M., Mrak-Poljšak, K., Mazare, A., Artenjak, A., et al. (2015). Binding of plasma proteins to titanium dioxide nanotubes with different diameters. Int. J. Nanomedicine 10, 1359–1373. doi:10.2147/ijn.S77492
Kunrath, M. F., and Gerhardt, M. d. N. (2023). Trans-mucosal platforms for dental implants: strategies to induce muco-integration and shield peri-implant diseases. Dent. Mater 39, 846–859. doi:10.1016/j.dental.2023.07.009
Kutwin, M., Sosnowska, M. E., Strojny-Cieślak, B., Jaworski, S., Trzaskowski, M., Wierzbicki, M., et al. (2021). MicroRNA delivery by graphene-based complexes into glioblastoma cells. Molecules 26, 5804. doi:10.3390/molecules26195804
Laird, N. Z., Malkawi, W. I., Chakka, J. L., Acri, T. M., Elangovan, S., and Salem, A. K. (2020). A proof of concept gene-activated titanium surface for oral implantology applications. J. Tissue Eng. Regen. Med. 14, 622–632. doi:10.1002/term.3026
Larjava, H., Koivisto, L., Häkkinen, L., and Heino, J. (2011). Epithelial integrins with special reference to oral epithelia. J. Dent. Res. 90, 1367–1376. doi:10.1177/0022034511402207
Lee, C. S., Bishop, E. S., Zhang, R., Yu, X., Farina, E. M., Yan, S., et al. (2017). Adenovirus-mediated gene delivery: potential applications for gene and cell-based therapies in the new era of personalized medicine. Genes Dis. 4, 43–63. doi:10.1016/j.gendis.2017.04.001
Lee, H., Dellatore, S. M., Miller, W. M., and Messersmith, P. B. (2007). Mussel-inspired surface chemistry for multifunctional coatings. Science 318, 426–430. doi:10.1126/science.1147241
Li, D., Müller, M. B., Gilje, S., Kaner, R. B., and Wallace, G. G. (2008). Processable aqueous dispersions of graphene nanosheets. Nat. Nanotechnol. 3, 101–105. doi:10.1038/nnano.2007.451
Lin, J., Cai, W., Peng, Q., Meng, F., Zhang, D., and Chen, J. (2021). Preparation of TiO2 nanotube array on the pure titanium surface by anodization method and its hydrophilicity. Scanning 2021, 1–7. doi:10.1155/2021/2717921
Lin, Q., Ding, X., Qiu, F., Song, X., Fu, G., and Ji, J. (2010). In situ endothelialization of intravascular stents coated with an anti-CD34 antibody functionalized heparin-collagen multilayer. Biomaterials 31, 4017–4025. doi:10.1016/j.biomaterials.2010.01.092
Liu, M., Zeng, G., Wang, K., Wan, Q., Tao, L., Zhang, X., et al. (2016). Recent developments in polydopamine: an emerging soft matter for surface modification and biomedical applications. Nanoscale 8, 16819–16840. doi:10.1039/c5nr09078d
Liu, S., Yu, J.-M., Gan, Y.-C., Qiu, X.-Z., Gao, Z.-C., Wang, H., et al. (2023a). Biomimetic natural biomaterials for tissue engineering and regenerative medicine: new biosynthesis methods, recent advances, and emerging applications. Mil. Med. Res. 10, 16. doi:10.1186/s40779-023-00448-w
Liu, S., Zhang, C., Zhou, Y., Zhang, F., Duan, X., Liu, Y., et al. (2023b). MRI-visible mesoporous polydopamine nanoparticles with enhanced antioxidant capacity for osteoarthritis therapy. Biomaterials 295, 122030. doi:10.1016/j.biomaterials.2023.122030
Lu, R., Wang, C., Wang, X., Wang, Y., Wang, N., Chou, J., et al. (2018). Effects of hydrogenated TiO2 nanotube arrays on protein adsorption and compatibility with osteoblast-like cells. Int. J. Nanomedicine 13, 2037–2049. doi:10.2147/ijn.S155532
Mantz, A., Rosenthal, A., Farris, E., Kozisek, T., Bittrich, E., Nazari, S., et al. (2019). Free polyethylenimine enhances substrate-mediated gene delivery on titanium substrates modified with RGD-functionalized poly(acrylic acid) brushes. Front. Chem. 7, 51. doi:10.3389/fchem.2019.00051
Martinez-Marquez, D., Gulati, K., Carty, C. P., Stewart, R. A., and Ivanovski, S. (2020). Determining the relative importance of titania nanotubes characteristics on bone implant surface performance: a quality by design study with a fuzzy approach. Mater Sci. Eng. C Mater Biol. Appl. 114, 110995. doi:10.1016/j.msec.2020.110995
Mathur, A., Kharbanda, O. P., Koul, V., Dinda, A. K., Anwar, M. F., and Singh, S. (2022). Fabrication and evaluation of antimicrobial biomimetic nanofiber coating for improved dental implant bioseal: an in vitro study. J. Periodontol. 93, 1578–1588. doi:10.1002/jper.21-0255
Mendonca, G., Mendonca, D. B., Aragao, F. J., and Cooper, L. F. (2008). Advancing dental implant surface technology--from micron-to nanotopography. Biomaterials 29, 3822–3835. doi:10.1016/j.biomaterials.2008.05.012
Nievers, K. I., Geerts, D., Leigh, I. M., and Sonnenberg, A. (2000). Formation of hemidesmosome-like structures in the absence of ligand binding by the α6β4 integrin requires binding of HD1/plectin to the cytoplasmic domain of the β4 integrin subunit. J. Cell Sci. 113, 963–973. doi:10.1242/jcs.113.6.963
Palchesko, R. N., Funderburgh, J. L., and Feinberg, A. W. (2016). Engineered basement membranes for regenerating the corneal endothelium. Adv. Healthc. Mater 5, 2942–2950. doi:10.1002/adhm.201600488
Park, J., Cimpean, A., Tesler, A. B., and Mazare, A. (2021). Anodic TiO(2) nanotubes: tailoring osteoinduction via drug delivery. Nanomater. (Basel) 11, 2359. doi:10.3390/nano11092359
Peng, L., Mendelsohn, A. D., LaTempa, T. J., Yoriya, S., Grimes, C. A., and Ta, D. (2009). Long-term small molecule and protein elution from TiO2 nanotubes. Nano Lett. 9, 1932–1936. doi:10.1021/nl9001052
Puhl, D. L., Funnell, J. L., Fink, T. D., Swaminathan, A., Oudega, M., Zha, R. H., et al. (2023). Electrospun fiber-mediated delivery of neurotrophin-3 mRNA for neural tissue engineering applications. Acta Biomater. 155, 370–385. doi:10.1016/j.actbio.2022.11.025
Qin, S., Lu, Z., Gan, K., Qiao, C., Li, B., Chen, T., et al. (2022). Construction of a BMP-2 gene delivery system for polyetheretherketone bone implant material and its effect on bone formation in vitro. J. Biomed. Mater Res. B Appl. Biomater. 110, 2075–2088. doi:10.1002/jbm.b.35062
Radunovic, M., De Colli, M., De Marco, P., Di Nisio, C., Fontana, A., Piattelli, A., et al. (2017). Graphene oxide enrichment of collagen membranes improves DPSCs differentiation and controls inflammation occurrence. J. Biomed. Mater Res. A 105, 2312–2320. doi:10.1002/jbm.a.36085
Ramirez, M. C., and Osendi, M. I. (2022). Graphene-based materials, their composites, and potential applications. Materials 15, 7184. doi:10.3390/ma15207184
Raslan, A., Saenz del Burgo, L., Ciriza, J., and Pedraz, J. L. (2020). Graphene oxide and reduced graphene oxide-based scaffolds in regenerative medicine. Int. J. Pharm. 580, 119226. doi:10.1016/j.ijpharm.2020.119226
Saso, I., and Lee, R. (2018). Comparison of peri-implant and periodontal marginal soft tissues in health and disease. Periodontol 76, 116–130. doi:10.1111/prd.12150
Sharma, A., Gupta, S., Sampathkumar, T. S., and Verma, R. S. (2022). Modified graphene oxide nanoplates reinforced 3D printed multifunctional scaffold for bone tissue engineering. Biomater. Adv. 134, 112587. doi:10.1016/j.msec.2021.112587
Shrivas, S., Samaur, H., Yadav, V., and Boda, S. K. (2024). Soft and hard tissue integration around percutaneous bone-anchored titanium prostheses: toward achieving holistic biointegration. ACS Biomater. Sci. Eng. 10, 1966–1987. doi:10.1021/acsbiomaterials.3c01555
Stern, I. B. (1981). Current concepts of the dentogingival junction: the epithelial and connective tissue attachments to the tooth. J. Periodontol. 52, 465–476. doi:10.1902/jop.1981.52.9.465
Takeuchi, S., Matsunaga, T., Yamamoto, G., Baba, K., and Tachikawa, T. (2010). Temporal expression pattern of adhesion genes in human oral mucosal keratinocytes on type IV collagen-coated titanium. J. Biomed. Mater Res. A 95, 305–311. doi:10.1002/jbm.a.32814
Tamayo-Angorrilla, M., López de Andrés, J., Jiménez, G., and Marchal, J. A. (2022). The biomimetic extracellular matrix: a therapeutic tool for breast cancer research. Transl. Res. 247, 117–136. doi:10.1016/j.trsl.2021.11.008
Taniguchi, Y., Takizawa, M., Li, S., and Sekiguchi, K. (2020). Bipartite mechanism for laminin-integrin interactions: identification of the integrin-binding site in LG domains of the laminin α chain. Matrix Biol. 87, 66–76. doi:10.1016/j.matbio.2019.10.005
Te Molder, L., de Pereda, J. M., and Sonnenberg, A. (2021). Regulation of hemidesmosome dynamics and cell signaling by integrin α6β4. J. Cell Sci. 134, jcs259004. doi:10.1242/jcs.259004
Tiwari, S., Patil, R., Dubey, S. K., and Bahadur, P. (2020). Graphene nanosheets as reinforcement and cell-instructive material in soft tissue scaffolds. Adv. Colloid Interface Sci. 281, 102167. doi:10.1016/j.cis.2020.102167
Weinstein, T., Clauser, T., Del Fabbro, M., Deflorian, M., Parenti, A., Taschieri, S., et al. (2020). Prevalence of peri-implantitis: a multi-centered cross-sectional study on 248 patients. Dent. J. (Basel) 8, 80. doi:10.3390/dj8030080
Wu, X., Li, L., Tao, W., Hong, H., Zhang, L., Zheng, S., et al. (2023). Built-up sodium alginate/chlorhexidine multilayer coating on dental implants with initiating anti-infection and cyto-compatibility sequentially for soft-tissue sealing. Biomater. Adv. 151, 213491. doi:10.1016/j.bioadv.2023.213491
Xie, X., Tang, J., Xing, Y., Wang, Z., Ding, T., Zhang, J., et al. (2021). Intervention of polydopamine assembly and adhesion on nanoscale interfaces: state-of-the-art designs and biomedical applications. Adv. Healthc. Mater 10, e2002138. doi:10.1002/adhm.202002138
Xu, K., Zhou, M., Chen, W., Zhu, Y., Wang, X., Zhang, Y., et al. (2021). Bioinspired polydopamine/graphene oxide/collagen nanofilms as a controlled release carrier of bioactive substances. Chem. Eng. J. 405, 126930. doi:10.1016/j.cej.2020.126930
You, X., Wang, Z., Wang, L., Liu, Y., Chen, H., Lan, X., et al. (2024). Graphene oxide/ε-poly-L-lysine self-assembled functionalized coatings improve the biocompatibility and antibacterial properties of titanium implants. Front. Bioeng. Biotechnol. 12, 1381685. doi:10.3389/fbioe.2024.1381685
Zeng, J., Sasaki, N., Correia, C. R., Mano, J. F., and Matsusaki, M. (2020). Fabrication of artificial nanobasement membranes for cell compartmentalization in 3D tissues. Small 16, e1907434. doi:10.1002/smll.201907434
Zhang, J., Wang, H., Wang, Y., Dong, W., Jiang, Z., and Yang, G. (2018). Substrate-mediated gene transduction of LAMA3 for promoting biological sealing between titanium surface and gingival epithelium. Colloids Surf. B Biointerfaces 161, 314–323. doi:10.1016/j.colsurfb.2017.10.030
Keywords: titanium implants, nanofilms, bioinspired design, sustained-release carrier, multifunctional surface
Citation: Wang C, Lu R, Cao X, Mu Y and Chen S (2024) Multifunctional and bioinspired titanium surface with multilayer nanofilms for novel dental implant applications. Front. Chem. 12:1426865. doi: 10.3389/fchem.2024.1426865
Received: 02 May 2024; Accepted: 17 June 2024;
Published: 05 July 2024.
Edited by:
Agata Blacha-Grzechnik, Silesian University of Technology, PolandReviewed by:
Rajnish Kumar, University of North Carolina at Chapel Hill, United StatesPreeti Gupta, Leibniz Institute for Solid State and Materials Research Dresden (IFW Dresden), Germany
Kui Xu, Anhui University of Chinese Medicine, China
Copyright © 2024 Wang, Lu, Cao, Mu and Chen. This is an open-access article distributed under the terms of the Creative Commons Attribution License (CC BY). The use, distribution or reproduction in other forums is permitted, provided the original author(s) and the copyright owner(s) are credited and that the original publication in this journal is cited, in accordance with accepted academic practice. No use, distribution or reproduction is permitted which does not comply with these terms.
*Correspondence: Su Chen, Y2hlbnN1QG1haWwuY2NtdS5lZHUuY24=