- 1Department of Chemistry, Universidad Técnica Particular de Loja, Loja, Ecuador
- 2INFN-Laboratori Nazionali di Frascati, Frascati, Italy
- 3Surface Nanoscience Group, Department of Physics, University of Calabria, Rende, Italy
- 4Departamento de Producción, Facultad de Ciencias Exactas y Naturales, Universidad Técnica Particular de Loja, Loja, Ecuador
- 5Grupo de Investigación en Materiales Avanzados (GIMA), Facultad de Ciencias, Escuela Superior Politécnica de Chimborazo (ESPOCH), Riobamba, Ecuador
- 6Faculty of Mechanical Engineering, Escuela Superior Politécnica de Chimborazo (ESPOCH), Riobamba, Ecuador
- 7Department of Information Engineering, Polytechnic University of Marche, Ancona, Italy
- 8UNICARIBE Research Center, University of Calabria, Rende, Italy
- 9Laboratorio de Nanotecnología, Area de Ciencias Básicas y Ambientales, Instituto Tecnológico de Santo Domingo, Santo Domingo, Dominican Republic
- 10Nanoresearch Laboratory, Excellent Center, Baku State University, Baku, Azerbaijan
Pathogen-induced infections and the rise of antibiotic-resistant bacteria, such as Escherichia coli (E. coli) and Staphylococcus aureus (S. aureus), pose significant global health challenges, emphasizing the need for new antimicrobial strategies. In this study, we synthesized graphene oxide (GO)-based composites functionalized with silver nanoparticles (AgNPs) and copper nanoparticles (CuNPs) as potential alternatives to traditional antibiotics. The objective is to assess the antibacterial properties of these composites and explore their efficacy against E. coli and S. aureus, two common bacterial pathogens. The composites are prepared using eco-friendly and conventional methods to ensure effective nanoparticle attachment to the GO surface. Structural and morphological characteristics are confirmed through SEM, AFM, EDS, XRD, UV-vis, FTIR, and Raman spectroscopy. The antibacterial efficacy of the composites is tested through disk diffusion assays, colony-forming unit (CFU) counts, and turbidimetry analysis, with an emphasis on understanding the effects of different nanoparticle concentrations. The results demonstrated a dose-dependent antibacterial effect, with GO/AgNP-1 showing superior antibacterial activity over GO/AgNP-2, particularly at lower concentrations (32.0 μg/mL and 62.5 μg/mL). The GO/CuNP composite also exhibited significant antibacterial properties, with optimal performance at 62.5 μg/mL for both bacterial strains. Turbidimetry analysis confirmed the inhibition of bacterial growth, especially at moderate concentrations, although slight nanoparticle aggregation at higher doses reduced efficacy. Lastly, both GO/AgNP and GO/CuNP composites demonstrated significant antibacterial potential. The results emphasize the need to fine-tune nanoparticle concentration and refine synthesis techniques to improve their efficacy, positioning these composites as strong contenders for antimicrobial use.
1 Introduction
Despite significant advancements in medical science, pathogen-induced infections remain a pressing global health challenge, aggravated by the emergence and spread of antibiotic-resistant bacterial strains, commonly referred to as “superbacteria” (Cao et al., 2022; Zhang et al., 2024). The overuse and misuse of antibiotics have accelerated the rise of these resistant strains, stressing the urgent need for alternative antimicrobial strategies that can effectively combat bacterial infections without contributing to antibiotic resistance (Mithuna et al., 2024; Raghavan and Kim, 2024). In this context, nanotechnology offers a promising solution, particularly through the development of nanomaterials and nanoparticle-based composites, which have demonstrated remarkable potential in antimicrobial applications due to their unique properties, including high surface area, chemical reactivity, and tunable antibacterial effects (Yadav et al., 2024; Izadi et al., 2024).
Among the nanomaterials that have garnered attention, graphene oxide (GO) and metal nanoparticles—especially silver or copper nanoparticles—stand out for their promising antibacterial capabilities (Barani et al., 2024; Faid et al., 2024). GO, a highly oxidized form of graphene (Jaramillo-Fierro and Cuenca, 2024; Vacacela Gomez et al., 2016), features functional groups that not only enhance its dispersibility in aqueous environments but also enable it to act as an excellent platform for the stabilization of metal nanoparticles (Badoni and Prakash, 2024). This makes GO a versatile material for the development of hybrid and novel nanocomposites with enhanced antibacterial properties.
On the other hand, silver nanoparticles (AgNPs) have been widely recognized for their broad-spectrum antibacterial, antifungal, and antiviral activities (Said et al., 2024; Ohiduzzaman et al., 2024). Their mechanisms of action, which include the release of silver ions and the generation of reactive oxygen species (ROS), allow them to effectively disrupt bacterial cell membranes and interfere with intracellular processes such as DNA replication, leading to cell death (Alfei et al., 2024). Also, copper nanoparticles (CuNPs), although less explored than AgNPs, offer a more cost-effective alternative with comparable antimicrobial efficacy. Like AgNPs, CuNPs release ions and produce ROS, which disrupt bacterial structures and processes (Salas-Orozco et al., 2024; Ivanova et al., 2024).
Keeping this in mind, combining GO with metal nanoparticles such as AgNPs and CuNPs has shown significant promise in enhancing antibacterial efficacy (Vodnik et al., 2024). In particular, GO can serve as a stable platform that prevents nanoparticle aggregation and facilitates the controlled release of metal ions, thereby improving the whole antimicrobial performance of the composite materials. Furthermore, the ability of GO to produce ROS and interact with bacterial membranes enhances the antibacterial activity of the composite (Rojas-Andrade et al., 2017). Recent studies have demonstrated that GO/AgNP composites exhibit superior antibacterial properties compared to their individual components (Jin et al., 2023). However, the exploration of GO/CuNP composites in this context has been limited.
Given the potential of both silver and copper nanoparticles in combating bacterial infections, the motivation for this study lies in the synthesis, characterization, and evaluation of GO-based composites decorated with AgNPs and CuNPs for antibacterial applications. These composites represent an interesting class of materials with enhanced antimicrobial properties that could offer effective alternatives to traditional antibiotics. Specifically, this study focuses on the eco-friendly synthesis of GO/metal nanoparticle composites using a green chemistry approach (Tene et al., 2024). In particular, silver nitrate (AgNO₃) is reduced to its respective nanoparticles using Calendula officinalis extract, which serves as a natural reducing and stabilizing agent (Tene et al., 2024). The preparation of CuNPs follows a conventional approach (Dang et al., 2011).
The synthesized nanoparticles are then incorporated into GO dispersions to create GO/AgNP and GO/CuNP composites, which are thoroughly characterized using various morphological and spectroscopic techniques. These techniques allow for detailed analysis of the composite structure, surface characteristics, and chemical composition, providing valuable insights into how these properties influence their antibacterial efficacy. To assess the antibacterial properties of the prepared composites, we employ disk diffusion assays, minimum bactericidal concentration (MBC) determination, and colony-forming unit (CFU) counts, using Escherichia coli (Gram-negative) and Staphylococcus aureus (Gram-positive) as model bacteria. By comparing the antibacterial activity of GO/AgNP and GO/CuNP composites, we aim to highlight the potential of silver or copper nanoparticles as powerful alternatives, thereby broadening the scope of nanotechnology in combating bacterial infections.
2 Materials and methods
2.1 Materials
All chemicals enlisted below were used in their received form without undergoing further purification. The synthesis of the composites is illustrated in Figure 1.
• Graphite powder (<150 μm, 99.99%, Sigma Aldrich).
• H2SO4 (sulfuric acid, ACS reagent, 95.0%–98.0%, Sigma Aldrich).
• KMnO4 (potassium permanganate, ACS reagent, ≥99.0%, Sigma Aldrich).
• HCl (Hydrochloric acid, ACS reagent, 37%, Sigma Aldrich).
• H2O2 (hydrogen peroxide, 30%, Merk).
• AgNO3 (silver nitrate, ACS reagent, ≥99.0%, Sigma Aldrich).
• Na3C6H5O7 (sodium citrate dihydrate, ACS reagent, ≥99.0%, Sigma Aldrich).
• NaCl (sodium chloride, ACS reagent, ≥99.0%).
• CuSO4 · 5H2O (Copper (II) sulfate pentahydrate salt, ≥98% purity, Merck).
• NaBH4 (Sodium borohydride, ≥99%, Sigma-Aldrich).
• C6H8O7 (Citric acid, ACS reagent, ≥99.5%, Sigma Aldrich).
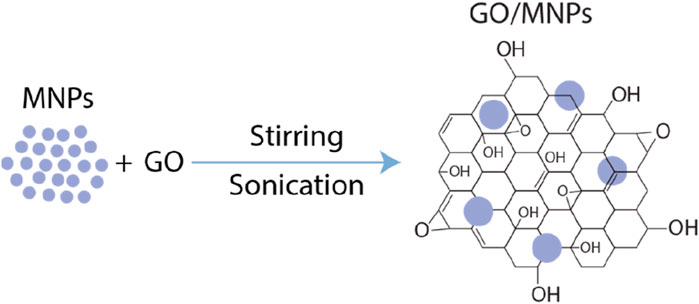
Figure 1. Schematic representation of the synthesis process of metal nanoparticles (MNPs) on graphene oxide (GO).
2.2 Preparation of GO
The synthesis of GO followed the methodology detailed in our previous works (Tene et al., 2020; Cruz Salazar et al., 2023; Tene et al., 2022). Initially, 3.0 g of graphite powder was dispersed in 70.0 mL of concentrated sulfuric acid under continuous stirring. The mixture was cooled in an ice bath, and 9.0 g of potassium permanganate was slowly added, ensuring the temperature remained below 20°C. The solution was then heated to 50°C in a water bath for 30 min, while stirring was maintained. Following this, 150 mL of distilled water was added incrementally over 20 min, keeping the temperature under 90°C. Subsequently, 500 mL of distilled water and 15 mL of H₂O₂ were introduced. After an hour, the resulting precipitate was subjected to several centrifugation cycles (10,000 rpm for 10 min each) with a 1:10 HCl/distilled water solution for thorough washing. The material was then dried at 45°C for 48 h to produce graphite oxide powder. This powder was sonicated in 500 mL of distilled water for 30 min, centrifuged at 500 rpm for 10 min, and then dried once again at 45°C for another 48 h.
2.3 Synthesis of metal nanoparticles
AgNPs were synthesized following the Turkevich method (Turkevich et al., 1951). In brief, 150 mg of AgNO₃ was dissolved in 50 mL of ultrapure water and refluxed for 40 min. Once the solution reached boiling, 25 mL of Calendula officinalis seed extract was added dropwise. The reaction was maintained at 110°C–130°C for 30 min. The resulting AgNP dispersion was dialyzed for 24 h in ultrapure water to remove residual salts and then stored in a light-protected, chilled container. These nanoparticles, designated as AgNP-1, had a measured pH of 6.11.
Then, a solution of 5.0 g of AgNO₃ in 500 mL of ultrapure water was heated to reflux. Once the temperature reached 110°C, 5.0 g of sodium citrate dissolved in 50 mL of ultrapure water was added dropwise. The reaction was maintained at 130°C for 50 min under constant conditions. The nanoparticles produced were labeled AgNP-2, with a measured pH of 6.03.
On the other hand, the synthesis of CuNPs began under the following approach (Dang et al., 2011) by dissolving copper (II) sulfate pentahydrate (0.01 M) in deionized water. Polyethylene glycol 6000 (PEG 6000, 0.02 M) was then added. Ascorbic acid (0.02 M) and sodium hydroxide (0.1 M) were subsequently introduced. To conclude, a sodium borohydride (NaBH₄, 0.1 M) solution was added under rapid stirring, leading to an immediate color change from yellow to black/red, indicating the onset of the reduction reaction. These nanoparticles, designated as CuNPs, had a measured pH of 6.07.
2.4 Preparation of GO/AgNP composite
To simplify the process, the functionalization of AgNPs (i.e., AgNP-1 and AgNP-2) and CuNPs onto the GO surface begins by dissolving GO in ultrapure water to a concentration of 650 μg/mL. Aqueous AgNP solutions were then prepared at varying concentrations: 32.0 μg/mL, 62.5 μg/mL, 125.0 μg/mL, and 250.0 μg/mL. The composites were formed by mixing 10 mL of the GO solution with 10 mL of each nanoparticle solution. This mixture was stirred at 1,000 rpm for 15 min, followed by 10 min of sonication at 400 Hz. Afterward, the solution was stirred again for 15 min at 1,000 rpm and finally centrifuged at 13,000 rpm for 10 min to separate the composites. The entire procedure was conducted at room temperature.
2.5 Culturing of testing bacteria
The culture medium for E. coli (E. coli) consisted of Luria Bertani (LB) broth, prepared by dissolving 5.0 g/L of sodium chloride, 5.0 g/L of yeast extract, and 10.0 g/L of casein peptone in distilled water, followed by sterilization through autoclaving. Once the LB agar plates were prepared, E. coli was inoculated and incubated at 37°C for 24 h. After incubation, E. coli colonies were harvested using a sterilized loop and suspended in sterile saline or peptone water. Serial dilutions were performed by transferring 1 mL of the bacterial suspension into 9 mL of sterile diluent, repeating the process until the desired dilution was reached. The turbidity of the final suspension was adjusted to correspond to the 0.5 McFarland standard, which is approximately 1.5 × 108 CFU/mL. The same procedure was applied for S. aureus (S. aureus).
2.6 Antibacterial tests
The following tests have been employed in the present study:
• Mueller-Hinton agar plates were prepared, and 100 µL of bacterial inoculum, standardized to 107 CFU/mL, was evenly spread across the surface using a sterile spreader for uniform distribution. Disks, 5 mm in diameter, were pre-soaked with 20 µL of six different solutions (32.0 μg/mL, 62.5 μg/mL, 125.0 μg/mL, 250.0 μg/mL of the composite solution, distilled water as a negative control, and a GO solution as a comparison standard) and placed on the agar surface using sterile forceps to avoid cross-contamination. The plates were incubated at 37°C, and the zones of inhibition were measured after 24 and 48 h to evaluate antibacterial activity, with measurements taken under visible light.
• For the MBC assay, test tubes containing 10 mL of Luria Bertani (LB) broth were prepared. To each tube, 20 µL of bacterial inoculum, standardized to approximately 1.5 × 108 CFU/mL (0.5 McFarland standard), was added along with 20 µL of composite solutions at varying concentrations (32.0 μg/mL, 62.5 μg/mL, 125.0 μg/mL, 250.0 μg/mL). The tubes were incubated at 37°C for 24 h. After incubation, bacterial growth was assessed by checking for turbidity, indicating suspended particles from bacterial presence. Tubes showing no turbidity suggested bacterial inhibition at the corresponding concentration. To confirm bacterial death (bactericidal activity) rather than mere inhibition, the contents of the non-turbid tubes were subcultured onto Tryptone Soy Agar plates. A 100 µL aliquot from each non-turbid tube was spread onto the plates, which were incubated at 37°C for an additional 24 h. Post-incubation, the plates were examined for bacterial colonies. The presence of colonies indicated that the bacteria were inhibited but not killed, classifying the composite as bacteriostatic at that concentration. In contrast, the absence of colonies confirmed bactericidal activity.
• For the CFU count, Mueller-Hinton agar plates were prepared. To each plate, a mixture of 100 µL of bacterial inoculum (standardized to approximately 1.5 × 108 CFU/mL, or 0.5 McFarland standard) and 100 µL of the composite solution at different concentrations (32.0 μg/mL, 62.5 μg/mL, 125.0 μg/mL, 250.0 μg/mL) was added. The plates were incubated at 37°C for 24 h. After incubation, the colonies were counted by dividing the plate into quadrants, counting the colonies in one quadrant, and multiplying by four to estimate the total colony count per plate (Tene et al., 2024).
2.7 Characterization
The absorption spectra for all composites were acquired using a Thermo Scientific Evolution 220 spectrophotometer. Fourier-transform infrared (FT-IR) spectra were obtained with a Jasco FT-IR 4000 spectrometer, while Raman spectra were collected using a Jasco NRS-500 spectrometer equipped with a 532 nm laser (×0.3mW, ×100 objective). X-ray diffraction (XRD) patterns were recorded on a Panalytical Pro X-ray diffractometer, utilizing Cu Kα radiation at 60 kV and 55 mA. Surface morphology and compositional analysis were carried out through scanning electron microscopy (SEM, JSM-IT100 InTouchScope, JEOL) combined with energy-dispersive X-ray spectroscopy (EDS) at an accelerating voltage of 15 kV. Additionally, atomic force microscopy (AFM) was conducted at room temperature using a NaioAFM system with an SMENA SFC050L scanning head in dynamic force mode to provide detailed surface characterization under near-operational conditions. The spectral data were processed using a 7-point moving average for smoothing.
3 Results and discussions
3.1 SEM and EDS characterization
To begin, Figure 2A shows the typical morphology of GO, characterized by large, flat sheets with visible wrinkles and folds, which are consistent with the inherent layered structure of GO (Tene et al., 2021; Tene et al., 2023a; Tene et al., 2023b). In contrast, Figure 2B shows the GO/AgNP-1 composite, where nanoparticles are uniformly distributed across the GO surface. This even dispersion suggests efficient anchoring of AgNPs onto the GO sheets, likely due to the stabilization provided by the oxygen-containing functional groups on GO. Figure 2C presents the GO/AgNP-2 composite, where the distribution of AgNPs is more heterogeneous. Clusters of AgNPs can be observed, with a broader size distribution indicating a polydisperse nature. This aggregation of nanoparticles suggests less control over nanoparticle size and dispersion during synthesis, leading to larger, uneven clusters on the GO surface. In Figure 2D, the GO/CuNP composite is shown, where CuNPs are dispersed across the GO sheets. The CuNPs appear more sparsely distributed compared to the AgNPs in the previous composites. This difference in distribution may be attributed to variations in the synthesis process or the interaction between CuNPs and the GO surface. Despite the sparser coverage, the CuNPs seem to be well-adhered to the GO sheets, suggesting that the functionalization process was successful in anchoring the copper nanoparticles onto the GO surface.
The EDS spectra and elemental analysis data (Figure 2; Supplementary Table S1) provide insights into the elemental composition and distribution of carbon (C), oxygen (O), silver (Ag), and copper (Cu) in the GO and composite materials. Figure 3A represents the EDS spectrum of pristine GO, showing the expected dominant peaks for carbon (C) and oxygen (O), which are the primary components of graphene oxide. According to Supplementary Table S1, GO consists of 58.12% C and 41.88% O, reflecting the oxidized nature of the material and confirming the successful synthesis of GO. Figure 3B shows the EDS spectrum for the GO/AgNP-1 composite. In addition to the prominent C and O peaks, distinct Ag peaks are observed, indicating the successful functionalization of GO with silver nanoparticles. The elemental analysis in Supplementary Table S1 reveals that the Ag content is 2.60%, while the C and O percentages are slightly reduced (53.23% and 44.17%, respectively) due to the introduction of AgNPs onto the GO surface. This suggests that the GO structure has been covered by silver nanoparticles, which is consistent with the SEM observations of uniform AgNP distribution (Figure 2B).
Figure 3C illustrates the EDS spectrum for the GO/AgNP-2 composite. Similar to GO/AgNP-1, Ag peaks are present, confirming the attachment of AgNPs. However, the Ag content (2.07%) is slightly lower compared to GO/AgNP-1, while the C (57.11%) and O (40.82%) contents are comparable to the pristine GO values. This decrease in Ag content, combined with the observed polydispersity in the SEM images (Figure 2C), suggests a less uniform distribution of AgNPs in this sample. Figure 3D displays the EDS spectrum for the GO/CuNP composite, where characteristic peaks for both copper (Cu) and oxygen (O) are clearly seen. The Cu content is notably higher, at 17.30%, as shown in Supplementary Table S1. This substantial copper loading is accompanied by a reduction in C (48.40%) and O (34.30%) content, reflecting the successful deposition of copper nanoparticles onto the GO sheets. The significant Cu percentage indicates efficient CuNP incorporation, which is consistent with the SEM image (Figure 2D) showing a sparse but well-adhered distribution of CuNPs on the GO surface.
3.2 AFM characterization
The AFM images in Figures 4A–C and the size distribution histograms in Figures 4D–F provide a clear representation of the surface morphology and nanoparticle size distribution for GO, GO/AgNP-1, and GO/CuNP composites. Although AFM images for GO/AgNP-2 are not displayed in Figure 4, they show similar results to GO/AgNP-1 in terms of particle size distribution and surface morphology, so the data for GO/AgNP-2 are omitted from the figure. Then, from Figure 4A, the AFM image of pristine GO shows its smooth, layered structure with typical flat sheets and no visible nanoparticle deposition. However, in Figure 4B (GO/AgNP-1), silver nanoparticles are clearly visible on the GO surface, with a relatively uniform distribution. Figure 4C illustrates the GO/CuNP composite, where copper nanoparticles are dispersed across the GO surface, though the nanoparticle density appears lower compared to GO/AgNP-1.
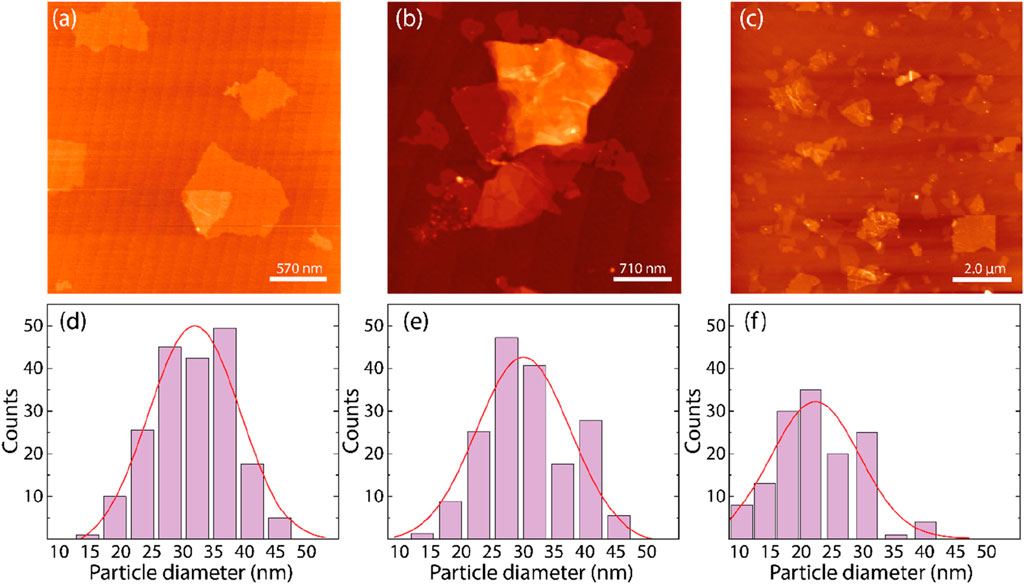
Figure 4. AFM images of (A) GO, (B) GO/AgNP-1, and (C) GO/CuNPs. Size distribution of silver nanoparticles onto (D) GO/AgNP-1, (E) GO/AgNP-2, and (F) GO/CuNPs.
The particle size distribution for GO/AgNP-1, GO/AgNP-2, and GO/CuNP composites was determined by analyzing AFM images. A total of 70 individual nanoparticles were measured for each composite. The resulting size distributions, as shown in Figures 4D–F, reflect the particle diameters measured, with the average particle size and standard deviations reported in Supplementary Table S2. For GO/AgNP-1, the average particle size was found to be 31.79 ± 0.70 nm (Figure 4D). Similarly, GO/AgNP-2 exhibited a comparable average particle size of 30.43 ± 1.26 nm, which aligns with the data for GO/AgNP-1, indicating a consistent AgNP size distribution between the two synthesis methods. Therefore, the GO/AgNP-2 data is not reported separately in the AFM results as it shows no significant deviation from GO/AgNP-1. In the case of GO/CuNP, the average particle size was determined to be 22.64 ± 1.33 nm, as shown in Figure 4F. This smaller particle size, compared to AgNPs, suggests a more compact distribution of copper nanoparticles on the GO surface, which might contribute to enhanced surface interaction.
3.3 XRD analysis
The XRD patterns for GO, GO/AgNP-1, GO/AgNP-2, and GO/CuNP composites are shown in Figure 5, with characteristic peaks that provide insight into the structural properties of the synthesized materials. In Figure 5A, the XRD pattern of pristine GO shows two distinct peaks at 2θ values of 11.8° and 42.5°, corresponding to the (001) and (002) planes, respectively. The peak at 11.8° is indicative of the layered structure of GO, representing the interlayer spacing between the oxidized graphene sheets due to the presence of oxygen-containing functional groups. The peak at 42.5° corresponds to the graphitic stacking, which is characteristic of a GO partially ordered structure (Anandalakshmi et al., 2016).
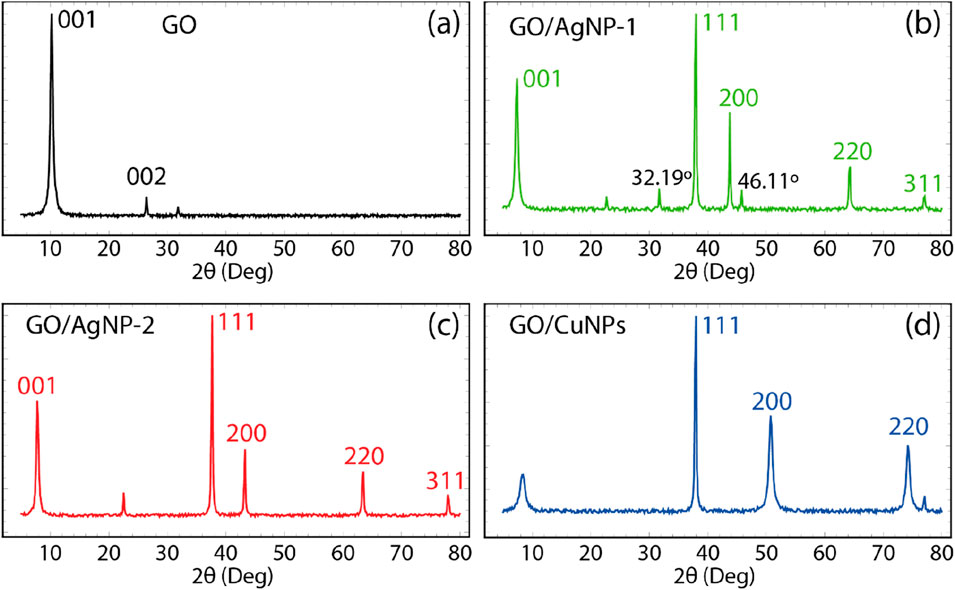
Figure 5. XRD pattern of (A) GO, (B) GO/AgNP-1, (C) GO/AgNP-2, and (D) GO/CuNPs. Spectra data were smoothed using a 7-point moving average.
On the other hand, the XRD pattern of GO/AgNP-1, shown in Figure 5B, displays prominent peaks at 2θ values of 38.1°, 44.3°, 64.5°, and 77.3°, corresponding to the (111), (200), (220), and (311) planes of face-centered cubic (FCC) silver (Anandalakshmi et al., 2016). These peaks confirm the successful incorporation of AgNPs onto the GO surface. Additionally, two minor peaks at 32.19° and 46.11°, not typically associated with silver, are observed. These are likely due to residual bioorganic compounds from the green synthesis using Calendula officinalis extract (Suviith and Philip, 2014; Jeeva et al., 2014). The characteristic peak of GO at 11.8° (001) is still present, indicating that the GO structure remains intact after the nanoparticle functionalization. The XRD pattern for GO/AgNP-2 in Figure 5C is similar to that of GO/AgNP-1, showing strong peaks for silver at 38.1°, 44.3°, 64.5°, and 77.3°. However, the absence of the unassigned peaks (seen in GO/AgNP-1) suggests a cleaner synthesis with fewer bioorganic residues. This cleaner formation results from the different synthesis approaches used for AgNP-2. The peak at 11.8° for GO remains visible, confirming the retention of the GO structure even after AgNP deposition.
Figure 5D presents the XRD pattern for the GO/CuNP composite, where distinct peaks at 2θ values of 43.4°, 50.6°, and 74.1° correspond to the (111), (200), and (220) planes of face-centered cubic copper (Cu), confirming the successful attachment of CuNPs onto the GO surface. Unlike the AgNP composites, no additional unassigned peaks are observed, indicating a relatively pure copper deposition. The characteristic peak for GO at around 11.8° is still visible, confirming that the GO structure is preserved after functionalization with CuNPs.
3.4 UV-vis, FTIR, and Raman characterization
The UV-visible absorption spectra for the different composites are presented in Figure 6 (and Supplementary Table S3), which provides insights into the electronic interactions and optical properties of these materials. Figure 6A shows the UV-vis spectrum of pristine GO. Two characteristic peaks are observed (Tene et al., 2023a): the first at 227.30 nm, corresponding to the π→π* transitions of the C–C bonds in the aromatic rings, and the second at 321.22 nm, attributed to the n→π* transitions typically associated with the C=O bonds in the GO structure. On the other hand, the UV-vis spectra for the GO/AgNP-1 and GO/AgNP-2 composites are shown in Figures 6B,C, respectively. Both spectra exhibit two distinct peaks: one corresponding to the π→π* transitions of GO, and the other attributed to the surface plasmon resonance (SPR) of the silver nanoparticles (Agpeak) (He et al., 2024). For GO/AgNP-1, the π→π transition* appears at 222.12 nm, while the Agpeak is located at 411.10 nm. Similarly, GO/AgNP-2 shows a π→π* transition at 221.22 nm and an Agpeak at 410.05 nm. The absence of the n→π* transition in these spectra is likely due to the overlap with the silver nanoparticle absorption band. We hypothesize that the observed blueshift in the π→π transition* is due to a strong interaction between GO and the silver nanoparticles, likely due to charge transfer phenomena from GO to AgNPs.
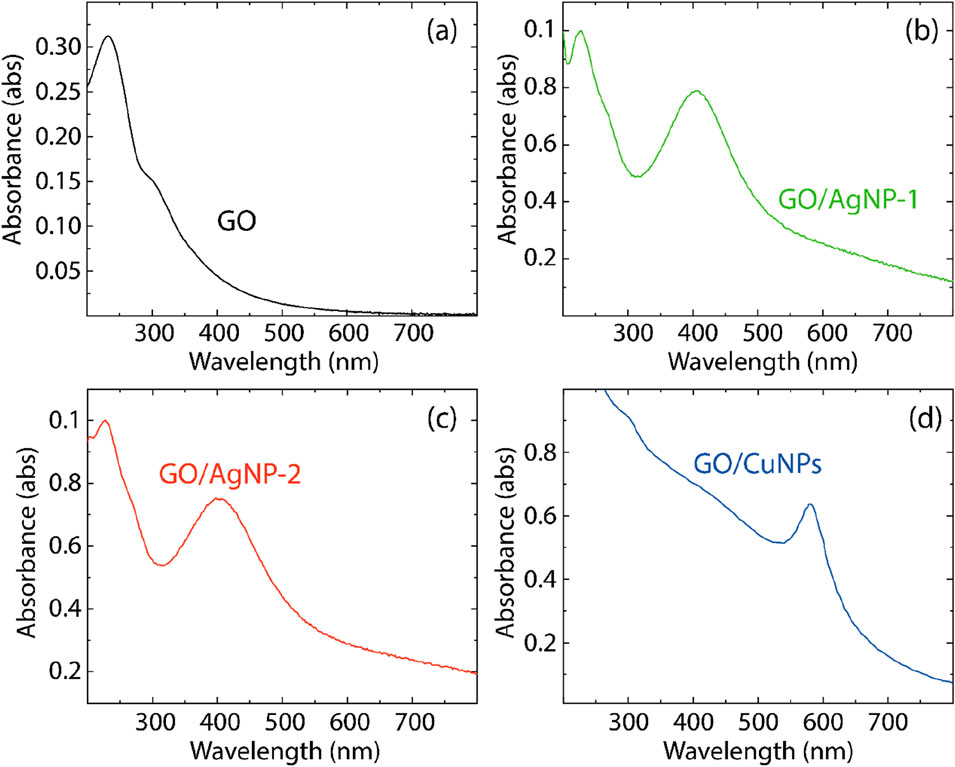
Figure 6. UV-vis spectra of (A) GO, (B) GO/AgNP-1, (C) GO/AgNP-2, and (D) GO/CuNPs. Spectra data were smoothed using a 7-point moving average.
The UV-vis spectrum of the GO/CuNP composite, shown in Figure 6D, reveals a characteristic absorption peak at 573.13 nm, corresponding to the SPR band of copper nanoparticles (Dang et al., 2011). We assume that the absence of a distinct π→π* transition in this spectrum is because the optical properties of GO are largely dominated by the copper nanoparticle absorption, which masks the GO transitions. Also, the GO/CuNP composite shows no observable shoulder from the n→π* transition, further indicating that the electronic interaction between GO and CuNPs may be affecting the electronic structure of pristine GO.
The FTIR spectra for the obtained composites are presented in Figure 7, and the corresponding vibrational band assignments are listed in Supplementary Table S4. In the FTIR spectrum of pristine GO, shown in Figure 7A, several characteristic vibrational bands are observed. Notably, the O–H stretching vibrations, indicating hydroxyl groups, appear in the range of 3,020 cm⁻1–3,800 cm⁻1. The peaks between 2,700 cm⁻1 and 2,950 cm⁻1 are attributed to C–H asymmetric and symmetric stretching, characteristic of hydrocarbon chains. Additionally, the C=O stretching vibrations of carbonyl groups are detected between 1720 cm⁻1 and 1740 cm⁻1. The C=C stretching vibrations from sp2-hybridized carbon atoms appear between 1,570 cm⁻1 and 1,600 cm⁻1, and C–O vibrations are seen around 1,250 cm⁻1, indicating the presence of oxygen functional groups typical of oxidized graphene structures (Tene et al., 2020).
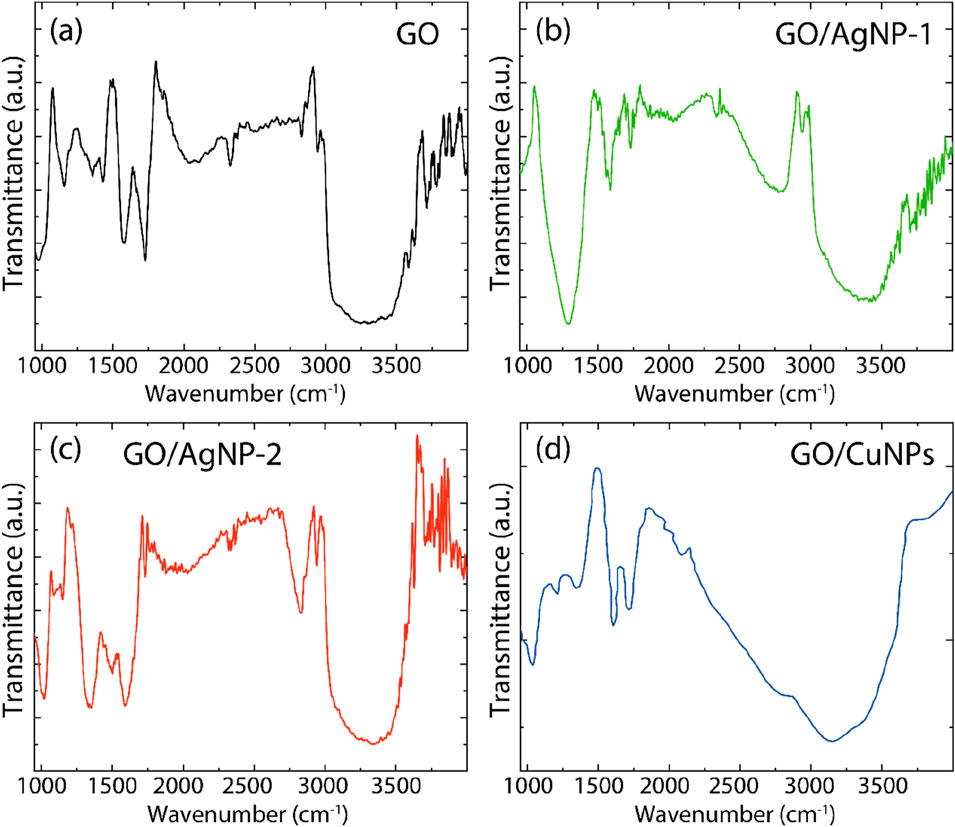
Figure 7. FT-IR spectra of (A) GO, (B) GO/AgNP-1, (C) GO/AgNP-2, and (D) GO/CuNPs. Spectra data were smoothed using a 7-point moving average.
The FTIR spectra for GO/AgNP-1 and GO/AgNP-2, shown in Figures 7B,C, respectively, display additional vibrational bands in the range from 840 cm⁻1–3,700 cm⁻1, indicating the presence of capping agents on the silver nanoparticles. These bands likely arise from bioorganic residues present from the green synthesis process or stabilizing agents used during AgNP formation. Notable changes in the FTIR spectra for these composites occur between 1,000 cm⁻1 and 3,000 cm⁻1. In this region, there are shifts in the positions of some bands, attenuation of others, and increased intensity for certain peaks. These modifications suggest the successful interaction and functionalization of GO with AgNPs. For instance, the C=O stretching band in GO, originally around 1727 cm⁻1, shifts to slightly different positions in GO/AgNP-1 (1721.85 cm⁻1) and GO/AgNP-2 (1731.19 cm⁻1), indicating changes in the chemical environment around the carbonyl groups. Similarly, the C–O vibrations show shifts from 1,250 cm⁻1 in GO to 1,293.30 cm⁻1 in GO/AgNP-1 and 1,341.85 cm⁻1 in GO/AgNP-2, further confirming the interaction between GO and the silver nanoparticles.
In the FTIR spectrum of the GO/CuNP composite (Figure 7D), several significant peaks provide insights into the interaction between GO and copper nanoparticles. A prominent peak at 1,034 cm⁻1 is attributed to C–O–C stretching vibrations, while a peak at 1,404 cm⁻1 corresponds to C–O stretching from carboxyl groups. The C=C stretching vibrations of sp2 hybridized carbon atoms in GO are observed at 1,585 cm⁻1. Additionally, a broad peak in the range of 3,020 cm⁻1–3,600 cm⁻1 indicates O–H stretching vibrations, likely associated with hydroxyl groups or adsorbed water. These peaks suggest the successful incorporation of copper nanoparticles into the GO matrix, with slight shifts and changes in intensity reflecting modifications in the chemical environment of the oxygen-containing functional groups due to the presence of CuNPs.
Raman spectroscopy provides insights into the structural properties of carbon-based materials by analyzing the D and G bands, which correspond to different vibrational modes of carbon atoms. The D-band represents the breathing mode of sp2 carbon atoms associated with structural defects or disorder, while the G-band corresponds to the stretching of the sp2 carbon atoms in graphitic materials (Tene et al., 2021). The ID/IG ratio is commonly used as an indicator of the degree of disorder in graphene-based materials, with higher values suggesting greater defect density. With this in mind, the Raman spectrum of pristine GO, shown in Figure 8A (and Supplementary Table S5), displays prominent D and G peaks at 1,342.20 cm⁻1 and 1,570.51 cm⁻1, respectively. The ID/IG ratio of 1.18 indicates a moderate degree of disorder in the GO structure, which is typical for GO due to the oxygen-containing functional groups introduced during the oxidation process (Tene et al., 2021). The presence of these groups disrupts the sp2 carbon network, increasing the defect density.
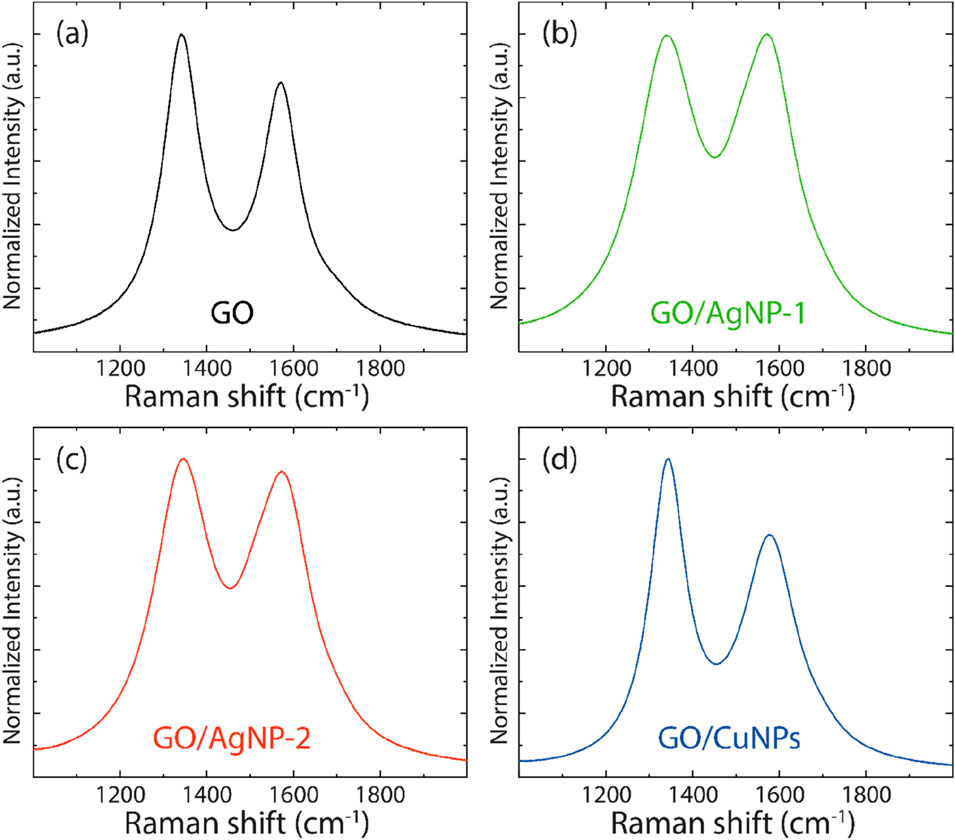
Figure 8. Raman spectra of (A) GO, (B) GO/AgNP-1, (C) GO/AgNP-2, and (D) GO/CuNPs. Spectra data were smoothed using a 7-point moving average.
The Raman spectra for the GO/AgNP-1 and GO/AgNP-2 composites, shown in Figures 8B,C, exhibit similar D and G peaks. For GO/AgNP-1, the D-band is located at 1,340.05 cm⁻1 and the G-band at 1,568.87 cm⁻1, with an ID/IG ratio of 1.01. In the case of GO/AgNP-2, the D-band appears at 1,343.30 cm⁻1 and the G-band at 1,570.49 cm⁻1, with an ID/IG ratio of 1.03. The slight decrease in the ID/IG ratio for both GO/AgNP composites compared to pristine GO suggests a reduction in the number of defects or disorders in the GO structure upon functionalization with silver nanoparticles. This could be attributed to the reduction of GO during the synthesis of AgNPs, as the interaction between GO and the nanoparticles may result in partial restoration of the sp2 carbon network. The proximity of the ID/IG ratios for GO/AgNP-1 and GO/AgNP-2 indicates that both synthesis methods lead to a similar degree of defect reduction.
The Raman spectrum for the GO/CuNP composite is shown in Figure 8D, with the D-band located at 1,341.88 cm⁻1 and the G-band at 1,571.11 cm⁻1. The ID/IG ratio of 1.33 for GO/CuNPs is higher than that of both pristine GO and the GO/AgNP composites, suggesting an increase in disorder within the GO structure upon copper nanoparticle functionalization. This increased disorder may be due to the interaction between copper nanoparticles and the oxygen-containing groups on GO, which could result in further oxidation or defect creation during the CuNP deposition process.
3.5 Antibacterial properties of GO/AgNP composites
Figure 9 presents the antibacterial activity of GO, GO/AgNP-1, and GO/AgNP-2 against E. coli and S. aureus, evaluated through disk diffusion and CFU count assays. The results highlight the dose-dependent antibacterial effects of these composites over 24 h and 48 h, providing key insights into how nanoparticle concentration influences efficacy.
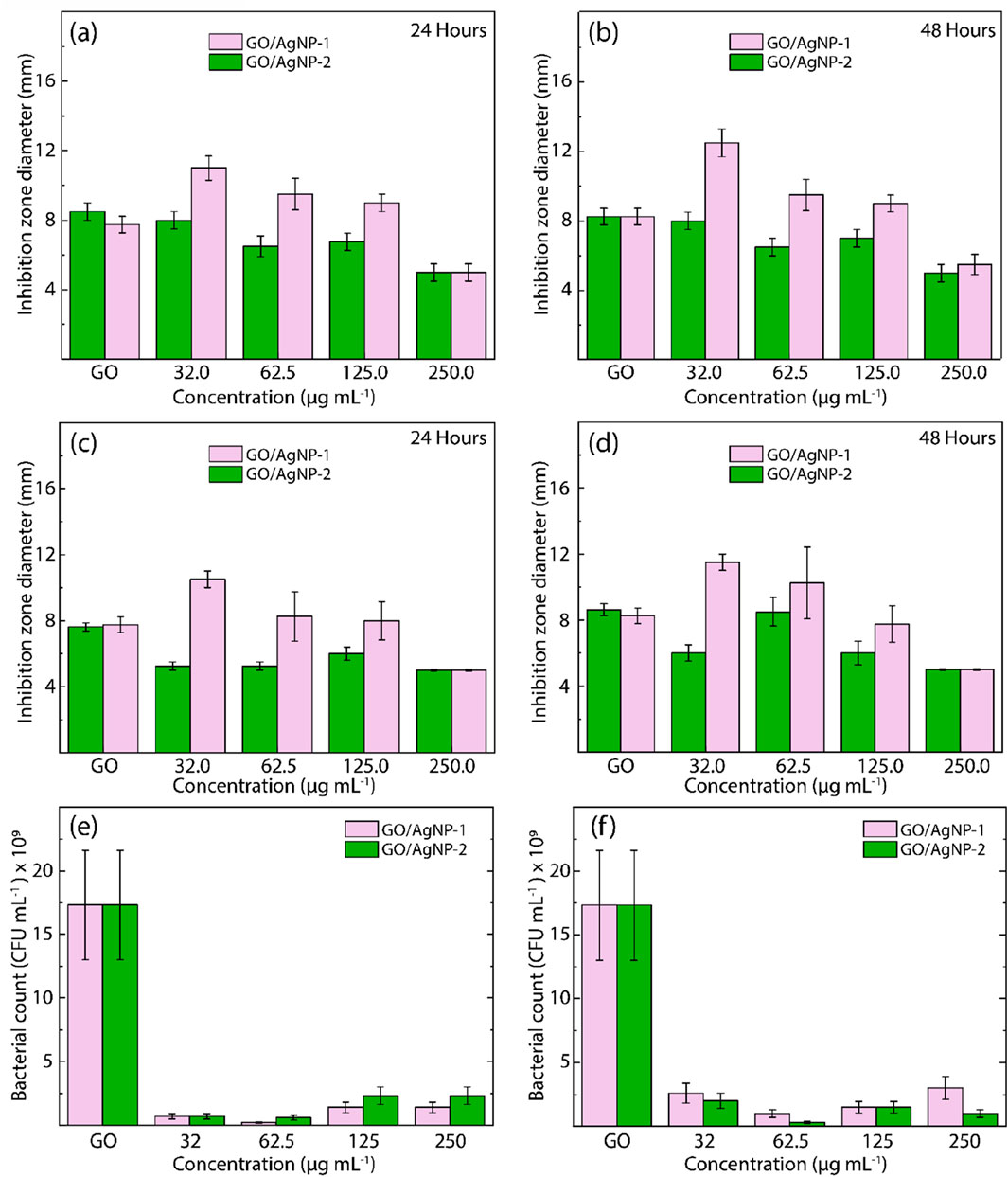
Figure 9. Antibacterial activity of GO, GO/AgNP-1, and GO/AgNP-2 against (A, B) E. Coli and (C, D) S. aureus after exposure to visible light for 24 h and 48 h, respectively, at different nanoparticle concentrations. Cell culturability of (E) E. Coli and (F) S. aureus exposed to GO/AgNP-1 and GO/AgNP-2 under different nanoparticle concentrations for 24 h.
The inhibition zone diameter for both GO/AgNP composites demonstrates a concentration-dependent antibacterial effect. At 24 h (Figure 9A), GO/AgNP-1 exhibited larger inhibition zones compared to GO/AgNP-2 across most concentrations. The maximum inhibition zone for GO/AgNP-1 was observed at 62.5 μg/mL, followed by a slight decline at higher concentrations (125.0 μg/mL and 250.0 μg/mL). This suggests that GO/AgNP-1 is more effective at lower concentrations, with antibacterial activity diminishing slightly as the nanoparticle concentration increases. At 48 h (Figure 9B), this trend remains consistent, with GO/AgNP-1 continuing to outperform GO/AgNP-2, particularly at 62.5 μg/mL, where the largest inhibition zones are observed. Similar results are observed for S. aureus, where GO/AgNP-1 again demonstrates superior antibacterial efficacy compared to GO/AgNP-2. At 24 h (Figure 9C), the largest inhibition zones for GO/AgNP-1 were seen at 62.5 μg/mL and 125.0 μg/mL, suggesting optimal activity within this concentration range. Interestingly, GO/AgNP-1 showed a sustained antibacterial effect after 48 h (Figure 9D), with a noticeable increase in inhibition zones at 62.5 μg/mL, further confirming the effectiveness of the composite at this lower concentration. GO/AgNP-2 consistently exhibited smaller inhibition zones compared to GO/AgNP-1 across all concentrations, reinforcing the superior performance of GO/AgNP-1 in combating both bacterial strains.
The CFU counts for E. coli exposed to GO/AgNP composites for 24 h highlight a significant reduction in bacterial viability across all concentrations (Figure 9E). GO/AgNP-1 consistently demonstrated the most substantial reduction in CFU counts at 62.5 μg/mL and 125.0 μg/mL, with nearly complete bacterial inhibition observed. GO/AgNP-2, while still effective, showed a less pronounced reduction in CFU counts at the same concentrations. These results indicate that GO/AgNP-1 exerts a more potent bactericidal effect at lower nanoparticle concentrations, whereas GO/AgNP-2 may require higher concentrations to achieve similar levels of inhibition. The results for S. aureus follow a similar pattern, with GO/AgNP-1 showing superior antibacterial activity, particularly at 62.5 μg/mL, where bacterial viability is almost eliminated (Figure 9F). GO/AgNP-2, while still effective, shows higher CFU counts compared to GO/AgNP-1, indicating reduced efficacy. This suggests that GO/AgNP-1 is not only more effective at lower concentrations but also maintains consistent antibacterial properties against both gram-negative and gram-positive bacteria.
In general, these results emphasize the concentration-dependent nature of the antibacterial properties of both GO/AgNP composites. In particular, GO/AgNP-1 demonstrates optimal antibacterial activity at 62.5 μg/mL, while GO/AgNP-2 exhibits reduced effectiveness across all concentrations. The diminished antibacterial activity of GO/AgNP-2, especially at higher concentrations, may be due to differences in nanoparticle distribution or size, as suggested by previous characterization data (e.g., AFM and SEM). Additionally, the bactericidal effect appears to plateau beyond 62.5 μg/mL, indicating that higher nanoparticle concentrations do not necessarily enhance antibacterial performance and may even reduce efficacy due to aggregation or saturation effects.
The turbidimetry analysis presented in Tables 1, 2 provides a detailed comparison of the antibacterial efficacy of GO/AgNP-1 and GO/AgNP-2 composites against E. coli and S. aureus. Turbidity indicates bacterial growth, with its absence signaling effective antibacterial activity. Then, at the lowest concentration of 32.0 μg/mL, GO/AgNP-2 demonstrates both turbidity and bacterial growth, indicating that this concentration is insufficient to effectively inhibit E. coli. In contrast, GO/AgNP-1 shows no turbidity or bacterial growth at this concentration, suggesting a significantly stronger antibacterial effect. This demonstrates that GO/AgNP-1 can inhibit bacterial proliferation even at a lower dose, making it a more potent composite. At 62.5 μg/mL, GO/AgNP-2 shows no turbidity but bacterial growth is still detected, indicating incomplete antibacterial activity. GO/AgNP-1, however, continues to show complete inhibition of bacterial growth, with no turbidity or bacterial colonies. This further reinforces the enhanced bactericidal properties of GO/AgNP-1, which consistently outperforms GO/AgNP-2 at similar concentrations.
Now, similar trends are observed for S. aureus. At 32.0 μg/mL, GO/AgNP-2 shows both turbidity and bacterial growth, indicating limited antibacterial action. On the other hand, GO/AgNP-1 shows no turbidity or growth, confirming its ability to inhibit S. aureus effectively at this low concentration. This result highlights the potential of composite as a powerful antibacterial agent, even at reduced doses. At 62.5 μg/mL, GO/AgNP-2 continues to show no turbidity, but bacterial growth is still detected, suggesting partial inhibition. GO/AgNP-1, once again, demonstrates superior performance by exhibiting neither turbidity nor bacterial growth, indicating full bactericidal activity. This trend of superior efficacy at lower concentrations persists for GO/AgNP-1 across both bacterial strains.
3.6 Antibacterial properties of GO/CuNP composite
The antibacterial activity of the GO/CuNP composite against both E. coli and S. aureus is presented in Figure 10, which details the inhibition zone measurements over 24 and 48 h, and the bacterial count for E. coli after 24 h.
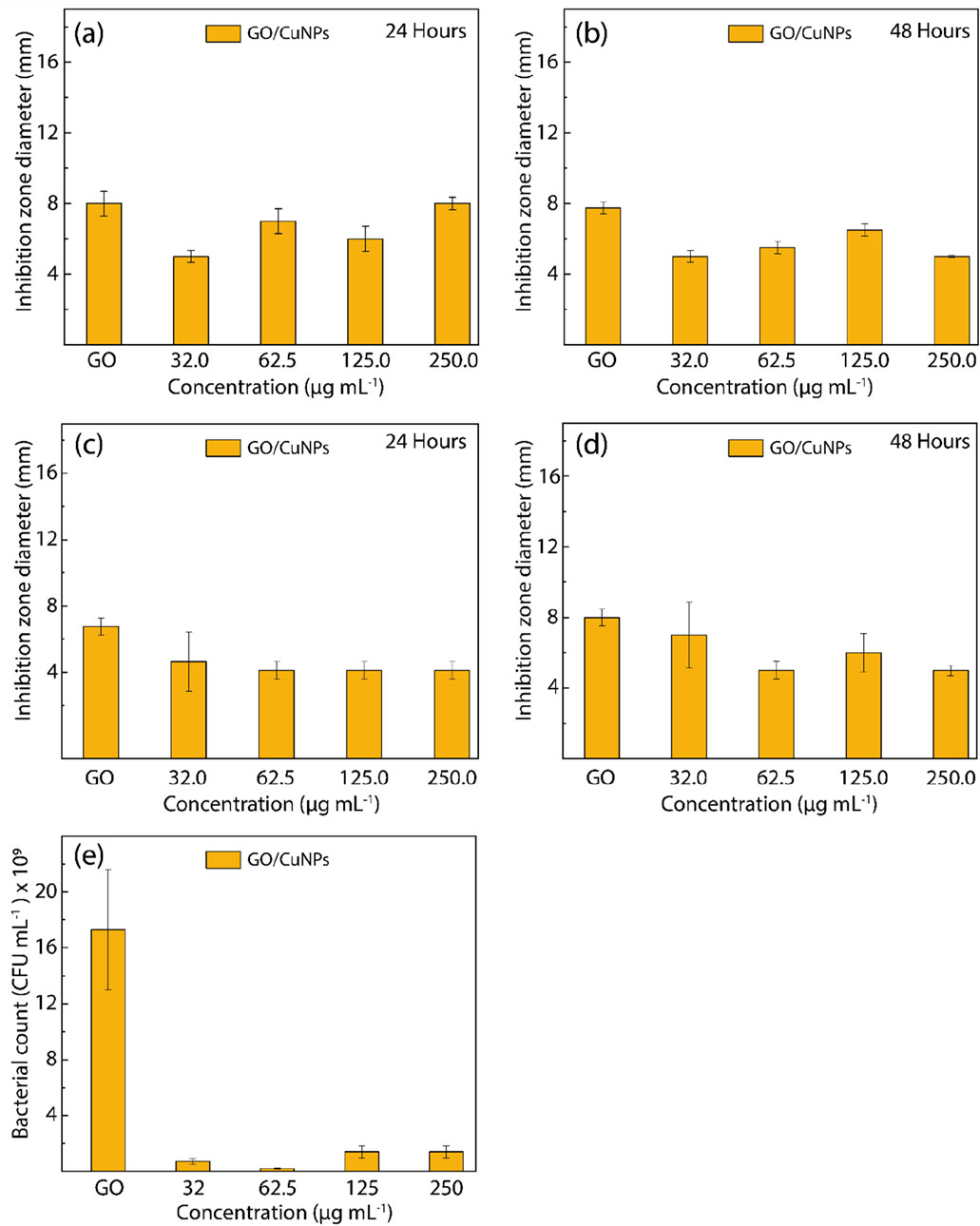
Figure 10. Antibacterial activity of GO and GO/CuNPs against (A, B) E. Coli and (C, D) S. aureus after exposure to visible light for 24 h and 48 h, respectively, at different nanoparticle concentrations. Cell culturability of (E) E. Coli exposed to GO/CuNPs under different nanoparticle concentrations for 24 h.
For E. coli, the disk diffusion test results shown in Figures 10A,B reveal a concentration-dependent antibacterial response. At 24 h, the GO/CuNP composite displays moderate antibacterial activity across all tested concentrations, with the most pronounced inhibition zone observed at 62.5 μg/mL. Interestingly, at higher concentrations (125.0 μg/mL and 250.0 μg/mL), there is a slight reduction in inhibition zone diameter, suggesting that further increases in CuNP concentration do not enhance antibacterial effectiveness. This trend remains consistent after 48 h, where 62.5 μg/mL continues to show the highest antibacterial activity, highlighting the presence of an optimal concentration for the antibacterial action. Similarly, for S. aureus (Figures 10C,D), the GO/CuNP composite demonstrates moderate antibacterial activity across the same concentration range, with 62.5 μg/mL again showing the largest inhibition zone. After 48 h, the inhibition zone diameters remain stable, indicating the sustained antibacterial properties of the composite. As with E. coli, the antibacterial effect appears to plateau at higher concentrations, reinforcing that 62.5 μg/mL is the most effective concentration for inhibiting bacterial growth.
The CFU assay for E. coli, presented in Figure 10E, provides a direct measure of bacterial viability after 24 h of exposure to the GO/CuNP composite. The bacterial count is significantly reduced across all concentrations, with the most substantial reduction observed at 62.5 μg/mL, where bacterial viability is almost completely inhibited. At 32.0 μg/mL, the bacterial count remains relatively high, indicating that this concentration does not fully inhibit bacterial growth. However, as the concentration increases to 62.5 μg/mL, bacterial viability is nearly eliminated, confirming the optimal antibacterial action at this concentration. Interestingly, higher concentrations (125.0 μg/mL and 250.0 μg/mL) show a slight increase in bacterial count compared to 62.5 μg/mL, suggesting that further increases in nanoparticle concentration do not enhance antibacterial performance, possibly due to nanoparticle aggregation at higher doses. While Figure 10E provides CFU count data for E. coli, the corresponding data for S. aureus is not shown. This absence is due to time constraints in completing the necessary analysis rather than a lack of experimental results. However, the absence of this data does not detract from the overall findings, as the disk diffusion tests demonstrate the clear antibacterial effectiveness of the GO/CuNP composite against S. aureus. Further studies could complement these findings by including CFU data for S. aureus to reinforce the broad-spectrum antibacterial properties of the composite.
Table 3 provides the turbidimetry analysis results for the GO/CuNP composite against E. coli after 24 h of exposure. The obtained results indicate whether turbidity or the absence of growth was observed at two different concentrations: 32.0 μg/mL and 62.5 μg/mL. Then, at a concentration of 32.0 μg/mL, the results show no turbidity and no bacterial growth, indicating that this concentration of the GO/CuNP composite is sufficient to inhibit the growth of E. coli. The absence of both turbidity and growth suggests that the composite is highly effective at this relatively low concentration. This aligns with the results seen in disk diffusion tests where moderate concentrations such as 32.0 μg/mL show substantial antibacterial activity. At the higher concentration of 62.5 μg/mL, turbidity is observed, indicating the presence of bacterial growth. However, it is noted no bacterial growth at this concentration, suggesting that while the composite creates some disturbance in the medium (leading to turbidity), it ultimately prevents bacterial proliferation. This could imply that although the higher concentration affects the bacterial environment, it does not allow the bacteria to survive or reproduce effectively.
3.7 Comparison with previous works
Based on the previously discussed antibacterial properties of the synthesized composites, we present a simplified schematic representation (Figure 11) to illustrate the proposed mechanism of antibacterial action. This visual model aims to clarify the step-by-step process by which the GO/AgNP and GO/CuNP composites interact with bacterial cells, leading to their disruption and eventual cell death. The diagram highlights key interactions, such as the release of metal ions, the generation of reactive oxygen species (ROS), and the structural damage to bacterial membranes, all contributing to the observed antibacterial efficacy.
Now, the results presented in Table 4 provide a comparative analysis of the antibacterial activity of GO-based composites functionalized with AgNPs and CuNPs from the present study alongside data from previous studies (Halkai et al., 2018; Akhavan and Ghaderi, 2010; Zhao et al., 2014; Vanlalveni et al., 2021; Yang et al., 2022; He et al., 2011; Mao et al., 2021). Then, our GO/AgNP-1 composite, synthesized via green methods using Calendula officinalis, demonstrated an inhibition zone of 12 mm at 62.5 μg/mL against E. coli and S. aureus. This result is comparable to other studies that reported a 20 mm inhibition zone at 50 μg/mL for a GO/AgNP composite synthesized through green methods (Vanlalveni et al., 2021). This evidence stresses the effectiveness of plant-based green synthesis in generating potent antibacterial agents. Meanwhile, our GO/AgNP-2 composite, synthesized through conventional methods, showed a smaller inhibition zone of 10 mm at the same concentration, highlighting the enhanced efficacy of green synthesis at similar nanoparticle concentrations.
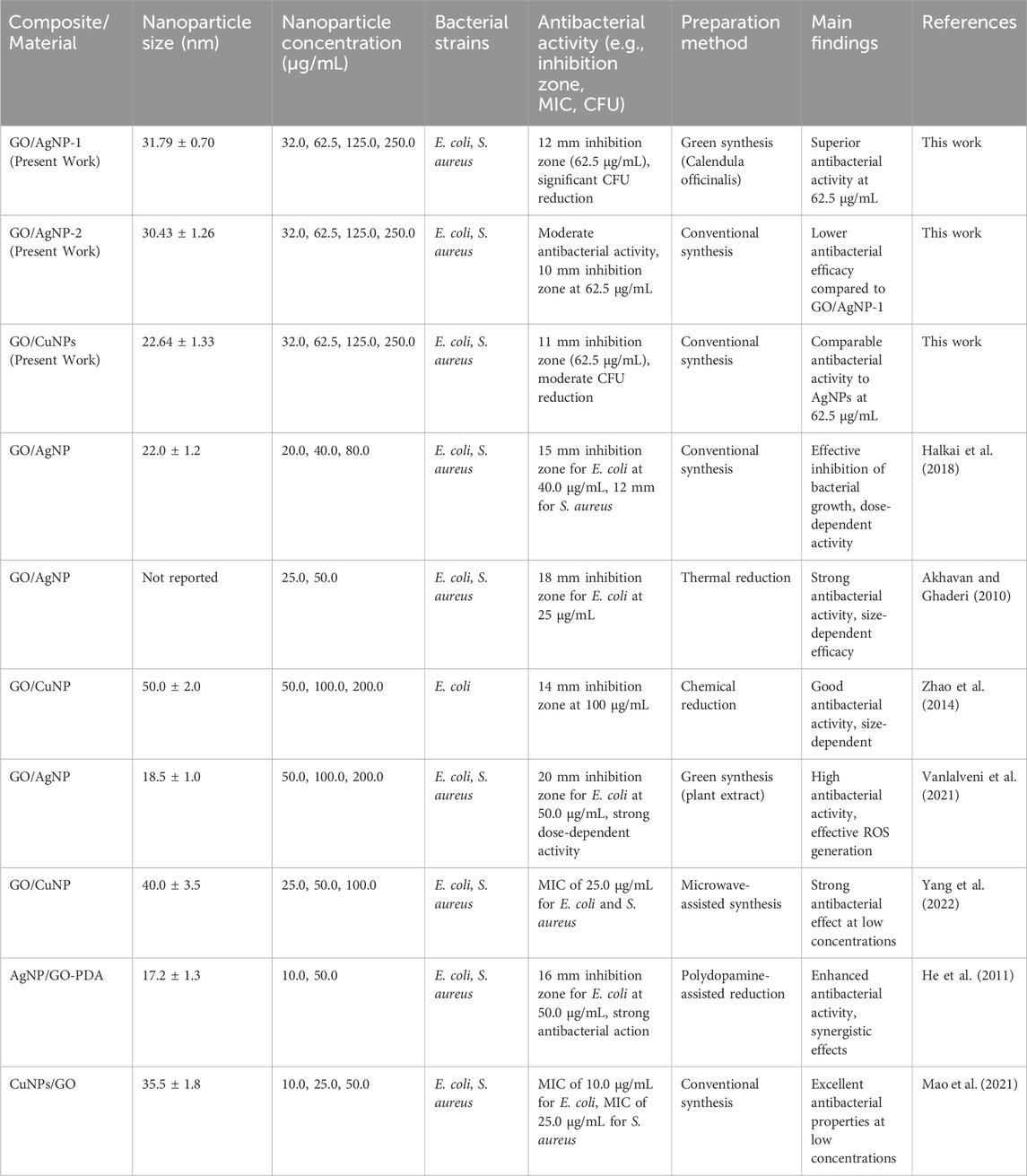
Table 4. Summary of antibacterial activity and preparation methods of GO-based composites functionalized with silver (AgNPs) and copper nanoparticles (CuNPs) from the present work and peer-reviewed studies.
For GO/CuNPs, our composite displayed an 11 mm inhibition zone at 62.5 μg/mL, which is consistent with results from studies showing that CuNP composites can achieve a 14 mm inhibition zone at 100 μg/mL (Yang et al., 2022). This comparable antibacterial efficacy of GO/CuNP composites demonstrates that CuNPs can be a cost-effective alternative to silver-based composites, particularly for applications where the high cost of silver is a limitation (Halkai et al., 2018; Yang et al., 2022). Additionally, other studies have reported that CuNP-based composites can achieve low MIC values of 25 μg/mL, which aligns with our findings (Zhao et al., 2014).
In terms of synthesis methods, our green synthesis approach for AgNPs offers significant advantages over conventional methods. Studies employing thermally reduced GO/AgNPs have reported strong antibacterial effects, with inhibition zones of up to 18 mm, which is comparable to our results (Akhavan and Ghaderi, 2010). Moreover, research utilizing microwave-assisted synthesis for GO/CuNPs demonstrated effective antibacterial properties with low MIC values, reinforcing the versatility of GO-based nanocomposites across different synthesis techniques (Yang et al., 2022). Our green synthesis in the case of GO/AgNP-1 composite, not only competes with these conventional methods but also provides the added benefit of being environmentally friendly, making it an attractive alternative for large-scale applications.
In addition, we highlight the following works by Subhadarshini et al. (Subhadarshini et al., 2021) and Gong et al. (2024) which offer complementary insights to our study and pave the way for future innovative antibacterial materials. The silver nanodot-copper foam system demonstrates robust surface-based bactericidal activity, while our GO/AgNP and GO/CuNP composites extend this functionality to both surface and solution-phase applications, creating a versatile antibacterial platform. The use of green synthesis in both studies, including our use of Calendula officinalis for AgNPs, highlights the importance of eco-friendly approaches. Furthermore, our introduction of GO/CuNP composites adds a cost-effective alternative with comparable antibacterial efficacy, broadening the scope of applications. Together, these works suggest promising avenues for the development of scalable, multifunctional antibacterial materials that are both environmentally sustainable and effective for a wide range of biomedical and environmental uses.
4 Conclusion
This study successfully synthesized GO-based composites functionalized with AgNPs and CuNPs and evaluated their antibacterial properties against E. coli and S. aureus. The structural integrity and nanoparticle attachment were confirmed using SEM, AFM, EDS, XRD, and UV-vis analyses, which demonstrated the successful incorporation of nanoparticles on the GO surface. The crystalline nature of both silver and copper was validated through XRD patterns, while FTIR and Raman results showed changes in the GO structure due to nanoparticle functionalization. The antibacterial studies revealed that GO/AgNP-1 consistently demonstrated superior antibacterial activity compared to GO/AgNP-2, particularly at lower concentrations (32.0 μg/mL and 62.5 μg/mL). This was evident in both disk diffusion and CFU assays, where GO/AgNP-1 exhibited significant bactericidal effects against both E. coli and S. aureus. The GO/CuNP composite also showed effective antibacterial properties, with 62.5 μg/mL being the most optimal concentration, exhibiting strong inhibition of bacterial growth. The turbidimetry analysis further confirmed the antibacterial potency of the composites, showing no bacterial growth at the tested concentrations. However, particle aggregation at higher concentrations (above 62.5 μg/mL) may have slightly reduced efficacy, indicating that moderate nanoparticle concentrations are ideal for maximizing antibacterial activity. Finally, both GO/AgNP and GO/CuNP composites show strong potential as antibacterial agents. The study highlights the importance of nanoparticle concentration and synthesis methods in enhancing the antibacterial properties of these composites, making them promising candidates for applications in antimicrobial treatments.
Data availability statement
The original contributions presented in the study are included in the article/Supplementary Material, further inquiries can be directed to the corresponding authors.
Author contributions
TT: Conceptualization, Data curation, Formal Analysis, Funding acquisition, Investigation, Methodology, Project administration, Resources, Software, Supervision, Validation, Visualization, Writing–original draft, Writing–review and editing. SB: Conceptualization, Data curation, Formal Analysis, Funding acquisition, Investigation, Methodology, Project administration, Resources, Software, Supervision, Validation, Visualization, Writing–original draft, Writing–review and editing. JP: Investigation, Methodology, Writing–original draft, Writing–review and editing. MC-L: Investigation, Methodology, Validation, Writing–original draft, Writing–review and editing. GT-U: Investigation, Methodology, Validation, Writing–original draft, Writing–review and editing. MG: Data curation, Visualization, Writing–original draft, Writing–review and editing. MLP: Formal Analysis, Investigation, Writing–original draft, Writing–review and editing. YCS: Investigation, Methodology, Validation, Visualization, Writing–original draft, Writing–review and editing. AS: Investigation, Methodology, Writing–original draft, Writing–review and editing. MAP: Writing–review and editing, Data curation, Formal Analysis, Investigation, Methodology, Validation, Writing–original draft. LRG: Formal Analysis, Writing–original draft, Writing–review and editing. CVG: Writing–original draft, Writing–review and editing. LSC: Conceptualization, Data curation, Formal Analysis, Funding acquisition, Investigation, Methodology, Project administration, Resources, Software, Supervision, Validation, Visualization, Writing–original draft, Writing–review and editing.
Funding
The author(s) declare that financial support was received for the research, authorship, and/or publication of this article. This work was partially funded by the internal fund project of Instituto Tecnológico de Santo Domingo (INTEC), titled “Biohybrid materials based on nanofibers for the delivery of microorganisms of industrial interest”. This work was funded and supported by Universidad Técnica Particular de Loja under grant No.: POA_VIN-56.
Acknowledgments
JP, TT, MG, and CVG, would like to express their gratitude to Escuela Superior Politécnica de Chimborazo for their warm hospitality throughout the duration of this work. The research was financially supported by Universidad Técnica Particular de Loja (UTPL-Ecuador).
Conflict of interest
The authors declare that the research was conducted in the absence of any commercial or financial relationships that could be construed as a potential conflict of interest.
Publisher’s note
All claims expressed in this article are solely those of the authors and do not necessarily represent those of their affiliated organizations, or those of the publisher, the editors and the reviewers. Any product that may be evaluated in this article, or claim that may be made by its manufacturer, is not guaranteed or endorsed by the publisher.
Supplementary material
The Supplementary Material for this article can be found online at: https://www.frontiersin.org/articles/10.3389/fchem.2024.1426179/full#supplementary-material
References
Akhavan, O., and Ghaderi, E. (2010). Toxicity of graphene and graphene oxide nanowalls against bacteria. ACS Nano 4 (10), 5731–5736. doi:10.1021/nn101390x
Alfei, S., Schito, G. C., Schito, A. M., and Zuccari, G. (2024). Reactive oxygen species (ROS)-Mediated antibacterial oxidative therapies: available methods to generate ROS and a novel option proposal. Int. J. Mol. Sci. 25, 7182. doi:10.3390/ijms25137182
Anandalakshmi, K., Venugobal, J., and Ramasamy, V. (2016). Characterization of silver nanoparticles by green synthesis method using pedalium murex leaf extract and their antibacterial activity. Appl. Nanosci. 6, 399–408. doi:10.1007/s13204-015-0449-z
Badoni, A., and Prakash, J. (2024). Noble metal nanoparticles and graphene oxide-based hybrid nanostructures for antibacterial applications: recent advances, synergistic antibacterial activities, and mechanistic approaches. Micro Nano Eng. 22, 100239. doi:10.1016/j.mne.2024.100239
Barani, M., Mir, A., Roostaee, M., Sargazi, G., and Adeli-Sardou, M. (2024). Green synthesis of copper oxide nanoparticles via moringa peregrina extract incorporated in graphene oxide: evaluation of antibacterial and anticancer efficacy. Bioprocess Biosyst. Eng. 47, 1915–1928. doi:10.1007/s00449-024-03077-2
Cao, M., Wang, S., Hu, J.-H., Lu, B.-H., Wang, Q.-Y., and Zang, S.-Q. (2022). Silver cluster-porphyrin-assembled materials as advanced bioprotective materials for combating superbacteria. Adv. Sci. 9, 2103721. doi:10.1002/advs.202103721
Cruz Salazar, Y., Bellucci, S., Guevara, M., Vacacela Gomez, C., Caicedo, I., Buñay, J., et al. (2023). An eco-friendly bandgap engineering of semiconductor graphene oxide. Solid-State Electron 207, 108705. doi:10.1016/j.sse.2023.108705
Dang, T. M. D., Le, T. T. T., Fribourg-Blanc, E., and Dang, M. C. (2011). Synthesis and optical properties of copper nanoparticles prepared by a chemical reduction method. Adv. Nat. Sci. Nanosci. Nanotechnol. 2, 015009. doi:10.1088/2043-6262/2/1/015009
Faid, A. H., Abdel Rafea, M., Gad, S., Sharaky, M., and Ramadan, M. A. (2024). Antitumor efficiency and photostability of newly green synthesized silver/graphene oxide nanocomposite on different cancer cell lines. Cancer Nanotechnol. 15, 17. doi:10.1186/s12645-024-00255-5
Gong, X., Jadhav, N. D., Lonikar, V. V., Kulkarni, A. N., Zhang, H., Sankapal, B. R., et al. (2024). An overview of green synthesized silver nanoparticles towards bioactive antibacterial, antimicrobial, and antifungal applications. Adv. Colloid Interface Sci. 323, 103053. doi:10.1016/j.cis.2023.103053
Halkai, K. R., Mudda, J. A., Shivanna, V., Rathod, V., and Halkai, R. (2018). Antibacterial efficacy of biosynthesized silver nanoparticles against Enterococcus faecalis biofilm: an in vitro study. Contemp. Clin. Dent. 9 (2), 237–241. doi:10.4103/ccd.ccd_828_17
He, D., Peng, Z., Gong, W., Luo, Y., Zhao, P., and Kong, L. (2024). Mechanism of a green graphene oxide reduction with reusable potassium carbonate. RSC Adv. 14, 11966–11972. doi:10.1039/C4RA14511A
He, L., Liu, Y., Mustapha, A., and Lin, M. (2011). Antifungal activity of zinc oxide nanoparticles against botrytis cinerea and Penicillium expansum. Microbiol. Res. 166, 207–215. doi:10.1016/j.micres.2010.03.003
Ivanova, I. A., Daskalova, D. S., Yordanova, L. P., and Pavlova, E. L. (2024). Copper and copper nanoparticles applications and their role against infections: a minireview. Processes 12, 352. doi:10.3390/pr12020352
Izadi, A., Paknia, F., Roostaee, M., Mousavi, S. A. A., and Barani, M. (2024). Advancements in nanoparticle-based therapies for multidrug-resistant candidiasis infections: a comprehensive review. Nanotechnology 35, 332001. doi:10.1088/1361-6528/ad4bed
Jaramillo-Fierro, X., and Cuenca, G. (2024). Theoretical and experimental analysis of hydroxyl and epoxy group effects on graphene oxide properties. Nanomaterials 14, 714. doi:10.3390/nano14080714
Jeeva, K., Thiyagarajan, M., Elangovan, V., Geetha, N., and Venkatachalam, P. (2014). Caesalpinia coriaria leaf extracts mediated biosynthesis of metallic silver nanoparticles and their antibacterial activity against clinically isolated pathogens. Ind. Crops Prod. 52, 714–720. doi:10.1016/j.indcrop.2013.11.037
Jin, H., Cai, M., and Deng, F. (2023). Antioxidation effect of graphene oxide on silver nanoparticles and its use in antibacterial applications. Polymers 15, 3045. doi:10.3390/polym15143045
Mao, M., Zhang, W., Huang, Z., Huang, J., Wang, J., Li, W., et al. (2021). Graphene oxide-copper nanocomposites suppress cariogenic Streptococcus mutans biofilm formation. Int. J. Nanomed. 16, 7727–7739. doi:10.2147/IJN.S303521
Mithuna, R., Tharanyalakshmi, R., Jain, I., Singhal, S., Sikarwar, D., Das, S., et al. (2024). Emergence of antibiotic resistance due to the excessive use of antibiotics in medicines and feed additives: a global scenario with emphasis on the Indian perspective. Emerg. Contam. 10, 100389. doi:10.1016/j.emcon.2024.100389
Ohiduzzaman, M., Khan, M. N. I., Khan, K. A., and Paul, B. (2024). Biosynthesis of silver nanoparticles by banana pulp extract: characterizations, antibacterial activity, and bioelectricity generation. 10, e25520. doi:10.1016/j.heliyon.2024.e25520
Raghavan, S., and Kim, K.-S. (2024). Host immunomodulation strategies to combat pandemic-associated antimicrobial-resistant secondary bacterial infections. Int. J. Antimicrob. Agents 64 (4), 107308. doi:10.1016/j.ijantimicag.2024.107308
Rojas-Andrade, M. D., Chata, G., Rouholiman, D., Liu, J., Saltikov, C., and Chen, S. (2017). Antibacterial mechanisms of graphene-based composite nanomaterials. Nanoscale 9, 994–1006. doi:10.1039/c6nr08733g
Said, A., Abu-Elghait, M., Atta, H. M., and Salem, S. S. (2024). Antibacterial activity of green synthesized silver nanoparticles using lawsonia inermis against common pathogens from urinary tract infection. Appl. Biochem. Biotechnol. 196, 85–98. doi:10.1007/s12010-023-04482-1
Salas-Orozco, M. F., Lorenzo-Leal, A. C., de Alba Montero, I., Patiño Marín, N., Casillas Santana, M. A., and Bach, H. (2024). Mechanism of escape from the antibacterial activity of metal-based nanoparticles in clinically relevant bacteria: a systematic review. Nanomedicine 55, 102715. doi:10.1016/j.nano.2023.102715
Subhadarshini, S., Singh, R., Mandal, A., Roy, S., Mandal, S., Mallik, S., et al. (2021). Silver nanodot decorated dendritic copper foam as a hydrophobic and mechano-chemo bactericidal surface. Langmuir 37 (31), 9356–9370. doi:10.1021/acs.langmuir.1c00698
Suviith, V. S., and Philip, D. (2014). Catalytic degradation of methylene blue using biosynthesized gold and silver nanoparticles. Spectrochim. Acta Part A Mol. Biomol. Spectrosc. 118, 526–532. doi:10.1016/j.saa.2013.09.016
Tene, T., Arias, F. A., Guevara, M., Nuñez, A., Villamagua, L., Tapia, C., et al. (2022). Removal of mercury(II) from aqueous solution by partially reduced graphene oxide. Sci. Rep. 12, 6326. doi:10.1038/s41598-022-10259-z
Tene, T., Bellucci, S., Pachacama, J., Cuenca-Lozano, M. F., Tubon-Usca, G., Guevara, M., et al. (2024). A facile and green approach for the preparation of silver nanoparticles on graphene oxide with favorable antibacterial activity. Nanomaterials 14, 1455. doi:10.3390/nano14171455
Tene, T., Guevara, M., Benalcázar Palacios, F., Morocho Barrionuevo, T. P., Vacacela Gomez, C., and Bellucci, S. (2023a). Optical properties of graphene oxide. Front. Chem. 11, 1214072. doi:10.3389/fchem.2023.1214072
Tene, T., Guevara, M., Valarezo, A., Salguero, O., Arias Arias, F., Arias, M., et al. (2021). Drying-time study in graphene oxide. Nanomaterials 11, 1035. doi:10.3390/nano11041035
Tene, T., Jiménez-Gaona, Y., Campoverde-Santos, D. K., Cevallos, Y., La Pietra, M., Vacacela Gomez, C., et al. (2023b). Tunable optical and semiconducting properties of eco-friendly-prepared reduced graphene oxide. Front. Chem. 11, 1267199. doi:10.3389/fchem.2023.1267199
Tene, T., Tubon Usca, G., Guevara, M., Molina, R., Veltri, F., Arias, M., et al. (2020). Toward large-scale production of oxidized graphene. Nanomaterials 10 (2), 279. doi:10.3390/nano10020279
Turkevich, J., Stevenson, P. C., and Hillier, J. A. (1951). A study of the nucleation and growth processes in the synthesis of colloidal gold. Discuss. Faraday Soc. 11, 55–75. doi:10.1039/df9511100055
Vacacela Gomez, C., Robalino, E., Haro, D., Tene, T., Escudero, P., Haro, A., et al. (2016). Structural and electronic properties of graphene oxide for different degree of oxidation. Mat. Today Proc. 3, 796–802. doi:10.1016/j.matpr.2016.02.011
Vanlalveni, C., Lallianrawna, S., Biswas, A., Selvaraj, M., Changmai, B., and Rokhum, S. L. (2021). Green synthesis of silver nanoparticles using plant extracts and their antimicrobial activities: a review of recent literature. RSC Adv. 11, 2804–2837. doi:10.1039/d0ra09941d
Vodnik, V., Stamenović, U., and Vukoje, I. (2024). Nanocomposites of metal nanoparticles and polymer as platform of alternative approach in combating antimicrobial resistance. Nanotechnol. Based Strategies Combating Antimicrob. Resist., 489–510. doi:10.1007/978-981-97-2023-1_18
Yadav, K. K., Cabral-Pinto, M. M. S., Gacem, A., Fallatah, A. M., Ravindran, B., Rezania, S., et al. (2024). Recent advances in the application of nanoparticle-based strategies for water remediation as a novel clean technology—a comprehensive review. Mat. Today Chem. 40, 102226. doi:10.1016/j.mtchem.2024.102226
Yang, S., Zhou, Y., Zhao, Y., Wang, D., and Luan, Y. (2022). Microwave synthesis of graphene oxide decorated with silver nanoparticles for slow-release antibacterial hydrogel. Mat. Today Commun. 31, 103663. doi:10.1016/j.mtcomm.2022.103663
Zhang, J., Xu, J., Ma, H., Bai, H., Liu, L., Shu, C., et al. (2024). “Designing an amino-fullerene derivative C70-(EDA)8 to fight super bacteria,” in Supporting information. Beijing: Chinese Academy of Sciences. doi:10.1021/acsami.9b02201
Keywords: graphene oxide, silver nanoparticles, cooper nanoparticles, calendula officinalis, seed extract, E. coli, S. aureus, antibacterial activity
Citation: Tene T, Bellucci S, Pachacama J, Cuenca-Lozano MF, Tubon-Usca G, Guevara M, La Pietra M, Cruz Salazar Y, Scarcello A, Arias Polanco M, Gahramanli LR, Vacacela Gomez C and Caputi LS (2024) Synthesis of metal nanoparticles on graphene oxide and antibacterial properties. Front. Chem. 12:1426179. doi: 10.3389/fchem.2024.1426179
Received: 30 April 2024; Accepted: 01 October 2024;
Published: 14 October 2024.
Edited by:
Renata Katsuko Takayama Kobayashi, State University of Londrina, BrazilReviewed by:
Aziz-Ur-Rahim Bacha, Harbin Institute of Technology, ChinaNarayan Das, Indian Institute of Technology Kharagpur, India
Copyright © 2024 Tene, Bellucci, Pachacama, Cuenca-Lozano, Tubon-Usca, Guevara, La Pietra, Cruz Salazar, Scarcello, Arias Polanco, Gahramanli, Vacacela Gomez and Caputi. This is an open-access article distributed under the terms of the Creative Commons Attribution License (CC BY). The use, distribution or reproduction in other forums is permitted, provided the original author(s) and the copyright owner(s) are credited and that the original publication in this journal is cited, in accordance with accepted academic practice. No use, distribution or reproduction is permitted which does not comply with these terms.
*Correspondence: Talia Tene, dGJ0ZW5lQHV0cGwuZWR1LmVj; Stefano Bellucci, YmVsbHVjY2lAbG5mLmluZm4uaXQ=