- 1Chemistry of Natural Compounds Department, Pharmaceutical and Drug Industries Research Institute, National Research Center, Dokki, Egypt
- 2Colorectal Research Chair, Department of Surgery, College of Medicine, King Saud University, Riyadh, Saudi Arabia
- 3Department of Chemistry, College of Science, Northern Border University, Arar, Saudi Arabia
- 4Laboratory of Genetics and Physiology of Mycobacterium, Institute of Medical Biology of the Polish Academy of Sciences, Lodz, Poland
- 5Department of Green Chemistry, National Research Center, Dokki, Egypt
- 6Institute of Chemistry, Vietnam Academy of Science and Technology (VAST), Hanoi, Vietnam
- 7Department of Chemistry, Graduate University of Science and Technology, Hanoi, Vietnam
- 8Institute of Natural Products Chemistry, Vietnam Academy of Science and Technology, Hanoi, Vietnam
- 9Department of Pharmaceutical Chemistry, Faculty of Pharmacy, Kafrelsheikh University, Kafrelsheikh, Egypt
- 10Department of Molecular Microbiology, Faculty of Biology and Environmental Protection, University of Lodz, Lodz, Poland
- 11Department of Pharmaceutical Chemistry, Faculty of Pharmacy, Pharos University in Alexandria, Alexandria, Egypt
The spread of drug-resistant tuberculosis strains has become a significant economic burden globally. To tackle this challenge, there is a need to develop new drugs that target specific mycobacterial enzymes. Among these enzymes, InhA, which is crucial for the survival of Mycobacterium tuberculosis, is a key target for drug development. Herein, 24 compounds were synthesized by merging 4-carboxyquinoline with triazole motifs. These molecules were then tested for their effectiveness against different strains of tuberculosis, including M. bovis BCG, M. tuberculosis, and M. abscessus. Additionally, their ability to inhibit the InhA enzyme was also evaluated. Several molecules showed potential as inhibitors of M. tuberculosis. Compound 5n displayed the highest efficacy with a MIC value of 12.5 μg/mL. Compounds 5g, 5i, and 5n exhibited inhibitory effects on InhA. Notably, 5n showed significant activity compared to the reference drug Isoniazid. Molecular docking analysis revealed interactions between these molecules and their target enzyme. Additionally, the molecular dynamic simulations confirmed the stability of the complexes formed by quinoline-triazole conjugate 5n with the InhA. Finally, 5n underwent in silico analysis to predict its ADME characteristics. These findings provide promising insights for developing novel small compounds that are safe and effective for the global fight against tuberculosis.
1 Introduction
Since its identification in 1882, Mycobacterium tuberculosis (MTB), commonly referred to as Koch’s bacillus, has continued to exert a significant impact on global health (Barberis et al., 2017). Tuberculosis (TB), caused by the bacterium MTB is consistently listed among the leading 10 causes of mortality worldwide (Ravimohan et al., 2018). At present, approximately 1.7 billion people, constituting 23% of the world’s population, grapple with MTB, resulting in over 10 million new TB cases annually (Daley, 2019). Current TB treatment is lengthy, arduous, and associated with numerous side effects; a course of antibiotics is typically prescribed for a duration of 6–9 months for drug-susceptible tuberculosis, while cases of multidrug-resistant tuberculosis (MDR-TB) or instances of emerging drug resistance may necessitate treatment durations of 9–20 months (Esmail et al., 2014). A strain of tuberculosis that exhibits resistance to the two primary drugs, rifampicin and isoniazid (INH), is classified as MDR-TB (Seung et al., 2015). Conversely, the extensively drug-resistant tuberculosis strain (XDR-TB) represents an MDR-TB form that is resistant not only towards additional fluoroquinolones but also to at least one of levofloxacin, moxifloxacin, bedaquiline, and linezolid (WHO Report, 2020).
TB agents cannot reach the target site due to the intricate structure and poor permeability of mycobacteria’s cell envelope (Jackson, 2014). Fatty acid synthase type I and type II (FAS-I and FAS-II) control the synthesis of the mycobacterial cell envelope (Marrakchi et al., 2000; Lu and Tonge, 2008). While FAS-I is exclusive to eukaryotic cells, the FAS-II enzyme emerges as a viable candidate for pharmaceutical development. Enoyl acyl carrier protein reductase (InhA), an enzyme of the FAS-II system, assumes a pivotal role in the saturation of double bonds in fatty acid chains linked to acyl carrier protein (ACP) (Rožman et al., 2017). Isoniazid, a primary treatment for tuberculosis, inhibits the enzyme InhA, thereby blocking mycolic acid production (Dessen et al., 1995). However, for INH to exhibit its pharmacological activity, it must undergo an activation process facilitated by the catalase-peroxidase enzyme KatG. Such a process entails the covalent INH binding to the NADH cofactor located in the InhA binding cavity (Munir et al., 2021). Over time, different strains of MTB have acquired resistance to INHdue to genetic mutations in the KatG gene (Muthaiah et al., 2017). Consequently, researchers have been motivated to explore new compounds that directly target InhA without relying on KatG activation (Almeida Da Silva and Palomino, 2011).
Quinoline is a commonly occurring structural framework present in many natural anti-tuberculosis products and medications (Keri and Patil, 2014), besides its diverse biological effects (Mohamede et al., 2015; Abdelrahman et al., 2022; Elbadawi et al., 2022; Elkaeed et al., 2022; Sabt et al., 2023a; Sabt et al., 2023b; Khaleel et al., 2024). Bedaquiline I (TMC207, Sirturo) (Figure 1) which contains a diarylquinoline core, received approval from the US-FDA for the treatment of pulmonary MDR-TB, marking the end of a 40-year delay. As an inhibitor of ATP synthase, this compound exhibits remarkable potency against both replicating and non-replicating strains (Pym et al., 2016). 1n 2023, Quimque and colleagues (Quimque et al., 2023) created and synthesized new arylated quinoline carboxylic acids (QCAs) that effectively inhibited the pathogen MTB. Compound II (Figure 1) was found to have the highest potency, with a minimum inhibitory concentration (MIC) of around 16 μM. Furthermore, anti-mycobacterial action was also demonstrated for 4-aminoquinoline-isoindolindione-isoniazidhybrid III (Figure 1), with a MIC of 5.1 µM (Zhou et al., 2010). Yaddanapudi and colleagues (Kumar Sahoo et al., 2022) exploited the quinoline motif and combined it with isoxazole alkyl ester to synthesize potent hybrid compounds IVa-b (Figure 1) with high activity towards MTB, exhibiting a MIC value of 1 μg/mL.
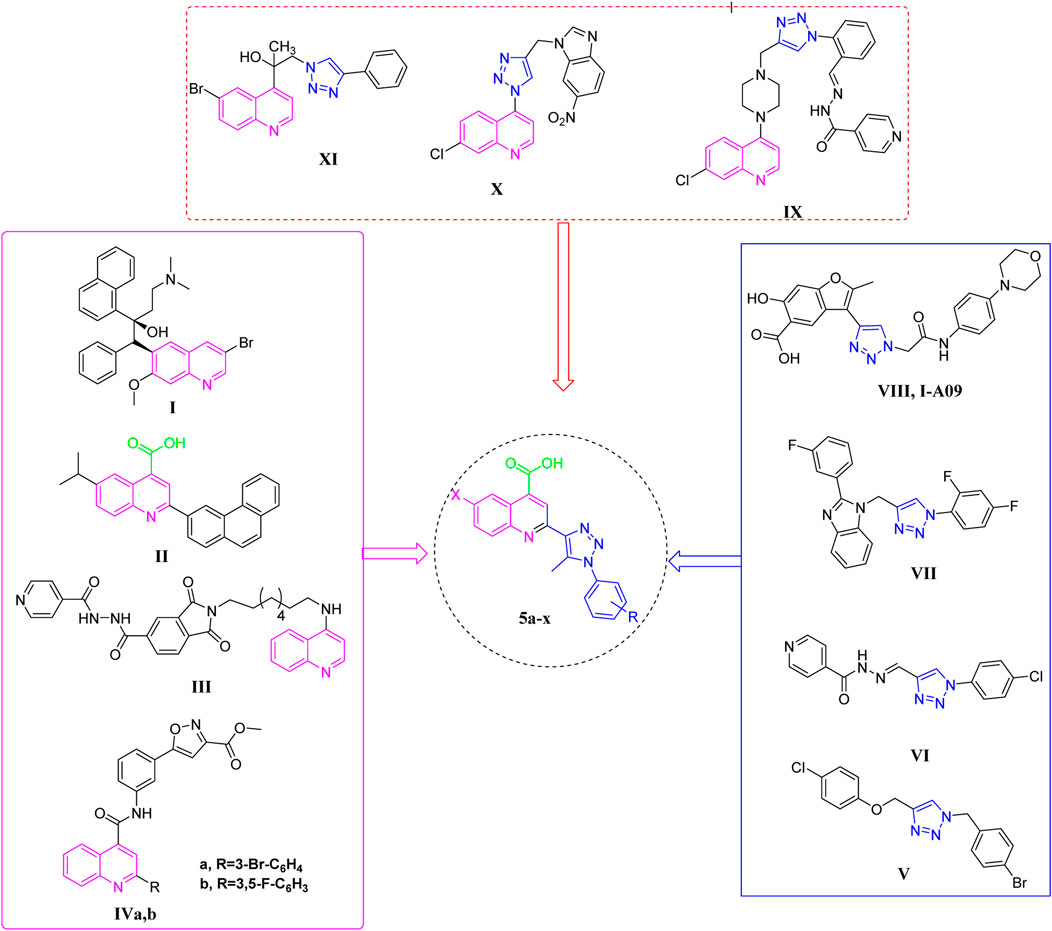
Figure 1. Reported antitubercular agents containing bioactive cores quinoline (I-IV), triazoles (V-VIII), quinoline-triazole hybrids (IX-XI), and our newly designed compounds (5a-x).
In recent times, triazole tethered small molecules have emerged as a significant category of organic compounds due to their diverse array of biological applications, e.g., anti-tubercular (Dhameliya et al., 2023), antibacterial (Li and Zhang, 2022), anti-viral (Senthil et al., 2015), and anticancer (Said et al., 2020; Azab et al., 2022; Elsawi et al., 2023; Elsawi et al., 2024). Notably, derivatives of 1,2,3-triazole have demonstrated promising anti-tubercular activity, prompting the development of numerous synthetic methodologies for their production (Zhang et al., 2017; El-Shoukrofy et al., 2023). Recent research has focused on the synthesis of a spectrum of small molecules containing conjugated 1,2,3-triazoles, which have exhibited various bioactivities. For instance, Shingate and colleagues have reported the inhibitory activities of 1,4-disubstituted 1,2,3-triazole-based molecules (Compound V, Figure 1) against MTB (Shaikh et al., 2015). Additionally, the clubbed 1,2,3-triazoles with INH, such as compound VI (Figure 1), have been identified as inhibitors of the MTB H37Rv strain MIC = 0.62 ug/mL (Boechat et al., 2011). Furthermore, the hybridization of fluorine-containing benzimidazole series and triazoles resulted in compound VII (Figure 1) that have also been reported to be a potent inhibitor of tuberculosis with MIC = 6.25 ug/mL (Gill et al., 2008). Notably, in clinical trials, I-A09, a compound containing triazole (VIII, Figure 1) is being studied as an anti-TB drug (Dadlani et al., 2022). In addition, certain quinoline-appended triazoles IX-XI (Figure 1) showed potent antitubercular actions (Alcaraz et al., 2022; Nyoni et al., 2023; Shinde et al., 2023).
Based on the facts mentioned above, along with the biological importance of quinoline and 1,2,3-triazole cores, coupled with the ongoing exploration for novel anti-infective compounds as potential anti-tubercular agents, the present investigation aims to explore the potential inhibitory impacts for 4-carboxy quinolino-triazole hybrids on the InhA target as anti-tuberculous agents. The current work employs a molecular hybridization strategy to design these molecules with the potential inhibitory antitubercular effects. The research involves the synthesis of a range of derivatives of 4-carboxy quinoline core, each featuring distinct substituents within the triazole moiety, such as halogens, methoxy, and nitro. Subsequently, the synthesized compounds undergo assessment for their effectiveness against various strains such as Mycobacterium bovis BCG, M. abscesses, and M. tuberculosis. The most potent molecules are subjected to further scrutiny for the inhibitory efficacy towards the MTB InhA. Furthermore, molecular docking investigations, as well as molecular dynamics (MD) studies, are performed to scrutinize the interactions between these biologically active analogues and the InhA enzyme. Lastly, the pharmacokinetics parameters for select analogues undergo exploration utilizing web-based ADMET predictors.
2 Results and discussion
2.1 Organic chemistry work
The synthetic procedures used to prepare the quinolino-triazole hybridized molecules 5 are described in Scheme 1. As previously reported, the key intermediates acetyl triazoles 3a-h were prepared via a 1,3-dipolar cycloaddition reaction (Bekheit et al., 2021), that was performed through the diazotization of aniline derivatives 1a-h before reacting with sodium azide to yield the corresponding azido derivatives 2a-h. The reaction of azido compounds 2a-h with acetylacetone was done in the presence of anhydrous potassium carbonate in refluxing ethanol, producing acetyl triazole derivatives 3a-h. The desired products, quinoline triazole conjugates 5a-x, were synthesized using Pfitzinger conditions (Elghamry and Al-Faiyz, 2016) by refluxing acetyl triazole derivatives 3a-h with the isatin derivatives 4a-c and adding an aqueous solution of KOH, then acidifying with dilute hydrochloric acid producing the 4-carboxyquinoline-triazole derivatives 5a-x. The newly synthesized compounds underwent microanalyses and spectrum analysis, including 1H-NMR and 13C-NMR. The collected data aligned with the predetermined structures of the produced molecules.
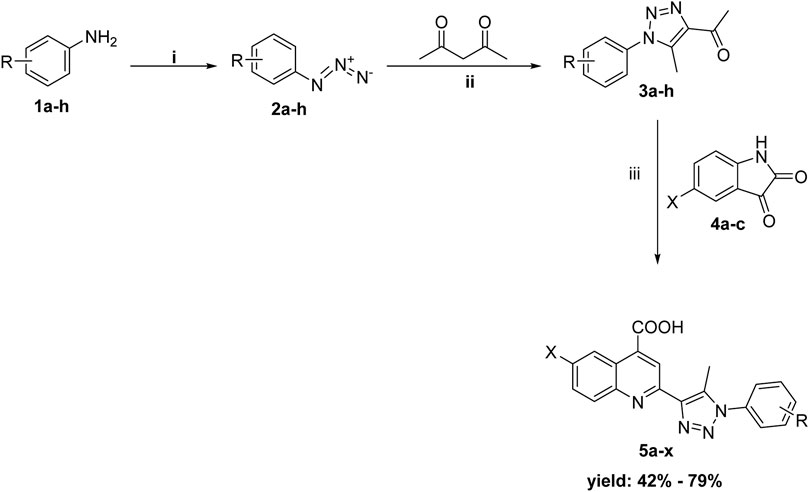
Scheme 1. Synthesis of the target conjugates (5a-x); Reagents and conditions: (i) a) NaNO2/HCl/0–5°C,1h, then r.t 1h b) NaN3/stirring/rt/3h; (ii) K2CO3/EtOH/reflux; (iii) a) aq KOH/EtOH/reflux for 12 h, b) H3O+.
2.2 Biological activities
2.2.1 Antimycobacterial assessment
All carboxy quinoline triazole compounds underwent assessment for potential anti-tubercular efficacy towards tubercle bacilli, encompassing both M. bovis BCG and MTB, as well as nontuberculous opportunistic pathogen represented by fast-growing mycobacteria, notably M. abscessus. This type of mycobacteria is characterized by a heightened resistance to most anti-tuberculous drugs (Johansen et al., 2020). The primary screening was performed for all compounds at the same concentration of 125 μg/mL. None of the compounds displayed an anti-tubercular effect at the tested concentration against M. abscessus. Conversely, all 24 tested compounds suppressed the development of M. bovis BCG and MTB. Next, using MABA (microplate alamar blue assay), the MICs against MTB were assessed for all the reported molecules (Table 1). The majority of the compounds investigated demonstrated favorable to moderate anti-tubercular activity against MTB.
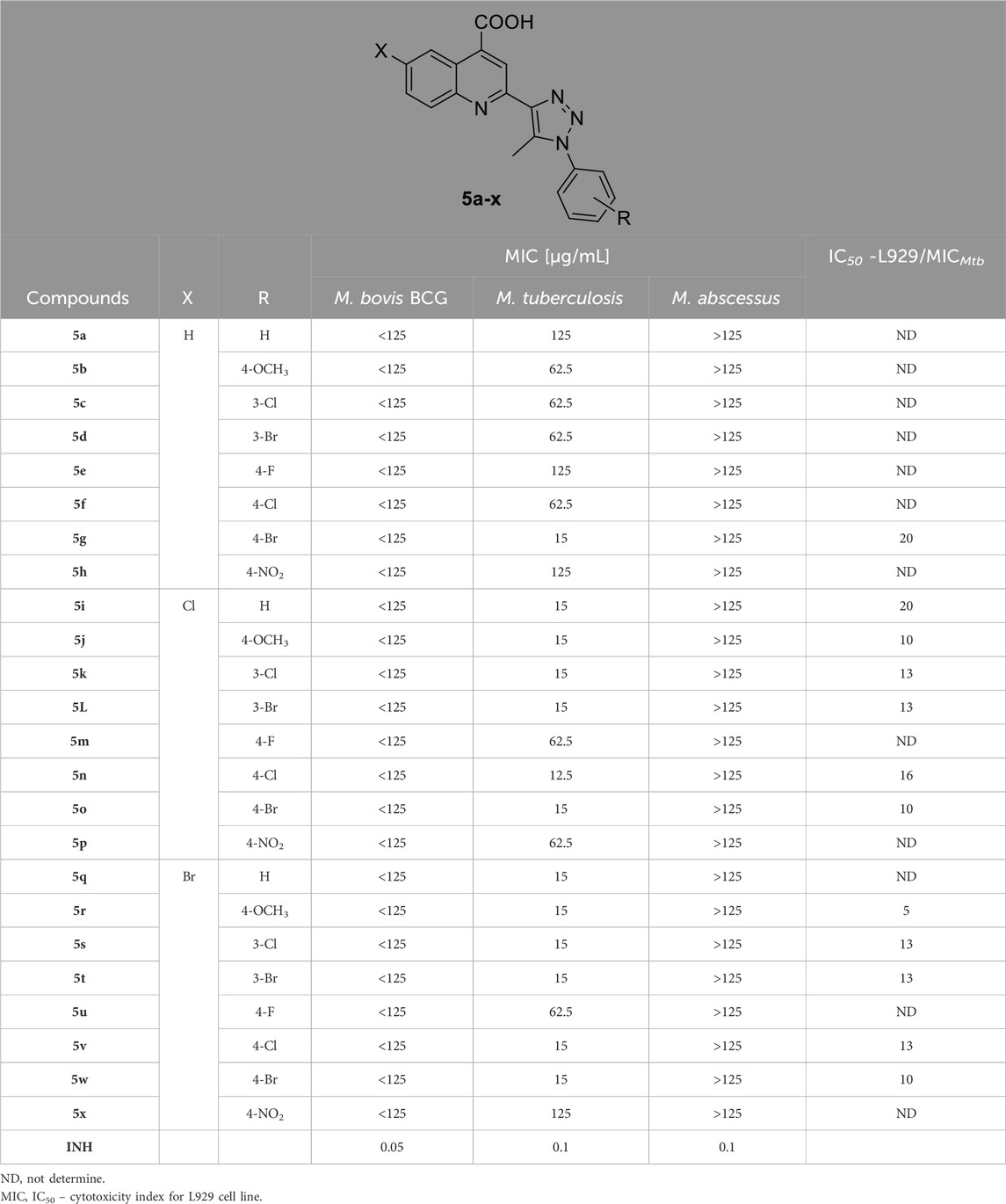
Table 1. The anti-tubercular effectiveness (MICs; µg/mL) of the reported compounds (5a-x) tested toward Mycobacterium bovis BCG, M. tuberculosis, and M. abscessus strains.
The derivative 5n exhibited the most potent anti-tubercular effect toward MTB, with an MIC value of 12.5 μg/mL. Conversely, twelve of the compounds investigated (5g, 5i-l, 5o, 5q-t, 5v, and 7w) demonstrated effective activity, with an MIC value equal 15 μg/mL. Additionally, seven compounds (5b-d, 5f, 5m, 5p, and 5u) displayed moderate anti-tubercular efficacy towards Mycobacterium (MIC = 62.5 μg/mL). The remaining derivatives (5a, 5e, 5h, and 5x) exhibited lower activity, with the lowest MIC recorded at 125 μg/mL (Table 1). Moreover, compounds demonstrating the highest potency with MIC values ≤ 15 μg/mL underwent a more comprehensive assessment of their cytotoxicity effects. This evaluation adhered precisely to international standards (ISO 10993-5:2009(E)), employing L929 cells and the MTT protocol (Bekier et al., 2021). The determination of the half maximal inhibitory concentration (IC50) values was carried out for 12 out of the 13 compounds tested, considering the occurrence of derivative precipitation in the growth medium for L929 cells (Table 1). With the exception of 5r, the tested compounds showed low cytotoxicity at concentrations up to 10xMIC. For two compounds (5g and 5i), the discriminatory index (IC50/MIC) was identified at the level of 20.
Within the synthesized quinoline compounds with phenyltriazole substituents, compound 5g containing a 4-bromo substituent showed the strongest anti-tubercular effects against M. tuberculosis with a MIC value of 15 μg/mL. Moreover, compounds 4b, 5c, 5d, and 5f with 4-methoxyphenyl, 3-chlorophenyl, 3-bromophenyl, and 4-chlorophenyl substituents, respectively exhibited decreased activity with MIC values of 62.5 μg/mL, whereas, compounds 5a, 5e, and 5h with unsubstituted phenyltriazole, 4-fluorophenyl, and 4-nitrophenyl showed even lower activity with MIC values of 125 μg/mL.
In the case of 6-chloroquinoline compounds with phenyltriazole substituents, compound 5n with a 4-chlorophenyl substituent demonstrated the highest activity with a MIC of 12.5 μg/mL. Substituting this chlorophenyl with unsubstituted, 4-methoxy, 3-chloro, 3-bromo, and 4-bromo in compounds 5i, 5j, 5k, 5L, and 5o, respectively, led to a slight decrease in activity with a MIC of 15 μg/mL. However, compounds 5m and 5p with 4-fluorophenyl and 4-nitrophenyl substituents showed a significant decrease in activity with a MIC of 62.5 μg/mL.
For 6-bromoquinoline compounds with phenyltriazole substituents, compounds 5q, 5r, 5s, 5t, 5v, and 5w containing unsubstituted phenyl, 4-methoxyphenyl, 3-chlorophenyl, 3-bromophenyl, 4-chlorophenyl, and 4-bromophenyl, respectively exhibited the most potent anti-tubercular effects with MIC values of 15 μg/mL. On the other hand, counterparts 5u and 5x with 4-fluorophenyl and 4-nitrophenyl showed reduced activity with MIC values of 62.5 and 125 μg/mL. Overall, compounds with substituted quinoline containing chloro and bromo groups displayed significant activity.
2.2.2 Inhibitory effect toward MTB InhA
To assess the effectiveness of 4-carboxyquinoline-triazole hybrids 5g, 5i, and 5n, which displayed notable cytotoxic effects and potent activity against MTB, further scrutiny was conducted to gauge their capacity to impede the InhA enzyme. In this analysis, INH served as a positive control. The outcomes, as displayed in Table 2, reveal that the examined derivatives effectively suppressed the InhA enzyme within the micromolar concentration ranges, recording IC50 values ranging from 0.72 ± 0.03 to 11.83 ± 0.49 µM. Remarkably, compound 5n, exhibiting the best activity toward MTB with a MIC value of 12.5 μg/mL, also demonstrated substantial inhibition of the InhA enzyme (IC50 = 0.72 ± 0.03 µM), on par with that of INH (IC50 = 0.24 ± 0.01 µM). These results validated the inhibitory activity of compound 5n against the InhA enzyme. The plausible binding mode for these analogues (5g, 5i, and 5n) in their interaction with InhA was explored through molecular docking, a discussion of which follows in the subsequent section.
2.3 In silico insights
2.3.1 Molecular docking analysis
In order to enhance comprehension of the interaction mechanisms occurring at the binding site of a specific protein, molecular docking simulations were performed on compounds exhibiting strong in vitro effects towards the InhA enzyme of MTB. The docking results disclosed that 4-carboxyquinoline-triazole hybrids 5g, 5i, and 5n exhibited binding affinities (ΔGdock) of −8.182, −9.337, and −9.493 kcal/mol, respectively. Notably, compound 5n demonstrated the highest binding affinity within the active site of InhA, consistent with in vitro experimental findings.
The 2D and 3D interactions of the three compounds (5g, 5i, and 5n) are detailed in Figure 2. These compounds formed hydrogen bonds between the oxygen atom of the carboxylic functionality in the molecule and the N-H of the amino acid residue Lys165. The hydrogen bond distances for these compounds with this residue were observed to be 2.51 Å, 2.45 Å, and 2.33 Å, respectively. Specifically, compound 5n exhibited the shortest hydrogen bond distance, explaining its strongest affinity among the studied compounds due to the crucial role of hydrogen bonding in the protein-ligand complex. Furthermore, halogen bonding was identified in the InhA complex with this compound at residue Pro156. Various interactions, including pi-pi stacked, pi-sigma, alkyl, pi-alkyl, pi-sulfur, pi-donor hydrogen bonding, and van der Waals were observed in the binding site of InhA, contributing to the stability of the complex. Generally, the fused benzene ring of the quinoline scaffold in the studied compounds, and pi-sigma interactions with residue Ile21 were established.
In more detail, compound 5g established alkyl interactions with residues Leu197 and Ala198, along with pi-sigma interactions with Ala198 and Ile21. Compound 5i formed three pi-sulfur interactions with Met199 and pi-pi stacked interactions with Phe149. Pi-alkyl and alkyl interactions were also noticed in the InhA-5i complex with amino acid residues Ile215, Tyr158, and Ile21. This was also evident in the complexes InhA-5n and InhA-reference. Furthermore, compound 5n exhibited additional pi-alkyl and alkyl interactions with other amino acid residues such as Met199 and Ala157.
2.3.2 Molecular dynamics simulation
The best-docked pose with the most negative binding affinity of the top-lead compound 5n obtained from the AutoDock Vina program was utilized as the input structure for a 100 ns MD simulation. The output result of the best docking was used to establish this procedure in a high-throughput fashion and scrutinize the dynamic binding modes of the ligand at the protein’s active site in explicit water conditions.
The examination of alterations in the conformation of the ligand-protein complex was evaluated by calculating the root-mean-square deviation (RMSD) from the original structure in order to assess the structural stability. The average RMSD value for the compound 5n was determined to be approximately 0.229 nm, Figure 3A. From the protein RMSD chart, it can be observed that the structure undergoes slight changes, with the RMSD value increasing slightly over the first 20 ns and then stabilizing to form a well-defined complex throughout the simulation with an RMSD value around 2.3 Å. The accepted range for RMSD values is typically less than 3.0 Å, with lower RMSD values signifying enhanced stability within the system (Kufareva and Abagyan, 2012). In contrast, the ligand 5n in the complex shows minor changes with an RMSD value less than 2.0 Å. Its average RMSD value is calculated as 0.11 nm, demonstrating the durability of the ligand within the active site of the InhA protein, which is illustrated in Figure 3B.
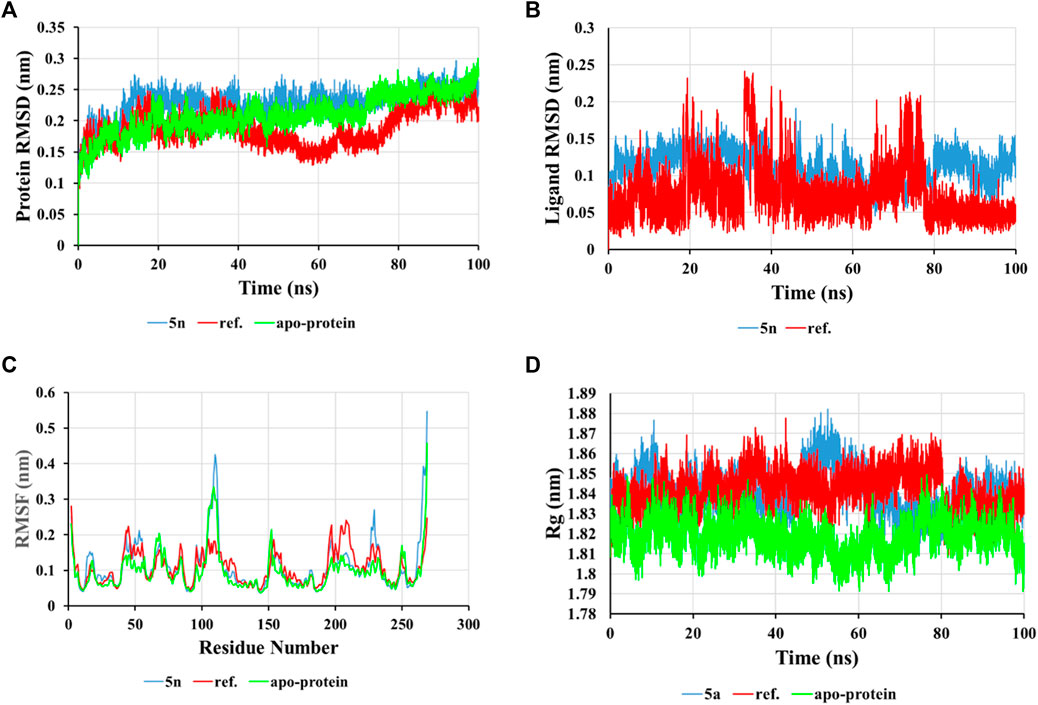
Figure 3. RMSD of protein backbone (A) and ligand (B) in solvated InhA-5n complex, RMSF values of the complex (C), and Radius of gyration (Rg) of the complex (D) during 100 ns simulation time.
The root-mean-square fluctuation (RMSF) for the ligand-protein complex was graphed using the 100 ns MD trajectory to assess the mean fluctuation and flexibility of each specific amino acid (Figure 3C). The RMSF chart indicates the varying degrees of fluctuation observed in amino acid residues within the protein during the interaction state with the ligand over certain time intervals. This chart illustrates that the fluctuations in residues during interaction with compound 5n are greater compared to the residues of the apo-protein, particularly concentrated in the regions from 100 to 120 and 227 to 230. This observation implies that the compound 5n has a comparable stabilizing effect on this protein section.
The compactness of the InhA-5n complex was analyzed using a radius of gyration (Rg) chart. From the displayed results, a small change in Rg was observed at the 40–60 ns time point, followed by sustained stability for the ligand-protein complex during the simulation, with an average value of 1.841 nm, similar to the reference compound and apo-protein (Rg values of 1.841 and 1.819 nm, respectively), indicating the tightness of the structure (Figure 3D). In summary, the results indicate that the InhA-5n system remains stable throughout the 100 ns MD simulation under virtual physiological conditions.
2.3.3 ADMET
The compound 5n, with its molecular weight of 399.23 g/mol, 5 hydrogen bond acceptors, 1 hydrogen bond donor, molar refractivity of 104.04, and LogP of 3.71, is being investigated for its potential to inhibit InhA, and its drug-likeness and pharmacokinetics are currently under research. According to Supplementary Table S1, all these parameter values meet Lipinski’s proposed criteria (Lipinski et al., 1997). Additionally, the Absorption, Distribution, Metabolism, Excretion, and Toxicity (ADMET) profile of the synthesized compound 5n has been preliminarily assessed to make pharmacokinetic predictions for potential drug candidates in clinical studies.
As shown in Supplementary Table S2, ADMET profiling results indicate that compound 5n has a high human intestinal absorption value of 94.021%. It exhibits high permeability through Caco-2 with a predicted value of 1.389 (>0.90). The skin permeation ability is high with a logKp value of −2.735 (<−2.5). CNS permeability study demonstrates good penetration (logPS value of −1.934 > −2), while the compound cannot cross the blood-brain barrier (logBB = −1.043 < −1) (Supplementary Table S2, Figure 4). Cytochrome P450 enzymes in the liver are generally not inhibited, except for cytochrome P2C9, a key enzyme in drug metabolism, which may be inhibited by this compound. The excretion is evaluated based on the total clearance, an important parameter in determining the dosing interval. Data indicates that the compound 5n has a total clearance value of 0.192 log mL/min/kg. Toxicity parameters assessed in the ADMET profile of the new InhA inhibitor 5n show inactivity with carcinogenicity, immunotoxicity, mutagenicity, cytotoxicity, no AMES toxicity, and no inhibition of hERG (I and II). However, it exhibits undesired hepatotoxicity. The toxicity level of 4, as indicated by the ProTox II web server, suggests the relative toxicity of compound 5n (Banerjee et al., 2018a). Furthermore, the estimated Oral Rat Acute Toxicity (LD50) and Oral Rat Chronic Toxicity values for the synthesized compound 5n are 2.822 mol/kg and 0.528 log mg/kg_bw/day, respectively.
3 Conclusion
In conclusion, the synthesized carboxyquinoline-triazole compounds 5a-x in this investigation demonstrated promising potential as anti-tubercular agents, particularly against MTB. Despite not exhibiting significant activity against the highly resistant M. abscessus, all 24 compounds effectively suppressed the growth of M. bovis BCG and MTB. Among them, derivative 5n emerged as the most potent, with a MIC value of 12.5 μg/mL against MTB, comparable to the standard drug INH. Moreover, the cytotoxicity assessment revealed low toxicity for most compounds, with compound 5n demonstrating a favorable IC50-L929/MICMTB ratio of 16. Further investigation into the inhibition of the MTB InhA enzyme confirmed the efficacy of derivatives 5g, 5i, and 5n, with compound 5n displaying substantial inhibition with an IC50 value closely mirrored that of INH. Molecular docking simulations elucidated the binding affinities and interactions of these compounds within the active site of InhA, suggesting a comparable binding mode to reported InhA inhibitors and emphasizing the importance of hydrogen and halogen bonding, as well as various other interactions contributing to stability. Additionally, an MD analysis supported the stability of the InhA-5n complex over 100 ns, corroborating the binding mode observed in docking studies. ADMET profiling indicated favorable drug-like properties for compound 5n that comply with Lipinski’s rule of five, including high intestinal absorption, permeability, and minimal toxicity, albeit with some potential for hepatotoxicity. Overall, compound 5n stands out as a promising lead compound warranting further exploration as a potential anti-tubercular agent with favorable pharmacokinetic properties and low toxicity profile, offering a potential avenue for the development of novel compounds to combat tuberculosis and mitigate the challenge of resistance.
4 Experimental section
4.1 Chemistry
Melting points have been determined using the Electrothermal IA-9000 apparatus and have been reported without correction. The 1H NMR and 13C NMR spectra were recorded using Bruker Avance 500 MHz spectrometer (500 MHz 1H and 126 MHz 13C NMR). Deuterated dimethylsulfoxide (DMSO-d6) was used as a solvent in all samples. The progression of the reactions was observed through TLC using silica gel on aluminium sheets 60 F254 from Merck, with CHCl3/MeOH (9.5: 0.5 v/v) as the eluent, and iodine-potassium for visualization. It is important to mention that compounds 3a-h had been synthesized previously (Dong et al., 2010; Singh et al., 2013; Bekheit et al., 2021).
4.1.1 General procedures for preparing targeted 4-carboxyquinoline-triazole derivatives (5a-x)
Stirring a solution of isatin derivatives 4a-c (1 mmol) and potassium hydroxide (2.5 mmol) in 5 mL of water was conducted at room temperature for 15–30 min. Following this, the reaction mixture received an addition of acetyl triazoles derivatives 3a-h (1 mmol) and 10 mL of ethyl alcohol. Refluxing the reaction took place for a duration of 12 h, and subsequently, the mixture was acidified to achieve a pH of 2–3 using diluted HCl, resulting in the formation of a precipitate. This precipitate was then subjected to filtration, and underwent recrystallization after a water-washing process, ultimately yielding the pure target products 4-carboxyquinoline-triazole derivatives 5a-x.
2-(5-Methyl-1-phenyl-1H-1,2,3-triazol-4-yl)benzo [b]pyridine-4-carboxylic acid (5a).
Orange powder; mp 278°C–280°C; yield (63%); 1H NMR (500 MHz, DMSO-d6) δ = 2.60 (s, 3H, triazole CH3), 6.86-6.88 (m, 1H, H-Arm), 7.01-7.04 (m, 1H, H-Arm), 7.45-7.47 (m, 1H, H-Arm), 7.53-7.56 (m, 1H, H-Arm), 7.59-7.65 (m, 6H, H3 of quinoline and H-Arm), 11.00 (brs, 1H, C(O)OH); 13C NMR (126 MHz, DMSO-d6) δ 10.23 (triazole CH3), 112.73, 118.35, 123.29, 125.20, 125.95 (2C), 130.27 (2C), 130.67, 135.54, 138.21, 138.90 (Arm), 143.38 (=C-N, triazole), 151.27 (C9 of quinoline), 159.88 (C2 of quinoline), 184.91 (C(O)OH); Analysis for C19H14N4O2, M.wt. (330.35 g/mol), Calcd.: % C, 69.08; H, 4.27; N, 16.96; Actual: % C, 68.96; H, 4.29; N, 17.03 (Pokhodylo et al., 2009).
2-(1-(4-Methoxyphenyl)-5-methyl-1H-1,2,3-triazol-4-yl)benzo[b]pyridine-4-carboxylic acid (5b).
Yellow powder; mp 222°C–224°C; yield (65%); 1H NMR (500 MHz, DMSO-d6) δ = 2.62 (s, 3H, triazole CH3), 3.85 (s, 3H, OCH3), 7.16 (d, 2H, J = 8.8 Hz, H-Arm), 7.50-7.56 (m, 5H, H-Arm), 7.58 (dd, 1H, J = 8.8 and 2.5 Hz, H-Arm), 8.73 (s, 1H, H3 of quinoline), 11.06 (s, 1H, C(O)OH); 13C NMR (126 MHz, DMSO-d6) = δ 9.67 (triazole CH3), 55.65 (OCH3), 112.25, 114.79 (2C), 117.80, 122.76, 124.67, 125.55, 126.92 (2C), 127.76, 134.60, 130.23, 137.74, 138.38 (Arm), 142.68 (=C-N, triazole), 150.79 (C9 of quinoline), 159.40 (C2 of quinoline), 167.38 (C(O)OH). Analysis for C20H16N4O3, M.wt. (360.37 g/mol), Calcd: % C, 66.66; H, 4.48; N, 15.55; Actual: % C, 66.78; H, 4.46; N, 15.49.
2-(1-(3-Chlorophenyl)-5-methyl-1H-1,2,3-triazol-4-yl)benzo [b]pyridine-4-carboxylic acid (5c).
Orange powder; mp 276°C–277°C; yield (51%); 1H NMR (500 MHz, DMSO-d6) δ = 2.59 (s, 3H, triazole CH3), 6.86 (d, 1H, J = 7.5 Hz, H-Arm), 7.00-7.03 (m, 1H, H-Arm), 7.44 (d, 1H, J = 7.5 Hz, H-Arm), 7.52-7.55 (m, 1H, H-Arm), 7.59 (d, 1H, J = 7.5 Hz, H-Arm), 7.63-7.69 (m, 3H, H-Arm), 7.97 (s, 1H, H3 of quinoline), 11.01 (brs, 1H, C(O)OH); 13C NMR (126 MHz, DMSO-d6) δ = 10.13 (triazole CH3), 112.74, 118.31, 123.27, 124.81, 125.17, 125.92, 130.71, 131.90, 134.48, 136.67, 138.51, 138.88 (Arm), 143.35 (=C-N, triazole), 151.27 (C9 of quinoline), 159.85 (C2 of quinoline), 185.06 (C(O)OH); Analysis for C19H13ClN4O2, M.wt. (364.79 g/mol), Calcd.: % C, 62.56; H, 3.59; N, 15.36; Actual: % C, 62.54; H, 3.60; N, 15.32.
2-(1-(3-Bromophenyl)-5-methyl-1H-1,2,3-triazol-4-yl)benzo [b]pyridine-4-carboxylic acid (5d).
Buff powder; mp 248°C–250°C; yield (78%); 1H NMR (500 MHz, DMSO-d6) δ = 2.60 (s, 3H, triazole CH3), 6.87 (d, 1H, J = 8.0 Hz, H-Arm), 7.01–7.04 (m, 1H, H-Arm), 7.45 (d, 1H, J = 7.5 Hz, H-Arm), 7.53–7.60 (m, 2H, H-Arm), 7.64-7.65 (m, 1H, H-Arm), 7.81 (d, 2H, J = 8.0 Hz, H-Arm), 7.90 (s, 1H, H3 of quinoline); 11.01 (brs, 1H, C(O)OH); 13C NMR (126 MHz, DMSO-D6) δ = 10.90 (triazole CH3), 114.37, 115.30, 119.62, 121.52, 124.62, 125.02, 127.34, 127.47, 128.89, 131.09, 131.93, 132.84, 135.31, 137.78(Arm), 141.91(=C-N, triazole), 152.50 (C9 of quinoline), 160.67(C2 of quinoline), 183.87(C(O)OH).Analysis for C19H13BrN4O2, M.wt. (409.24 g/mol), Calcd.: % C, 55.76; H, 3.20; N, 13.69; Actual: % C, 55.95; H, 3.19; N, 13.63.
2-(1-(4-Fluorophenyl)-5-methyl-1H-1,2,3-triazol-4-yl)benzo [b]pyridine-4-carboxylic acid (5e).
Buff powder; mp 290°C–291°C; yield (62%); 1H NMR (500 MHz, DMSO-d6) δ = 2.81 (s, 3H, triazole CH3), 7.49–7.52 (m, 2H, H-Arm), 7.67–7.70 (m, 1H, H-Arm), 7.75–7.84 (m, 3H, H-Arm), 8.09 (d, 1H, J = 8.0 Hz, H-Arm), 8.70-8.71 (m, 2H, H3 and H8 of quinoline), 13.98 (brs, 1H, C(O)OH); 13C NMR (126 MHz, DMSO-d6) δ = 11.01 (triazole CH3), 117.12, 117.33, 120.22, 123.90, 126.11, 128.37, 128.50, 128.56, 129.99, 130.80, 135.31, 137.41, 142.41 (=C-N, triazole), 148.75 (C9 of quinoline), 151.88 (C-F), 157.21 (C2 of quinoline), 167.88 (C(O)OH); Analysis for C19H13FN4O2, M.wt. (348.34 g/mol), Calcd.: % C, 65.51; H, 3.76; N, 16.08; Actual: % C, 65.56; H, 3.78; N, 16.04.
2-(1-(4-Chlorophenyl)-5-methyl-1H-1,2,3-triazol-4-yl)benzo [b]pyridine-4-carboxylic acid (5f).
Yellow powder; mp 246°C–248 °C; yield (63%); 1H NMR (500 MHz, DMSO-d6) δ = 2.60 (s, 3H, triazole CH3), 6.86 (d, 1H, J = 8.0 Hz, H-Arm), 7.01–7.10 (m, 1H, H-Arm), 7.45 (d, 1H, J = 7.5 Hz, H-Arm), 7.53–7.56 (m, 1H, H-Arm), 7.81–7.85 (m, 3H, H-Arm), 8.01 (d, 1H, J = 8.0 Hz, H-Arm), 8.7 (s, 1H, H3 of quinoline), 11.00 (brs, 1H, C(O)OH); 13C NMR (126 MHz, DMSO-d6) δ = 9.65 (triazole CH3), 112.19, 122.74, 124.65, 125.55, 127.21, 127.78, 129.77, 130.22, 133.79, 134.81, 137.89, 138.35 (Arm), 142.85 (=C-N, triazole), 150.70 (C9 of quinoline), 159.46 (C2 of quinoline), 167.33 (C(O)OH). Analysis for C19H13ClN4O2, M.wt. (364.79 g/mol), Calcd.: % C, 62.56; H, 3.59; N, 15.36; Actual: % C, 62.58; H, 3.58; N, 15.40 (Pokhodylo et al., 2009).
2-(1-(4-Bromophenyl)-5-methyl-1H-1,2,3-triazol-4-yl)benzo [b]pyridine-4-carboxylic acid (5g).
Yellow powder; mp 228°C–229°C; yield (58%); 1H NMR (500 MHz, DMSO-d6) δ = 2.61 (s, 3H, triazole CH3), 7.58–7.60 (m, 3H, H-Arm), 7.82–7.85 (m, 4H, H-Arm), 8.10 (d, 1H, J = 8.0 Hz, H-Arm), 8.71 (s, 1H, H3 of quinoline), 11.00 (brs, 1H, C(O)OH); 13C NMR (126 MHz, DMSO-d6) δ = 10.17 (triazole CH3), 123.34, 123.88, 125.18, 127.97, 130.92, 133.44, 134.78, 135.18, 136.53, 138.41, 138.66, 138.94 (Arm), 143.49 (=C-N, triazole), 154.63 (C9 of quinoline), 158.83 (C2 of quinoline), 194.22 (C(O)OH); Analysis for C19H13BrN4O2, M.wt. (409.24 g/mol), Calcd.: % C, 55.76; H, 3.20; N, 13.69; Actual: % C, 55.60; H, 3.21; N, 13.75.
2-(5-Methyl-1-(4-nitrophenyl)-1H-1,2,3-triazol-4-yl)benzo [b]pyridine-4-carboxylic acid (5h).
Yellow powder; mp 265°C–267°C; yield (67%); 1H NMR (500 MHz, DMSO-d6) δ = 2.62 (s, 3H, triazole CH3), 7.49–7.74 (m, 1H, H-Arm), 7.84–8.00 (m, 3H, H-Arm), 8.10–8.16 (m, 1H, H-Arm), 8.29 (d, 1H, J = 7.5 Hz, H-Arm), 8.44–8.52 (m, 2H, H-Arm), 8.72 (s, 1H, H3 of quinoline), 11.03 (brs, 1H, C(O)OH); 13C NMR (126 MHz, DMSO-d6) δ = 10.12 (triazole CH3), 119.87, 122.75, 123.37, 125.64, 127.05, 130.32, 130.39, 132.40, 137.59, 138.65, 138.89, 140.35 (Arm), 143.71 (=C-N, triazole), 148.53 (CH-NO2-CH), 157.95 (C2 of quinoline), 194.21 (C(O)OH); Analysis for C19H13N5O4, M.wt. (375.34 g/mol), Calcd.: % C, 60.80; H, 3.49; N, 18.66; Actual: % C, 60.81; H, 3.48; N, 18.69.
6-Chloro-2-(5-methyl-1-phenyl-1H-1,2,3-triazol-4-yl)benzo [b]pyridine-4-carboxylic acid (5i).
Yellow powder; mp > 300°C; yield (79%); 1H NMR (500 MHz, DMSO-d6) δ = 2.80 (s, 3H, triazole CH3), 7.61–7.69 (m, 5H, H-Arm), 7.82 (d, 1H, J = 9.0 Hz, H-Arm), 8.09 (d, 1H, J = 8.5 Hz, H-Arm), 8.79 (s, 1H, H3 of quinoline), 8.82 (brs, 1H, H5 of quinoline), 14.17 (brs, 1H, C(O)OH); 13C NMR (126 MHz, DMSO-d6) δ = 10.97 (triazole CH3), 121.56, 124.71, 125.05, 126.00, 130.27, 130.49, 131.19, 131.98, 132.93, 135.27, 135.99, 136.09, 142.16 (=C-N, triazole), 147.29 (C9 of quinoline), 152.40 (C2 of quinoline), 167.34 (C(O)OH); Analysis for C19H13ClN4O2, M.wt. (364.78 g/mol), Calcd.: % C, 62.56; H, 3.59; N, 15.36; Actual: % C, 62.67; H, 3.60; N, 15.30.
6-Chloro-2-(1-(4-methoxyphenyl)-5-methyl-1H-1,2,3-triazol-4-yl)benzo [b]pyridine-4-carboxylic acid (5j).
White powder; mp 287°C–289°C; yield (54%); 1H NMR (500 MHz, DMSO-d6) δ = 2.73 (s, 3H, triazole CH3), 3.83 (s, 3H, OCH3), 7.15 (d, 2H, J = 8.5 Hz, H-Arm), 7.56 (d, 2H, J = 8.5 Hz, H-Arm), 7.77 (d, 1H, J = 9.0 Hz, H-Arm), 8.01 (dd, 1H, J = 3.5 and 8.5 Hz, H7 of quinoline), 7.74 (d, 1H, J = 3.5 Hz, H5 of quinoline); 8.78 (s, 1H, H3 of quinoline); 11.11 (brs, 1H, C(O)OH); 13C NMR (126 MHz, DMSO-d6) δ = 10.90 (triazole CH3), 56.17 (OCH3), 114.37, 115.30, 119.62, 121.52, 124.62, 127.47, 131.09, 131.91, 132.84, 135.31, 137.78, 141.91 (=C-N, triazole), 149.77 (C9 of quinoline), 159.63 (C2 of quinoline), 160.67, 167.32 (C(O)OH); Analysis for C20H15ClN4O3, M.wt. (394.81 g/mol), Calcd.: % C, 60.84; H, 3.83; N, 14.19; Actual: % C, 60.90; H, 3.85; N, 14.15.
6-Chloro-2-(1-(3-chlorophenyl)-5-methyl-1H-1,2,3-triazol-4-yl)benzo [b]pyridine-4-carboxylic acid (5k).
Yellow powder; mp > 300°C; yield (62%); 1H NMR (500 MHz, DMSO-d6) δ = 2.79 (s, 3H, triazole CH3), 7.66-7.69 (m, 3H, H-Arm), 7.78 (d, 1H, J = 9.0 Hz, H-Arm), 7.84 (s, 1H, H-Arm), 8.03 (d, 1H, J = 9.0 Hz, H-Arm), 8.73 (s, 1H, H3 of quinoline); 8.78 (brs, 1H, H5 of quinoline); 14.10 (brs, 1H, C(O)OH); 13C NMR (126 MHz, DMSO-d6) δ = 10.97 (triazole CH3), 121.56, 124.71, 125.05, 126.00, 130.27, 130.49, 131.19, 131.98, 132.93, 135.27, 135.99, 136.09, 142.16 (=C-N, triazole), 147.29 (C9 of quinoline), 152.40 (C2 of quinoline), 167.34 (C(O)OH); Analysis for C19H12Cl2N4O2, M.wt. (399.23 g/mol), Calcd.: % C, 57.16; H, 3.03; N, 14.03; Actual: % C, 57.29; H, 3.03; N, 13.99.
2-(1-(3-Bromophenyl)-5-methyl-1H-1,2,3-triazol-4-yl)-6-chlorobenzo [b]pyridine-4-carboxylic acid (5L).
Buff powder; mp 248°C–250°C; yield (71%); 1H NMR (500 MHz, DMSO-d6) δ = 2.80 (s, 3H, triazole CH3), 7.55-7.63 (m, 1H, H-Arm), 7.71 (d, 1H, J = 9.0 Hz, H-Arm), 7.80–7.84 (m, 2H, H-Arm); 7.97–7.98 (m, 1H, H-Arm); 8.06 (d, 1H, J = 9.0 Hz, H-Arm), 8.76 (s, 1H, H3 of quinoline), 8.78 (d, 1H, J = 2.0 Hz, H-Arm), 14.10 (brs, 1H, C(O)OH); 13C NMR (126 MHz, DMSO-d6) δ = 10.86 (triazole CH3), 121.46, 122.64, 124.59, 124.90, 125.07, 125.15, 128.61, 131.04, 131.85, 132.11, 132.93, 133.63, 135.36, 137.20, 142.06 (=C-N, triazole), 147.07 (C9 of quinoline), 151.99 (C2 of quinoline), 167.23 (C(O)OH); Analysis for C19H12BrClN4O2, M.wt. (443.68 g/mol), Calcd.: % C, 51.43; H, 2.73; N, 12.63; Actual: % C, 51.25; H, 2.74; N, 12.67.
6-Chloro-2-(1-(4-fluorophenyl)-5-methyl-1H-1,2,3-triazol-4-yl)benzo [b]pyridine-4-carboxylic acid (5m).
Yellow powder; mp 298°C–299°C; yield (64%); 1H NMR (500 MHz, DMSO-d6) δ = 2.77 (s, 3H, triazole CH3), 7.48–7.51 (m, 2H, H-Arm), 7.74–7.76 (m, 2H, H-Arm), 7.79 (dd, 1H, J = 2.0 and 9.0 Hz, H-Arm), 8.04 (d, 1H, J = 9.0 Hz, H-Arm), 8.74 (s, 1H, H3 of quinoline), 8.79 (d, 1H, J = 2.0 Hz, H-Arm), 14.13 (brs, 1H, C(O)OH); 13C NMR (126 MHz, DMSO-d6) δ = 10.85 (triazole CH3), 117.10, 117.29, 121.48, 124.63, 124.96, 128.38, 128.45, 131.01, 131.84, 132.87, 135.39, 135.66, 142.01 (=C-N, triazole), 147.14 (C9 of quinoline), 152.19 (C2 of quinoline), 162.02 (C-F), 167.26 (C(O)OH); Analysis for C19H12ClFN4O2, M.wt. (382.77 g/mol), Calcd.: % C, 59.62; H, 3.16; N, 14.64; Actual: % C, 59.54; H, 3.17; N, 14.67.
6-Chloro-2-(1-(4-chlorophenyl)-5-methyl-1H-1,2,3-triazol-4-yl)benzo [b]pyridine-4-carboxylic acid (5n).
Pale yellow powder; mp > 300°C; yield (48%); 1H NMR (500 MHz, DMSO-d6) δ = 2.78 (s, 3H, triazole CH3), 7.72-7.73 (m, 4H, H-Arm), 7.79–7.82 (m, 1H, H-Arm), 8.04 (dd, 1H, J = 3.5 and 9.0 Hz, H-Arm), 8.74 (d, 1H, J = 3.5 Hz, H-Arm), 8.79 (brs, 1H, H3 of quinoline), 14.13 (brs, 1H, C(O)OH); 13C NMR (126 MHz, DMSO-d6) δ = 10.92 (triazole CH3), 121.51, 124.71, 125.04, 127.78, 130.31, 131.16, 131.96, 132.94, 134.90, 135.14, 135.43, 135.98, 142.22 (=C-N, triazole), 147.24 (C9 of quinoline), 152.24 (C2 of quinoline), 167.31 (C(O)OH); Analysis for C19H12Cl2N4O2, M.wt. (399.23 g/mol), Calcd.: % C, 57.16; H, 3.03; N, 14.03; Actual: % C, 57.13; H, 3.04; N, 14.01.
2-(1-(4-Bromophenyl)-5-methyl-1H-1,2,3-triazol-4-yl)-6-chlorobenzo [b]pyridine-4-carboxylic acid (5o).
White powder; mp > 300°C; yield (66%); 1H NMR (500 MHz, DMSO-d6) δ = 2.77 (s, 3H, triazole CH3), 7.64 (d, 2H, J = 8.5 Hz, H-Arm), 7.77 (dd, 1H, J = 2.0 and 9.0 Hz, H-Arm), 7.84 (d, 2H, J = 8.0 Hz, H-Arm), 8.02 (d, 1H, J = 8.5 Hz, H-Arm), 8.73 (brs, 1H, H3 of quinoline), 8.78 (d, 1H, J = 1.5 Hz, H-Arm), 14.10 (brs, 1H, C(O)OH), 13C NMR (126 MHz, DMSO-d6) δ = 10.91 (triazole CH3), 121.51, 123.66, 124.67, 125.00, 127.94, 131.08, 131.90, 132.93, 133.24, 135.30, 135.32, 135.78, 142.21 (=C-N, triazole), 147.18 (C9 of quinoline), 152.17 (C2 of quinoline), 167.26 (C(O)OH). Analysis for C19H12BrClN4O2, M.wt. (443.68 g/mol), Calcd.: % C, 51.43; H, 2.73; N, 12.63; Actual: % C, 51.45; H, 2.73; N, 12.60.
6-Chloro-2-(5-methyl-1-(4-nitrophenyl)-1H-1,2,3-triazol-4-yl)benzo [b]pyridine-4-carboxylic acid (5p).
Orange powder; mp 235°C–237°C; yield (47%); 1H NMR (500 MHz, DMSO-d6) δ = 2.90 (s, 3H, triazole CH3), 6.88-6.90 (m, 3H, H-Arm), 7.52 (s, 1H, H-Arm), 7.57-7.59 (m, 1H, H-Arm), 7.86-8.16 (m, 2H, H-Arm), 8.44-8.50 (m, 1H, H-Arm), 11.10 (brs, 1H, C(O)OH); Analysis for C19H12ClN5O4, M.wt. (409.78 g/mol), Calcd.: % C, 55.69; H, 2.95; N, 17.09; Actual: % C, 55.79; H, 2.94; N, 17.04.
6-Bromo-2-(5-methyl-1-phenyl-1H-1,2,3-triazol-4-yl)benzo [b]pyridine-4-carboxylic acid (5q).
Yellow powder; mp > 300°C; yield (74%); 1H NMR (500 MHz, DMSO-d6) δ = 2.77 (s, 3H, triazole CH3), 7.60-7.68 (m, 5H, H-Arm), 7.89 (dd, 1H, J = 2.0 and 9.0 Hz, H-Arm), 7.95 (d, 1H, J = 9.0 Hz, H-Arm), 8.74 (s, 1H, H3 of quinoline), 8.95 (s, 1H, H5 of quinoline), 14.11 (brs, 1H, C(O)OH); 13C NMR (126 MHz, DMSO-d6) δ = 11.39 (triazole CH3), 121.49, 121.67, 125.12, 125.97, 128.26, 130.28, 130.46, 131.98, 133.64, 135.22, 135.67, 136.08, 142.13 (=C-N, triazole), 147.36 (C9 of quinoline), 152.40 (C2 of quinoline), 167.29 (C(O)OH); Analysis for C19H13BrN4O2, M.wt. (409.24 g/mol), Calcd.: % C, 55.76; H, 3.20; N, 13.69; Actual: % C, 55.69; H, 3.21; N, 13.66.
6-Bromo-2-(1-(4-methoxyphenyl)-5-methyl-1H-1,2,3-triazol-4-yl)benzo [b]pyridine-4-carboxylic acid (5r).
Yellow powder; mp 294°C–295°C; yield (53%); 1H NMR (500 MHz, DMSO-d6) δ = 2.74 (s, 3H, triazole CH3), 3.84 (s, 3H, OCH3), 7.15 (d, 2H, J = 9.5 Hz, H-Arm), 7.57 (d, 2H, J = 9.0 Hz, H-Arm), 7.90 (dd, 1H, J = 2.5 and 9.5 Hz, H-Arm), 7.97 (d, 1H, J = 9.5 Hz, H-Arm); 8.75 (s, 1H, H3 of quinoline), 8.96 (d, 1H, J = 2.5 Hz, H5 of quinoline), 14.13 (s, 1H, C(O)OH); 13C NMR (126 MHz, DMSO-d6) δ = 10.90 (triazole CH3), 56.17 (OCH3), 115.30, 121.48, 125.10, 127.46, 128.23, 128.90, 131.95, 133.63, 135.07, 135.31, 135.67, 141.89 (=C-N, triazole), 147.38 (C9 of quinoline), 152.49 (C2 of quinoline), 160.67, 167.29 (C(O)OH); Analysis for C20H15BrN4O3, M.wt. (439.26 g/mol), Calcd.: % C, 54.69; H, 3.44; N, 12.75; Actual: % C, 54.76; H, 3.43; N, 12.70.
6-Bromo-2-(1-(3-chlorophenyl)-5-methyl-1H-1,2,3-triazol-4-yl)benzo [b]pyridine-4-carboxylic acid (5s).
Yellow powder; mp > 300°C; yield (67%); 1H NMR (500 MHz, DMSO-d6) δ = 2.79 (s, 3H, triazole CH3), 7.63-7.71 (m, 3H, H-Arm), 7.84-7.85 (m, 1H, H-Arm), 7.89 (dd, 1H, J = 2.0 and 9.0 Hz, H-Arm), 7.96 (d, 1H, J = 9.0 Hz, H-Arm), 8.73 (s, 1H, H3 of quinoline); 8.95 (s, 1H, H5 of quinoline), 14.14 (brs, 1H, C(O)OH); 13C NMR (126 MHz, DMSO-d6) δ = 10.90 (triazole CH3), 121.45, 124.79, 125.92, 128.22, 130.51, 131.89, 131.97, 133.67, 134.48, 135.48, 135.71, 137.20, 142.18 (=C-N, triazole), 147.34 (C9 of quinoline), 152.21 (C2 of quinoline), 167.25 (C(O)OH); Analysis for C19H12BrClN4O2, M.wt. (443.69 g/mol), Calcd.: % C, 51.43; H, 2.73; N, 12.63; Actual: % C, 51.57; H, 2.74; N, 12.58.
6-Bromo-2-(1-(3-bromophenyl)-5-methyl-1H-1,2,3-triazol-4-yl)benzo [b]pyridine-4-carboxylic acid (5t).
Yellow powder; mp 299°C–300°C; yield (71%); 1H NMR (500 MHz, DMSO-d6) δ = 2.80 (s, 3H, triazole CH3), 7.59-7.64 (m, 1H, H-Arm); 7.72-7.74 (m, 1H, H-Arm), 7.83-7.85 (m, 1H, H-Arm), 7.92-7.94 (m, 1H, H-Arm), 7.97-8.02 (m, 2H, H3 of quinoline and H-Arm), 8.75 (d, 1H, J = 5.0 Hz, H-Arm), 8.97 (d, 1H, J = 3.0 Hz, H5 of quinoline), 14.19 (brs, 1H, C(O)OH); 13C NMR (126 MHz, DMSO-d6) δ = 10.91 (triazole CH3), 121.46, 121.70, 122.65, 125.15, 128.22, 128.69, 131.95, 132.10, 133.41, 133.64, 135.47, 135.64, 137.29, 142.15 (=C-N, triazole), 147.32 (C9 of quinoline), 152.20 (C2 of quinoline), 167.25 (C(O)OH); Analysis for C19H12Br2N4O2, M.wt. (488.14 g/mol), Calcd.: % C, 46.75; H, 2.48; N, 11.48; Actual: % C, 46.86; H, 2.47; N, 11.45.
6-Bromo-2-(1-(4-fluorophenyl)-5-methyl-1H-1,2,3-triazol-4-yl)benzo [b]pyridine-4-carboxylic acid (5u).
Yellow powder; mp > 300°C; yield (42%); 1H NMR (500 MHz, DMSO-d6) δ = 2.77 (s, 3H, triazole CH3), 7.45-7.52 (m, 2H, H-Arm), 7.66-7.70 (m, 1H, H-Arm), 7.74-7.76 (m, 1H, H-Arm), 7.90 (dd, 1H, J = 2.0 and 9.0 Hz, H-Arm), 7.97 (d, 1H, J = 9.0 Hz, H-Arm), 8.74 (s, 1H, H3 of quinoline), 8.96 (d, 1H, J = 2.5 Hz, H5 of quinoline), 14.13 (s, 1H, C(O)OH); 13C NMR (126 MHz, DMSO-d6) δ = 10.68 (triazole CH3), 114.79, 115.03, 117.04, 117.23, 119.62, 121.21, 121.62, 127.33, 128.19, 131.68, 132.19, 135.21, 140.70, 141.87 (=C-N, triazole), 146.98 (C9 of quinoline), 151.82 (C2 of quinoline), 167.01 (C(O)OH); Analysis for C19H12BrFN4O2, M.wt. (427.22 g/mol), Calcd.: % C, 53.42; H, 2.83; N, 13.11; Actual: % C, 53.43; H, 2.84; N, 13.08.
6-Bromo-2-(1-(4-chlorophenyl)-5-methyl-1H-1,2,3-triazol-4-yl)benzo [b]pyridine-4-carboxylic acid (5v).
Yellow powder; mp > 300°C; yield (57%); 1H NMR (500 MHz, DMSO-d6) δ = 2.78 (s, 3H, triazole CH3), 7.65-7.73 (m, 4H, H-Arm); 7.89-7.92 (m, 1H, H-Arm), 7.97 (dd, 1H, J = 3.5 and 9.0 Hz, H-Arm), 8.73 (d, 1H, J = 4.0 Hz, H-Arm), 8.96 (d, 1H, J = 1.5 Hz, H5 of quinoline), 14.15 (brs, 1H, C(O)OH); 13C NMR (126 MHz, DMSO-d6) δ = 10.92 (triazole CH3), 121.45, 121.67, 125.12, 127.72, 128.22, 130.29, 131.94, 133.63, 134.88, 135.12, 135.38, 135.67, 142.20 (=C-N, triazole), 147.32 (C9 of quinoline), 152.24 (C2 of quinoline), 167.26 (C(O)OH); Analysis for C19H12BrClN4O2, M.wt. (443.69 g/mol), Calcd.: % C, 51.43; H, 2.73; N, 12.63; Actual: % C, 51.61; H, 2.72; N, 12.59.
6-Bromo-2-(1-(4-bromophenyl)-5-methyl-1H-1,2,3-triazol-4-yl)benzo [b]pyridine-4-carboxylic acid (5w).
Yellow powder; mp > 300°C, yield (60%); 1H NMR (500 MHz, DMSO-d6) δ = 2.77 (s, 3H, triazole CH3), 7.64 (d, 2H, J = 8.5 Hz, H-Arm), 7.84 (d, 2H, J = 8.5 Hz, H-Arm), 7.88 (dd, 1H, J = 2.0 and 9.0 Hz, H-Arm), 7.95 (d, 1H, J = 9.0 Hz, H-Arm), 8.72 (s, 1H, H3 of quinoline), 8.94 (d, 1H, J = 2.0 Hz, H5 of quinoline), 14.12 (s, 1H, C(O)OH); 13C NMR (126 MHz, DMSO-d6) δ = 10.93 (triazole CH3), 121.46, 121.68, 123.65, 125.12, 127.93, 128.22, 131.95, 133.24, 133.64, 135.29, 135.35, 135.67, 142.22 (=C-N, triazole), 147.33 (C9 of quinoline), 152.23 (C2 of quinoline), 167.25 (C(O)OH); Analysis for C19H12Br2N4O2, M.wt. (488.14 g/mol), Calcd.: % C, 46.75; H, 2.48; N, 11.48; Actual: % C, 46.56; H, 2.49; N, 11.51.
6-Bromo-2-(5-methyl-1-(4-nitrophenyl)-1H-1,2,3-triazol-4-yl)benzo [b]pyridine-4-carboxylic acid (5x).
Red powder; mp 236°C–238°C; yield (71%); 1H NMR (500 MHz, DMSO-d6) δ = 2.86 (s, 3H, triazole CH3), 6.82 (d, 1H, J = 8.0 Hz, H-Arm), 7.58 (s, 1H, H-Arm), 7.67 (d, 1H, J = 8.5 Hz, H-Arm), 7.94-8.02 (m, 3H, H-Arm), 8.45 (d, 1H, J = 8.5 Hz, H-Arm), 8.72 (s, 1H, H3 of quinoline), 11.13 (s, 1H, C(O)OH); 13C NMR (126 MHz, DMSO-d6) δ = 11.10 (triazole CH3), 114.83, 120.03, 121.84, 125.63, 126.90, 127.02, 127.39, 128.27, 132.05, 133.78, 135.68, 135.87, 140.58, 150.14 (C-NO2), 159.44 (C2 of quinoline), 167.24 (C(O)OH); Analysis for C19H12BrN5O4, M.wt. (454.24 g/mol), Calcd.: % C, 50.24; H, 2.66; N, 15.42; Actual: % C, 50.27; H, 2.65; N, 15.47.
4.2 Biological evaluations
The experimental procedures for the antimycobacterial (Franzblau et al., 1998) and MTB InhA inhibition (He et al., 2007) biological experiments were conducted using the reported protocols (Supplementary Material).
4.3 In silico studies
In silico studies involved molecular docking and molecular dynamics (MD) simulations using AutoDock Vina v1.2.3 (Eberhardt et al., 2021) and GROMACS v2023 (Van Der Spoel et al., 2005), respectively. The methods employed for the preparation of the tested compounds and InhA protein (PDB ID: 4TZK (He et al., 2006)) in both simulations and all the subsequent steps are elaborated in detail in the Supplementary Material section. Additionally, drug-likeness analyses and ADMET predictions were made using SwissADME (Daina et al., 2017) and pkCSM (Pires et al., 2015), and toxicity assessments via ProTox II (Banerjee et al., 2018b), following established protocols for thorough evaluation (Supplementary Material).
Data availability statement
The original contributions presented in the study are included in the article/supplementary materials; further inquiries can be directed to the corresponding authors.
Author contributions
AS: Conceptualization, Validation, Writing–review and editing. M-HA: Conceptualization, Writing–review and editing, Funding acquisition. ME: Writing–review and editing, Investigation, Methodology. JP: Investigation, Methodology, Writing–review and editing, Formal Analysis. HA: Methodology, Writing–review and editing, Data curation. NS: Methodology, Writing–review and editing, Software. NX: Methodology, Writing–review and editing, Formal Analysis. M-AV: Data curation, Writing–original draft. TT: Data curation, Writing–original draft, Funding acquisition. AE: Data curation, Writing–original draft, Formal Analysis, Validation. BD: Formal Analysis, Validation, Investigation, Resources, Writing–review and editing. JD: Resources, Validation, Writing–review and editing, Conceptualization. WE: Conceptualization, Resources, Writing–review and editing, Supervision, Visualization.
Funding
The author(s) declare that financial support was received for the research, authorship, and/or publication of this article. The authors extend their appreciation to the Deanship of Scientific Research, King Saud University, for funding through the Vice Deanship of Scientific Research Chairs, Research Chair of Colorectal Surgery. JP & JD were supported by the Ministry of Science and Higher Education, POL-OPENSCREEN, DIR/WK/2018/06 and National Science Centre, Poland UMO-2023/49/B/NZ7/01421.
Conflict of interest
The authors declare that the research was conducted in the absence of any commercial or financial relationships that could be construed as a potential conflict of interest.
The author(s) declared that they were an editorial board member of Frontiers, at the time of submission. This had no impact on the peer review process and the final decision.
Publisher’s note
All claims expressed in this article are solely those of the authors and do not necessarily represent those of their affiliated organizations, or those of the publisher, the editors and the reviewers. Any product that may be evaluated in this article, or claim that may be made by its manufacturer, is not guaranteed or endorsed by the publisher.
Supplementary material
The Supplementary Material for this article can be found online at: https://www.frontiersin.org/articles/10.3389/fchem.2024.1424017/full#supplementary-material
References
Abdelrahman, M. A., Almahli, H., Al-Warhi, T., Majrashi, T. A., Abdel-Aziz, M. M., Eldehna, W. M., et al. (2022). Development of novel isatin-tethered quinolines as anti-tubercular agents against multi and extensively drug-resistant mycobacterium tuberculosis. Molecules 27 (24), 8807. doi:10.3390/molecules27248807
Alcaraz, M., Sharma, B., Roquet-Baneres, F., Conde, C., Cochard, T., Biet, F., et al. (2022). Designing quinoline-isoniazid hybrids as potent anti-tubercular agents inhibiting mycolic acid biosynthesis. Eur. J. Med. Chem. 239, 114531. doi:10.1016/j.ejmech.2022.114531
Almeida Da Silva, P. E., and Palomino, J. C. (2011). Molecular basis and mechanisms of drug resistance in Mycobacterium tuberculosis: classical and new drugs. J. Antimicrob. Chemother. 66 (7), 1417–1430. doi:10.1093/jac/dkr173
Azab, A. E., Alesawy, M. S., Eldehna, W. M., Elwan, A., and Eissa, I. H. (2022). New [1, 2, 4] triazolo [4, 3-c] quinazoline derivatives as vascular endothelial growth factor receptor-2 inhibitors and apoptosis inducers: design, synthesis, docking, and antiproliferative evaluation. Arch. Pharm. 355 (10), 2200133. doi:10.1002/ardp.202200133
Banerjee, P., Eckert, A. O., Schrey, A. K., and Preissner, R. (2018a). ProTox-II: a webserver for the prediction of toxicity of chemicals. Nucleic acids Res. 46 (W1), W257–W263. doi:10.1093/nar/gky318
Banerjee, P., Eckert, A. O., Schrey, A. K., and Preissner, R. (2018b). ProTox-II: a webserver for the prediction of toxicity of chemicals. Nucleic acids Res. 46 (W1), W257–W263. doi:10.1093/nar/gky318
Barberis, I., Bragazzi, N. L., Galluzzo, L., and Martini, M. (2017). The history of tuberculosis: from the first historical records to the isolation of Koch’s bacillus. J. Prev. Med. Hyg. 58, E9–E12. doi:10.15167/2421-4248/jpmh2017.58.1.728
Bekheit, M. S., Mohamed, H. A., Abdel-Wahab, B. F., and Fouad, M. A. (2021). Design and synthesis of new 1, 4, 5-trisubstituted triazole-bearing benzenesulphonamide moiety as selective COX-2 inhibitors. Med. Chem. Res. 30, 1125–1138. doi:10.1007/s00044-021-02716-7
Bekier, A., Kawka, M., Lach, J., Dziadek, J., Paneth, A., Gatkowska, J., et al. (2021). Imidazole-thiosemicarbazide derivatives as potent anti-Mycobacterium tuberculosis compounds with antibiofilm activity. Cells 10 (12), 3476. doi:10.3390/cells10123476
Boechat, N., Ferreira, V. F., Ferreira, S. B., Ferreira, M. L. G., da Silva, F. C., Bastos, M. M., et al. (2011). Novel 1,2,3-triazole derivatives for use against Mycobacterium tuberculosis H37Rv (ATCC 27294) strain. J. Med. Chem. 54, 5988–5999. doi:10.1021/jm2003624
Dadlani, V. G., Chhabhaiya, H., Somani, R. R., and Tripathi, P. K. (2022). Synthesis, molecular docking, and biological evaluation of novel 1,2,4-triazole-isatin derivatives as potential Mycobacterium tuberculosis shikimate kinase inhibitors. Chem. Biol. Drug Des. 100 (2), 230–244. doi:10.1111/cbdd.14060
Daina, A., Michielin, O., and Zoete, V. (2017). SwissADME: a free web tool to evaluate pharmacokinetics, drug-likeness and medicinal chemistry friendliness of small molecules. Sci. Rep. 7 (1), 42717. doi:10.1038/srep42717
Daley, C. L. (2019). The global fight against tuberculosis. Surg. Clin. 29, 19–25. doi:10.1016/j.thorsurg.2018.09.010
Dessen, A., Quemard, A., Blanchard, J. S., Jacobs, Jr W. R., and Sacchettin, J. C. (1995). Crystal structure and function of the isoniazid target of Mycobacterium tuberculosis. Science 267 (5204), 1638–1641. doi:10.1126/science.7886450
Dhameliya, T. M., Vekariya, D. D., Patel, H. Y., and Patel, J. T. (2023). Comprehensive coverage on anti-mycobacterial endeavour reported during 2022. Eur. J. Med. Chem. 255, 115409. doi:10.1016/j.ejmech.2023.115409
Dong, H. S., Wang, H. C., Gao, Z. L., Li, R. S., and Cui, F. H. (2010). Tandem Michael addition/imino-nitrile cyclization synthesis of 2-amino-6-(1-aryl-5-methyl-1H-1,2,3-triazol-4yl)-4-phenylpyridine-3-carbonitrile. J. Heterocycl. Chem. 47, 389–395. doi:10.1002/jhet.336
Eberhardt, J., Santos-Martins, D., Tillack, A. F., and Forli, S. (2021). AutoDock Vina 1.2. 0: new docking methods, expanded force field, and python bindings. J. Chem. Inf. Model. 61 (8), 3891–3898. doi:10.1021/acs.jcim.1c00203
Elbadawi, M. M., Eldehna, W. M., El-Hafeez, A. A. A., Somaa, W. R., Albohy, A., Al-Rashood, S. T., et al. (2022). 2-Arylquinolines as novel anticancer agents with dual EGFR/FAK kinase inhibitory activity: synthesis, biological evaluation, and molecular modelling insights. J. Enzyme Inhibition Med. Chem. 37 (1), 355–378. doi:10.1080/14756366.2021.2015344
Elghamry, I., and Al-Faiyz, Y. (2016). A simple one-pot synthesis of quinoline-4-carboxylic acids by the Pfitzinger reaction of isatin with enaminones in water. Tetrahedron Lett. 57, 110–112. doi:10.1016/j.tetlet.2015.11.070
Elkaeed, E. B., Taghour, M. S., Mahdy, H. A., Eldehna, W. M., El-Deeb, N. M., Kenawy, A. M., et al. (2022). New quinoline and isatin derivatives as apoptotic VEGFR-2 inhibitors: design, synthesis, anti-proliferative activity, docking, ADMET, toxicity, and MD simulation studies. J. Enzyme Inhibition Med. Chem. 37 (1), 2191–2205. doi:10.1080/14756366.2022.2110869
Elsawi, A. E., Elbadawi, M. M., Nocentini, A., Almahli, H., Giovannuzzi, S., Shaldam, M., et al. (2023). 1, 5-diaryl-1, 2, 4-triazole ureas as new SLC-0111 analogues endowed with dual carbonic anhydrase and VEGFR-2 inhibitory activities. J. Med. Chem. 66 (15), 10558–10578. doi:10.1021/acs.jmedchem.3c00721
Elsawi, A. E., Shahin, M. I., Elbendary, H. A., Al-Warhi, T., Hassan, F. E., and Eldehna, W. M. (2024). 1, 2, 4-triazole-tethered indolinones as new cancer-fighting small molecules targeting VEGFR-2: synthesis, biological evaluations and molecular docking. Pharmaceuticals 17 (1), 81. doi:10.3390/ph17010081
El-Shoukrofy, M. S., Atta, A., Fahmy, S., Sriram, D., Mahran, M. A., and Labouta, I. M. (2023). New tetrahydropyrimidine-1, 2, 3-triazole clubbed compounds: antitubercular activity and Thymidine Monophosphate Kinase (TMPKmt) inhibition. Bioorg. Chem. 131, 106312. doi:10.1016/j.bioorg.2022.106312
Esmail, H., Barry, C. E., Young, D. B., and Wilkinson, R. J. (2014). The ongoing challenge of latent tuberculosis. Philos. Trans. R. Soc. Lond. B Biol. Sci. 369, 20130437. doi:10.1098/rstb.2013.0437
Franzblau, S. G., Witzig, R. S., McLaughlin, J. C., Torres, P., Madico, G., Hernandez, A., et al. (1998). Rapid, low-technology MIC determination with clinical Mycobacterium tuberculosis isolates by using the microplate Alamar Blue assay. J. Clin. Microbiol. 36 (2), 362–366. doi:10.1128/jcm.36.2.362-366.1998
Gill, C., Jadhav, G., Shaikh, M., Kale, R., Ghawalkar, A., Nagargoje, D., et al. (2008). Clubbed [1,2,3] triazoles by fluorine benzimidazole: a novel approach to H37Rv inhibitors as a potential treatment for tuberculosis. Bioorg. Med. Chem. Lett. 18 (23), 6244–6247. doi:10.1016/j.bmcl.2008.09.096
He, X., Alian, A., and Ortiz de Montellano, P. R. (2007). Inhibition of the Mycobacterium tuberculosis enoyl acyl carrier protein reductase InhA by arylamides. Bioorg Med. Chem. 15 (21), 6649–6658. doi:10.1016/j.bmc.2007.08.013
He, X., Alian, A., Stroud, R., and Ortiz de Montellano, P. R. (2006). Pyrrolidine carboxamides as a novel class of inhibitors of enoyl acyl carrier protein reductase from Mycobacterium tuberculosis. J. Med. Chem. 49 (21), 6308–6323. doi:10.1021/jm060715y
Jackson, M. (2014). The mycobacterial cell envelope-lipids. Cold Spring Harb. Perspect. Med. 4 (10), a021105. doi:10.1101/cshperspect.a021105
Johansen, M. D., Herrmann, J. L., and Kremer, L. (2020). Non-tuberculous mycobacteria and the rise of Mycobacterium abscessus. Nat. Rev. Microbiol. 18 (7), 392–407. doi:10.1038/s41579-020-0331-1
Keri, R. S., and Patil, S. A. (2014). Quinoline: a promising antitubercular target. Biomed. Pharmacother. 68, 1161–1175. doi:10.1016/j.biopha.2014.10.007
Khaleel, E. F., Ahmed, S., Korycka-Machala, M., Mustafa Badi, R., Son, N. T., Xuan Ha, N., et al. (2024). Identification of new anti-mycobacterial agents based on quinoline-isatin hybrids targeting enoyl acyl carrier protein reductase (InhA). Bioorg. Chem. 144, 107138. doi:10.1016/j.bioorg.2024.107138
Kufareva, I., and Abagyan, R. (2012). Methods of protein structure comparison. Methods Mol. Biol. 857, 231–257. doi:10.1007/978-1-61779-588-6_10
Kumar Sahoo, S., Naiyaz Ahmad, M., Kaul, G., Nanduri, S., Dasgupta, A., Chopra, S., et al. (2022). Exploration of isoxazole-carboxylic acid methyl ester based 2-substituted quinoline derivatives as promising antitubercular agents. Chem. Biodivers. 19 (7), e202200324. doi:10.1002/cbdv.202200324
Li, J., and Zhang, J. (2022). The antibacterial activity of 1, 2, 3-triazole-and 1, 2, 4-triazole-containing hybrids against Staphylococcus aureus: an updated review (2020-present). Curr. Top. Med. Chem. 22 (1), 41–63. doi:10.2174/1568026621666211111160332
Lipinski, C. A., Lombardo, F., Dominy, B. W., and Feeney, P. J. (1997). Experimental and computational approaches to estimate solubility and permeability in drug discovery and development settings. Adv. Drug Deliv. Rev. 23 (1–3), 3–25. doi:10.1016/S0169-409X(96)00423-1
Lu, H., and Tonge, P. J. (2008). Inhibitors of FabI, an enzyme drug target in the bacterial fatty acid biosynthesis pathway. Acc. Chem. Res. 41 (1), 11–20. doi:10.1021/ar700156e
Marrakchi, H., Lanéelle, G., and Ak, Q. (2000). InhA, a target of the antituberculous drug isoniazid, is involved in a mycobacterial fatty acid elongation system, FAS-II. Microbiology 146 (2), 289–296. doi:10.1099/00221287-146-2-289
Mohamede, A. S., Elneairy, M. A., and Eldine, S. M. (2015). 2, 4-Cycloaddition reactions: preparation and cytotoxicity of novel quinoline and pyrrolo [3, 4-f] quinoline derivatives. Int. J. Pharm. Pharm. Sci. 7 (12), 64–68.
Munir, A., Wilson, M. T., Hardwick, S. W., Chirgadze, D. Y., Worrall, J. A. R., Blundell, T. L., et al. (2021). Using cryo-EM to understand antimycobacterial resistance in the catalase-peroxidase (KatG) from Mycobacterium tuberculosis. Structure 29 (8), 899–912.e4. doi:10.1016/j.str.2020.12.008
Muthaiah, M., Shivekar, S. S., Cuppusamy Kapalamurthy, V. R., Alagappan, C., Sakkaravarthy, A., and Brammachary, U. (2017). Prevalence of mutations in genes associated with rifampicin and isoniazid resistance in Mycobacterium tuberculosis clinical isolates. J. Clin. Tuberc. Other Mycobact. Dis. 8, 19–25. doi:10.1016/j.jctube.2017.06.001
Nyoni, N. T., Ncube, N. B., Kubheka, M. X., Mkhwanazi, N. P., Senzani, S., Singh, T., et al. (2023). Synthesis, characterization, in vitro antimycobacterial and cytotoxicity evaluation, DFT calculations, molecular docking and ADME studies of new isomeric benzimidazole-1, 2, 3-triazole-quinoline hybrid mixtures. Bioorg. Chem. 141, 106904. doi:10.1016/j.bioorg.2023.106904
Pires, D. E., Blundell, T. L., and Ascher, D. B. (2015). pkCSM: predicting small-molecule pharmacokinetic and toxicity properties using graph-based signatures. J. Med. Chem. 58 (9), 4066–4072. doi:10.1021/acs.jmedchem.5b00104
Pokhodylo, N. T., Savka, R. D., Matiichuk, V. S., and Obushak, N. D. (2009). Synthesis and selected transformations of 1-(5-methyl-1-aryl-1 H-1, 2, 3-triazol-4-yl) ethanones and 1-[4-(4-R-5-methyl-1 H-1, 2, 3-triazol-1-yl) phenyl] ethanones. Russ. J. General Chem. 79, 309–314. doi:10.1134/s1070363209020248
Pym, A. S., Diacon, A. H., Tang, S.-J., Conradie, F., Danilovits, M., Chuchottaworn, C., et al. (2016). Bedaquiline in the treatment of multidrug- and extensively drug-resistant tuberculosis. Eur. Respir. J. 47 (2), 564–574. doi:10.1183/13993003.00724-2015
Quimque, M. T., Go, A. D., Lim, J. A., Vidar, W. S., and Macabeo, A. P. (2023). Mycobacterium tuberculosis inhibitors based on arylated quinoline carboxylic acid backbones with anti-mtb gyrase activity. Int. J. Mol. Sci. 24 (14), 11632. doi:10.3390/ijms241411632
Ravimohan, S., Kornfeld, H., Weissman, D., and Bisson, G. P. (2018). Tuberculosis and lung damage: from epidemiology to pathophysiology. Eur. Respir. Rev. 27 (147), 170077. doi:10.1183/16000617.0077-2017
Rožman, K., Sosič, I., Fernandez, R., Young, R. J., Mendoza, A., Gobec, S., et al. (2017). A new ‘golden age’for the antitubercular target InhA. Drug Discov. Today 22 (3), 492–502. doi:10.1016/j.drudis.2016.09.009
Sabt, A., Abdelraof, M., Hamissa, M. F., and Noamaan, M. A. (2023b). Antibacterial activity of quinoline-based derivatives against methicillin-resistant Staphylococcus aureus and Pseudomonas aeruginosa: design, synthesis, DFT and molecular dynamic simulations. Chem. Biodivers. 20, e202300804. doi:10.1002/cbdv.202300804
Sabt, A., Eldehna, W. M., Ibrahim, T. M., Bekhit, A. A., and Batran, R. Z. (2023a). New antileishmanial quinoline linked isatin derivatives targeting DHFR-TS and PTR1: design, synthesis, and molecular modeling studies. Eur. J. Med. Chem. 246, 114959. doi:10.1016/j.ejmech.2022.114959
Said, M. A., Eldehna, W. M., Nocentini, A., Bonardi, A., Fahim, S. H., Bua, S., et al. (2020). Synthesis, biological and molecular dynamics investigations with a series of triazolopyrimidine/triazole-based benzenesulfonamides as novel carbonic anhydrase inhibitors. Eur. J. Med. Chem. 185, 111843. doi:10.1016/j.ejmech.2019.111843
Senthil, S., Gopi, R., and Raj, C. G. (2015). Synthesis, characterization and antimicrobial activity of somequinoline appended 1, 2, 3-triazoles. J. INDIAN Chem. Soc. 92 (6), 956–959.
Seung, K. J., Keshavjee, S., and Rich, M. L. (2015). Multidrug-resistant tuberculosis and extensively drug-resistant tuberculosis. Cold Spring Harb. Perspect. Med. 5 (9), a017863. . doi:10.1101/cshperspect.a017863
Shaikh, M. H., Subhedar, D. D., Nawale, L., Sarkar, D., Khan, F. A., Sangshetti, J. N., et al. (2015). 1, 2, 3-Triazole derivatives as antitubercular agents: synthesis, biological evaluation and molecular docking study. MedChemComm 6 (6), 1104–1116. doi:10.1039/c5md00057b
Shinde, A., Thakare, P. P., Nandurkar, Y., Bhoye, M., Chavan, A., and Mhaske, P. C. (2023). Synthesis of 2-(6-substituted quinolin-4-yl)-1-(4-aryl-1H-1, 2, 3-triazol-1-yl) propan-2-ol as potential antifungal and antitubercular agents. Eur. J. Med. Chem. Rep. 7, 100102. doi:10.1016/j.ejmcr.2023.100102
Singh, H., Sindhu, J., and Khurana, J. M. (2013). Synthesis of biologically as well as industrially important 1,4,5-trisubstituted-1,2,3-triazoles using a highly efficient, green and recyclable DBU–H2O catalytic system. RSC Adv. 3, 22360–22366. doi:10.1039/c3ra44440f
Van Der Spoel, D., Lindahl, E., Hess, B., Groenhof, G., Mark, A. E., and Berendsen, H. J. (2005). GROMACS: fast, flexible, and free. J. Comput. Chem. 26 (16), 1701–1718. doi:10.1002/jcc.20291
WHO Report (2020). WHO. Available at: https://www.who.int/publications/i/item/9789240018662.
Zhang, S., Xu, Z., Gao, C., Ren, Q. C., Chang, L., Lv, Z. S., et al. (2017). Triazole derivatives and their anti-tubercular activity. Eur. J. Med. Chem. 138, 501–513. doi:10.1016/j.ejmech.2017.06.051
Keywords: quinoline, triazole, biological evaluations, molecular docking, MD simulation
Citation: Sabt A, Abdulla M-H, Ebaid MS, Pawełczyk J, Abd El Salam HA, Son NT, Ha NX, Vaali Mohammed M-A, Traiki T, Elsawi AE, Dziadek B, Dziadek J and Eldehna WM (2024) Identification of 2-(N-aryl-1,2,3-triazol-4-yl) quinoline derivatives as antitubercular agents endowed with InhA inhibitory activity. Front. Chem. 12:1424017. doi: 10.3389/fchem.2024.1424017
Received: 26 April 2024; Accepted: 12 June 2024;
Published: 07 August 2024.
Edited by:
Afzal Basha Shaik, Jawaharlal Nehru Technological University, Kakinada, IndiaReviewed by:
Adil Shareef Mohammed, Temple University, United StatesVamshi Krishna Reddy Sammeta, National Cancer Institute at Frederick (NIH), United States
Copyright © 2024 Sabt, Abdulla, Ebaid, Pawełczyk, Abd El Salam, Son, Ha, Vaali Mohammed, Traiki, Elsawi, Dziadek, Dziadek and Eldehna. This is an open-access article distributed under the terms of the Creative Commons Attribution License (CC BY). The use, distribution or reproduction in other forums is permitted, provided the original author(s) and the copyright owner(s) are credited and that the original publication in this journal is cited, in accordance with accepted academic practice. No use, distribution or reproduction is permitted which does not comply with these terms.
*Correspondence: Wagdy M. Eldehna, d2FnZHkyMDAwQGdtYWlsLmNvbQ==; Maha-Hamadien Abdulla, bWFiZHVsbGFAa3N1LmVkdS5zYQ==; Jaroslaw Dziadek, amR6aWFkZWtAY2JtLnBhbi5wbA==