- 1Institute of Medical Microbiology and Hospital Hygiene, Heinrich Heine University Düsseldorf, University Hospital Düsseldorf, Düsseldorf, Germany
- 2Institute of Bioorganic Chemistry, Heinrich Heine University Düsseldorf at Forschungszentrum Jülich, Jülich, Germany
- 3Institute of Biochemistry, Heinrich Heine University Düsseldorf, Düsseldorf, Germany
- 4Center for Structural Studies, Heinrich Heine University, Düsseldorf, Germany
- 5Institute of Bio- and Geosciences (IBG-1): Biotechnology, Forschungszentrum Jülich GmbH, Jülich, Germany
Toxoplasmosis is a zoonotic disease caused by Toxoplasma gondii, an apicomplexan parasite that infects approximately a third of the world’s human population. This disease can cause serious complications during pregnancy and can be fatal in immunocompromised hosts. The current treatment options for toxoplasmosis face several limitations. Thus, to address the urgent medical need for the discovery of novel anti-toxoplasma potential drug candidates, our research focused on exploring a series of monomeric and dimeric chalcones, polyphenolic molecules belonging to the class of flavonoids. Chalcones 1aa—1bg and axially chiral A-A′-connected bichalcones 2aa—2bg were evaluated in vitro against the proliferation of the parasite in a cell-based assay. A comparison of the efficacy demonstrated that, in several cases, bichalcones exhibited increased bioactivity compared to their corresponding monomeric counterparts. Among these compounds, a bichalcone with a phenyl substituent and a methyl moiety 2ab showed the most potent and selective inhibitory activity in the nanomolar range. Both enantiomers of this bichalcone were synthesized using an axially chiral biphenol building block. The biaryl bond was forged using Suzuki cross-coupling in water under micellar catalysis conditions. Separation of the atropisomers of this biphenol building block was conducted by chiral HPLC on a preparative scale. The biological evaluation of the enantiomers revealed that the (Ra)-enantiomer (Ra)-2ab is the eutomer. These studies suggest that bichalcones may be important drug candidates for further in vivo evaluations for the discovery of anti-toxoplasma drugs.
1 Introduction
Toxoplasma gondii (T. gondii), the causative agent of toxoplasmosis, is a coccidian parasite that belongs to the phylum Apicomplexa (Montoya and Liesenfeld, 2004; Hill et al., 2018). This large phylum includes also other unicellular eukaryotes (Levine, 1988) that survive by infecting a wide range of hosts and cause severe diseases such as malaria [Plasmodium falciparum (Phillips et al., 2017)], babesiosis [Babesia spp. (Homer et al., 2000)], or cryptosporidiosis [Cryptosporidium parvum (Helmy and Hafez, 2022)]. Like all other apicomplexans, T. gondii displays a heteroxenous and complex life cycle. It alternates between sexual replication that occurs exclusively in the Felidae family members (the definitive hosts), and asexual replication in a variety of warm-blooded intermediate hosts, including humans, via three infectious stages: tachyzoites, bradyzoites, and sporozoites (Dubey et al., 1998; Tenter et al., 2000). Due to its widespread distribution, T. gondii is often referred to as one of the most successful parasites (Delgado et al., 2022). Multiple modes of transmission can result in human infections: foodborne, via ingestion of contaminated water and raw or undercooked meat; fecal-oral, via unintentional ingestion of oocysts from cat feces; and also via several minor modes such as congenital transmission and blood or organ transplantation (Dubey, 2021). According to the Centers for Disease Control and Prevention (CDC), it has been estimated that more than 40 million people have been infected with T. gondii in the United States alone (CDC, 2024).
In immunocompetent individuals, the infection is often subclinical or asymptomatic in the acute phase, but can trigger behavioral disorder during the latent phase (Fekadu et al., 2010). Without proper treatment, severe disease, or even death, can occur in immunocompromised individuals or fetuses infected congenitally (Wang et al., 2017; Strang et al., 2020). An increased frequency of toxoplasmic encephalitis has been reported in patients with AIDS (Acquired Immunodeficiency Syndrome) with significant immunosuppression (Lejeune et al., 2011).
The current gold-standard treatment for toxoplasmosis relies on the administration of pyrimethamine (PYR) and sulfadiazine (SDZ) (Konstantinovic et al., 2019). Since its discovery in 1953 by Eyles and Coleman (Eyles and Coleman, 1953), the synergistic nature of this combination therapy has been well-established. This synergy is achieved because both drugs interfere with different steps in the folate pathway of the tachyzoite stage of the parasite, and therefore the acute phase of the infection (Sheffield and Melton, 1975). To mitigate the harmful side effects associated with this PYR-SDZ regimen, including its bone marrow myelosuppression, folinic acid (leucovorin) has been included in the combination (Van Delden and Hirschel, 1996; Alday and Doggett, 2017). Unfortunately, these and other current therapies are still burdened with strong side effects that often lead to patient noncompliance and discontinuation of therapy. Moreover, the lack of drugs that specifically target the cyst form of the parasite, which is responsible for chronic and latent infection, remains a critical limitation. Thus, there is an urgent need for the discovery and development of novel, potent, and well-tolerated treatments to overcome these challenges and improve the wellbeing of patients inflicted with toxoplasmosis (Konstantinovic et al., 2019).
Natural products play a critical role in the field of infectious diseases drug discovery (Newman and Cragg, 2020). These compounds usually offer a wide and diverse range of biological activities, but often show only moderate or weak potency in early evaluations. Thus, synthetic work on natural products is important for the identification of natural product analogs as novel hits and lead compounds (Deng et al., 2019).
A very prominent class of natural products are flavonoids. Among these phenylpropanoid-based organic compounds are chalcones, polyphenolic secondary metabolites of plants (Elkanzi et al., 2022). In particular chalcones, with their α,β-unsaturated ketone moiety (Michael system), reveal ubiquitous bioactive structural motifs (Zhuang et al., 2017; Salehi et al., 2020). Synthetic chalcone derivatives thus have been investigated, incorporating various substitutions in the A- and B-rings, to modulate both potency and bioactivity (Mezgebe et al., 2023; Oliveira et al., 2023). These molecules have attracted great interest in medicinal chemistry and are now considered privileged structures for their simple scaffold and their wide variety of pharmacological activities and characteristics (Zhuang et al., 2017), including antibacterial (Dan and Dai, 2020), anti-inflammatory (Vale et al., 2023), antiviral (Fu et al., 2020), anticancer (Ouyang et al., 2021), antioxidant (Bale et al., 2021), antidiabetic (Rocha et al., 2020), and antimalarial (Qin et al., 2020) properties, among others. In addition, several studies have demonstrated the potential of chalcones as novel anti-toxoplasma agents (Si et al., 2018; Touquet et al., 2018; Al-Hilli et al., 2021; Jiang et al., 2022; Ghazzay et al., 2023), but their mode of action on T. gondii remains unclear. The α,β-unsaturated ketone moiety is considered the main pharmacophore, due to its reactivity and crucial role in various biological activities (Batovska and Todorova, 2010; Sahu et al., 2012). Loss of the ketone moiety has been shown to result in a significant decrease or absence of bioactivity of chalcones (Matos et al., 2015), which is also true for their anti-toxoplasma activity (Jiang et al., 2022). Thus, several synthetic chalcone derivatives have been investigated, preserving the linkage and incorporating various substitutions in the A- and B-rings to modulate both potency and bioactivity (Mezgebe et al., 2023; Oliveira et al., 2023). Overall, three main strategies are used to derivatize the chalcone scaffold: modification of the aryl A- and B-rings, substitution of the aryl rings with heteroaryl rings, and molecular hybridization by conjugating the chalcone scaffold with biologically active scaffolds to enhance a specific bioactivity (Ouyang et al., 2021). Chalcones have been shown to exhibit enhanced antitubercular, antibacterial, antifungal, and antioxidant activities when their aromatics rings feature electron-withdrawing and/or electron-donating substitutions (Gupta and Jain, 2015; da Silva et al., 2023). Notably, the inclusion of the electron-donating amino group on the A aryl ring and an electron-withdrawing group on the aryl B-ring resulted in particularly higher anti-toxoplasma activity (Jiang et al., 2022).
Bichalcones (also commonly referred to as “bis-chalcones”), a subclass of chalcones and thus of the broader class of flavonoids, have been shown to possess intriguing biological activities, including antiplasmodial activity (Ram et al., 2000; Domínguez et al., 2013; Sharma et al., 2018). Despite this, these molecules have hardly been investigated in comparison to chalcones (Pereira et al., 2023). These observations suggest that further research on bichalcones may reveal valuable insights and novel potential therapeutic applications of these molecules.
Bichalcones exist in a variety of different linkages with respect to the monomeric unit (Figure 1). Depending on their scaffolds, different bioactivities were observed. Common dimeric scaffolds include, among others (Masesane et al., 2000; Mdee et al., 2003; Zhang et al., 2013; Arslan et al., 2016; Zhuang et al., 2017; Karaman et al., 2018; Menezes and Diederich, 2019; Pereira et al., 2023) urea- or alkyl linker compounds with antimalarial activity (Ram et al., 2000; Domínguez et al., 2013), as well as A-B′-type or B-B′-type biaryl bichalcones with antiprotozoal (Mihigo et al., 2010) or antiplasmodial activity (Sharma et al., 2018), respectively. To date, only a few A-A′-bichalcones have been synthesized and their bioactivities have hardly been investigated. A particular challenge in their synthesis is the stereogenic biaryl bond, which makes these compounds axially chiral depending on the ortho-substituents of the axis. Lin et al. (Lin and Zhong, 1997) and Li et al. (Li et al., 1997) independently investigated synthetic routes towards enantiopure 8,8′-biflavones, obtaining enantiopure A-A′-bichalcones as key intermediates. Both strategies involved the tedious use of chiral auxiliaries to access the enantiopure products, limiting the scalability of these approaches.
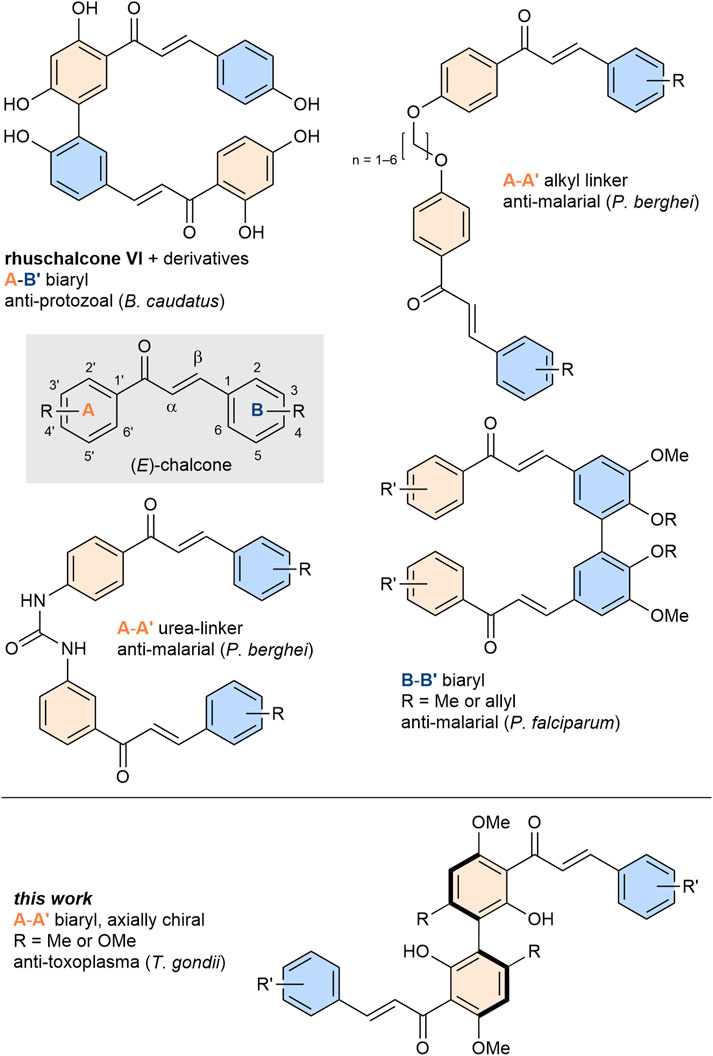
Figure 1. General structure of chalcones and various bichalcones with different linkages and their respective bioactivities. Colors are used to indicate A-rings (orange) and B-rings (blue) respectively for visual support.
In our previous study we investigated the anti-toxoplasma activities of various flavones and biflavones (Klischan et al., 2023; Klischan et al., 2024) (Scheme 1A). There, chalcones 1 and racemic A-A′-bichalcones 2 were obtained as intermediate products (Scheme 1B). However, the biological activity profiles of these compounds were not yet investigated. In this work, we report the anti-toxoplasma activities for this readily available library of simplified natural product analogues. Additionally, following a complementary synthetic strategy both enantiomers of the most active A-A′-bichalcone were synthesized and the first biological evaluation of this elusive class of flavonoids was conducted. Their in vitro anti-toxoplasma activities were evaluated against the T. gondii type II ME49 strain and compared with their monomer chalcone counterparts.
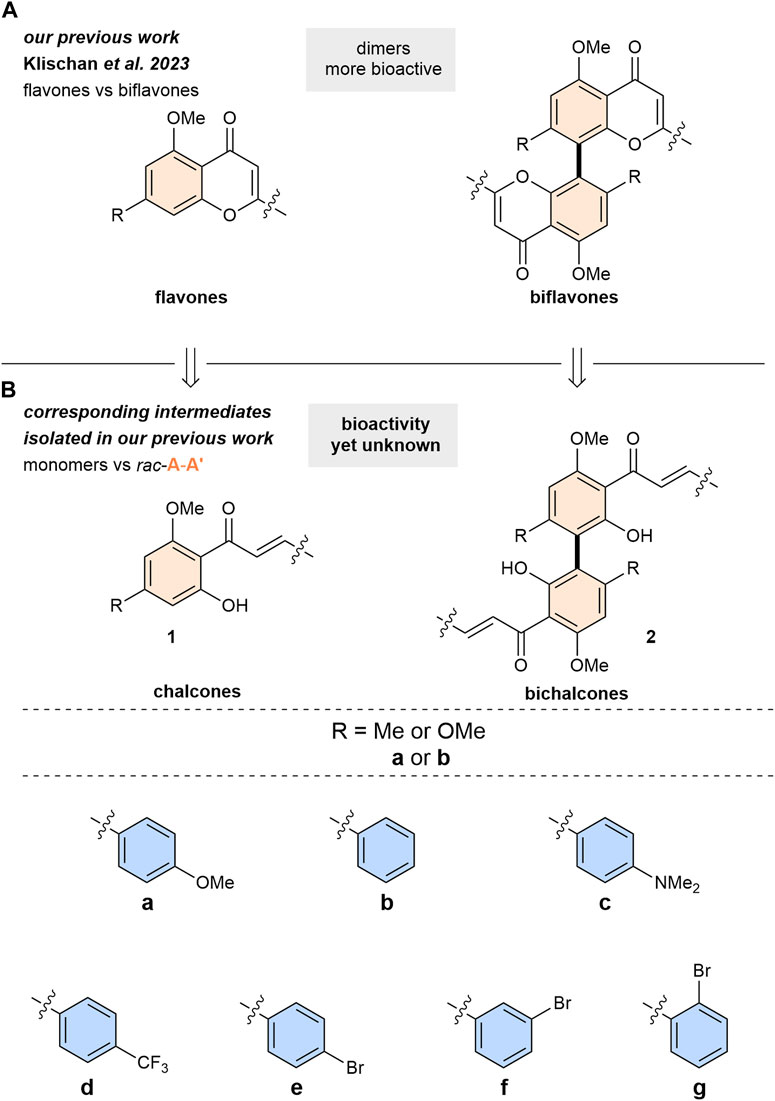
Scheme 1. Library of chalcones and bichalcones. For example: bichalcone (2) with R = Me (a) phenyl substitution at the B-ring (b) = 2ab. Colouring of the rings in accordance with Figure 1.
2 Materials and methods
2.1 Synthesis of chalcones and bichalcones
The chalcones and racemic bichalcones investigated in the present study have been synthesized following established procedures from our previous investigations on the bioactivity of biflavones (Klischan et al., 2023; Klischan et al., 2024). An overview of these syntheses as well as synthetic details, methodologies, and analytical data of the enantioselective synthetic sequence developed in this work are available in the Supplementary Material.
2.2 Parasite and cell culture
The tachyzoite stage of the ME49 strain type II of T. gondii (ATCC/LGC Standards GmbH, Wesel, Germany, #50611) was maintained and propagated by regular passages infecting monolayers of human foreskin fibroblasts Hs27 (ATCC/LGC Standards GmbH, Wesel, Germany, #CRL-1634), in 25 cm2 cell culture flasks, with 5 × 106 tachyzoites. Cultures were grown in Iscove’s Modified Dulbecco’s medium (IMDM; Gibco-Thermo Fisher Scientific, Braunschweig, Germany, #12440053) supplemented with 10% heat-inactivated fetal bovine serum (FBS Standard; South America origin, foetal bovine serum, 2 µm sterile filtered, PAN-Biotech, Aidenbach, Germany, #P30-3,306) and 50 mM 2-mercaptoethanol (Gibco-Thermo Fisher Scientific, Braunschweig, Germany, #21985023) at 37°C and 5% CO2 for 72 h. After incubation, the cell culture supernatant was centrifuged at 700 rpm for 5 min. The parasite density was then measured using a hemocytometer and adjusted accordingly to in vitro experimental infection analysis.
2.3 Compounds for in vitro analysis
Pyrimethamine, staurosporine, and all synthesized chalcones and bichalcones were dissolved in DMSO (dimethyl sulfoxide, ≥99%, Thermo Scientific Chemicals, Braunschweig, Germany, #A12380.36) as 10 mM stock solutions and stored at −20°C. Before use, these solutions were thawed and diluted in culture medium to obtain the appropriate concentrations (ranging from 200 μM to 0.02 µM).
2.4 Toxoplasma gondii in vitro inhibition assay
To evaluate the efficacy of the investigated chalcones and bichalcones against T. gondii proliferation, we conducted in vitro inhibition assays using a similar protocol as previously reported (Merkt et al., 2021; Mazzone et al., 2022; Klischan et al., 2023). Briefly, we seeded 3 × 104 Hs27 cells in 96-well microtiter plates with a total culture volume of 200 µL per well. To avoid edge effects, only the inner 60 wells of each plate were used. After harvesting T. gondii as described previously, the host cells were infected with 3 × 104 tachyzoites per well at a multiplicity of infection (MOI, parasite/host cell) ratio of 1:1. Simultaneously, the compounds were added to the cultures at various concentrations (ranging from 50 μM to 0.02 µM) as described previously. Controls included pyrimethamine (Alday and Doggett, 2017; Konstantinovic et al., 2019) (Merck, Darmstadt, Germany, #219864), T. gondii infected cells only, and human interferon γ (IFN γ) (Pfefferkorn, 1986) (Merck, Darmstadt, Germany, #I17001) pre-stimulated cells (300 U/mL for 24 h) which were subsequently infected with T. gondii. To quantify T. gondii proliferation, the parasites were labeled with tritiated uracil (3H-U; 5 mCi, Hartmann Analytic, Braunschweig, Germany, #ART1782, diluted 1:30) (Pfefferkorn and Pfefferkorn, 1977) after 48 h incubation and incubated for another 28–30 h. Prior to assay evaluation, the 96-well microtiter plates were frozen at −20°C. The plates were then thawed at room temperature, cells were extracted using a harvester (Basic96 Harvester; Zinsser Analytic, Skatron Instruments, Northridge, CA, United States) and transferred to glass-fiber filters (Printed Filtermat A 102 mm × 258 mm; PerkinElmer, Waltham, MA, United States). The filters were then dried in a cabinet at 130°C for 20 min, wetted in 10 mL of scintillation solution (Betaplate Scint; PerkinElmer, Waltham, MA, United States, #1205–440), and sealed with plastic covers (Sample Bag for Betaplate; PerkinElmer, Waltham, MA, United States, #1205–441). The filters were clamped into cassettes and analyzed using a beta-counter (Betaplate Liquid Scintillation Counter 1,205; LKB-WALLAK, Melbourne, Australia) to quantify the amount of radioactive uracil in T. gondii RNA. IC50 values (minimum concentration of compounds required for 50% inhibition in vitro) were determined by nonlinear regression analysis using GraphPad PRISM™ statistical software (version 9.5.1; San Diego, CA).
2.5 Cytotoxicity assay
The MTT [3-(4, 5-dimethylthiazole-2-yl)-2, 5-diphenyltetrazolium bromide] reduction assay (Mosmann, 1983) was used to quantify the cytotoxic effects of the examined chalcones and bichalcones on the host cells. To avoid edge effects, only the inner 60 wells of each plate were used. Briefly, 5 × 104 Hs27 cells per well were cultured in 96-well microtiter plates in Iscove’s modified Dulbecco’s medium (IMDM, Gibco–Thermo Fisher Scientific, Braunschweig, Germany, #12440053) with a volume of 100 µL per well and incubated overnight at 37°C until confluence. Different concentrations of the compounds (ranging from 200 μM to 0.09 µM) were then added to the Hs27 cells. Controls included untreated Hs27 cells treated with DMSO, and cells treated with staurosporine (0.031, 0.062, 0.125, 0.25, 0.5, 1 µM) (Merck, Darmstadt, Germany, #S4400), a natural product known for its potent activity as apoptosis inducer (Belmokhtar et al., 2001). After 24 h of incubation, the culture media were replaced with 100 µL of Dulbecco’s Modified Eagle Medium (DMEM) medium without red phenol (Gibco-Thermo Fisher Scientific, Braunschweig, Germany, #21041025) plus 10% heat-inactivated fetal bovine serum (FBS Standard, South America origin, fetal bovine serum, 2 µm sterile filtered, PAN-Biotech, Aidenbach, Germany, #P30-3,306), and 50 mM 2-mercaptoethanol (Gibco-Thermo Fisher Scientific, Braunschweig, Germany, #21985023). The experiment was performed following the manufacturer’s instructions (CyQuant MTT Cell Viability Assay Kit, Thermo Fisher Scientific, Braunschweig, Germany, #V-13154). Optical density (O.D.) was measured at 570 nm using a microplate reader (TECAN Sunrise, Männedorf, Switzerland). The half-maximal cytotoxic concentration (CC50) values of each compound against Hs27 cells relative to DMSO-treated samples were determined using GraphPad PRISM™ statistical software (version 9.5.1; San Diego, CA). In addition, the selectivity index (SI) of each compound was calculated from the ratio of CC50/IC50.
3 Results
3.1 Bichalcones and chalcones are effective inhibitors of Toxoplasma gondii tachyzoite growth
In view of previous studies demonstrating the effectiveness of chalcones against apicomplexans (Ram et al., 2000; Domínguez et al., 2013; Sharma et al., 2018; Si et al., 2018; Touquet et al., 2018; Qin et al., 2020; Al-Hilli et al., 2021; Jiang et al., 2022; Ghazzay et al., 2023), we evaluated and compared the activity of 14 chalcones 1 and 14 of their dimeric bichalcone 2 counterparts (Scheme 1B). All chalcones 1 and racemic bichalcones 2 were characterized and reported in our previous investigation (Klischan et al., 2023). An overview of the employed synthetic procedures is provided in Supplementary Scheme S1. To determine their potential against T. gondii ME49 tachyzoites, we performed an in vitro proliferation assay based on the uptake of radioactively labeled 3H-uracil (Pfefferkorn and Pfefferkorn, 1977). We then evaluated their IC50 values. As shown in Table 1 and Supplementary Figure S23, chalcones and bichalcones exhibited bioactivity against T. gondii proliferation.
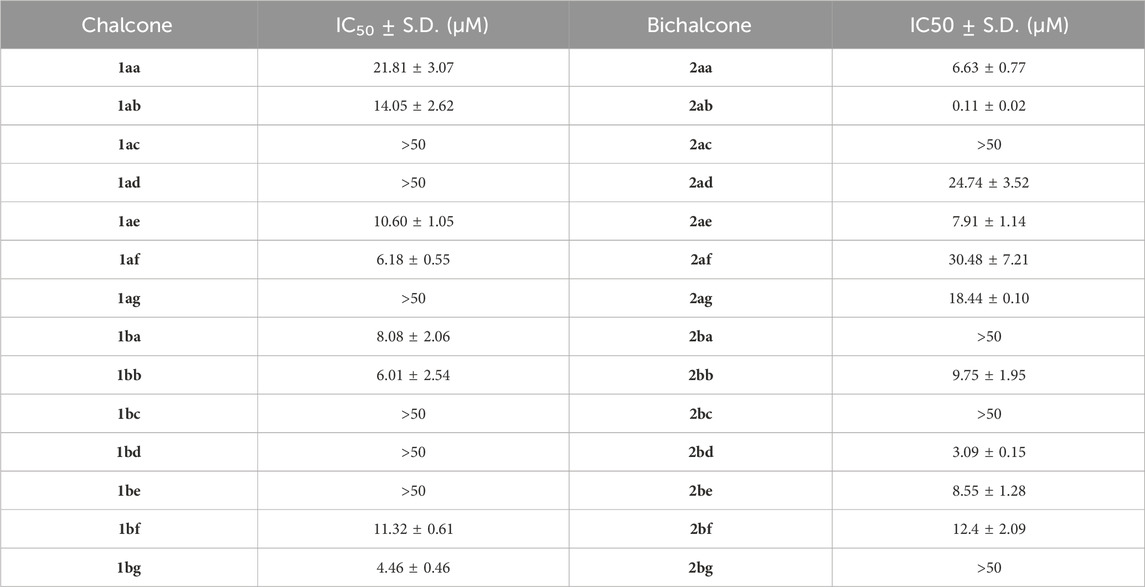
Table 1. In vitro activity of chalcones (1aa–bg) and bichalcones (2aa–bg) against Toxoplasma gondii ME49 tachyzoites. Values shown in the table represent the means of three independent experiments each done in duplicate (n = 6) ± S.D.
Especially, bichalcone 2ab showed an IC50 of 0.11 µM, more potent than its monomeric counterpart (1ab, 14.05 µM) by two orders of magnitude and, overall, more active than all other compounds in our dedicated library (3.09–30.48 µM). Bichalcone 2ab contains a phenyl moiety as the B-ring and a methyl substituent at the A-ring’s 4-position (R = Me) (Scheme 1). Interestingly the latter seems to be important for the anti-toxoplasma activity, since 2bb with a methoxy group (R = OMe) in the same position showed a comparatively low IC50 of 9.75 µM (Table 1; Supplementary Figure S23).
3.2 Bichalcone 2ab is a strong and selective inhibitor of Toxoplasma gondii
To investigate the selectivity of our (bi)chalcone library regarding parasite inhibition versus human host cell cytotoxicity, we assessed their cytotoxic potential in human fibroblasts Hs27 with the MTT reduction assay. The screening revealed weak to no cytotoxicity for chalcones and no cytotoxicity for all bichalcones (Table 2; Supplementary Figure S24). Compound 2ab had the highest selectivity index (SI) of >6,994, indicating low cytotoxicity against human fibroblasts and high activity against T gondii. Therefore, due to its high selectivity, it was selected as our lead compound for further investigation.
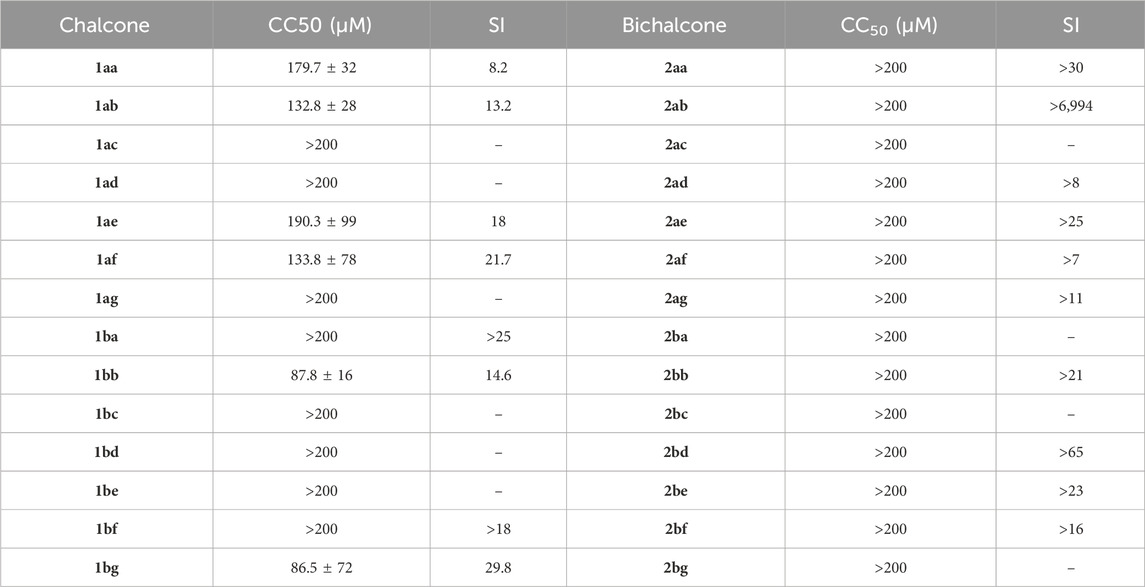
Table 2. In vitro cytotoxicity of chalcones (1aa–bg) and bichalcones (2aa–bg) on human fibroblasts Hs27 and their selectivity indexes (SI) Values shown in the table represent the means of three independent experiments each done in duplicate (n = 6).
3.3 Enantiopure synthesis of (Sa)- and (Ra)-2ab enantiomers
Since 2ab exhibits axial chirality and only its racemic mixture has been studied, we turned our attention to the investigation of the pure enantiomers of 2ab. The aim of this investigation was to discern potential variations in activity and selectivity between the enantiomers. To gain access to both atropisomers of enantiopure bichalcones, our synthetic strategy involved the use of biphenol 3, a building block used in previous studies for the synthesis of various natural product analogs (Ganardi et al., 2023; Greb et al., 2023) (Scheme 2). The readily available brominated starting material 4 was converted to the corresponding boronic acid ester 5 in a scalable manner via a halogen-metal exchange followed by treatment with trimethyl borate and pinacol. Next, the aryl-aryl bond formation was investigated. The sterically demanding tetra-ortho-substituted biaryl bond of 6 was constructed by Suzuki cross-coupling. After screening various conditions (Supplementary Material), we were able to exploit the use of Lipshutz’s TPGS-750-M amphiphile in water under micellar catalysis conditions (Lipshutz et al., 2011), yielding rac-6 in 95% yield and drastically reducing the amount of palladium required [compared to our previous protocol (Ganardi et al., 2023)]. Having acidically deprotected rac-6, we then needed to access enantiomerically pure biphenol 3. To our delight, we found that the separation of both enantiomers was possible by preparative normal phase HPLC using Lux i-Amylose-1 as the column. Due to the unusually good separation performance and high solubility in the polar elution medium, this process could be scaled up to 500 mg per run yielding (Sa)-3 and (Ra)-3 in 49% each, exceeding the scale of previously established synthesis protocols for enantiopure bichalcones (Li et al., 1997; Lin and Zhong, 1997).
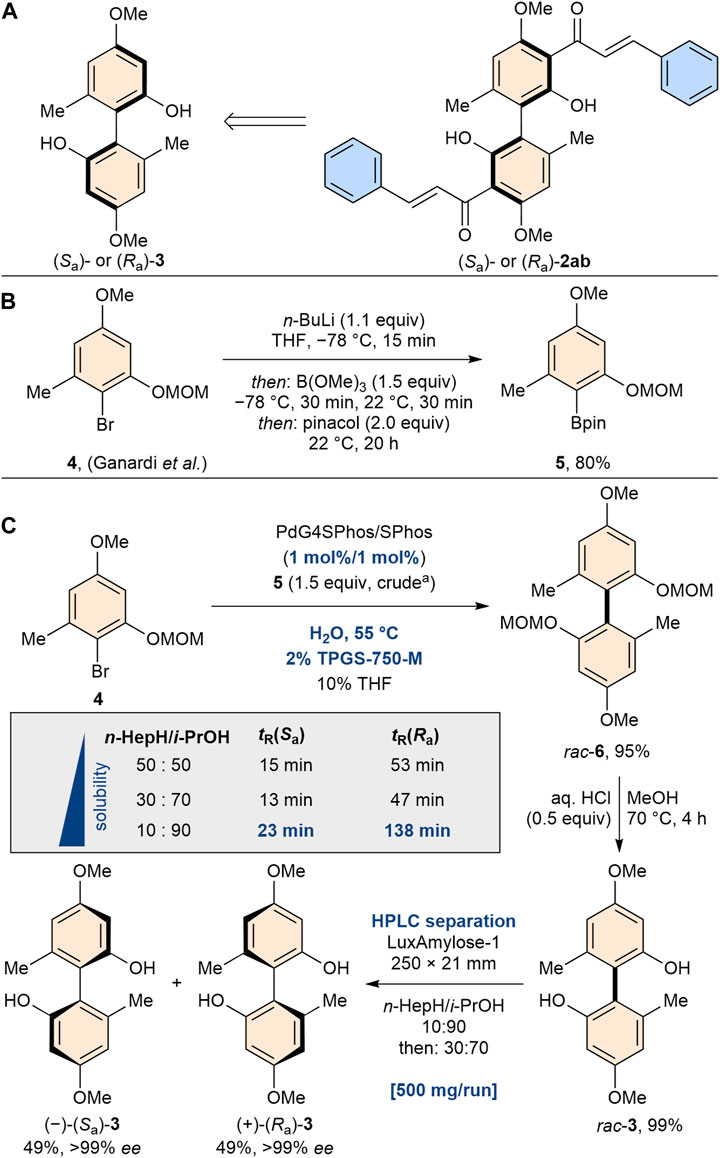
Scheme 2. (A) Synthesis strategy of both enantiomers of bichalcone 2ab. (B) Scalable borylation of readily available brominated starting material 4. (C) Pd-catalyzed (1 mol% Pd) Suzuki cross coupling using amphiphile TPGS-750-M. Separation of the enantiomers was achieved by HPLC because of drastically different elution times (ΔtR = 115 min for n-heptane: i-propanol 10:90 v/v) for both enantiomers using Lux i-Amylose-1.
With both enantiomers of biphenol 3 in hand, we focused our efforts on the synthesis of both enantiomers of bichalcone 2ab. The synthesis of acetophenone 7, following our previously established protocol (Greb et al., 2023), proceeded smoothly for both enantiomers in yields of 83% (Sa) and 76% (Ra), respectively (Scheme 3). We proceeded with the synthesis of both enantiomers of bichalcone 2ab. Racemic bichalcone 2ab was conveniently obtained from our previous investigations on the bioactivity of biflavones (Klischan et al., 2023). In contrast to the racemic mixture of 2ab isolation by column chromatography was feasible. Both enantiomers were obtained in yields of 54% (Sa) and 61% (Ra), respectively, with >99%ee each as determined by HPLC. We attribute the lower yield compared to the racemic mixture to increased formation of flavanone side products.
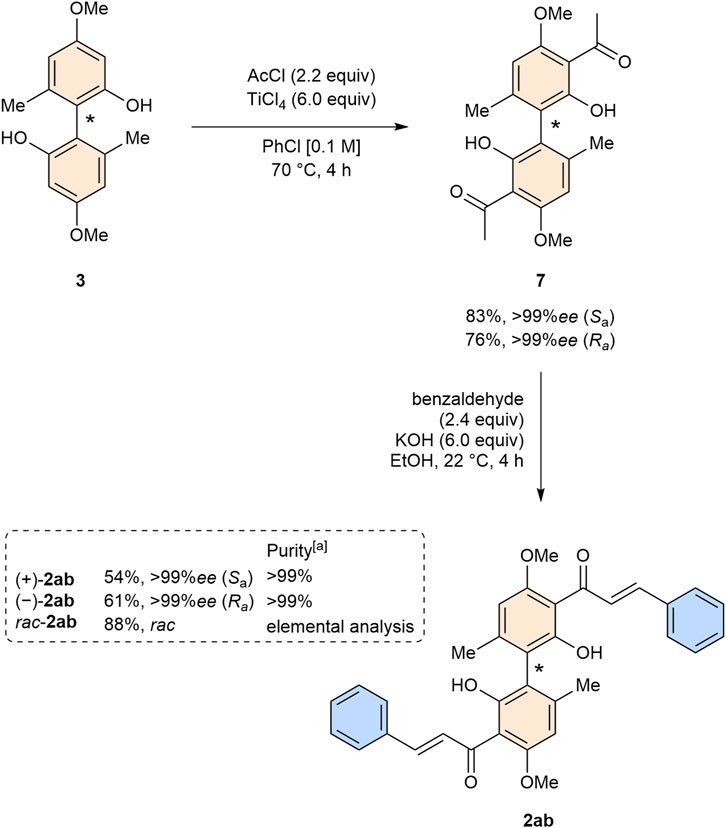
Scheme 3. Synthesis of both enantiomers of bichalcone 2ab. (a) Purity by normal phase and reversed phase HPLC.
3.4 Biological evaluation of the enantiomers of bichalcone 2ab
After the successful synthesis of enantiopure (Sa)-2ab and (Ra)-2ab, we performed a comparative evaluation of their activity against T. gondii tachyzoites and their potential cytotoxicity on Hs27 cells to determine if their absolute configuration has an effect on the observed biological activity. As shown in Table 3 and Supplementary Figure S25, both enantiomers are highly active against T. gondii proliferation with IC50 values in the nanomolar range and no cytotoxicity on Hs27, similar to the racemic mixture. Interestingly (Ra)-2ab was found to be the eutomer (more potent enantiomer) with an IC50 of 0.10 µM, less than half that of the enantiomer (Sa)-2ab, and slightly more active than the racemic mixture. A comparison with PYR–the gold-standard treatment for toxoplasmosis (Konstantinovic et al., 2019)—[IC50 of 0.22 µM, literature 0.4 µM (Touquet et al., 2018)] highlights the significance of our findings (Table 3).
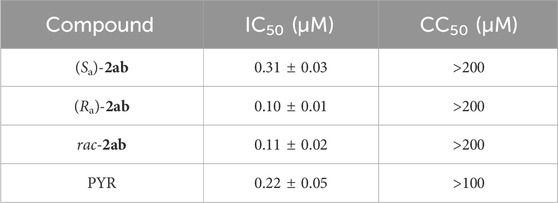
Table 3. In vitro activity (IC50) against Toxoplasma gondii, cytotoxicity (CC50) on human Hs27 cells of (Sa)-2ab (Ra)-2ab, rac-2ab, and pyrimethamine (PYR) Values shown in the table represent the means of three independent experiments each done in duplicate (n = 6) ± S.D.
3.5 ADME prediction of bichalcone 2ab
To additionally evaluate the pharmacokinetic properties of the tested compounds and to assess their drug-likeness, corresponding metrics were calculated using SwissADME (Daina et al., 2017; Bakchi et al., 2022). Table 4 shows a subset of key parameters for selected compounds from these predictions. Among the bichalcones, the most active compound 2ab is indeed the smallest molecule and also has the lowest topological polar surface area (TPSA). In addition, 2ab has the least number of rotatable bonds, which results in it being the only bichalcone with no violations of Veber’s rules for oral bioavailability (Veber et al., 2002) while at the same time showing some solubility and only one violation of Lipinski’s rule of five (Lipinski et al., 2001). Compared to the monomeric chalcones (1aa and 1bb are shown as representatives), 2ab, as well as all other bichalcones, are predicted to show relatively low gastrointestinal (GI) absorption and no blood-brain barrier (BBB) permeation. Interestingly, 2ab is not a substrate of P-glycoproteins (P-gp), transporters belonging to the ATP-binding cassette (ABC) superfamily, that actively efflux small molecules through biological membranes reducing drug accumulation in the GI tract and in the brain by enhancing their elimination (Chen et al., 2018). Furthermore, bichalcone 2ab is predicted to not inhibit any of the cytochrome P450 isoforms, critical metabolic enzymes responsible for drug biotransformation (Zhao et al., 2021). Inhibition of these enzymes can lead to drug-drug interactions (Deodhar et al., 2020). Therefore, bichalcones show overall acceptable drug-like properties, with 2ab fulfilling the most criteria of the bichalcones tested.
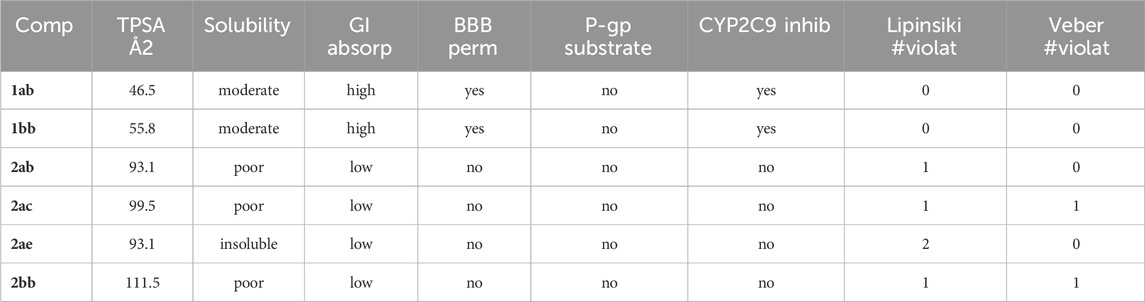
Table 4. Pharmacokinetics and drug-likeness predictions for selected chalcones and bichalcones. Predictions were performed using the SwissADME webtool (Daina et al., 2017; Bakchi et al., 2022). The detailed dataset of predicted property data for all chalcones and bichalcones is given in Supplementary Tables S9–S19.
4 Discussion
Toxoplasmosis, the disease caused by the apicomplexan parasite T. gondii, is the most common infection worldwide, affecting virtually all warm-blooded animals, including humans. Currently available treatments are only able to control the acute infection caused by the actively replicating tachyzoite stage, while having little or no effect on the chronic infection caused by the slowly replicating bradyzoite stage. Furthermore, more than 60 years after its discovery, the combination therapy of pyrimethamine-sulfadiazine (PYR-SDZ) remains the frontline treatment and only few new drugs have been approved for the treatment of toxoplasmosis in humans in the meantime (Dunay et al., 2018).
Previous studies have demonstrated the potential of flavonoids as novel anti-toxoplasma entities in vitro and in vivo, including flavones (Abugri et al., 2018; Klischan et al., 2023) and chalcones (Si et al., 2018; Touquet et al., 2018; Qin et al., 2020; Al-Hilli et al., 2021; Jiang et al., 2022; Ghazzay et al., 2023).
We have recently shown that biflavones are more potent than their monomeric counterpart, for both their antioxidant capacity and anti-toxoplasma in vitro activity (Klischan et al., 2023). Therefore, the objective of the present study was to explore the anti-toxoplasma in vitro activity of chalcones, key synthetic intermediates of flavones obtained in our previous study. With a special focus on the biological comparison between monomers and dimers, this study aims to demonstrate the potential of the less investigated bichalcone counterparts.
Comparison of the in vitro anti-toxoplasma potential of monomers and their axially chiral dimeric counterparts revealed that bichalcones are in some cases more potent than chalcones as was also observed for flavones and biflavones in our previous study (Klischan et al., 2023). In addition, all bichalcones had no cytotoxic effect on human fibroblasts Hs27, thus possessing a better cytotoxic profile than their monomeric counterparts. Bichalcone 2ab exhibits the highest potency and selectivity, being the only one with an IC50 in the nanomolar range. Additionally, predictions of the ADME properties identified 2ab to be rather drug-like thus overall having the potential to be a novel anti-toxoplasma lead compound.
Having identified a highly active racemic compound, we then established the synthesis of both enantiomers of 2ab. In addition to our previous racemic synthesis strategy, we successfully established a complementary atropselective access starting from enantiopure biphenol 3. Synthesis of rac-3 was successfully performed using low amounts of palladium catalyst enabled by an amphiphilic additive. We then separated both enantiomers using chiral HPLC on preparative scale. Finally, both enantiomers of the most active bichalcone 2ab were obtained in satisfactory yields and excellent purity. Comparison of the activity of the two enantiomers (Sa)-2ab and (Ra)-2ab showed that stereochemistry plays a key role in potency, leading to the identification of (Ra)-2ab as the eutomer. In addition, a comparison of the activity with the activity of PYR demonstrated that the enantiomer (Ra)-2ab and the racemic mixture are more potent than the anti-toxoplasma gold standard treatment.
Interestingly, Karaman et al. (2018) performed a structure-based virtual screening to identify of novel sirtuin inhibitors and modulators. Docking studies identified two bichalcones, a rhuschalcone IV and a rhuschalcone I analog, as active inhibitors of human SIRT-1 and SIRT-2 (Karaman et al., 2018). Sirtuins are highly conserved nicotinamide adenine dinucleotide (NAD+)-dependent enzymes. In humans, seven sirtuins are classified as class III histone deacetylases (HDACs) that regulate a wide variety of important intracellular activities, such as metabolism, transcription, and genome stability (Finkel et al., 2009; Abbotto et al., 2022). The T. gondii genome encodes a SIR2 subtype homolog (class III) (Yu et al., 2021a; Yu et al., 2021b). The function of SIR2-like proteins in apicomplexans has not been fully elucidated, although they have been shown to be involved in the epigenetic regulation of virulence genes essential for P. falciparum pathogenesis and persistence (Religa and Waters, 2012). Therefore, we performed a docking study of (Sa)-2ab and (Ra)-2ab with the TgSIR2 homolog to explore whether it could be a potential target of the bichalcones. Unfortunately, the docking studies did not result in a stable protein-ligand complex that could explain the T. gondii growth inhibitory properties. Since these are in silico experiments, there may be several reasons to explain this, but first, initial in vitro binding studies need to be performed to conclusively suggest that this TgSIR2 protein is indeed the target of bichalcones. Thus, further research is needed to elucidate the mechanism of action and to identify the target of 2ab in T. gondii, in particular to investigate the binding interaction with the two enantiomers of 2ab. In addition, in vivo studies are needed to explore their activity and safety in a mouse model system in the future.
Data availability statement
The original contributions presented in the study are included in the article/Supplementary Material, further inquiries can be directed to the corresponding authors.
Ethics statement
Ethical approval was not required for the studies on humans in accordance with the local legislation and institutional requirements because only commercially available established cell lines were used.
Author contributions
FM: Conceptualization, Data curation, Formal Analysis, Investigation, Methodology, Validation, Visualization, Writing–original draft, Writing–review and editing. MK: Conceptualization, Data curation, Formal Analysis, Investigation, Methodology, Validation, Visualization, Writing–original draft, Writing–review and editing. JG: Conceptualization, Data curation, Formal Analysis, Investigation, Methodology, Validation, Visualization, Writing–original draft, Writing–review and editing. SS: Formal Analysis, Investigation, Methodology, Resources, Writing–original draft, Writing–review and editing. JP: Conceptualization, Funding acquisition, Project administration, Resources, Supervision, Writing–original draft, Writing–review and editing. KP: Conceptualization, Funding acquisition, Project administration, Resources, Supervision, Writing–original draft, Writing–review and editing.
Funding
The author(s) declare that financial support was received for the research, authorship, and/or publication of this article. This work was supported by the Deutsche Forschungsgemeinschaft (DFG, German Research Foundation) – project number 270650915/GRK2158 (to SS, JP and KP).
Acknowledgments
We thank Karin Buchholz, Daniel Degrandi, Ursula Sorg, Birgit Henßen and Max Schlamkow for scientific consultation and synthesis support.
Conflict of interest
The authors declare that the research was conducted in the absence of any commercial or financial relationships that could be construed as a potential conflict of interest.
Publisher’s note
All claims expressed in this article are solely those of the authors and do not necessarily represent those of their affiliated organizations, or those of the publisher, the editors and the reviewers. Any product that may be evaluated in this article, or claim that may be made by its manufacturer, is not guaranteed or endorsed by the publisher.
Supplementary material
The Supplementary Material for this article can be found online at: https://www.frontiersin.org/articles/10.3389/fchem.2024.1406307/full#supplementary-material
References
Abbotto, E., Scarano, N., Piacente, F., Millo, E., Cichero, E., and Bruzzone, S. (2022). Virtual screening in the identification of sirtuins’ activity modulators. Molecules 27 (17), 5641. doi:10.3390/molecules27175641
Abugri, D. A., Witola, W. H., Russell, A. E., and Troy, R. M. (2018). In vitro activity of the interaction between taxifolin (dihydroquercetin) and pyrimethamine against Toxoplasma gondii. Chem. Biol. Drug Des. 91 (1), 194–201. doi:10.1111/cbdd.13070
Alday, P. H., and Doggett, J. S. (2017). Drugs in development for toxoplasmosis: advances, challenges, and current status. Drug Des. devel. Ther. 11, 273–293. doi:10.2147/dddt.s60973
Al-Hilli, E. S. A., Ghazzay, M. H., Hasan, S. A., Al-Kelaby, K. K. A., and Zarka, M. A. (2021). Therapeutic effect of chalcone on toxoplasma gondii isolated from recurrent pregnancy loss cases in Al-Najaf City "in vitro study. J. Nat. Remedies 22 (1 (2), 32–40.
Arslan, T., Celik, G., Celik, H., Şentürk, M., Yaylı, N., and Ekinci, D. (2016). Synthesis and biological evaluation of novel bischalcone derivatives as carbonic anhydrase inhibitors. Arch. Pharm. 349 (9), 741–748. doi:10.1002/ardp.201600122
Bakchi, B., Krishna, A. D., Sreecharan, E., Ganesh, V. B. J., Niharika, M., Maharshi, S., et al. (2022). An overview on applications of SwissADME web tool in the design and development of anticancer, antitubercular and antimicrobial agents: a medicinal chemist's perspective. J. Mol. Struct. 1259, 132712. doi:10.1016/j.molstruc.2022.132712
Bale, A. T., Salar, U., Khan, K. M., Chigurupati, S., Fasina, T., Ali, F., et al. (2021). Chalcones and bis-chalcones analogs as DPPH and ABTS radical scavengers. Lett. Drug Des. Discov. 18 (3), 249–257. doi:10.2174/1570180817999201001155032
Batovska, D. I., and Todorova, I. T. (2010). Trends in utilization of the pharmacological potential of chalcones. Curr. Clin. Pharmacol. 5 (1), 1–29. doi:10.2174/157488410790410579
Belmokhtar, C. A., Hillion, J., and Ségal-Bendirdjian, E. (2001). Staurosporine induces apoptosis through both caspase-dependent and caspase-independent mechanisms. Oncogene 20 (26), 3354–3362. doi:10.1038/sj.onc.1204436
Center for Disease Control and Prevention (2024) “Toxoplasmosis: causes and how it spreads,” in Toxoplasmosis. Available at: https://www.cdc.gov/toxoplasmosis/causes/index.html (Accessed June 10, 2024).
Chen, C., Lee, M. H., Weng, C. F., and Leong, M. K. (2018). Theoretical prediction of the complex P-glycoprotein substrate efflux based on the novel hierarchical support vector regression Scheme. Molecules 23 (7), 1820. doi:10.3390/molecules23071820
Daina, A., Michielin, O., and Zoete, V. (2017). SwissADME: a free web tool to evaluate pharmacokinetics, drug-likeness and medicinal chemistry friendliness of small molecules. Sci. Rep. 7, 42717. doi:10.1038/srep42717
Dan, W., and Dai, J. (2020). Recent developments of chalcones as potential antibacterial agents in medicinal chemistry. Eur. J. Med. Chem. 187, 111980. doi:10.1016/j.ejmech.2019.111980
da Silva, L., Donato, I. A., Gonçalves, C. A. C., Scherf, J. R., Dos Santos, H. S., Mori, E., et al. (2023). Antibacterial potential of chalcones and its derivatives against Staphylococcus aureus. 3 Biotech. 13 (1), 1. doi:10.1007/s13205-022-03398-7
Delgado, I. L. S., Zúquete, S., Santos, D., Basto, A. P., Leitão, A., and Nolasco, S. (2022). The apicomplexan parasite toxoplasma gondii. Encyclopedia 2 (1), 189–211. doi:10.3390/encyclopedia2010012
Deng, Y., Wu, T., Zhai, S.-Q., and Li, C.-H. (2019). Recent progress on anti-Toxoplasma drugs discovery: design, synthesis and screening. Eur. J. Med. Chem. 183, 111711. doi:10.1016/j.ejmech.2019.111711
Deodhar, M., Al Rihani, S. B., Arwood, M. J., Darakjian, L., Dow, P., Turgeon, J., et al. (2020). Mechanisms of CYP450 inhibition: understanding drug-drug interactions due to mechanism-based inhibition in clinical practice. Pharmaceutics 12 (9), 846. doi:10.3390/pharmaceutics12090846
Domínguez, J. N., Gamboa de Dominguez, N., Rodrigues, J., Acosta, M. E., Caraballo, N., and León, C. (2013). Synthesis and antimalarial activity of urenyl Bis-chalcone in vitro and in vivo. J. Enzyme Inhibition Med. Chem. 28 (6), 1267–1273. doi:10.3109/14756366.2012.733383
Dubey, J., Lindsay, D., and Speer, C. (1998). Structures of Toxoplasma gondii tachyzoites, bradyzoites, and sporozoites and biology and development of tissue cysts. Clin. Microbiol. Rev. 11 (2), 267–299. doi:10.1128/cmr.11.2.267
Dubey, J. P. (2021). Toxoplasmosis of animals and humans. 3rd ed. Boca Raton, FL: CRC Press. doi:10.1201/9781003199373
Dunay, I. R., Gajurel, K., Dhakal, R., Liesenfeld, O., and Montoya, J. G. (2018). Treatment of toxoplasmosis: historical perspective, animal models, and current clinical practice. Clin. Microbiol. Rev. 31 (4), 000577-e117. doi:10.1128/cmr.00057-17
Elkanzi, N. A., Hrichi, H., Alolayan, R. A., Derafa, W., Zahou, F. M., and Bakr, R. B. (2022). Synthesis of chalcones derivatives and their biological activities: a review. ACS omega 7 (32), 27769–27786. doi:10.1021/acsomega.2c01779
Eyles, D., and Coleman, N. (1953). Synergistic effect of sulfadiazine and daraprim against experimental toxoplasmosis in the mouse. Antibiot. Chemother. 3 (5), 483–490.
Fekadu, A., Shibre, T., and Cleare, A. J. (2010). Toxoplasmosis as a cause for behaviour disorders-overview of evidence and mechanisms. Folia Parasitol. (Praha) 57 (2), 105–113. doi:10.14411/fp.2010.013
Finkel, T., Deng, C.-X., and Mostoslavsky, R. (2009). Recent progress in the biology and physiology of sirtuins. Nature 460 (7255), 587–591. doi:10.1038/nature08197
Fu, Y., Liu, D., Zeng, H., Ren, X., Song, B., Hu, D., et al. (2020). New chalcone derivatives: synthesis, antiviral activity and mechanism of action. RSC Adv. 10 (41), 24483–24490. doi:10.1039/d0ra03684f
Ganardi, R. C., Greb, J., Henssen, B., and Pietruszka, J. (2023). Atroposelective total synthesis of (+)-Isokotanin A via combined metal and enzyme catalysis. Adv. Synth. Catal. 365, 3512–3520. doi:10.1002/adsc.202300698
Ghazzay, M. H., Hasan, S. A., DeliKhudhair, O., and Abbas, K. K. (2023). Synthesis, characterization, and anti-parasitic activity evaluation of the synthesized chalcone against toxoplasma gondii isolated from cases of abortion in Al-najaf city. J. Med. Chem. Sci. doi:10.26655/jmchemsci.2023.1.16
Greb, J., Drennhaus, T., Klischan, M. K., Schroeder, Z. W., Frey, W., and Pietruszka, J. (2023). A common C2-symmetric 2, 2′-biphenol building block and its application in the synthesis of (+)-di-epi-Gonytolide A. Chemistry–A Eur. J. 29 (34), e202300941. doi:10.1002/chem.202300941
Gupta, D., and Jain, D. K. (2015). Chalcone derivatives as potential antifungal agents: synthesis, and antifungal activity. J. Adv. Pharm. Technol. Res. 6 (3), 114–117. doi:10.4103/2231-4040.161507
Helmy, Y. A., and Hafez, H. M. (2022). Cryptosporidiosis: from prevention to treatment, a narrative review. Microorganisms 10 (12), 2456. doi:10.3390/microorganisms10122456
Hill, D. E., and Dubey, J. P. (2018) “Toxoplasma gondii,” in Foodborne parasites. Editors Y. R. Ortega, and C. R. Sterling (Cham: Springer International Publishing), 119–138. doi:10.1007/978-3-319-67664-7_6
Homer, M. J., Aguilar-Delfin, I., Telford, S. R., Krause, P. J., and Persing, D. H. (2000). Babesiosis. Clin. Microbiol. Rev. 13 (3), 451–469. doi:10.1128/cmr.13.3.451
Jiang, L., Liu, B., Hou, S., Su, T., Fan, Q., Alyafeai, E., et al. (2022). Discovery and evaluation of chalcone derivatives as novel potential anti-Toxoplasma gondii agents. Eur. J. Med. Chem. 234, 114244. doi:10.1016/j.ejmech.2022.114244
Karaman, B., Alhalabi, Z., Swyter, S., Mihigo, S. O., Andrae-Marobela, K., Jung, M., et al. (2018). Identification of bichalcones as sirtuin inhibitors by virtual screening and in vitro testing. Molecules 23 (2), 416. doi:10.3390/molecules23020416
Klischan, M. K. T., Mazzone, F., Berning, L., Greb, J., Schlamkow, M., Haase, M., et al. (2023). Modular approach for the synthesis and bioactivity profiling of 8,8′-biflavones. ACS Omega 8 (44), 41816–41834. doi:10.1021/acsomega.3c06503
Klischan, M. K. T., David, C., Grudzinski, D., Frey, W., Stork, B., and Pietruszka, J. (2024). Application of cyclic diaryliodonium salts in the synthesis of axially chiral natural product analogues. Org. Lett. 26 (25), 5258–5262. doi:10.1021/acs.orglett.4c01308
Konstantinovic, N., Guegan, H., Stäjner, T., Belaz, S., and Robert-Gangneux, F. (2019). Treatment of toxoplasmosis: current options and future perspectives. Food waterborne Parasitol. 15, e00036. doi:10.1016/j.fawpar.2019.e00036
Lejeune, M., Miró, J. M., De Lazzari, E., García, F., Claramonte, X., Martínez, E., et al. (2011). Restoration of T Cell responses to toxoplasma gondii after successful combined antiretroviral therapy in patients with AIDS with previous toxoplasmic encephalitis. Clin. Infect. Dis. 52 (5), 662–670. doi:10.1093/cid/ciq197
Levine, N. D. (1988). Progress in taxonomy of the Apicomplexan protozoa. J. protozoology 35 (4), 518–520. doi:10.1111/j.1550-7408.1988.tb04141.x
Li, H.-Y., Nehira, T., Hagiwara, M., and Harada, N. (1997). Total synthesis and absolute stereochemistry of the natural atropisomer of the biflavone 4 ‘, 4 ‘‘‘, 7, 7 ‘‘-tetra-O-methylcupressuflavone. J. Org. Chem. 62 (21), 7222–7227. doi:10.1021/jo970670w
Lin, G.-Q., and Zhong, M. (1997). The first enantioselective synthesis of optically pure (R)- and (S)-5,5″-dihydroxy-4′,4‴,7,7″-tetramethoxy-8,8″-biflavone and the reconfirmation of their absolute configuration. Tetrahedron Lett. 38 (6), 1087–1090. doi:10.1016/s0040-4039(96)02475-6
Lipinski, C. A., Lombardo, F., Dominy, B. W., and Feeney, P. J. (2001). Experimental and computational approaches to estimate solubility and permeability in drug discovery and development settings 1PII of original article: S0169-409X(96)00423-1. The article was originally published in Advanced Drug Delivery Reviews 23 (1997) 3–25. 1. Adv. Drug Deliv. Rev. 46 (1-3), 3–26. doi:10.1016/s0169-409x(00)00129-0
Lipshutz, B. H., Ghorai, S., Abela, A. R., Moser, R., Nishikata, T., Duplais, C., et al. (2011). TPGS-750-M: a second-generation amphiphile for metal-catalyzed cross-couplings in water at room temperature. J. Org. Chem. 76 (11), 4379–4391. doi:10.1021/jo101974u
Masesane, I. B., Yeboah, S. O., Liebscher, J., Mügge, C., and Abegaz, B. M. (2000). A bichalcone from the twigs of Rhus pyroides. Phytochemistry 53 (8), 1005–1008. doi:10.1016/s0031-9422(99)00553-1
Matos, M. J., Vazquez-Rodriguez, S., Uriarte, E., and Santana, L. (2015). Potential pharmacological uses of chalcones: a patent review (from June 2011 - 2014). Expert Opin. Ther. Pat. 25 (3), 351–366. doi:10.1517/13543776.2014.995627
Mazzone, F., Simons, V. E., van Geelen, L., Frank, M., Mándi, A., Kurtán, T., et al. (2022). In vitro biological activity of natural products from the endophytic fungus paraboeremia selaginellae against toxoplasma gondii. Antibiotics 11 (9), 1176. doi:10.3390/antibiotics11091176
Mdee, L. K., Yeboah, S. O., and Abegaz, B. M. (2003). Rhuschalcones II− VI, five new bichalcones from the root bark of rhus p yroides. J. Nat. Prod. 66 (5), 599–604. doi:10.1021/np020138q
Menezes, J. C., and Diederich, M. F. (2019). Natural dimers of coumarin, chalcones, and resveratrol and the link between structure and pharmacology. Eur. J. Med. Chem. 182, 111637. doi:10.1016/j.ejmech.2019.111637
Merkt, F. K., Mazzone, F., Sazzadeh, S. S., Bonda, L., Hinz, L. K., Gruber, I., et al. (2021). Fluorescent indolo [3,2-a] phenazines against toxoplasma gondii: concise synthesis by gold-catalyzed cycloisomerization with 1,2-silyl migration and ipso-iodination Suzuki sequence. Chemistry–A Eur. J. 27 (38), 9774–9781. doi:10.1002/chem.202101391
Mezgebe, K., Melaku, Y., and Mulugeta, E. (2023). Synthesis and pharmacological activities of chalcone and its derivatives bearing N-heterocyclic scaffolds: a review. ACS Omega 8 (22), 19194–19211. doi:10.1021/acsomega.3c01035
Mihigo, S. O., Mammo, W., Bezabih, M., Andrae-Marobela, K., and Abegaz, B. M. (2010). Total synthesis, antiprotozoal and cytotoxicity activities of rhuschalcone VI and analogs. Bioorg. Med. Chem. 18 (7), 2464–2473. doi:10.1016/j.bmc.2010.02.055
Montoya, J. G., and Liesenfeld, O. (2004). Toxoplasmosis. Lancet 363 (9425), 1965–1976. doi:10.1016/s0140-6736(04)16412-x
Mosmann, T. (1983). Rapid colorimetric assay for cellular growth and survival: application to proliferation and cytotoxicity assays. J. Immunol. Methods 65 (1-2), 55–63. doi:10.1016/0022-1759(83)90303-4
Newman, D. J., and Cragg, G. M. (2020). Natural products as sources of new drugs over the nearly four decades from 01/1981 to 09/2019. J. Nat. Prod. 83 (3), 770–803. doi:10.1021/acs.jnatprod.9b01285
Oliveira, A., Cenci, A., Gonçalves, L., Thedy, M. E., Justino, A., Braga, A., et al. (2023). Chalcone derivatives as antibacterial agents: an updated overview. Curr. Med. Chem. doi:10.2174/0929867330666230220140819
Ouyang, Y., Li, J., Chen, X., Fu, X., Sun, S., and Wu, Q. (2021). Chalcone derivatives: role in anticancer therapy. Biomolecules 11 (6), 894. doi:10.3390/biom11060894
Pereira, R., Silva, A. M., Ribeiro, D., Silva, V. L., and Fernandes, E. (2023). Bis-chalcones: a review of synthetic methodologies and anti-inflammatory effects. Eur. J. Med. Chem. 252, 115280. doi:10.1016/j.ejmech.2023.115280
Pfefferkorn, E. (1986) “Interferon gamma and the growth of Toxoplasma gondii in fibroblasts,” in Annales de l'Institut Pasteur/Microbiologie. Elsevier.
Pfefferkorn, E. R., and Pfefferkorn, L. C. (1977). Specific labeling of intracellular Toxoplasma gondii with uracil. J. Protozool. 24 (3), 449–453. doi:10.1111/j.1550-7408.1977.tb04774.x
Phillips, M. A., Burrows, J. N., Manyando, C., van Huijsduijnen, R. H., Van Voorhis, W. C., and Wells, T. N. C. (2017). Malaria. Nat. Rev. Dis. Prim. 3 (1), 17050. doi:10.1038/nrdp.2017.50
Qin, H.-L., Zhang, Z.-W., Lekkala, R., Alsulami, H., and Rakesh, K. (2020). Chalcone hybrids as privileged scaffolds in antimalarial drug discovery: a key review. Eur. J. Med. Chem. 193, 112215. doi:10.1016/j.ejmech.2020.112215
Ram, V. J., Saxena, A. S., Srivastava, S., and Chandra, S. (2000). Oxygenated chalcones and bischalcones as potential antimalarial agents. Bioorg. Med. Chem. Lett. 10 (19), 2159–2161. doi:10.1016/s0960-894x(00)00409-1
Religa, A. A., and Waters, A. P. (2012). Sirtuins of parasitic protozoa: in search of function(s). Mol. Biochem. Parasitol. 185 (2), 71–88. doi:10.1016/j.molbiopara.2012.08.003
Rocha, S., Ribeiro, D., Fernandes, E., and Freitas, M. (2020). A systematic review on anti-diabetic properties of chalcones. Curr. Med. Chem. 27 (14), 2257–2321. doi:10.2174/0929867325666181001112226
Sahu, N. K., Balbhadra, S. S., Choudhary, J., and Kohli, D. V. (2012). Exploring pharmacological significance of chalcone scaffold: a review. Curr. Med. Chem. 19 (2), 209–225. doi:10.2174/092986712803414132
Salehi, B., Quispe, C., Chamkhi, I., El Omari, N., Balahbib, A., Sharifi-Rad, J., et al. (2020). Pharmacological properties of chalcones: a review of preclinical including molecular mechanisms and clinical evidence. Front. Pharmacol. 11, 592654. doi:10.3389/fphar.2020.592654
Sharma, U. K., Mohanakrishnan, D., Sharma, N., Equbal, D., Sahal, D., and Sinha, A. K. (2018). Facile synthesis of vanillin-based novel bischalcones identifies one that induces apoptosis and displays synergy with Artemisinin in killing chloroquine resistant Plasmodium falciparum. Eur. J. Med. Chem. 155, 623–638. doi:10.1016/j.ejmech.2018.06.025
Sheffield, H. G., and Melton, M. L. (1975). Effect of pyrimethamine and sulfadiazine on the fine structure and multiplication of toxoplasma gondii in cell cultures. J. Parasitol. 61 (4), 704–712. doi:10.2307/3279470
Si, H., Xu, C., Zhang, J., Zhang, X., Li, B., Zhou, X., et al. (2018). Licochalcone A: an effective and low-toxicity compound against Toxoplasma gondii in vitro and in vivo. Int. J. Parasitol. Drugs Drug Resist. 8 (2), 238–245. doi:10.1016/j.ijpddr.2018.02.006
Strang, A. G., Ferrari, R. G., do Rosário, D. K., Nishi, L., Evangelista, F. F., Santana, P. L., et al. (2020). The congenital toxoplasmosis burden in Brazil: systematic review and meta-analysis. Acta Trop. 211, 105608. doi:10.1016/j.actatropica.2020.105608
Tenter, A. M., Heckeroth, A. R., and Weiss, L. M. (2000). Toxoplasma gondii: from animals to humans. Int. J. Parasitol. 30 (12), 1217–1258. doi:10.1016/s0020-7519(00)00124-7
Touquet, B., Pelissier, L., Cavailles, P., Yi, W., Bellini, V., Mercier, C., et al. (2018). High-content imaging assay to evaluate Toxoplasma gondii infection and proliferation: a multiparametric assay to screen new compounds. PLoS One 13 (8), e0201678. doi:10.1371/journal.pone.0201678
Vale, A., Lucas, M., Ribeiro, D., and Fernandes, E. (2023). Research into new molecules with anti-inflammatory activity. Med. Sci. Forum. 21, 12. doi:10.3390/ECB2023-14095
Van Delden, C., and Hirschel, B. (1996). Folinic acid supplements to pyrimethamine-sulfadiazine for Toxoplasma encephalitis are associated with better outcome. J. Infect. Dis. 173 (5), 1294–1295. doi:10.1093/infdis/173.5.1294
Veber, D. F., Johnson, S. R., Cheng, H.-Y., Smith, B. R., Ward, K. W., and Kopple, K. D. (2002). Molecular properties that influence the oral bioavailability of drug candidates. J. Med. Chem. 45 (12), 2615–2623. doi:10.1021/jm020017n
Wang, Z.-D., Liu, H.-H., Ma, Z.-X., Ma, H.-Y., Li, Z.-Y., Yang, Z.-B., et al. (2017). Toxoplasma gondii infection in immunocompromised patients: a systematic review and meta-analysis. Front. Microbiol. 8, 389. doi:10.3389/fmicb.2017.00389
Yu, Z., Chen, S., Aleem, M., He, S., Yang, Y., Zhou, T., et al. (2021a). Histone deacetylase SIR2 in Toxoplasma gondii modulates functions of murine macrophages in vitro and protects mice against acute toxoplasmosis in vivo. Microb. Pathog. 154, 104835. doi:10.1016/j.micpath.2021.104835
Yu, Z., Lu, Y., Cao, W., Aleem, M. T., Liu, J., Luo, J., et al. (2021b). Nano DNA vaccine encoding Toxoplasma gondii histone deacetylase SIR2 enhanced protective immunity in Mice. Pharmaceutics 13 (10), 1582. doi:10.3390/pharmaceutics13101582
Zhang, E.-H., Wang, R.-F., Guo, S.-Z., and Liu, B. (2013). An update on antitumor activity of naturally occurring chalcones. Evid. Based Complement. Altern. Med. 2013, 1–22. doi:10.1155/2013/815621
Zhao, M., Ma, J., Li, M., Zhang, Y., Jiang, B., Zhao, X., et al. (2021). Cytochrome P450 enzymes and drug metabolism in humans. Int. J. Mol. Sci. 22 (23), 12808. doi:10.3390/ijms222312808
Keywords: Toxoplasma gondii, bichalcones, anti-infective, anti-toxoplasma, stereoisomers, biaryl, bioactivity
Citation: Mazzone F, Klischan MKT, Greb J, Smits SHJ, Pietruszka J and Pfeffer K (2024) Synthesis and In vitro evaluation of bichalcones as novel anti-toxoplasma agents. Front. Chem. 12:1406307. doi: 10.3389/fchem.2024.1406307
Received: 24 March 2024; Accepted: 24 June 2024;
Published: 22 July 2024.
Edited by:
Søren Brøgger Christensen, University of Copenhagen, DenmarkReviewed by:
Afzal Basha Shaik, Jawaharlal Nehru Technological University, Kakinada, IndiaSudarsan (Sudarshan) Kasireddy, Purdue University, United States
Copyright © 2024 Mazzone, Klischan, Greb, Smits, Pietruszka and Pfeffer. This is an open-access article distributed under the terms of the Creative Commons Attribution License (CC BY). The use, distribution or reproduction in other forums is permitted, provided the original author(s) and the copyright owner(s) are credited and that the original publication in this journal is cited, in accordance with accepted academic practice. No use, distribution or reproduction is permitted which does not comply with these terms.
*Correspondence: Jörg Pietruszka, ai5waWV0cnVzemthQGZ6LWp1ZWxpY2guZGU=; Klaus Pfeffer, a2xhdXMucGZlZmZlckBoaHUuZGU=
†These authors share first authorship