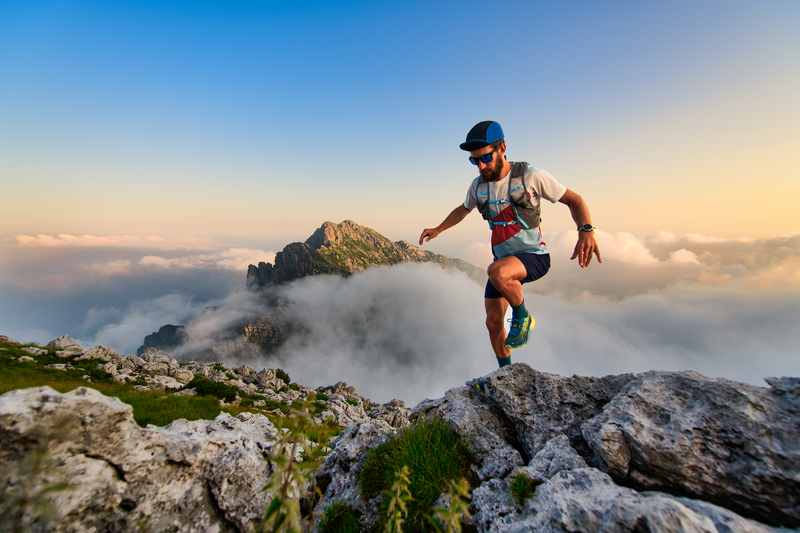
95% of researchers rate our articles as excellent or good
Learn more about the work of our research integrity team to safeguard the quality of each article we publish.
Find out more
ORIGINAL RESEARCH article
Front. Chem. , 26 July 2024
Sec. Nanoscience
Volume 12 - 2024 | https://doi.org/10.3389/fchem.2024.1425693
This article is part of the Research Topic Advanced Nanomaterials for Chemiresistive Gas Sensors View all 3 articles
Heterostructure engineering is crucial for enhancing gas sensing performance. However, achieving rapid response for room-temperature NO2 sensing through rational heterostructure design remains a challenge. In this study, a Bi2Se3/SnSe2 2D/2D heterostructure was synthesized by hydrothermal method for the rapid detection of NO2 at room temperature. By combining Bi2Se3 nanosheets with SnSe2 nanosheets, the Bi2Se3/SnSe2 sensor demonstrated and the lowest detection limit for NO2 a short response time (15 s) to 10 ppm NO2 at room temperature, reaches 25 ppb. Furthermore the sensor demonstrates significantly larger response to NO2 than to other interfering gases, including 10 ppm NO2, H2S, NH3, CH4, CO, and SO2,demonstrating its outstanding selectivity. And we discuss the mechanism of related performance enhancement.
Flexible sensors for pressure, temperature, humidity, strain, and heat flux have garnered significant attention in the fields of electronic skin, environmental monitoring, and body temperature monitoring (Ansari et al., 2023). Gas sensing has become a critical aspect in atmospheric environment monitoring, industrial production, and medical healthcare applications (Yang et al., 2023).NO2 stands as one of the most prevalent air pollutants. Studies indicate that even brief exposure to concentrations as low as 3 ppm can cause eye and lung irritation in human, while also compromising respiratory infection resistance, potentially resulting in fatality in severe cases (Spirtas et al., 1986; Qureshi et al., 2003; Rathi and Pal 2020).Therefore, the development of highly responsive and rapid gas sensing technologies for the detection of low-concentration NO2 at routine temperatures assumes paramount importance in practical applications.
Two-dimensional metal chalcogenides (TMDs) have attracted considerable interest in gas sensing owing to their superior electrical properties, large surface area, and abundant adsorption sites (Zheng et al., 2016; Sardana et al., 2022; Yang et al., 2022; Wang et al., 2023; Yang et al., 2024).Huang et al. modified two-dimensional material MoS2 with zinc oxide nanoparticles to improve the response of MoS2 to NO2 (Han et al., 2018).While tin (Sn) is not classified as a transition metal, its selenide compound can adopt a 2D-layered hexagonal structure similar to that of TMDs (D'Olimpio et al., 2022; Harish and Sathyakam, 2022). Moreover heterostructures offer significant potential for a broad applications, including high-speed electronics and optoelectronic devices. By integrating SnS2 nanosheets with the Ti3C2Tx skeleton platform, Han et al. achieved a good response to 50ppm triethylamine at room temperature (Han et al., 2023). So the fabrication of heterostructures has been widely utilized to further enhance the sensing performance of gas sensors based on SnSe2. For instance, Li et al. fabricated a chemiresistive sensor based on a SnSe2/SnO2 heterojunction using the thermal oxidation method for the detection of NO2 gas (Li et al., 2020). While significant advancements have been achieved in enhancing the sensing capabilities of SnSe2 for NO2 detection, there is still a need for further improvement, particularly in terms of reducing the response time, as well as improving selectivity. An important limitation of two-dimensional materials for room temperature gas sensing is the long response recovery time due to the slow desorption process. It is well understood that in comparison to 0D/2D and 1D/2D heterostructures, 2D/2D heterostructures offer closer interfacial contacts, facilitating enhanced charge transfer and thereby improving sensing properties. It is anticipated that the development of these 2D/2D heterostructures on SnSe2 could efficiently accelerate the response time.
Enhancing selectivity is a critical factor for gas sensing equipment. Current strategies employed to improve the selectivity of gas sensing devices encompass precious metal modification, heterostructural construction, ultraviolet irradiation, and external auxiliary heatin (Majhi et al., 2015; Zhang et al., 2016; Joshi et al., 2018) Bi2Se3, a representative 2D semiconductor, has gained significant interest across various fields like optoelectronic circuit, photocatalysis, and batteries due to its favorable bandgap, exceptional stability, and ease of synthesis (Huang et al., 2020; Li et al., 2022). Moreover, the lower work function of Bi2Se3 compared to SnSe2 promotes facilitates electron migration from Bi2Se3 to SnSe2, resulting in a decrease in the work function of SnSe2. This reduced work function of SnSe2 facilitates electron transfer to NO2 molecules during sensing (Li et al., 2024). Additionally, the remarkable catalytic properties of Bi2Se3 contribute to lowering the activation energy needed for gas sensing, ultimately enhancing sensing performance, particularly in reducing response time and increasing selectivity. Consequently, the engineering of 2D/2D heterostructures by combining Bi2Se3 nanosheets and SnSe2 holds promise for developing sensors with improved sensitivity and rapid response at room temperatures.
In this study, 2D/2D heterostructures of SnSe2/Bi2Se3 were synthesized using a combination of colloidal method and solvothermal method with a laminated stack structure. The optimized SnSe2/Bi2Se3 sensor demonstrated a notable reduction in response time for 10 ppm NO2, dropping from 73 to 15 s, and a decrease in recovery time from 300 to 110 s. The optimized sensor also exhibits a high response to NO2, with a high response rate of 130% to 10ppm of NO2. These improved sensing capabilities can be ascribed to enhanced transfer of charges and the larger number of adsorption sites, which are both enabled by the SnSe2/Bi2Se3 2D/2D heterostructures. Additionally, the fatigue tests of the sensor after 100, 500, and 1,000 cycles of bending and relaxation showed no significant decrease in response values, demonstrating its excellent mechanical performance. These findings present a novel and effective strategy for developing a practical NO2 gas sensor based on SnSe2.
Firstly, 0.5 mmol SnCl4·5H2O, 1.5 mmol SeO2, and 0.5 mmol 1, 10 -phenanthroline were dissolved in a three-neck flask. The mixture was stirred for 10 min under a highly pure N2 atmosphere at room temperature. Following a 10-min N2 degassing, the solution underwent heating to 110 °C while being stirred magnetically for an additional 10 min. Then, further increased to 260 °C and maintained for another 30 min with continuous stirring. Cooling down to room temperature, the resulting products were collected and centrifuged under three rounds of washing: first with cyclohexane and then with ethanol. Ultimately, the products were kept at 70°C for 10 h.
The preparation of Bi2Se3/SnSe2 heterostructures involved a simple solvothermal method. Its synthesis process is shown in Figure 1A. Precisely, 0.2 mmol of the pre-synthesized SnSe2 was gently dispersed in 20 mL ethanol and stirred for 0.5 h. Subsequently, a specific quantity of Bi(NO3)3·5H2O was added to the suspension and vigorously stirred for another 0.5 h. The resulting mixture was then moved to a 25 mL Teflon-lined autoclave and the solvothermal reaction was performed at 180 °C for 12 h. Finally, the resulting product was collected and subjected to three washes with ethanol and water.
Figure 1. (A) Diagram showing the synthetic process of Bi2Se3/SnSe2 heterostructure; SEM, TEM, HRTEM images, and EDX elemental mapping of (B–D) SnSe2 and (E–G) Bi2Se3/SnSe2 heterostructure.
For simplicity, the Bi2Se3/SnSe2 heterostructures were labeled as BS-1, BS-2, and BS-5, reflecting the amounts of Bi salt added (0.020, 0.025, and 0.040 mmol) respectively. In contrast, the synthesis of pure Bi2Se3 involved the addition of 1 mmol Bi2O3, 3 mmol SeO2, and 0.4 g NaOH into 40 mL of ethylene glycol. After being vigorously stirred for 1 h, the resulting suspension was placed in a Teflon-lined autoclave and the reaction was performed at 180°C for 7 h. Subsequently, the resultant products were collected, subjected to multiple washes with ethanol and deionized water, and finally dried at 60°C for 10 h.
The morphologies of the samples were inspected using both FESEM (SIRION 200) and TEM (JEOL-1400). Elemental composition was characterized by EDX and elemental mapping, while crystal structure was analyzed by XRD measurements (Bruker D8 Advance). The chemical states were determined through XPS analysis using an ESCALAB 250 spectrometer.
The gas sensors were produced through applying a solution of sensing materials through drop-casting (10 mg/mL in ethanol) onto Ag-Pd interdigital electrodes on an alumina substrate measuring 6.6 × 6.0 mm. To evaluate the gas sensing performance, a homemade sensor-testing system was employed, and a simplified experimental setup is depicted in Supplementary Figure S1. The real-time changes in conductivity of the sensors was collected via the electrochemical workstation (CHI 630E). In the sensing experiments, a precise volume of test gas was injected into the 4 L test chamber via a syringe. The relative humidity levels were regulated using a CK-80G commercial humidity chamber by Kingjo. All tests were performed in room air at 25°C with a relative humidity of 40%–50%. The sensing response (S) was determined using the equation of S = (Rg-Ra)/Ra, where Rg and Ra represented the sensor resistance in the target gas and in air, respectively. The response and recovery times represent the duration it takes for the sensor to reach 90% of the resistance change after a target gas was injected and released, respectively.
The flexible chemical sensor, incorporating Bi2Se3/SnSe2 heterostructures, was intricately applied to a polyethylene terephthalate substrate with interdigital gold patterns. The fabrication procedure for the device is meticulous and mirrors a method similar to the one previously documented. (Wang et al., 2016; Wang et al., 2017).
Characterized by SEM, TEM, and HRTEM. Figures 1B, E display the SEM images of SnSe2 and BS-2 samples, respectively, and their insets are the corresponding TEM images. The SnSe2 exhibits nanoplates morphology with the uniform dimension of 500–800 nm. After the solvothermal process, the SnSe2 still retain its original shape, and the hexagonal Bi2Se3 nanoplates grow on the surface of SnSe2, forming a heterostructure with intimate contact. The HRTEM images in Figures 1C, F clearly demonstrate the presence of a heterojunction interface. The lattice spacings of 0.310 and 0.225 nm belong to the (002) and (012) planes of SnSe2, respectively, while the 0.360 nm spacing corresponds to the (101) plane of Bi2Se3. In addition, the EDX mapping results demonstrate the uniform distribution of Sn, Se, and Bi elements (Figures 1D, G), which further indicates the formation of interconnected structures between SnSe2 and Bi2Se3.
A control sample of nanoplate-like Bi2Se3 was synthesized employing a solvothermal method for comparative analysis (Supplementary Figure S2). The crystalline characteristics of SnSe2, Bi2Se3, and the Bi2Se3/SnSe2 heterostructures were investigated via XRD analysis, with the resulting patterns depicted in Figure 2A. The diffraction peaks observed in the pristine samples were unambiguously assigned to hexagonal SnSe2 (JCPDS 89-3197) and hexagonal Bi2Se3 (JCPDS 33-0214). Specifically, the well-aligned distinct peaks at 14.41°, 30.73°, 40.08°, 47.69°, 52.57°, 57.81°, and 64.00° corresponded favorably to the (001), (011), (012), (110), (103), (201), and (202) crystallographic planes of SnSe2, respectively. Similarly, the peaks observed at 18.56°, 25.00°, 29.36°, 43.69°, 47.84°, and 53.54° were unequivocally attributed to the (006), (012), (015), (110), (116), and (205) crystallographic planes of Bi2Se3. With respect to the Bi2Se3/SnSe2 heterostructures, an increase in the amount of added Bi salt led to an augmentation in the diffraction peaks of Bi2Se3 and a simultaneous decrease in the peak assigned to SnSe2, without any noticeable impurities, thus indicating the high purity of these synthesized products.
Figure 2. (A) XRD patterns of SnSe2, Bi2Se3, and SnSe2/Bi2Se3 heterostructure; XPS spectra of SnSe2, Bi2Se3 and BS-2 samples: (B) Survey, (C) Sn 3d, (D) Se 3d, and (E) Bi 4f and Se 3p.
XPS measurement was performed to analyze the chemical compositions and chemical states of the samples. The survey spectra of the BS-2 sample demonstrates the co-existence of Sn, Se, and Bi elements (Figure 2B), which is in line with the findings from the EDX mapping. In the detailed XPS spectrum of Sn3d, peaks at 485.9 and 494.3 eV are corresponding to Sn 3d5/2 and Sn 3d3/2 (Figure 2C), respectively, indicating the presence of Sn4+ in SnSe2. Two peaks around 52.8 and 53.6 eV belong to the 3d5/2 and 3d3/2 of Se2- species (Figure 2D), respectively. In the Bi 4f and Se 3p spectra presented in Figure 2E, the distinctive peaks at 157.8 and 162.8 eV are indicative of Bi 4f7/2 and Bi 4f5/2, respectively, indicating the chemical state of Bi3+. The peaks that located at 159.7 and 165.4 eV could be separately assigned to the Se 3p3/2 and Se 3p1/2 orbitals of Se2- (Xu et al., 2019). Notably, in comparison with pristine SnSe2, the binding energy of Sn 3d in BS-2 slightly shifts to higher energy, while the binding energy of Bi 4f in BS-2 are slightly lower than that of pure Bi2Se3. Such migration shifts of the binding energies may be ascribed to the change in electron density on the surfaces of the samples, and the above results confirm that the electrons in the heterostructure transfer from SnSe2 to Bi2Se3. The analyses conducted affirm the successful creation of Bi2Se3/SnSe2 heterostructures.
The NO2 sensing capabilities of Bi2Se3/SnSe2 heterostructures were examined through testing the change in resistance upon exposed to target gases. The initial investigation focused on assessing the influence of the Bi2Se3 to SnSe2 ratio on the sensing performance of the heterostructures. As the ratio of Bi2Se3 increased, the sensing response increases first then decreased (Figure 3). The BS-2 performs the most significant sensing response to NO2. By contrast, the sensors based on pure SnSe2 and Bi2Se3 exhibit inadequate response and sluggish recovery. The significantly improved sensing performance may be credited to the formed heterointerface between SnSe2 and Bi2Se3, which facilitates charge transfer and provides abundant active sites. According to the detailed sensing characteristics outlined in Supplementary Table S1, the Bi2Se3/SnSe2-2 sensor has been chosen for further research due to its impressive performance, showing the largest response rate of 130% and the shortest response/recovery times of 15/110 s to 10 ppm NO2.
Figure 3. Response curves of the sensors based on SnSe2, Bi2Se3 and Bi2Se3/SnSe2 heterostructures to 10 ppm NO2 at room-temperature.
The dynamic response curve of BS-2 sensor toward different NO2 concentration was further measured. As illustrated in Figure 4A, the sensor shows excellent response and recover ability, and the lowest detection limit for NO2 reaches 25 ppb. Besides, the correlation equation relating response value to gas concentration at ppb (Figure 4B) and ppm level (Figure 4C) could be separately calculated as a good linear relationship, which suggests its promising potential for sensor calibration purpose. Additionally, the wide detection range of the BS-2 sensor enables it to have a broad range of applications.
Figure 4. (A) Dynamic response curves of BS-2 sensor to NO2 (20 ppm–25 ppb); (B, C) The correlation between gas concentration and sensor response value.
The selectivity of the BS-2 sensor was studied upon exposure to different analytes, including 10 ppm NO2, H2S, NH3, CH4, CO, and SO2. As shown in Figure 5A, the sensor demonstrates significantly larger response to NO2 than to other interfering gases, demonstrating its outstanding selectivity. To assess long-term stability, the responses of the BS-2 sensor to 10 ppm NO2 were recorded at a 5-day interval. The sensor exhibits a slight response recession (Figure 5B) and nearly identical sensing behaviors toward target gases within 50 days (Supplementary Figure S3), thereby affirming its significant stability and reliability. In addition, the BS-2 sensor presents notable stability when working in moderate humidity levels (25%–55%), making it suitable for practical applications (Figure 5C).
Figure 5. (A) Selective responses of BS-2 sensor to 10 ppm NO2, H2S, NH3, CH4, CO and SO2; (B) Long-term stability and (C) effects of humidity on the sensing responses of BS-2 sensor to 10 ppm NO2.
For a comprehensive assessment of sensing ability, Table 1 sums up a comparison between the optimal sensor in this study and other reported sensors based on 2D materials. The Bi2Se3/SnSe2 sensor shows superior NO2 sensing performance, featuring minimal power usage and heightened sensitivity, and notable response/recovery characteristics.
Table 1. Comparison of NO2 responses of Bi2Se3/SnSe2 sensor with that of other materials reported in literature.
The sensor based on Bi2Se3/SnSe2 demonstrates outstanding NO2 sensing performance, showcasing minimal power usage and heightened sensitivity and remarkable response/recovery characteristics. To explore the potential capability, the mechanical flexibility properties of the BS-2 sensor were further studied. The flexible gas sensor was produced by depositing the Bi2Se3/SnSe2 heterostructures onto a PET substrate with gold interdigitated electrodes (Figures 6A–C). The gas sensing characteristics of the sensor under bending angle of 30° were then determined. As displays in Figure 6D the dynamic response and recovery curves in each trial are consistent with the flat condition. Furthermore, the fatigue test of the sensor after 100, 500, and 1,000 cycles bending and relaxing processes shows no significant degradation in response values (Figure 6E). The results presented above showcase the promising application potential of the flexible Bi2Se3/SnSe2 sensor in wearable sensing devices.
Figure 6. (A–C) Optical images of the flexible gas sensing device before and after bending; (D) Response/recovery curves of the flexible sensor without and with bending to 10 ppm NO2; (E) Gas sensing stability measured after 100, 500, and 1,000 bending cycles for NO2.
The gas sensing mechanism of semiconductive materials is established on the modification of resistance caused by the interaction between gas molecules and the sensing materials. (Zappa et al., 2018; Bag and Lee, 2019). The sensing mechanism of SnSe2 has been extensively discussed in the literature. As depicted in Figure 7, when exposed to air, O2 molecules adhere to the surface of n-type SnSe2, resulting in the creation of O2− (ads) by capturing free electrons from the conduction band of SnSe2. At temperatures below 100°C, the main form of adsorbed oxygen is O2−.The redox reaction described above is illustrated in Eq. 1. (Kim et al., 2017).
When SnSe2 is exposed to NO2, the gas molecules can efficiently capture electrons from the conduction band of SnSe2 owing to the greater electronegativity of NO2 in comparison to O2. Furthermore, the reaction process can be delineated by Eqs 2, 3 below. Nevertheless, unmodified SnSe2 demonstrates a diminished response when compared to Bi2Se3/SnSe2. This phenomenon arises from the relatively low coverage of chemisorbed oxygen on the pristine SnSe2 surface. Consequently, NO2 can directly withdraw electrons from SnSe2. (Li et al., 2020). As a result, physical adsorption (Eq. 3) predominantly influences the sensing process.
The superior NO2 sensing properties of Bi2Se3/SnSe2 heterostructures are primarily attributed to the following factors building upon the foundational sensing mechanism discussed earlier:
Initially, the created n–n heterojunction between Bi2Se3 and SnSe2 plays a vital part in boosting the sensing response of the heterostructures. The electronic effects resulting from the construction of the SnSe2/Bi2Se3 heterojunction heterostructure are primarily attributed to band alignment. As depicted in Figure 8, the work function of Bi2Se3 is 4.3 eV, while the work function of SnSe2 is 4.9 eV. Therefore, the Fermi level of Bi2Se3 is notably higher than that of SnSe2. Consequently, upon the formation of the heterojunction, electrons transfer from Bi2Se3 to SnSe2, and holes transfer from SnSe2 to Bi2Se3, leading to the separation of holes and electrons. This results in the generation of an electron accumulation layer on the SnSe2 side and an electron depletion layer on the Bi2Se3 side, leading to electron accumulation on one side of SnSe2 and an improvement in the electronic structure at the interface.
Figure 8. Schematic energy band diagram of SnSe2/Bi2Se3 heterostructure (A) before equilibrium; (B) After balancing.
During the sensing process of NO2 gas-sensitive materials, when NO2 gas molecules come into contact with the material surface in a detection gas environment, the gas is first adsorbed by the material. Due to the oxidizing nature of NO2, it then captures electrons from the surface of material, resulting in a change in the carrier concentration and leading to variations in resistance and circuit current. The sensing process can be divided into two primary steps: gas adsorption, where active sites on the material surface adsorb gas, and electron reaction, where gas interacts with electrons on the material surface. (Gong et al., 2019).
In the 2D-2D SnSe2/Bi2Se3 heterostructure material, where the sensing material remains SnSe2, the aggregation of electrons on the surface of SnSe2 after heterostructure equilibrium is achieved enhances the interaction between NO2 gas and the material. This leads to a larger change in resistance signal, thereby improving the sensitivity of the material to NO2 gas. The synthesized Bi2Se3/SnSe2 2D/2D heterostructure is lamellae-stacked, which has relatively more adsorption sites and large specific surface area, which is conducive to the adsorption and desorption of NO2. Moreover, the heterostructure exhibits significantly reduced response and recovery time duing to the enhanced electron transfer rate within the material.
In summary, Bi2Se3/SnSe2 2D/2D heterostructures are successfully synthesized and used for NO2 detection. The optimized Bi2Se3/SnSe2 heterostructure exhibited rapid response towards NO2 gas. Compared to pure SnSe2, the response time was significantly reduced from 73 to 15 s (10 ppm). The enhanced sensing performance is a direct result of the abundant n-n heterojunctions, improved interface charge transfer, and increased active sites that are inherent in the SnSe2/Bi2Se3 heterostructure. Furthermore, the sensor displayed excellent selectivity, with a low detection limit of 10 ppb and a broad detection range from 10 ppb to 20 ppm. Furthermore, the fatigue test of the sensor after 100, 500, and 1,000 cycles bending and relaxing processes shows no significant degradation in response values. These results offer important insights for selecting materials and designing heterostructures to achieve effective room temperature gas detection in a range of applications.
The original contributions presented in the study are included in the article/Supplementary Material, further inquiries can be directed to the corresponding authors.
SY: Conceptualization, Data curation, Investigation, Writing–original draft. CC: Data curation, Formal Analysis, Methodology, Writing–original draft. MY: Investigation, Methodology, Writing–original draft. JH: Funding acquisition, Project administration, Supervision, Writing–review and editing. YW: Funding acquisition, Resources, Supervision, Writing–review and editing.
The author(s) declare that financial support was received for the research, authorship, and/or publication of this article. This work was supported by the National Natural Science Foundation of China (Nos. 52272147 and 52072093), Heilongjiang Touyan Team (HITTY-20190034), and the Fundamental Research Funds for the Central Universities (No. 2022FRFK02015).
The authors declare that the research was conducted in the absence of any commercial or financial relationships that could be construed as a potential conflict of interest.
All claims expressed in this article are solely those of the authors and do not necessarily represent those of their affiliated organizations, or those of the publisher, the editors and the reviewers. Any product that may be evaluated in this article, or claim that may be made by its manufacturer, is not guaranteed or endorsed by the publisher.
The Supplementary Material for this article can be found online at: https://www.frontiersin.org/articles/10.3389/fchem.2024.1425693/full#supplementary-material
Ansari, H. R., Mirzaei, A., Shokrollahi, H., Kumar, R., Kim, J.-Y., Kim, H. W., et al. (2023). Flexible/wearable resistive gas sensors based on 2D materials. J. Mater. Chem. C 11 (20), 6528–6549. doi:10.1039/d3tc00806a
Bag, A., and Lee, N.-E. (2019). Gas sensing with heterostructures based on two-dimensional nanostructured materials: a review. J. Mater. Chem. C 7 (43), 13367–13383. doi:10.1039/c9tc04132j
D'Olimpio, G., Farias, D., Kuo, C.-N., Ottaviano, L., Lue, C. S., Boukhvalov, D. W., et al. (2022). Tin Diselenide (SnSe2) Van der Waals Semiconductor: Surface Chemical Reactivity, Ambient Stability, Chemical and Optical Sensors. Materials 15 (3), 1154. doi:10.3390/ma15031154
Gong, Y., Wu, X., Zhou, X., Li, X., Han, N., and Chen, Y. (2019). High acetone sensitive and reversible P- to N-type switching NO2 sensing properties of Pt@Ga-ZnO core-shell nanoparticles. Sensors Actuators B-Chemical 289, 114–123. doi:10.1016/j.snb.2019.03.085
Guo, R., Han, Y., Su, C., Chen, X., Zeng, M., Hu, N., et al. (2019). Ultrasensitive room temperature NO2 sensors based on liquid phase exfoliated WSe2 nanosheets. Sensors Actuators B-Chemical 300, 127013. doi:10.1016/j.snb.2019.127013
Han, Y., Ding, Y., Zhang, W., Zhuang, H., Wang, Z., Li, Z., et al. (2023). SnS2/Ti3C2Tx hybrids for conductometric triethylamine detection at room temperature. Sensors Actuators B-Chemical 381, 133360. doi:10.1016/j.snb.2023.133360
Han, Y., Huang, D., Ma, Y., He, G., Hu, J., Zhang, J., et al. (2018). Design of hetero-nanostructures on MoS2 nanosheets to boost NO2 room-temperature sensing. Acs Appl. Mater. Interfaces 10 (26), 22640–22649. doi:10.1021/acsami.8b05811
Harish, S., and Sathyakam, P. U. (2022). A review of tin selenide-based electrodes for rechargeable batteries and supercapacitors. J. Energy Storage 52, 104966. doi:10.1016/j.est.2022.104966
Huang, Y., Wang, K., Guo, T., Li, J., Wu, X., and Zhang, G. (2020). Construction of 2D/2D Bi2Se3/g-C3N4 nanocomposite with High interfacial charge separation and photo-heat conversion efficiency for selective photocatalytic CO2 reduction. Appl. Catal. B-Environmental 277, 119232. doi:10.1016/j.apcatb.2020.119232
Joshi, N., Hayasaka, T., Liu, Y., Liu, H., Oliveira, O. N., and Lin, L. (2018). A review on chemiresistive room temperature gas sensors based on metal oxide nanostructures, graphene and 2D transition metal dichalcogenides. Microchim. Acta 185 (4), 213. doi:10.1007/s00604-018-2750-5
Kim, J.-H., Lee, J.-H., Mirzaei, A., Kim, H. W., and Kim, S. S. (2017). Optimization and gas sensing mechanism of n-SnO2-p-Co3O4 composite nanofibers. Sensors Actuators B-Chemical 248, 500–511. doi:10.1016/j.snb.2017.04.029
Kim, Y., Lee, S., Song, J.-G., Ko, K. Y., Woo, W. J., Lee, S. W., et al. (2020). Highly stable contact doping in organic field effect transistors by dopant-blockade method. Adv. Funct. Mater. 30 (43), 2000058. doi:10.1002/adfm.202000058
Li, M., Yang, D., Jacas Biendicho, J., Han, X., Zhang, C., Liu, K., et al. (2022). Enhanced polysulfide conversion with highly conductive and electrocatalytic iodine-doped bismuth selenide nanosheets in lithium-sulfur batteries. Adv. Funct. Mater. 32 (26). doi:10.1002/adfm.202200529
Li, X., Jia, F., Luo, N., Cai, H., Chen, J., Ren, W., et al. (2024). Self-assembly tourmaline@BiFeO3 composites with enhanced polarization for dual-selective C3H6O and H2S detection. Sensors Actuators B Chem. 399, 134806. doi:10.1016/j.snb.2023.134806
Li, X., Liu, W., Huang, B., Liu, H., and Li, X. (2020). Layered SnSe2 microflakes and SnSe2/SnO2 heterojunctions for low-temperature chemiresistive-type gas sensing. J. Mater. Chem. C 8 (44), 15804–15815. doi:10.1039/d0tc02589e
Liu, J., Hu, Z., Zhang, Y., Li, H.-Y., Gao, N., Tian, Z., et al. (2020). MoS2 nanosheets sensitized with quantum dots for room-temperature gas sensors. Nano-Micro Lett. 12 (1), 59. doi:10.1007/s40820-020-0394-6
Majhi, S. M., Rai, P., and Yu, Y.-T. (2015). Facile approach to synthesize Au@ZnO core-shell nanoparticles and their application for highly sensitive and selective gas sensors. Acs Appl. Mater. Interfaces 7 (18), 9462–9468. doi:10.1021/acsami.5b00055
Qureshi, M. A., Shah, N. J., Hemmen, C. W., Thill, M. C., and Kruse, J. A. (2003). Exposure of intensive care unit nurses to nitric oxide and nitrogen dioxide during therapeutic use of inhaled nitric oxide in adults with acute respiratory distress syndrome. Am. J. Crit. Care 12 (2), 147–153. doi:10.4037/ajcc2003.12.2.147
Rathi, K., and Pal, K. (2020). Fabrication of MoSe2–graphene hybrid nanoflakes for toxic gas sensor with tunable sensitivity. Adv. Mater. Interfaces 7 (12). doi:10.1002/admi.202000140
Sardana, S., Singh, Z., Sharma, A. K., Kaur, N., Pati, P. K., and Mahajan, A. (2022). Self-powered biocompatible humidity sensor based on an electrospun anisotropic triboelectric nanogenerator for non-invasive diagnostic applications. Sensors Actuators B-Chemical 371, 132507. doi:10.1016/j.snb.2022.132507
Spirtas, R., Steinberg, M., Wands, R. C., and Weisburger, E. K. (1986). “Identification and classification of carcinogens: procedures of the chemical substances threshold limit value committee, ACGIH. American Conference of Governmental Industrial Hygienists. ACGIH. Am. Conf. Gov. Industrial Hygienists.” Am. J. public health 76 (10), 1232–1235. doi:10.2105/ajph.76.10.1232
Wang, H., Xu, M., Ji, H., He, T., Li, W., Zheng, L., et al. (2023). Laser-assisted synthesis of two-dimensional transition metal dichalcogenides: a mini review. Front. Chem. 11, 1195640. doi:10.3389/fchem.2023.1195640
Wang, L., Jackman, J. A., Ng, W. B., and Cho, N.-J. (2016). Flexible, graphene-biocomposite for highly sensitive, real-time molecular detection. Adv. Funct. Mater. 26 (47), 8623–8630. doi:10.1002/adfm.201603550
Wang, L., Jackman, J. A., Park, J. H., Tan, E.-L., and Cho, N.-J. (2017). A flexible, ultra-sensitive chemical sensor with 3D biomimetic templating for diabetes-related acetone detection. J. Mater. Chem. B 5 (22), 4019–4024. doi:10.1039/c7tb00787f
Xu, T., Han, Y., Lin, L., Xu, J., Fu, Q., He, H., et al. (2019). Self-power position-sensitive detector with fast optical relaxation time and large position sensitivity basing on the lateral photovoltaic effect in tin diselenide films. J. Alloys Compd. 790, 941–946. doi:10.1016/j.jallcom.2019.03.293
Yang, S., Yin, H., Wang, Z., Lei, G., Xu, H., Lan, Z., et al. (2023). Gas sensing performance of In2O3 nanostructures: a mini review. Front. Chem. 11, 1174207. doi:10.3389/fchem.2023.1174207
Yang, Y., Zhu, M., Zhang, H., Wang, B., Chen, C., Li, J., et al. (2024). Room temperature gas sensor based on rGO/Bi2S3 heterostructures for ultrasensitive and rapid NO2 detection. J. Chem. Eng. 490. doi:10.1016/j.cej.2024.151872
Yang, Z., Lv, S., Zhang, Y., Wang, J., Jiang, L., Jia, X., et al. (2022). Self-Assembly 3D porous crumpled MXene spheres as efficient gas and pressure sensing material for transient all-MXene sensors. Nano-Micro Lett. 14 (1), 56. doi:10.1007/s40820-022-00796-7
Zappa, D., Galstyan, V., Kaur, N., Arachchige, H. M. M. M., Sisman, O., and Comini, E. (2018). Metal oxide -based heterostructures for gas sensors" - a review. Anal. Chim. Acta 1039, 1–23. doi:10.1016/j.aca.2018.09.020
Zhang, J., Liu, X., Neri, G., and Pinna, N. (2016). Nanostructured materials for room-temperature gas sensors. Adv. Mater. 28 (5), 795–831. doi:10.1002/adma.201503825
Keywords: 2D, NO2 Sensing, heterostructure, room-temperature, flexible sensor
Citation: Yi S, Chen C, Yu M, Hao J and Wang Y (2024) 2D/2D Bi2Se3/SnSe2 heterostructure with rapid NO2 gas detection. Front. Chem. 12:1425693. doi: 10.3389/fchem.2024.1425693
Received: 30 April 2024; Accepted: 11 June 2024;
Published: 26 July 2024.
Edited by:
Shuang Fang Lim, North Carolina State University, United StatesReviewed by:
Niravkumar J. Joshi, University of Barcelona, SpainCopyright © 2024 Yi, Chen, Yu, Hao and Wang. This is an open-access article distributed under the terms of the Creative Commons Attribution License (CC BY). The use, distribution or reproduction in other forums is permitted, provided the original author(s) and the copyright owner(s) are credited and that the original publication in this journal is cited, in accordance with accepted academic practice. No use, distribution or reproduction is permitted which does not comply with these terms.
*Correspondence: Juanjuan Hao, anloYW9AaGl0LmVkdS5jbg==; You Wang, eS13YW5nQGhpdC5lZHUuY24=
†These authors have contributed equally to this work and share first authorship
Disclaimer: All claims expressed in this article are solely those of the authors and do not necessarily represent those of their affiliated organizations, or those of the publisher, the editors and the reviewers. Any product that may be evaluated in this article or claim that may be made by its manufacturer is not guaranteed or endorsed by the publisher.
Research integrity at Frontiers
Learn more about the work of our research integrity team to safeguard the quality of each article we publish.