- 1Department of Dairy Microbiology, SMC College of Dairy Science, Kamdhenu University, Anand, Gujarat, India
- 2Department of Rural Development and Agricultural Production, North-Eastern Hill University, Tura Campus, Chasingre, Meghalaya, India
- 3Departmentof Agriculture Biotechnology, Anand Agricultural University, Anand, Gujarat, India
- 4Regional Center for Biotechnology, Faridabad, Haryana, India
- 5Healthy Gut Research Group, Food and Nutritional Biotechnology Division, National Agri-Food Biotechnology Institute, SAS Nagar, Punjab, India
- 6Department of Microbiology, School of Interdisciplinary and Applied Sciences, Central University of Haryana, Mahendergarh, India
- 7School of Food and Biological Engineering, Shaanxi University of Science and Technology, Xi’an, China
- 8Department of Food Process Engineering, National Institute of Technology, Rourkela, India
- 9Department of Animal Genetics and Breeding, College of Veterinary Science, Kamdhenu University, Anand, Gujarat, India
This study investigated the synthesis of bioactive peptides from sheep milk through fermentation with Limosilactobacillus fermentum KGL4 MTCC 25515 strain and assessed lipase inhibition, ACE inhibition, α-glucosidase inhibition, and α-amylase inhibition activities during the fermentation process. The study observed the highest activities, reaching 74.82%, 70.02%, 72.19%, and 67.08% (lipase inhibition, ACE inhibition, α-glucosidase inhibition, and α-amylase inhibition) after 48 h at 37°C, respectively. Growth optimization experiments revealed that a 2.5% inoculation rate after 48 h of fermentation time resulted in the highest proteolytic activity at 9.88 mg/mL. Additionally, fractions with less than 3 kDa of molecular weight exhibited superior ACE-inhibition and anti-diabetic activities compared to other fractions. Fermentation of sheep milk with KGL4 led to a significant reduction in the excessive production of NO, TNF-α, IL-6, and IL-1β produced in RAW 267.4 cells upon treatment with LPS. Peptides were purified utilizing SDS-PAGE and electrophoresis on 2D gels, identifying a maximum number of proteins bands ranging 10–70 kDa. Peptide sequences were cross-referenced with AHTPDB and BIOPEP databases, confirming potential antihypertensive and antidiabetic properties. Notably, the peptide (GPFPILV) exhibited the highest HPEPDOCK score against both α-amylase and ACE.
1 Introduction
Milk is a favorable substrate for the propagation of lactic acid bacteria (LAB) and the enzymatic breakdown of milk proteins, as indicated by Padhi et al. (2022), leading to the production of numerous bioactive peptides. Throughout the fermentation process, a wide range of microbial-associated proteases, involved in metabolic activities, alter the protein structure in food into peptides (Raveschot et al., 2018). The protein content in milk significantly impacts its nutritional value, and milk proteins and their fractions, especially casein and whey proteins, serve as valuable sources of various bioactive peptides with diverse biological functions. These include antithrombotic, antimicrobial, antioxidant, antihypertensive, and immunomodulatory activities (Park and Nam, 2015).
According to the FAO (2017), sheep milk accounts for approximately 36.5% of total small ruminant milk production globally. There is a rising global demand for sheep milk, and it is estimated that it will have surged by approximately 2.7 metric tonnes (+26%) by 2030 (FAO, 2018). Sheep milk, known for its abundant content of whey protein (1.02–1.3 g/100 g) and casein (4.2–5.2g/100 g) (Selvaggi et al., 2014; Dario et al., 2008) is particularly enriched with these essential components. The consumption of fermented sheep milk is linked to health advantages such as reduced acute inflammation and lowered risk of hypertension and type 2 diabetes. These peptides, derived from a diverse range of fermented foods, play a role in selecting natural and effective ACE inhibitors with minimal or no adverse effects (Baba et al., 2021).
In 2017, 6.7 million deaths globally were attributed to diabetes. Diabetes type 1 impacts over 1.2 million people aged 0–19, with 21 million live births (one in six) experiencing it. As of 26 April 2022, it is projected that 541 million adults will be affected by type 2 diabetes (Lynch, 2022). According to Kannan (2019), India is home to a diabetic population of 77 million, ranking second globally. In managing diabetes, therapeutic interventions target enzymes such as α-amylase, α-glucosidase, and dipeptidyl peptidase-IV (DPP-IV) (Ibrahim et al., 2019). Key enzymes such as α-amylase and α-glucosidase play pivotal roles in breaking down long-chain carbohydrates and releasing absorbable monosaccharides (Sarteshnizi et al., 2021).
Hypertension is a significant contributor to global mortality, leading to a collaborative endeavor to reduce its occurrence by 33% between 2010 and 2030, as outlined in the World Health Organization (WHO) objectives for 2021. In India, the impact of hypertension on the healthcare system is evident from the 2016 Global Burden of Disease (GBD) research, indicating that hypertension alone was responsible for 1.63 million deaths there (Vos et al., 2017). GBD data further disclose that elevated systolic blood pressure plays a substantial role in more than half of the deaths associated with ischemic heart disease (54.2%), chronic kidney disease (54.5%), and stroke (56.2%). With the prevalence of hypertension increasing in India, there is a pressing need to address this health concern (Gupta et al., 2019). The regulation of hypertension involves the renin–angiotensin system (RAS) or renin–angiotensin–aldosterone system, which is essential for controlling peripheral hypertension through ACE-I activity (Baba et al., 2021). Angiotensinogen, continually synthesized by the liver, undergoes transformation from powerful vasoconstrictor angiotensin II (an octapeptide) to angiotensin I (a decapeptide) through the catalytic action of secreted renin in the liver. Additionally, ACE worsens elevated blood pressure by breaking down bradykinin and enkephalins, which function as potent vasodilators (Ningrum et al., 2022).
Changes in contemporary dietary habits and lifestyles have led to an increased susceptibility to disease in humans. In contrast to pharmaceuticals that may have potential side effects, natural foods provide a myriad of health benefits. Small ruminant milk, such as sheep milk, is often required for medicinal purposes, particularly in the case of young children (Haenlein, 2001). The global demand for sheep milk is growing due to its abundant mineral content, elevated levels of protein and fat, and its function as a top supplier of bioactive, functional peptides (BAPs) (Mohapatra et al., 2019). Medical meals can be made with sheep milk, which contains bioactive components. Despite some credible experiences that highlight the medical significance of sheep milk (Razafindrakoto et al., 1993), there is limited research in this domain. However, there is some scholarship that discusses the creation of bioactive peptides (BAPs) from fermented sheep milk utilizing the Limosilactobacillus fermentum KGL4 MTCC 25515 strain. This study highlights the ACE-I (angiotensin-converting enzyme inhibition) and antidiabetic properties during the fermentation of sheep milk. The distinctiveness of this research concerns the isolation and identification of peptide sequences displaying ACE-I and antidiabetic effects, employing Lactobacillus from the fermented sheep milk of the Panchali breed in Gujarat, India. Additionally, this investigation assesses the potential anti-inflammatory properties of fermented sheep milk using RAW 267.4 cells and also explores the in silico analysis of the peptides through a molecular docking study.
2 Materials and methods
2.1 Culture
The LAB culture Limosilactobacillus fermentum KGL4 MTCC 25515 was taken from the Cultural Collection of the Department of Dairy Microbiology, SMC College of Dairy Science, KU, in Anand, Gujarat, India. During the investigation, the preservation and activation of both cultures were carried out using MRS broth media supplied by HiMedia, India, at 37°C.
2.2 Sample preparation
The Panchali breed sheep milk was procured from a non-commercial provider in Asodar, Anand, Gujarat, India, under hygienic conditions. The freshly obtained sheep milk underwent filtration using a sanitary muslin cloth followed by prompt heat treatment at 85°C for 10 min, as per Ashokbhai et al. (2022a). Subsequently, the treated milk was preserved in sterile glass containers under refrigerated conditions (5°C ± 1°C) for potential utilization in this research.
To initiate the growth of KGL4 culture, a 2% introduction was performed in sheep milk, and the blend underwent a 24-h incubation at 37°C. Following this, KGL4 was introduced at 2% rates into 10 mL of sterilized, heat-treated sheep milk. Incubation intervals were 0, 12, 24, 36, and 48 h at 37°C. Subsequently, the mixture was centrifuged at 4193 g force for 20 min at 4°C in an Indian-made Plasto Craft centrifuge. The resulting supernatant was then gathered and subjected to filtration with a 0.22-μm Millex®-HV syringe filter (MERK, Ireland). The supernatant was then employed in diverse assays of ACE inhibitory, anti-diabetic (α-glucosidase, lipase, and α-amylase inhibitions), and proteolytic activity.
2.3 Assessment of ACE (angiotensin-converting enzyme) inhibition
The percentage inhibition activity of the ACE enzyme using fermented sheep milk supernatants utilizing hippuryl-L-histidyl-L-leucine (HHL) as a substrate was evaluated as per Cushman and Cheung (1971).
2.4 ɑ-amylase inhibition activity analysis
The percentage of inhibition activity of the ɑ-amylase enzyme using fermented sheep milk supernatants with 3, 5-dinitrosalicylic acid (DNSA) and soluble starch as a substrate was evaluated (Ademiluyi and Oboh, 2013; Telagari and Hullatti, 2015).
2.5 Evaluating the α-glucosidase inhibitory activity
The percentage of inhibition activity of the ɑ-glucosidase enzyme using fermented sheep milk supernatants utilizing 4-nitrophenyl-D-glucopyranoside (P-NPG) as a substrate was determined (Yamaki and Mori, 2006; Shai et al., 2011).
2.6 Assessment of lipase inhibition
The percentage of inhibition activity of the lipase enzyme using fermented sheep milk supernatants utilizing 4MUO as a substrate was determined (Kurihara et al., 2003; Sergent et al., 2012).
2.7 Assessment of protease activity
The methodology was used to assess the proteolysis using O-phthalaldehyde (OPA). This activity was expressed as mg/mL unit (Hati et al., 2015; Solanki et al., 2017).
2.8 Purification, isolation, and characterization of anti-diabetic and ACE-I peptides
The optimization of growth environments involved initiating cultures with a 2.5% inoculation rate and maintaining incubation at 37°C for 48 h. The assessment of peptide content was conducted using the O-phthalaldehyde method (Solanki et al., 2017).
2.9 SDS-PAGE evaluation
SDS-PAGE as per Carrasco-Castilla et al. (2012) and LaemmLi (1970) was utilized in order to ascertain the molecular masses of specific protein fragments, following Solanki (2016). The examination involved samples obtained from both unfermented and fermented sheep milk, including the 3 kDa and 10 kDa fractions (permeate and retentate) from fermented sheep milk. A 12% separating gel and 4% stacking gel were employed in the analysis.
2.10 2D gel electrophoresis
The refinement process in peptide spots of processed sheep milk water-soluble extracts (WSEs) employed a two-dimensional gel electrophoresis method. This procedure followed Yang et al. (2014), with modifications made to electrical parameters based on the specifications in Panchal et al. (2020).
2.11 First dimension-isoelectric focusing (IEF)
A 125-µg protein sample obtained from fermented sheep milk was employed on a ready IPG strip (7 cm) from Bio-Rad, with a pH range of 3–10. Focusing via isoelectric means was conducted with electricity boundaries detailed by Panchal et al. (2020). Following isoelectric focusing, the strip underwent successive immersion in buffer-I equilibrium (10 min) and equilibrium buffer-II (10 min). A 30-s rinse using Bio-Rad’s 1X TGS buffer was then performed before the strip was exposed to SDS-PAGE for two-dimensional (2D) analysis. The gel for the separating phase was prepared as per LaemmLi (1970) and Carrasco-Castilla et al. (2012).
2.12 Analysis of peptide fractions through high-performance liquid chromatography in reverse phase (RP-HPLC)
An RP-HPLC system (LC-20 Shimadzu, Japan) for evaluating the synthesis of peptides from fermented sheep’s milk was specifically designed for separating distinct peptide peaks. A Column C18 of UMLSil (United States Thermo Fisher Scientific) featuring an analytical column size of 5 µ, 250 mm × 4.6 mm, white pore was employed in a binary gradient RP-HPLC framework for the separation procedure. A 20-µL micro-injector loop (HAMILTON Bonaduz AG, Switzerland) was utilized to inject the specimen. Eluent: A was composed of TFA at 0.01% (v/v) within deionized water, while Eluent: B had an 80:20 ratio deionized water to acetonitrile with the same TFA content. At room temperature, the separation was performed at a 0.25 mL/min flow rate. The peptides were discharged in gradient manner: 0–1 min, 10% B; 1–10 min, 20% B; 10–15 min, 25% B; 15–20 min, 35% B; 20–30 min, 50% B; 30–33 min, 60% B; 33–36 min, 70% B; 36–39 min, 80% B; 39–50 min, and 90%–100% B. The absorption was detected using a Shimadzu SPD-20A UV/Visible Wavelength Detector set at 214 nm. The integration of all peaks facilitated the calculation of the overall peak area (Parmar, 2017).
2.13 Production of peptides using high-performance liquid chromatography in the reverse phase
Peptides were separated from ultra-filtered fractions using the RP-HPLC method obtained from fermented sheep milk with KGL4 Lactobacillus. Inoculated at a 2.5% rate, KGL4 was centrifuged for 30 min at 4193 g force after being incubated for 48 h at 37°C. After centrifugation, an extract of water-soluble fermented sheep milk was isolated and underwent ultra-filtration employing membranes with two different molecular weight cut-offs (3 kDa and 10 kDa). Thus resulted in retentate and permeate fractions and was further analyzed for ACE inhibition (2.3) and antidiabetic assays, including lipase inhibition (2.5), α-amylase (2.4), and α-glucosidase inhibition (2.5)
2.14 Identification and characterization for separated peptides using RPLC/MS
2.14.1 Liquid chromatography
Liquid chromatography was carried out with an Ad hoc column (2.1 mm × 100 mm, 1.5 μm, India) with a sample temperature of 20°C and a column temperature of 40°C. The mobile phases A and B were acetonitrile (ACN) with 0.1% formic acid and water, respectively. In 2D gel, protein spots were isolated and underwent digestion by in-gel trypsin. The resulting peptides that were digested by trypsin were passed through a nylon filter measuring 0.22 µm before injection in 20 µL capacity flowing at 0.3 mL/min. The gradient column elution parameters were optimized based on Parmar (2017), and the procedure was then carried out.
The ABSCIEX QTRAP 4500 and Ekspert ultra LC 100 (Eksigent, United States), together with electron spray ionisation (ESI) interaction, were used for the mass spectrometry study. Recognizing unidentified peptides in fermented sheep milk was accomplished through reverse-phase liquid chromatography mass spectrometry (RPLC-MS), switching from enhanced product ion (EPI) to enhanced mass spectra for scanning. A tailored method was developed for identifying masses within the 350–2,000 dalton range in EMS scan mode, with specific parameters such as a delustering potential (DP) of 80, an electron potential (EP) of 10, and a core energy (CE) of 5500 ESI volts using a rolling-circle energy-ramping function. The EPI scan was configured to identify ions in the range of 100–2,000 daltons employing high collision-induced dissociation (CAD) and maintaining consistent DP and EP values. Criteria for information-dependent acquisition (IDA), involving tracking the top one to three peaks while maintaining a 250 mDa mass tolerance, were applied for mass scanning. Additionally, an isotopic pattern utilizing an enhanced resolution scan detected objects of different masses.
2.14.2 Analysis of peptide identification
The mass spectra produced by LC‒MS were examined utilizing ProteinPilot software to identify peptide sequences. These sequences were subsequently compared with the Anti-Hypertensive Peptides Database (AHTPDB) to confirm ACE inhibitory activity and with BIOPEP to validate antidiabetic activity.
2.15 Anti-inflammatory effects of fermented sheep milk
2.15.1 Cell culture
Cells were procured from the Centre for National Cell Science in Pune, India. DMEM (Dulbecco’s modified Eagle medium) and PBS (phosphate-buffered saline) were sourced through Lonza Bioscience in Switzerland, and P/S (penicillin/streptomycin) was acquired from Gibco, Thermofisher Scientific, in the USA. MP Biomedicals supplied the fetal bovine serum (FBS), and Cusabio Biotech in China provided the lipopolysaccharide (LPS). The ELISA kit for TNF-α, IL-6, and IL-1β was purchased via Elabscience in the United States.
2.15.2 Cell viability
The 264.7 RAW cells were subjected to subculture following a 48-h incubation with DMEM enhanced with 1% P/S and 10% FBS (fetal bovine serum) as per Khare et al. (2020). In accordance with this protocol, 96-well plates were employed, each containing 1 × 105 cells, and placed in a humid incubator at 37°C with 5% CO2 for 16 h. Afterward, the cells were exposed to fermented sheep milk (KGL4) at concentrations of 2, 1, and 0.5 mg/mL for 24 h. The MTT assay was then conducted by including 3-(4, 5-dimethylthiazol-2-yl)-2,5-diphenyltetrazolium bromide @ 0.5 mg/mL. Cells had been kept in darkness for 4 hours with 5% CO2 at 37°C. Formazan crystals were dissolved by adding 0.1 mL of DMSO (dimethyl sulfoxide), and a Tecan Life Science M200 PRO microplate reader was employed to detect at 570 nm.
2.15.3 Production of nitric oxide (NO)
The 264 RAW.7 cells were cultivated for 24 h in accordance with protocols after being deposited in a 48-well plate at a density of 2 × 105 cells per well. Subsequently, macrophages that were fully confluent were subjected to 1 μg/mL lipopolysaccharide (LPS) in combination with three specific Lactobacillus strains (KGL4) used for fermenting sheep milk. The concentrations of these Lactobacillus cultures were 2, 1, and 0.5 mg/mL. After this treatment, the cells were kept in a humidified CO2 incubator for 16 h. Using the Griess reagent, nitrite levels were ascertained, and absorption of optical density at 540 nm was noted. The supernatants that were extracted from the cultures were subjected to cytokine analysis.
2.15.4 IL-6, TNF-α, and IL-1β cytokine measurements
TNF-α, IL-6, and IL-1β levels in the supernatants from cell cultures were quantified using of a commercial ELISA kit as per the manufacturer’s instructions (Elabscience, United States).
2.16 Molecular docking
The methodology for the molecular docking of a specific peptide adhered to the procedure outlined by Singh et al. (2024). In summary, the peptide’s 3D structure was anticipated by employing the AlphaFold Colab tool by Google Deep Mind (Mirdita et al., 2022). The three-dimensional structures of pancreatic α-amylase (1DHK) and angiotensin-converting enzyme (1O86) were obtained from the RCSB PDB database. The protein-active sites were located using the Active Site Prediction server Singh et al. (2011). The chosen peptide served as the ligand, and the respective enzymes were identified as receptors for the Vina AutoDock v1.2.0 docking procedure (Trott and Olson, 2010). Additionally, the docking score for the peptide was predicted via HPEPDOCK 2.0, which was adaptable; blind peptide docking entails rapidly modeling amino acid configurations and then worldwide binding orientation sampling (Zhou et al., 2018).
2.17 Statistical analysis
Analysis was executed using a CRD (completely randomized design) as outlined by Steel and Torrie (1980). The importance of every parameter’s impact on the particular trait was evaluated @ a 5.0% significance level. Software, Inc. GraphPad Prism 8.0 (United States: La Jolla, CA) was utilized for data analysis. One-way ANOVA was used for multiple group comparisons and then post hoc analysis using Tukey. Statistical significance was considered at p ≤ 0.05. The entire set of experiments was replicated thrice.
3 Results and discussion
3.1 ACE-inhibitory activity of fermented sheep milk
The ACE-inhibitory activity of fermented sheep milk utilizing the Limosilactobacillus fermentum (KGL4) culture is shown in Figure 1. A significant increase in ACE inhibition activity (p < 0.05) was noted throughout the incubation period. The fermented sheep milk demonstrated ACE-inhibitory effects ranging from 8.91% ± 2.33% to 74.82% ± 1.2% within the 0–48-h time frame at 37°C (Figure 1). The highest ACE inhibitory activity, reaching 74.82% ± 1.2%, was noticed following a 48-h incubation period at 37°C, surpassing the inhibition levels recorded at other incubation times.
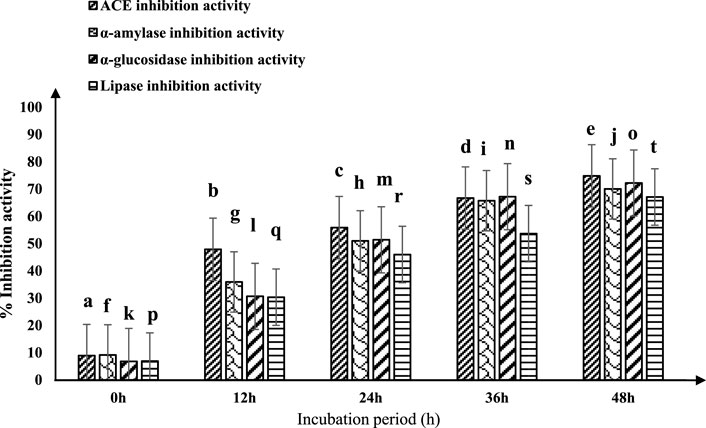
Figure 1. ACE-inhibitory and antidiabetic activities of fermented sheep milk using KGL4 *ACE-inhibitory and antidiabetic activity (%) mean ± SD of three replicate studies (n = 3); values with different superscripts differ significantly (p < 0.05).
The ACE inhibition (ACE-I) efficacy of fermented sheep milk displayed significant variability across different incubation durations, indicating a notable rise in ACE enzyme inhibition with prolonged incubation times (p < 0.05). This trend aligns with observations by Solanki et al. (2017). Fermented milk generated by diverse Lactobacillus species is known for its substantial ACE-I activity exceeding 50% (Hati et al., 2018; Wu et al., 2019). Changes in ACE-I activity observed between lactic acid bacteria are linked to their protease function in fermented milk, and specific components of lactic acid bacteria proteolysis play a role in influencing this activity (Chen et al., 2015).
Mudgil et al. (2023a) explored the inhibitory activity of the angiotensin-converting enzyme (ACE-I) of sheep milk which was fermented with five probiotic bacteria under refrigerated conditions for 0 , 7 , and 14 days. The ACE-IC50 values for sheep milk fermented with various probiotic microorganisms—L. fermentum, L. pentosus, L. plantarum ssp. Argentoratensis, E. hirae, and P. pentosaceus—were recorded, respectively, as 842.0, 1079, 1079, 1060.5, and 2071.4 µg protein equivalent per milliliter at 0 days, 668.3, 703.0, 659.8, 659.8, and 905.3 µg protein equivalent per milliliter after 7 days, and 301.0, 445.4, 430.6, 455.8, and 445.0 µg protein equivalent/mL after 14 days of storage. The ACE-IC50 values observed after 24 h, 7 days, and 14 days of fermentation ranged from 842.0 to 2071.4, 842.0 to 2071.4, and 659.8 to 905.3 protein equivalent/mL, respectively, suggesting a substantial increase in ACE inhibitory potential with prolonged incubation. This research supports our findings and also highlights a consistent upward trajectory in ACE-I activity with extended fermentation periods. Balthazar et al. (2019) conducted trials using semi-skimmed sheep milk blended with strawberries, contrasting it with yogurt starter cultures that included Lactobacillus plantarum strain (CECT 8328) possessing promising probiotic characteristics. They also integrated commercial prebiotic components into five distinct formulations—SMB1: yogurt-flavored drink; SMB2: possible beverage with probiotic culture; SMB3: a possible beverage with probiotic culture and inulin; SMB4: a potato starch beverage with a possible probiotic bacterium; SMB5: possible probiotic beverage made from potato starch cultures. These outcomes revealed an augmentation in the antihypertensive efficacy of all five prebiotic-enriched products during the initial 0–30 days of storage, with SMB5 demonstrating the most substantial antihypertensive effect (up to 60%). Balthazar et al. (2019) concluded that the combination of probiotics and prebiotics in sheep milk showcased ACE-I activity (60%). Nonetheless, our investigation proposes that the KGL4 culture, when used independently in fermentation, displayed a better capacity for peptide release than these observations.
3.2 Fermented sheep milk anti-diabetic activity
The efficacy of fermented sheep milk in producing antidiabetic effects is shown in Figure 1. Significant variations (p < 0.05) in antidiabetic outcomes were observed during the incubation period within the 0–48-h interval. The inhibitory actions against crucial enzymes associated with diabetes—α-amylase, α-glucosidase, and lipase—ranged from 9.16% ± 2.39% to 70.02% ± 1.48%, 6.77% ± 1.88% to 72.19% ± 0.42%, and 6.90% ± 1.93% to 67.08% ± 1.05%, respectively. Following 48 h of incubation at 37°C, fermented sheep milk showcased its most potent antidiabetic effects, achieving a 70.02% inhibition of alpha-amylase, 72.19% inhibition of alpha-glucosidase, and 67.08% inhibition of lipase. Larosa et al. (2021) observed extended incubation periods to enhance the potential antidiabetic effects of sheep milk kefir. Their research involved preparing six kefir formulations from sheep milk, incorporating different sugars—Brown (KIII), fructose (KIV), sucrose (KI), demerara (KII), coconut sugar (KV), and honey (KVI). This formulation underwent a 28-day fermentation process using a combination of Lactococcus lactis spp. lactis biovar diacetyl lactis, Lactobacillus brevis, Lactococcus lactis ssp. lactis, Leuconostoc spp., and Saccharomyces bayanus. The primary focus of the investigation centered on evaluating the α-glucosidase, α-amylase, and ACE-inhibitory activity in the samples of sheep milk kefir. Their findings indicated variations in ACE inhibitory activity (11.6%–49.2%), α-amylase inhibition (19.3%–56.7%), and α-glucosidase inhibition (24.3%–39.3%). Notably, incorporating non-traditional sugars (KII-KVI) improved the functional characteristics of fermented milk. This led to a noteworthy rise in α-amylase inhibition (18%–37.4%) and ACE inhibitory action (27.5%–37.6%) (p < 0.05) in contrast to the mixture containing sucrose. The most significant enhancements in functional properties were observed in kefir formulations containing honey (KVI) and coconut sugar (KV) for the ACE-inhibitory properties (p < 0.05), as well as kefir with fructose (KIV), brown sweets (KIII), and demerara sweets (KII) to inhibit α-amylase (p < 0.05). Furthermore, only KIII, KIV, and KV formulations demonstrated a noteworthy increase in α-glucosidase inhibition (p < 0.05). Balthazar et al. (2019) investigated fermented sheep milk and strawberry beverages utilizing Lactobacillus plantarum strain (CECT 8328), known for its possible probiotic qualities. The inhibitory effects of α-glucosidase and α-amylase at both 0 and 30 days of storage were assessed. The results revealed a significant increase of around 7% and 8%, respectively, in inhibition after 30 days compared to the initial measurements at 0 days. Among the formulations, SMB5, recognized as a potential probiotic culture potato starch beverage, demonstrated the highest α-amylase inhibition (>65%), while SMB4 exhibited more effective α-glucosidase inhibition at 36.13%. These findings are consistent with our own results, providing support for the observed improvement in the inhibition of α-glucosidase and α-amylase with extended incubation time.
Khakhariya et al. (2023) investigated the potential antidiabetic consequences of fermenting camel and buffalo milk using Lacticaseibacillus paracasei, MG027695 (M11) during incubation periods ranging from 12 to 48 h. The inhibition of α-glucosidase, α-amylase, and lipase with fermented buffalo milk varied 33.09%–59.95%, 57.09%–79.14%, and 40.75%–63.71%, respectively. Similarly, fermented camel milk exhibited inhibitory activities (inhibition of α-glucosidase, α-amylase, and lipase) of 46.27%–64.55%, 61.43%–81.66%, and 50.15%–69.83%, respectively. Notably, after incubation at 37°C for 48 h, buffalo and camel milk both demonstrated very robust effects against diabetes. Kinariwala et al. (2020) studied the fermented milk combined alongside L. fermentum (M2) and L. fermentum (M7) for 24 h at 37°C, reporting lipase inhibitory activity of 24.24% for M2 and 18.99% for M7. While their observations were made at 24 h, our study extended the incubation period to 48 h, resulting in higher lipase inhibition activity due to the prolonged incubation times with strong proteolytic activity. This is consistent with the observed increase in α-amylase, α-glucosidase, and lipase inhibitory action with longer incubation.
3.3 Optimizing fermented sheep milk for the production of peptides
The evaluation of enzymatic activity was carried out using O-phthalaldehyde (OPA) as a substrate in a spectrophotometric assay at 340 nm, as per Solanki et al. (2017). To optimize peptide production while sheep’s milk is fermenting, a KGL4 strain culture of Lactobacillus was employed. Different inoculation amounts (1.5%, 2.0%, and 2.5% v/v) of this specific strain were introduced into heat-treated milk from sheep and then cultivated for 0, 12, 24, 36, and 48 h at 37°C. Notable variations (p < 0.05) within proteolytic (OPA) activity were noted with respect to both incubation time and inoculation rate. The enzymatic activity demonstrated an increase with prolonged incubation periods at each inoculation rate (Figure 2). Maximum proteolytic (OPA) activity was recorded following a 2.5% inoculation rate for 48 h of incubation (9.88 ± 0.11 mg/mL), exceeding the rates of 1.5% (8.20 ± 0.34 mg/mL) and 2.0% (9.38 ± 0.27 mg/mL).
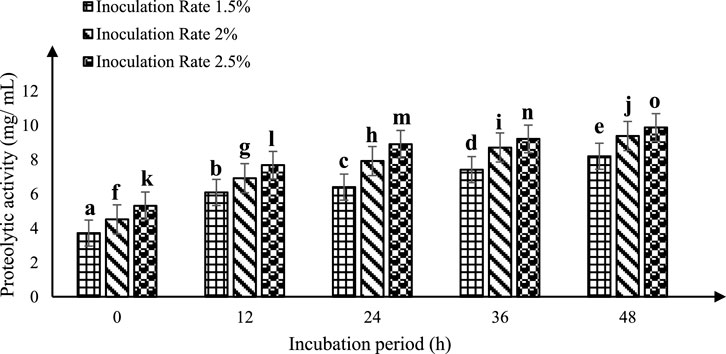
Figure 2. Proteolytic activity (mg/mL) of fermented sheep milk using KGL4 *Mean ± SD of three replicates; each sample was subjected to three replicates; values with different superscripts differ significantly (p < 0.05).
Ashokbhai et al. (2022a) explored the proteolytic capabilities of sheep milk using the OPA method. Additionally, they investigated the influence of fermenting sheep milk with Lactiplantibacillus plantarum (KGL3A) across different conditions, including incubation periods of 12, 24, 36, and 48 h, and rates of 1.5%, 2%, and 2.5% culture inoculations. The observed action of proteolysis ranged from 6.10 mg/mL (at 1.5% inoculation following 12-h incubation time) to 10.40 mg/mL (at 2.5% inoculation following 48-h incubation time ). Their findings revealed a significant augmentation in proteolytic activity as the incubation time prolonged (p < 0.05). Additionally, the level of proteolytic activity demonstrated variability based on the inoculation rate (1.5%, 2.0%, and 2.5%). Importantly, a noteworthy increase in proteolytic activity was noted after 48 h for all inoculation rates, compared to the 12-, 24-, and 36-h incubation periods. Parmar et al. (2020) investigated the proteolytic capabilities of two strains of Lactobacillus—L. fermentum (TDS030603) (MTCC 25067) (LF) and L casei (KR732325) (NK9)—in the context of Surti Indian breed goat milk (Capra aegagrus hircus). They observed a significant enhancement (p < 0.05) in the proteolytic activity of LF and NK9 in goat milk across various incubation durations (0, 6, 12, 24, and 48 h) and various rates of inoculation (1.0, 1.5, and 2.0%). Remarkably, both LAB cultures—LF (9.709 mg/mL) and NK9 (7.598 mg/mL) —exhibited substantial proteolytic effects after a 48-h incubation period. Our findings align with these results, highlighting that proteolytic activity is positively influenced by both incubation time and inoculation rate. Our investigation specifically revealed the maximum activity of proteolysis at 48 h, employing a rate of 2.5% inoculation. Moreover, the action of proteases of KGL4 also demonstrated a significant increase (p < 0.05) with an extended incubation times (0, 12, 24, 36, and 48 h), reaching its peak (9.88 ± 0.11 mg/mL) at 48 h with a 2.5% inoculum rate. Collectively, these studies affirm the consistent trend that proteolytic activity tends to rise with extended incubation time and is highest at an inoculation rate of 2.5% following a 48-h incubation period.
3.4 Assessment of the ACE-inhibitory and antidiabetic qualities of ultra-filtered WSE fractions from fermented sheep milk using RP-HPLC analysis
The present study included examining retentate and permeate samples resulting from the ultrafiltration of peptides derived from fermented sheep milk, employing molecular weight cut-offs of 3- and 10-kDa membranes. Figure 3a of unfermented sheep milk and Figures 3b–f of fermented sheep milk display RP-HPLC chromatograms obtained under optimized growth conditions (2.5% inoculation rate, up to 48 h, at 37°C). Table 1 presents a characterization of peptide fractions (< 3 kDa, > 3 kDa, < 10 kDa, > 10 kDa) derived from sheep milk post-fermentation, utilizing RP-HPLC.

Table 1. Characterization of <3 kDa, >3 kDa, <10 kDa, and >10 kDa peptides generated from fermented sheep milk measured using RP-HPLC.
Figure 3A illustrates the RP-HPLC chromatogram of non-fermented sheep milk, indicating a significant increase in peptide production in fermented samples compared to their non-fermented state. The KGL4 peaks, found in the 3–10 kDa range, especially displayed the highest number of peaks, with retention times of 24.08–57.52 min (Table 1). Non-fermented milk contained intact proteins, while fermented milk contained diverse protein fragments due to the strong proteolytic action of the KGL4 strain. Some fragments were also found in the control, possibly due to heating or inherent proteolytic enzymes in sheep milk. The fermented sheep milk’s 3 kDa permeate sample displayed the highest levels of hypoglycemic and ACE-inhibiting activities in contrast to membranes with different cut-off values. Specifically, the 3-kDa permeate exhibited optimal ACE-inhibitory (76.69% ± 2.11%), α-amylase inhibitory (75.69% ± 3.49%), α-glucosidase inhibitory (66.27% ± 2.69%), and lipase inhibitory (71.67% ± 1.75%) activities (Figure 4). ACE-I peptides, typically with a weight below 3 kDa and ranging from 6 to 16 amino acids, play a vital part. Furthermore, a peptide's effectiveness in addressing diabetes is influenced by its alanine sequence and place in the peptide order. Amino acids with a molecular weight below 3 kDa—including proline, phenylalanine, leucine, and glycine—exhibited sharp α-amylase inhibition, possibly due to the inhibitory effects of these specific amino acid residues. Additionally, Mu et al. (2023) emphasized that peptides containing two to eight amino acid compounds, particularly those with more amino acids that are hydrophobic such as proline and leucine, demonstrated increased inhibitory effects on α-glucosidase.
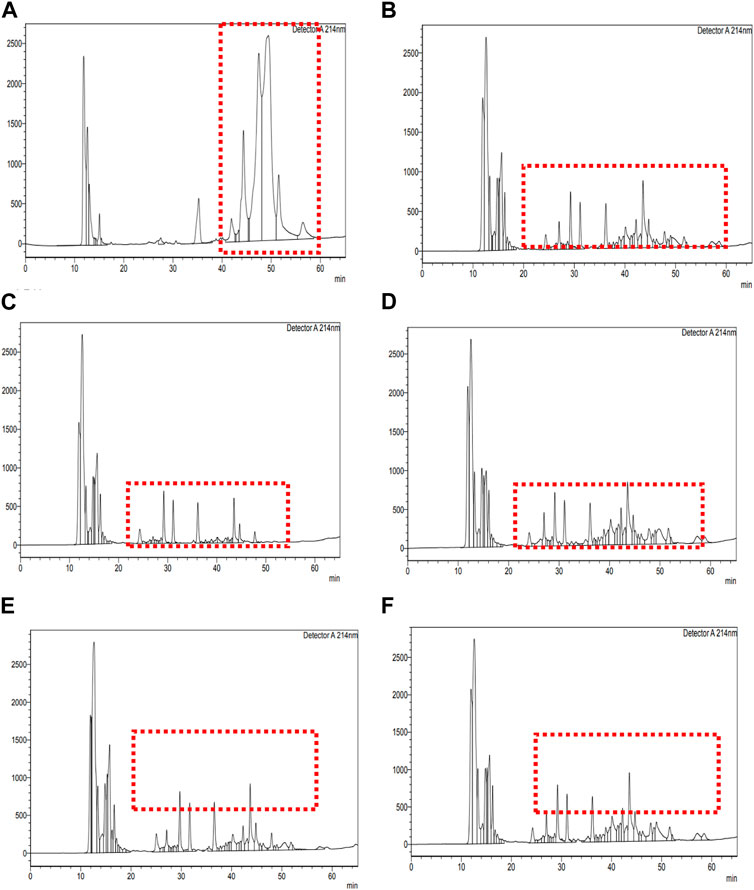
Figure 3. RP-HPLC chromatogram of unfermented sheep milk. RP-HPLC chromatograms of fermented sheep milk with KGL4 culture and fractionated components: (A) unfermented sheep milk; (B) fermented sheep milk using KGL4 culture; (C) 3 kDa permeate from fermented sheep milk using KGL4 culture; (D) 3 kDa retentate from fermented sheep milk using KGL4 culture; (E) 10 kDa permeate from fermented sheep milk using KGL4 culture; (F) 10 kDa retentate from fermented sheep milk using KGL4 culture.
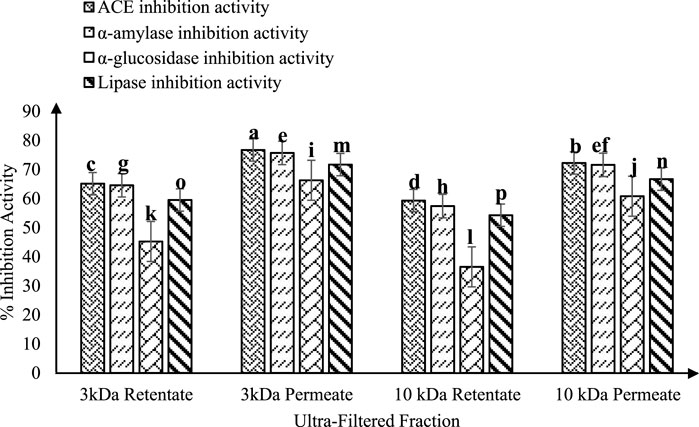
Figure 4. ACE-inhibitory and antidiabetic activities of ultra-filtered fractions (3 and 10 kDa permeate and retentate) from fermented sheep milk (KGL4). *Values with different superscripts differ significantly (p < 0.05), ACE-inhibitory and antidiabetic activities (%) Mean ± SD of three replicate experiments (n = 3).
According to Rubak et al. (2022), ACE inhibitory effects are observed in skimmed goat milk (11% w/v) fermented with L. lactis ssp. lactis BD17 (2% v/v) for up to 48 h. The study found significant ACE inhibition in the supernatant—portions of the filtered fermented milk that are >3 kDa and <3 kDa. The ACE inhibition percentages for the supernatant and the >3 kDa and <3 kDa fractions were 60.33%, 24.57%, and 57.31%, respectively. In particular, no statistically significant difference was observed (p > 0.05) within ACE-I action among the portion of 3 kDa and the aqueous phase. The research also identified an ACE-I peptide with a molecular weight of approximately 657–1,994 Da/2 kDa and composed of six–eighteen peptides. Parmar et al. (2018) investigated fermented goat milk using L. fermentum TDS030603 (LF) (MTCC 25067) and L. casei KR732325 (NK9). They utilized centrifugation and ultrafiltration through membranes of 3, 5, and 10 kDa to obtain retentate and permeate samples. The ACE-I activity percentages for NK9 were 36.26%, 22.00%, and 25.16% in the 3 kDa, 5 kDa, and 10 kDa permeate samples, respectively, while LF exhibited 14.40%, 22.28%, and 31.49% ACE-I activity, respectively. In the retentates of 3, 5, and 10 kDa, NK9 showed ACE-I action of 43.58%, 30.88%, and 33.19%, and LF demonstrated 29.46%, 33.62%, and 37.77% ACE-I activity, respectively. These results suggest that a molecular weight cut-off of 3 or 5 kDa could be deemed appropriate. Remarkably, their study substantiates our findings, indicating that the most potent ACE-I peptides are present in the molecular weight fraction below 3 kDa. Shirkhan et al. (2023) examined the effects of LAB strains (L. paracasei ssp. paracasei, L. delbrueckii, and L. helveticus) on UHT milk. These LAB strains were observed in order to diminish the efficacy of α-amylase and α-glucosidase enzymes in milk that was fermented and treated with fractions of 3 kDa, 5–10 kDa, and >10 kDa. While all fractions from L. helveticus displayed inhibiting impacts on α-glucosidase and α-amylase enzymes, certain peptide fractions from different strains did not demonstrate enzyme inhibition. The assessment of the antidiabetic potential of bioactive peptides relied on the IER (inhibitory efficiency ratio), suggesting that the lowest molecular weight peptide portions exhibited the most pronounced hypoglycemic effects. Shirkhan et al. (2023) underscored the noteworthy impact of fraction size on enzyme inhibition (p < 0.05) with the >10 kDa peptide fraction. Lower molecular-weight peptide fractions showed increased proteolysis and antidiabetic properties and displayed the lowest inhibition rate for both enzymes. Lower molecular-weight peptide fractions showed increased proteolysis and antidiabetic properties (p < 0.05). These findings corroborate the current results and suggest that anti-diabetic effects are more potent in low molecular-weight peptide fractions than in higher molecular-weight proteins.
3.5 Analysis of SDS-PAGE from fermented sheep milk
SDS-PAGE analysis was conducted on whole sheep-milk extracts cultured and fermented using the KGL4 strain. A low molecular-weight ladder ranging from 10 to 315 kDa was utilized. A comparison of SDS-PAGE profiles between unfermented and fermented sheep’s milk revealed an increased number of protein bands in the latter, attributed to the LAB’s robust proteolytic activity. Figure 5 illustrates that sheep milk without fermentation exhibited bands of protein of 26–315 kDa in the SDS-PAGE. However, sheep milk fermented with KGL4 displayed bands of 10–32 kDa and 51–70 kDa regions. Ashokbhai et al. (2022b) explored Lactobacillus plantarum (KGL3A) strains obtained from fermented and unfermented sheep milk through SDS‒PAGE analysis. Their results revealed that the unfermented sheep milk displayed larger protein bands than the fermented milk. The lactic acid bacteria action resulted in the breakdown of these protein bands into smaller peptides. In unfermented sheep milk, the bands of protein ranged between 10 and 42 kDa while, in fermented sheep milk, the bands had a length of 10–70 kDa. Sansi et al. (2023) utilized SDS‒PAGE to analyse goat milk casein and whey protein. They identified major whey proteins such as lactoferrin, serum albumin, immunoglobulin (IgG), α-lactalbumin (around 14 kDa), and β-lactoglobulin (ranging from 14 to 25 kDa) as associated with specific bands. Additionally, in bands of 25–75 kDa, the three main proteins in casein were found to be α, β, and κ-casein. However, our study observed protein bands ranging from less than 10 kDa to 124 kDa in length. Parmar et al. (2020) investigated the properties of whey protein concentrates from goat milk subjected to fermentation using L. fermentum (M5) (KU366365) and L. paracasei (M16) (KU366368). Compared to unfermented goat milk, SDS‒PAGE analysis indicated a decline in protein bands in fermented goat milk, attributed to the lactic acid bacteria’s proteolytic activities. Unfermented goat milk displayed bands of protein 75‒11 kDa. Both samples of fermented goat milk (M5 and M16) as well as goat milk without fermentation exhibited peptides of low molecular weight of about 25 kDa, with only M16 containing 11 kDa. The study’s findings supported the conclusion that lactic fermentation resulted in reduced native protein size due to protein breakdown.
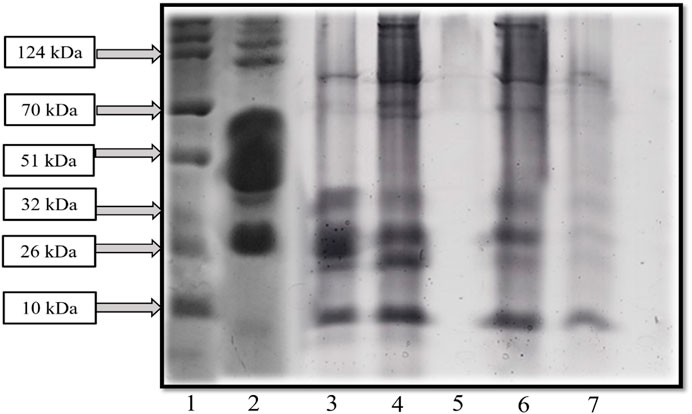
Figure 5. SDS-PAGE exhibited the protein profile of fermented sheep milk with KGL4 culture (1: protein ladder, 2: unfermented sheep milk, 3: fermented sheep milk, 4: 3 kDa retentate, 5: 3 kDa permeate, 6: 10 kDa retentate, and 7: 10 kDa permeate).
3.6 Two-dimensional gel electrophoresis of fermented sheep milk
Two-dimensional (2D) electrophoresis separation of proteins in fermented sheep milk employed an IPG strip with a pH range of 3–10, according to molecular weight and isoelectric point. In the examination of the KGL4 strain fermented with sheep milk, 36 unique spots were identified on the 2D gel electrophoresis. The fragments of protein produced by the KGL4 strain displayed a molecular weight distribution between 10 and 51 kDa, with the concentration of spots primarily falling within 10 kDa–32 kDa (Figure 6). The patterns observed in the figures suggest the occurrence of protein degradation and the presence of smaller molecular weight peptides during the lactic acid bacteria-mediated fermentation of sheep milk. Ashokbhai et al. (2022b) conducted a study that evaluated the protein composition of sheep milk fermented with Lactobacillus plantarum (KGL3A). Utilizing 2D gel electrophoresis, they identified 13 protein spots ranging in size from 10 to 70 kDa. The results indicated a reduction in protein size post-fermentation, attributed to proteolysis, with the observed spots predominantly falling within the smaller molecular weight range. This trend was consistent across all three cultures investigated in their study. Panchal et al. (2020) identified 39 spots in 2D electrophoresis on gels for Lactobacillus fermentum (M4) fermented proteins from goat milk. In our investigation, all three Lactobacillus cultures exhibited the maximum number of spots within the atomic molecular weight range of 10–32 kDa. Shukla et al. (2022) used L. plantarum KGL3A to investigate the amino acid content of fermented camel milk, with gel electrophoresis (2D) revealing 31 spots with atomic weights of 10 to 100 kDa, attributed to the proteolytic action of L. plantarum. Notably, the protein spots generated by KGL4 and M11 ranged from 10 to 51 kDa, while KGL3A exhibited a broader spectrum of 10–70 kDa. In their study on kefir proteomics, Rubak et al. (2020) employed 2D electrophoresis and identified protein spots ranging from 14 to 97 kDa, with a predominant presence in the 14–31 kDa range. Both investigations substantiate our findings, supporting the inference that the fermentation by lactic bacteria that produces lactic acid results in the formation of smaller molecular-weight proteins from milk protein.
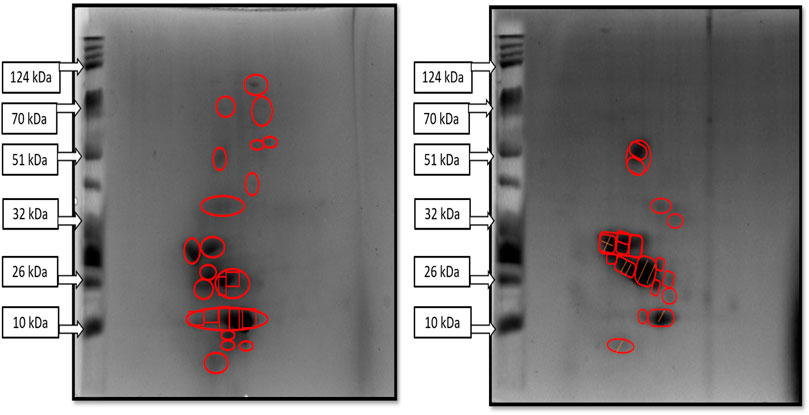
Figure 6. 2D gel electrophoretic image of sheep milk fermented with KGL4 culture. Two images showing different spots of purified protein samples in 2D gel images obtained from fermented sheep milk with KGL4 culture; these spots were injected in LCMS for peptide sequencing after in-gel trypsin digestion.
3.7 Identification of purified peptides and characterisation employing RP-LC/MS
The analysis of 2D spots from the fermentation of sheep milk produced with the KGL4 strain resulted in the detection of 36 spots. Subsequent trypsin digestion and RP-LC/MS study were performed on individual spots for peptide identification. Figure 7 illustrates ion chromatogram with protein score of the whole trypsin-digested 2D spots acquired through the fermentation of sheep milk with the KGL4 culture. Peptide sequences such as ALMGALIK and NMAIHPR from αs2-casein, DMPIQAFLLYQEPVLGPVR and GPFPILV from β-casein, FFSASCVPCVDR from lactotransferrin, LDQWLCE from α-lactalbumin, NMAIHPR through αs2-casein, SCQDQPTAMAR, and SPAQTLQWQVLPNAVPAK from κ-casein, were found based on peptide ranker scores (>0.45) and toxicity (Table 2).
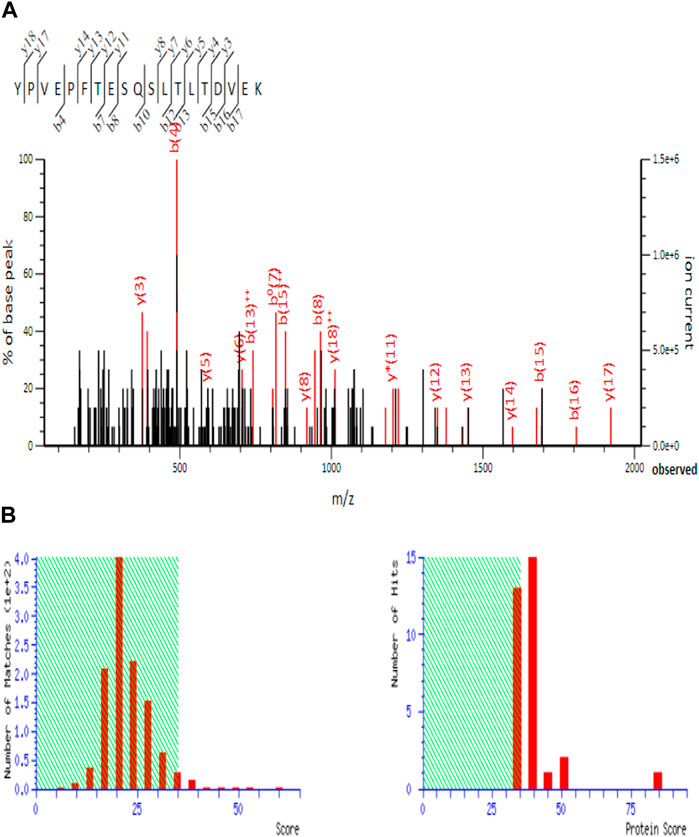
Figure 7. Total ion chromatogram of 2D spot obtained from fermented sheep milk with KGL4 culture (EMS to EPI scan in LC-MS). (A) MS/MS spectrum of the peptide sequence inspected in MASCOT database; (B) protein score of peptide sequence (found in SwissProt software).
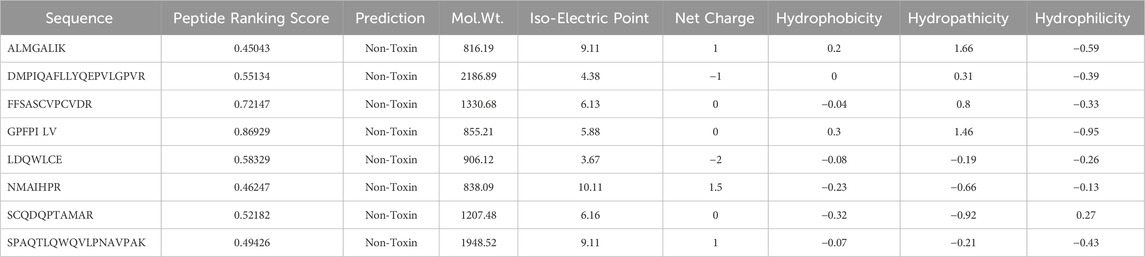
Table 2. Amino acid sequence obtained from 2D-PAGE of fermented sheep milk with peptide ranking score and physico-chemical properties (in silico analysis).
The peptides DMPIQAFLLYQEPVLGPVR, GPFPILV, QAFLLYQEPVLGPVR, and SCQDQPTAMAR identified in sheep milk fermented with KGL4 exhibited higher peptide ranker scores in Table 3. These sequences have similarities with peptides such as LGP, AF, GP, PT, LLYQEP, YQEPVL, LLYQQPV, AVP (Iwaniak and Dziuba, 2009), AFP, AVPYPQR (Wu et al., 2006), VLGP (Gutiez et al., 2013), GPVGPA (Oshima et al., 1979), LLYQQPV (Gutiez et al., 2013), YQEPVLGPVRGPF (Pihlanto and Makinen, 2013), GPFPILV (Zhang et al., 2016), VR (Gomez-Ruiz et al., 2007), VYPFPG (Wakai and Yamamoto, 2012), YQQPVLGPVR (Meisel and Schlimme, 1990), and FP and AVP (Hernandez-Ledesma and Hsieh, 2013) from the AHTPDB databases. This similarity further confirms their ACE inhibitory activity. In Table 4, the sequences QAFLLYQEPVLGPVR, DMPIQAFLLYQEPVLGPVR, GPFPILV, and HQGLPQEVLNENLLR were identified in sheep milk fermented with KGL4 after trypsin digestion. These sequences partially corresponded to antihypertensive peptide fractions such as QEPVLGPVRGPFP and VR (Gong et al., 2020), RCMAFLLSDGAAAAQQLLPQY (Siow and Gan, 2016), LPQNIPPL and LPQ (Uenishi et al., 2012), GPFPILV (Zhang et al., 2016), VPITPT (Uenishi et al., 2012), and WL (Nongonierma et al., 2014) in the BIOPEP database, providing confirmation of their antidiabetic activity.
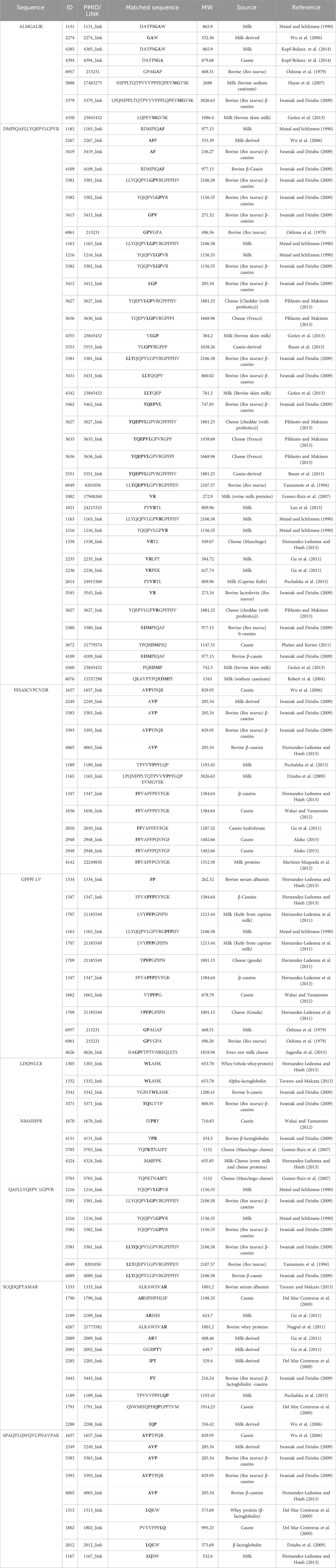
Table 3. Amino acid sequence with antihypertensive activity derived from 2D-PAGE of fermented sheep milk (KGL4) matched with the AHTPDB database.
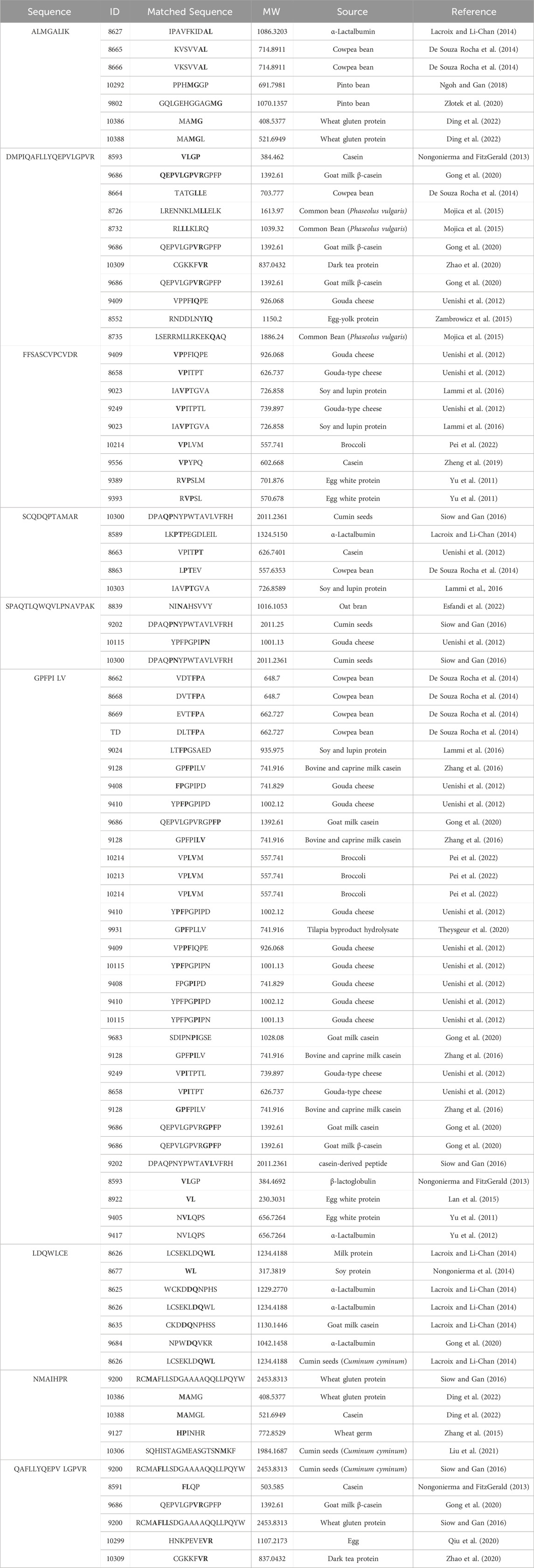
Table 4. Amino acid sequence with antidiabetic activity derived from 2D-PAGE of fermented sheep milk (KGL4) matched with the BIOPEP database.
3.8 The inhibitory effects of fermented sheep milk on RAW macrophage cells
3.8.1 Cell viability
The 264.7 RAW cells underwent MTT analysis to evaluate cell viability after being treated with sheep milk fermented using the Lactobacillus culture, Limosilactobacillus fermentum (KGL4). The experiment comprised six different concentrations of fermented sheep milk (0.5, 1, 2, 4, 6, and 8 mg/mL) along with a control group (without cultures). The findings depicted in Figure 8A reveal a decline in cell viability rising from 0.5 to 8 mg/mL in concentration. The KGL4 strain especially demonstrated the non-cytotoxic effects from 0.5, 1, and 2 mg/mL concentrations, resulting in nearly 100% maximum viability of cells. However, at higher concentrations (4, 6, and 8 mg/mL), cytotoxicity escalated, leading to a reduction in the viability of cells.
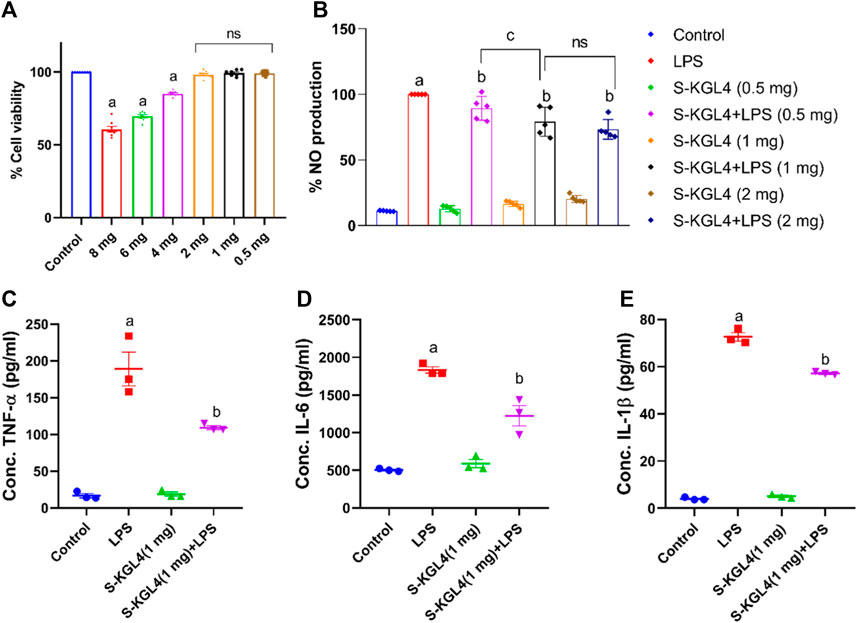
Figure 8. Effect of S-KGL4 on (A) cell viability (MTT assay) of RAW 264.7 cells, (B) NO production in S-KGL4, (C) TNF-α, (D) IL-6, (E) IL-1β measured in the supernatants of LPS-stimulated RAW 264.7 Cell data are presented as mean ± SEM; n = 3 and evaluated by one-way ANOVA followed by Tukey’s post hoc test. * relative to the control, # relative to the LPS, LPS: lipopolysaccharide. p-value for # is 0.0124, 0.0069, and <0.0001 in TNF-α, IL-6, and IL-1β, respectively.
3.8.2 Impact of cultured sheep milk upon pro-inflammatory cytokine and nitric oxide (NO) percentages in RAW 264.7 cells
Considerable production in inflammatory mediator nitric oxide occurs in RAW 264.7 cells induced with LPS. TNF-α, IL-6, and IL-1β are key contributors to the reaction of inflammation and the beginning of inflammation and are classified as pro-inflammatory cytokines. This research explores the influence of different concentrations of S-KGL4-fermented sheep milk on 264.7 RAW cells, assessing both the nitric oxide percentage and its impact on pro-inflammatory cytokines. As depicted in Figure 8B, there was a significant rise in nitrite levels observed in the LPS group, but this increase was effectively alleviated in the group receiving fermented sheep milk along with LPS treatment. The group treated with only fermented sheep milk exhibited the lowest nitrite production, nearly comparable to the control group. This finding implies that the generation of peptides via fermented sheep milk does not trigger any harmful effects on 264.7 RAW cells. The results presented in Figures 8C–E illustrate the impact of fermented sheep milk at a 1 mg/mL concentration on TNF-α, IL-6, and IL-1β pro-inflammatory cytokines. Investigation showed a noteworthy decline in the excessive manufacturing of TNF-α, IL-6, and IL-1β triggered via activation with LPS when using KGL4-fermented sheep milk. The values for S-KGL4-fermented sheep milk closely resembled those of the control, suggesting a noteworthy reduction in pro-inflammatory cytokine levels. In Khakhariya et al. (2023), 264.7 RAW cells underwent 24-h of incubation. Fully matured macrophages were exposed to lipopolysaccharide (LPS) at a concentration of 1 g/mL in a combination of Lacticaseibacillus paracasei (M11, MG027695) and Saccharomyces cerevisiae (WBS2A, MG101828). These bacteria were fermented with, respectively, buffalo and camel milk. Following this, the cells were cultured about 16 h in a CO2 incubator with controlled humidity. The study findings highlight the significance of the anti-inflammatory response in the context of inflammatory diseases. Camel and buffalo milk fermented with M11 and WBS2A shows promise as a potent source of anti-inflammatory peptides, demonstrated by a decrease in the production of cytokines associated with inflammation. These results indicate that the fermented sheep milk’s inflammatory properties were reduced due to the generation of bioactive compounds such as short-chain fatty acids and bioactive peptides during the fermentation process. Sansi et al. (2023) investigated the potential anti-inflammatory attributes of peptides obtained from CPH (casein protein hydrolysates) and WPH (whey protein hydrolysates) in combination with lipopolysaccharides (LPS). Within the dosage range of 50–550 g/mL, both CPH and WPH peptide fractions demonstrated no harmful effects on the cell line HT-29. The viability of the cells remained high for both types of peptides, showing survival rates of up to 80%, and no discernible statistically significant variations were noted. However, our outcomes indicate that the WPH and CPH peptide fractions exhibited moderate cytotoxicity while maintaining substantial cell selectivity in our study. Additionally, our examination of fermented sheep milk revealed a more potent anti-inflammatory impact on cells aimed at mitigating excessive production of inflammatory cytokines and mediators. Importantly, KGL4 did not exhibit any inflammatory effects in our assessment.
3.9 CLSM (confocal laser scanning microscopy) and particle size of fermented sheep milk
Figure 9 demonstrates the use of CLSM (confocal laser scanning microscopy) to examine the micro-organization of unfermented sheep milk (UFM), specifically focusing on aspects such as the protein network, peptide binding, and overall composition. When analyzing KGL4 strain-produced water-soluble extracts using fermented sheep’s milk (FSM) and its fractions, the application of fluorescein dye highlighted proteins in a green coloration. Notably, the UFM sample reveals the existence of larger particles, indicative of a native protein structure, in contrast to FSM, where proteins undergo degradation into peptides during the fermentation process. Additionally, FSM exhibits more intricate protein structures and aggregated larger protein formations. This phenomenon is attributed to the unique capability of the KGL4 strain of the Lactobacillus culture to convert proteins into diverse polymers throughout the fermentation process. The permeate and retentate specimens derived from FSM subjected to membrane treatment demonstrate molecular weight cut-offs of 3 and 10 kDa. The identification of smaller protein structures in the 3 kDa permeate sample implies the existence of diminutive peptides in the FSM sample following treatment with the 3 kDa membrane, in contrast to the 3 kDa retentate, 10 kDa permeate, and 10 kDa retentate models. No substantial distinctions were noted in FSM samples with molecular weights exceeding 3 kDa but less than 10 kDa. Nevertheless, the 10 kDa permeate sample exhibits larger particles than samples treated with other cut-off membranes. In Figure 10, it is clear that unfermented sheep milk (USM) exhibited greater particle dimensions than fermented sheep milk (FSM). This difference is attributed to the strong proteolytic activity of KGL4. As a result, smaller particles were observed in the 3 and 10 KDa permeates of FSM, contrasting with the 3 and 10 KDa retentates after the fermentation process. Ningtyas et al. (2021) studied the effect of fermentation in soymilk and yogurt through CLSM and particle size analysis. Fermented yogurt culture undergoes protein coagulation due to lactic acid production and enzymatic activity, forming a coagulated mass and significant observable changes in protein structures through CLSM. The fermented dairy protein network formed a looser protein network with a higher number of pores (Boeck et al., 2021). The microstructural analysis of yogurt using a different culture for fermentation showed significant differences between samples fermented by different cultures. These findings emphasize the influence of fermentation conditions and microbial strains on the final product’s microstructure in CLSM images (Nguyen et al., 2023).
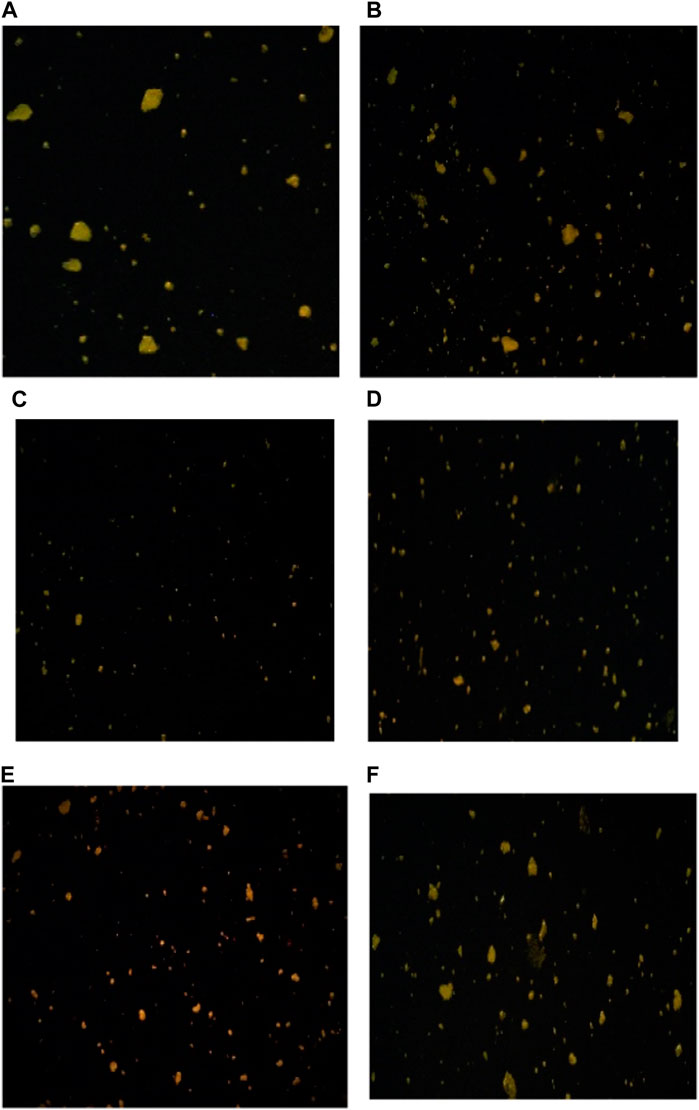
Figure 9. CLSM images showing the microstructure of fermented sheep milk using KGL4 strain. (A) Unfermented sheep milk sample, (B) fermented sheep milk sample, (C) <3 kDa permeate sample, (D) >3 kDa retentate sample, (E) <10 kDa permeate sample, and (F) >10 kDa retentate sample.
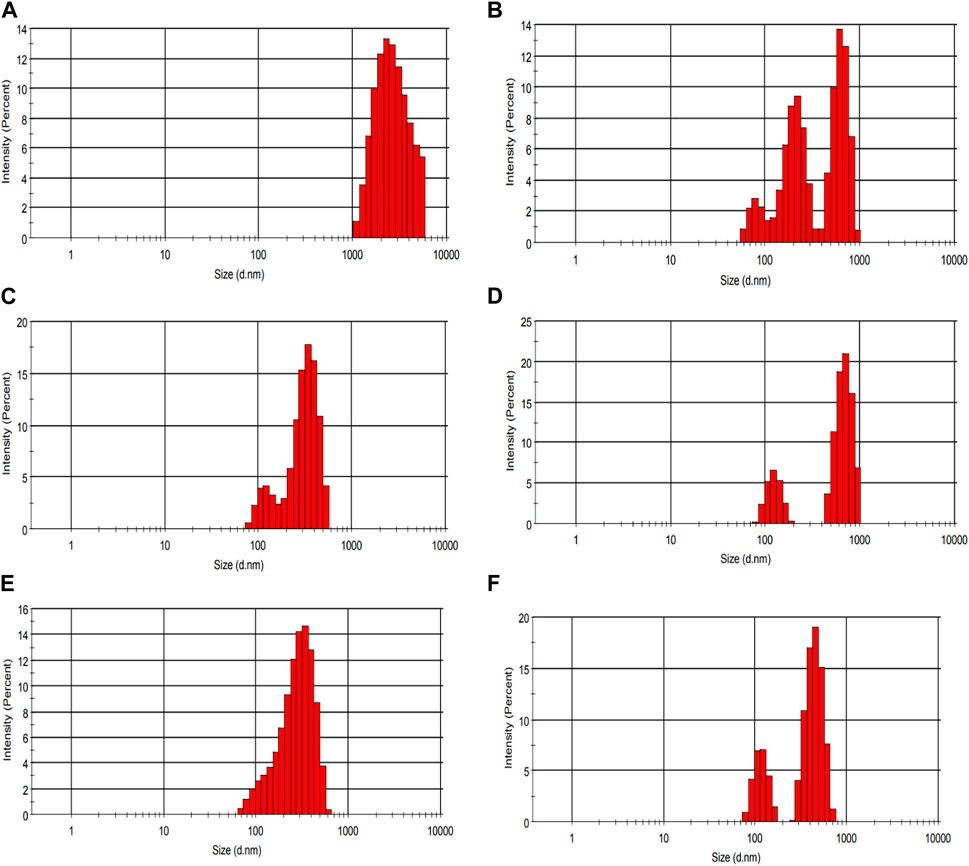
Figure 10. Particle size distribution of the waster-soluble extract of fermented sheep milk using KGL4 strain. (A) Unfermented sheep milk sample size, (B) fermented sheep milk sample size, (C) <3 kDa permeate sample size, (D) >3 kDa retentate sample size, (E) <10 kDa permeate sample size, (F) >10 kDa retentate sample size.
3.10 Molecular interaction study of peptides from fermented sheep milk
Over the last 10 years, molecular docking has become an essential instrument for examining the structural relationships between various ligands, including bioactive peptides, with specific enzyme active sites. This makes it possible for researchers to analyze and enhance the molecular connections between the objective proteins and examine peptide ligands (Singh et al., 2024). With this research, peptides were recognized through 2D gel and RP-LC/MS techniques, and in silico approaches were employed to investigate their interactions with particular enzymes. The peptide GPFPILV was selected for docking with pancreatic α-amylase and angiotensin-converting enzyme (ACE) based on its highest peptide ranking score and other relevant properties (Table 2). Considering the role of α-amylase in breaking down starch and glycogen for efficient absorption during digestion, it is reasonable to anticipate potential antidiabetic properties for the identified peptides using α-amylase (Mudgil et al., 2023b). Conversely, the possible antihypertensive effect of the discovered peptide GPFPILV was predicted using ACE. Within the angiotensin–renin system, ACE performs a crucial role in transforming angiotensin I to active angiotensin II vasoconstrictor, leading to blood vessel constriction and indirectly raising blood pressure (Singh et al., 2014). The RCSB-Protein Data Bank was utilized to obtain the 3D structures of the targeted enzymes, while the Active Site Prediction server was employed to predict their active sites. The peptide GPFPILV was 3D-structured using the Colab server according to the method outlined by Singh, Paul, and Goel in 2024. Docking experiments involving α-amylase and ACE enzymes were conducted using HPEPDOCK v2.0 and AutoDock Vina v1.2.0. The resultant complexes revealed diverse interactions between molecules, such as hydrophobic interactions and hydrogen bonding. As per LigPlot+ analysis conducted with AutoDock Vina, it was predicted that GPFPILV could form up to four hydrogen bonds with α-amylase, specifically interacting with residues Lys261, Lys13, Thr264, and Gly20. Additionally, the analysis indicated potential hydrophobic interactions with residues Leu18, Glu272, Ser270, Thr23, Ala22, Trp284, Lys257, Gly238, Leu237, and Gln19. Concerning ACE, the analysis suggested a maximum of three hydrogen bonds with residues Tyr135, Arg124, and Ser517, along with hydrophobic interactions involving residues Ile204, Glu123, Tyr62, Ser516, Asn85, Ile88, Trp59, Tyr360, Asn66, Leu139, Lpr702, and Trp220 (Supplementary Figure S1). These findings imply that GPFPILV might impede the active and binding sites of the enzymes, potentially hindering substrate binding and affecting their overall functionality. The robustness of protein–peptide complexes is ascribed to robust hydrogen bonds, a formidable intermolecular force. Furthermore, the affinity for binding the ligand within the connection pockets of the intended proteins is influenced by hydrophobic interactions (Khakhariya et al., 2023; Singh et al., 2024). Regarding binding affinity, GPFPILV demonstrated the most elevated values, registering −6.6 kcal/mol and −9.9 kcal/mol for α-amylase and ACE, respectively (Supplementary Table S1). The new HPEPDOCK web server intended for blind protein–peptide docking employs an algorithm with hierarchies, which eliminates the need for extensive modeling to improve the conformations of peptides. Instead, it integrates peptide pliability by utilizing a collection of conformations produced by the MODPEP de novo peptide modeling technique. In the investigation of potential binding mechanisms with specific enzymes, the peptide was subjected to docking analysis on the HPEPDOCK server (Mudgil et al., 2023b). Notably, the peptide GPFPILV demonstrated the highest HPEPDOCK scores of −171.717 and −200.641 against α-amylase and ACE, respectively. The comparison of findings across studies is challenging due to the varied scoring algorithms and patterns employed by different molecular docking tools. Nonetheless, the results of molecular docking analysis suggest that the peptides identified by interactions with various residues surrounding and within the active site are able to effectively limit the activities of the specific enzymes, principally through hydrogen bonding and hydrophobic interactions. Using this peptide structure, molecular docking is performed using Auto Dock Vina and HADDOCK software. The reason behind this is to validate the presence of hydrogen bonds and strong bonding. Further studies on dynamic simulations are required to assess the thermodynamic and dynamic properties.
4 Conclusion
The application of the Limosilactobacillus fermentum KGL4 (MF951099) culture in the fermentation of sheep milk demonstrated significant effects, including ACE inhibition, antidiabetic properties (such as inhibiting α-glucosidase, α-amylase, and lipase), and proteolytic activity. The highest levels of ACE inhibition (74.82%), α-amylase (70.02%), α-glucosidase (72.19%), and lipase inhibition (67.08%) were observed following a 48-h fermentation period, particularly when using a 2.5% inoculation rate (peptide: 9.88 mg/mL). The evaluation of proteolytic activity using OPA peaked after 48 h of incubation. The membrane fractions with an ultrafiltration cutoff showed a peak distribution predominantly between 3 and 10 kDa. Fermented sheep milk demonstrated notable inhibitory effects on ACE (76.69%), α-amylase (75.69%), α-glucosidase (66.27%), and lipase (71.67%). In experiments involving RAW 264.7 cells, S-KGL4 significantly attenuated excessive production of LPS activation inducing TNF-α, IL-6, and IL-1β which suggested potential anti-inflammatory properties. SDS-PAGE analysis detected protein fractions of 51–70 kDa and 10–32 kDa in fermented sheep milk, whereas 2D gel electrophoresis revealed 36 spots corresponding to the KGL4 strain with a molecular weight of 10–32 kDa. Furthermore, it was indicated that peptides with lower molecular weights demonstrate increased potential for ACE inhibition and antidiabetic effects. The study’s general inference is that fermented sheep milk containing Lactobacillus is a potentially valuable reservoir of peptides with antihypertensive and antidiabetic properties. However, it is crucial to conduct additional clinical studies to validate these health-related claims.
Data availability statement
The original contributions presented in the study are included in the article/Supplementary Material; further inquiries can be directed to the corresponding author.
Author contributions
RP: data curation, formal analysis, methodology, visualization, and writing–original draft. BB: writing–original draft and writing–review and editing. AS: data curation, formal analysis, investigation, software, supervision, visualization, and writing–original draft. RM: formal analysis, methodology, visualization, and writing–original draft. MB: writing–original draft and writing–review and editing. KK: resources, supervision, writing–original draft, and writing–review and editing. BS: data curation, formal analysis, software, visualization, writing–original draft, and writing–review and editing. SP: methodology, resources, software, visualization, and writing–original draft. ZL: writing–original draft and writing–review and editing. PS: formal analysis, investigation, methodology, project administration, visualization, and writing–original draft. AP: formal analysis, investigation, methodology, resources, and writing–original draft. SH: conceptualization, investigation, methodology, project administration, resources, supervision, writing–original draft, and writing–review and editing.
Funding
The authors declare that no financial support was received for the research, authorship, and/or publication of this article.
Conflict of interest
The authors declare that the research was conducted in the absence of any commercial or financial relationships that could be construed as a potential conflict of interest.
The authors declare that they were an editorial board member of Frontiers at the time of submission. This had no impact on the peer review process and final decision.
Publisher’s note
All claims expressed in this article are solely those of the authors and do not necessarily represent those of their affiliated organizations, or those of the publisher, the editors, and the reviewers. Any product that may be evaluated in this article, or claim that may be made by its manufacturer, is not guaranteed or endorsed by the publisher.
Supplementary material
The Supplementary Material for this article can be found online at: https://www.frontiersin.org/articles/10.3389/fchem.2024.1389846/full#supplementary-material
References
Ademiluyi, A. O., and Oboh, G. (2013). Soybean phenolic-rich extracts inhibit key enzymes linked to type 2 diabetes (α-amylase and α-glucosidase) and hypertension (angiotensin I converting enzyme) in vitro. Exp. Toxicol. Pathology 65 (3), 305–309. doi:10.1016/j.etp.2011.09.005
Aluko, R. E. (2015). Structure and function of plant protein-derived antihypertensive peptides. Curr. Opin. Food Sci. 4, 44–50. doi:10.1016/j.cofs.2015.05.002
Ashokbhai, J. K., Basaiawmoit, B., Das, S., Sakure, A., Maurya, R., Bishnoi, M., et al. (2022a). Antioxidative, antimicrobial and anti-inflammatory activities and release of ultra-filtered antioxidative and antimicrobial peptides during fermentation of sheep milk: in-vitro, in-silico and molecular interaction studies. Food Biosci. 47, 101666. doi:10.1016/j.fbio.2022.101666
Ashokbhai, J. K., Basaiawmoit, B., Sakure, A., Das, S., Patil, G. B., Mankad, M., et al. (2022b). Purification and characterization of antioxidative and antimicrobial peptides from lactic-fermented ewes milk. J. Food Sci. Technol., 1–11. doi:10.1007/s13197-022-05493-2
Baba, W. N., Baby, B., Mudgil, P., Gan, C. Y., Vijayan, R., and Maqsood, S. (2021). Pepsin generated camel whey protein hydrolysates with potential antihypertensive properties: identification and molecular docking of antihypertensive peptides. Food Sci. Technol. 143, 111135. doi:10.1016/j.lwt.2021.111135
Balthazar, C. F., Santillo, A., Guimarães, J. T., Capozzi, V., Russo, P., Caroprese, M., et al. (2019). Novel milk–juice beverage with fermented sheep milk and strawberry (Fragaria × ananassa): nutritional and functional characterization. J. Dairy Sci. 102 (12), 10724–10736. doi:10.3168/jds.2019-16909
Baum, F., Fedorova, M., Ebner, J., Hoffmann, R., and Pischetsrieder, M. (2013). Analysis of the endogenous peptide profile of milk: identification of 248 mainly casein-derived peptides. J. Proteome Res. 12 (12), 5447–5462. doi:10.1021/pr4003273
Boeck, T., Zannini, E., Sahin, A. W., Bez, J., and Arendt, E. K. (2021). Nutritional and rheological features of lentil protein isolate for yoghurt-like application. Foods 10 (8), 1692. doi:10.3390/foods10081692
Carrasco-Castilla, J., Hernandez, A., Lvarez, A. J., Jimenez-Martinez, C., JacintoHernandez, C., Alaiz, M., et al. (2012). Antioxidant and metal chelating activities of Phaseolus vulgaris L. var. Jamapa protein isolates, phaseolin and lectin hydrolysates. Food Chem. 131 (2), 1157–1164. doi:10.1016/j.foodchem.2011.09.084
Chen, Y., Li, C., Xue, J., Kwok, L. Y., Yang, J., Zhang, H., et al. (2015). Characterization of angiotensin-converting enzyme inhibitory activity of fermented milk produced by Lactobacillus helveticus. J. Dairy Sci. 98 (8), 5113–5124. doi:10.3168/jds.2015-9382
Cushman, D. W., and Cheung, H. S. (1971). Spectrophotometric assay and properties of the angiotensin-converting enzyme of rabbit lung. Biochem. Pharmacol. 20 (7), 1637–1648. doi:10.1016/0006-2952(71)90292-9
Dario, C., Carnicella, D., Dario, M., and Bufano, G. (2008). Genetic polymorphism of β-lactoglobulin gene and effect on milk composition in Leccese sheep. Small Ruminant Res. 74 (1-3), 270–273. doi:10.1016/j.smallrumres.2007.06.007
Del Mar Contreras, M., Carrón, R., Montero, M. J., Ramos, M., and Recio, I. (2009). Novel caseinderived peptides with antihypertensive activity. Int. Dairy J. 19 (10), 566–573. doi:10.1016/j.idairyj.2009.05.004
De Souza Rocha, T., Hernandez, L. M. R., Chang, Y. K., and de Mejía, E. G. (2014). Impact of germination and enzymatic hydrolysis of cowpea bean (Vigna unguiculata) on the generation of peptides capable of inhibiting dipeptidyl peptidase IV. Food Res. Int. 64, 799–809. doi:10.1016/j.foodres.2014.08.016
Ding, L., Ma, R., You, H., Li, J., Ge, Q., Yu, Z., et al. (2022). Identification and characterization of dipeptidyl peptidase IV inhibitory peptides from wheat gluten proteins. J. Cereal Sci. 103, 103396. doi:10.1016/j.jcs.2021.103396
Dziuba, M., Dziuba, B., and Iwaniak, A. (2009). Milk proteins as precursors of bioactive peptides. Acta Sci. Pol. Technol. Aliment. 8 (1), 71–90.
Esfandi, R., Seidu, I., Willmore, W., and Tsopmo, A. (2022). Antioxidant, pancreatic lipase, and α-amylase inhibitory properties of oat bran hydrolyzed proteins and peptides. J. Food Biochem. 46 (4), e13762. doi:10.1111/jfbc.13762
FAO (2017). FAO: data. Livestock primary. Available at: http://www.fao.org/faostat/en/#data/QL.
FAO (2018). Statistics database. Available at: http://www.fao.org/faostat/en/#data (Accessed February 02, 2018).
Gibbs, B. F., Zougman, A., Masse, R., and Mulligan, C. (2004). Production and characterization of bioactive peptides from soy hydrolysate and soy fermented food. Food Res. Int. 37 (3), 123–131. doi:10.1016/j.foodres.2003.09.010
Gómez-Ruiz, J. Á., Ramos, M., and Recio, I. (2007). Identification of novel angiotensin- converting enzyme-inhibitory peptides from ovine milk proteins by CE-MS and chromatographic techniques. Electrophoresis 28 (22), 4202–4211. doi:10.1002/elps.200700324
Gong, H., Gao, J., Wang, Y., Luo, Q. W., Guo, K. R., Ren, F. Z., et al. (2020). Identification of novel peptides from goat milk casein that ameliorate highglucose-induced insulin resistance in HepG2 cells. J. Dairy Sci. 103 (6), 4907–4918. doi:10.3168/jds.2019-17513
Gu, Y., Majumder, K., and Wu, J. (2011). QSAR-aided in silico approach in evaluation of food proteins as precursors of ACE inhibitory peptides. Food Res. Int. 44 (8), 2465–2474. doi:10.1016/j.foodres.2011.01.051
Gu, Y., and Wu, J. (2013). LC–MS/MS coupled with QSAR modeling in characterising of angiotensin I-converting enzyme inhibitory peptides from soybean proteins. Food Chem. 141 (3), 2682–2690. doi:10.1016/j.foodchem.2013.04.064
Gupta, R., Gaur, K., and S. Ram, C. V. (2019). Emerging trends in hypertension epidemiology in India. J. Hum. Hypertens. 33 (8), 575–587. doi:10.1038/s41371-018-0117-3
Gútiez, L., Gómez-Sala, B., Recio, I., del Campo, R., Cintas, L. M., Herranz, C., et al. (2013). Enterococcus faecalis strains from food, environmental, and clinical origin produce ACE-inhibitory peptides and other bioactive peptides during growth in bovine skim milk. Int. J. Food Microbiol. 166 (1), 93–101. doi:10.1016/j.ijfoodmicro.2013.06.019
Haenlein, G. F. W. (2001). Past, present, and future perspectives of small ruminant dairy research. J. Dairy Sci. 84 (9), 2097–2115. doi:10.3168/jds.S0022-0302(01)74655-3
Hati, S., Patel, N., and Mandal, S. (2018). Comparative growth behaviour and biofunctionality of lactic acid bacteria during fermentation of soy milk and bovine milk. Probiotics Antimicrob. Proteins 10, 277–283. doi:10.1007/s12602-017-9279-5
Hati, S., Sreeja, V., Solanki, D., and Prajapati, J. B. (2015). Influence of proteolytic Lactobacilli on ACE inhibitory activity and release of BAPs. Indian J. Dairy Sci. 68, 1–8. doi:10.3390/foods12102006
Hayes, M., Stanton, C., Slattery, H., O'sullivan, O., Hill, C., Fitzgerald, G. F., et al. (2007). Casein fermentate of Lactobacillus animalis DPC6134 contains a range of novel propeptide angiotensin-converting enzyme inhibitors. Appl. Environ. Microbiol. 73 (14), 4658–4667. doi:10.1128/AEM.00096-07
Hernández-Ledesma, B., del Mar Contreras, M., and Recio, I. (2011). Antihypertensive peptides: production, bioavailability and incorporation into foods. Adv. Colloid Interface Sci. 165 (1), 23–35. doi:10.1016/j.cis.2010.11.001
Hernández-Ledesma, B., and Hsieh, C. C. (2013). Bioactive food peptides in health and disease. Norderstedt, Germany: BoD–Books on Demand. doi:10.5772/3318
Ibrahim, H. R., Ahmed, A. S., and Miyata, T. (2017). Novel angiotensin-converting enzyme inhibitory peptides from caseins and whey proteins of goat milk. J. Adv. Res. 8 (1), 63–71. doi:10.1016/j.jare.2016.12.002
Ibrahim, M. A., Serem, J. C., Bester, M. J., Neitz, A. W., and Gaspar, A. R. (2019). Multiple antidiabetic effects of three α-glucosidase inhibitory peptides, PFP, YPL and YPG: dipeptidyl peptidase–IV inhibition, suppression of lipid accumulation in differentiated 3T3-L1 adipocytes and scavenging activity on methylglyoxal. Int. J. Biol. Macromol. 122, 104–114. doi:10.1016/j.ijbiomac.2018.10.152
Iwaniak, A., and Dziuba, J. (2009). Analysis of domains in selected plant and animal food proteinsprecursors of biologically active peptides-in silico approach. Food Sci. Technol. Int. 15 (2), 179–191. doi:10.1177/1082013208106320
Kannan, R. (2019). India is home to 77 million diabetics, second highest in the world. Available at: https://www.thehindu.com/sci-tech/health/indiahas-second-largest-number-of-people-with-diabetes/.
Khakhariya, R., Sakure, A. A., Maurya, R., Bishnoi, M., Kondepudi, K. K., Padhi, S., et al. (2023). A comparative study of fermented buffalo and camel milk with anti-inflammatory, ACE-inhibitory and anti-diabetic properties and release of bio active peptides with molecular interactions: in vitro, in silico and molecular study. Food Biosci. 52, 102373. doi:10.1016/j.fbio.2023.102373
Khare, P., Maurya, R., Bhatia, R., Mangal, P., Singh, J., Podili, K., et al. (2020). Polyphenol rich extracts of finger millet and kodo millet ameliorate high fat diet-induced metabolic alterations. Food & Funct. 11 (11), 9833–9847. doi:10.1039/d0fo01643h
Kinariwala, D., Panchal, G., Sakure, A., and Hati, S. (2020). Exploring the potentiality of lactobacillus cultures on the production of milk-derived bioactive peptides with antidiabetic activity. Int. J. Peptide Res. Ther. 26, 1613–1627. doi:10.1007/s10989-019-09958-5
Kopf-Bolanz, K. A., Schwander, F., Gijs, M., Vergères, G., Portmann, R., and Egger, L. (2014). Impact of milk processing on the generation of peptides during digestion. Int. Dairy J. 35 (2), 130–138. doi:10.1016/j.idairyj.2013.10.012
Kurihara, H., Asami, S., Shibata, H., Fukami, H., and Tanaka, T. (2003). Hypolipemic effect of Cyclocarya paliurus (Batal) Iljinskaja in lipid-loaded mice. Biol. Pharm. Bull. 26 (3), 383–385. doi:10.1248/bpb.26.383
Lacroix, I. M., and Li-Chan, E. C. (2014). Isolation and characterization of peptides with dipeptidyl peptidase-IV inhibitory activity from pepsin-treated bovine whey proteins. Peptides 54, 39–48. doi:10.1016/j.peptides.2014.01.002
Laemmli, U. K. (1970). Cleavage of structural proteins during the assembly of the head of bacteriophage T4. Nature 227 (5259), 680–685. doi:10.1038/227680a0
Lammi, C., Zanoni, C., Arnoldi, A., and Vistoli, G. (2016). Peptides derived from soy and lupin protein as dipeptidyl-peptidase IV inhibitors: in vitro biochemical screening and in silico molecular modeling study. J. Agric. Food Chem. 64 (51), 9601–9606. doi:10.1021/acs.jafc.6b04041
Lan, V. T. T., Ito, K., Ohno, M., Motoyama, T., Ito, S., and Kawarasaki, Y. (2015). Analyzing a dipeptide library to identify human dipeptidyl peptidase IV inhibitor. Food Chem. 175, 66–73. doi:10.1016/j.foodchem.2014.11.131
Larosa, C. P., Balthazar, C. F., Guimarães, J. T., Margalho, L. P., Lemos, F. S., Oliveira, F. L., et al. (2021). Can sucrose-substitutes increase the antagonistic activity against foodborne pathogens, and improve the technological and functional properties of ewes milk kefir? Food Chem. 351, 129290. doi:10.1016/j.foodchem.2021.129290
Lau, C. C., Abdullah, N., and Shuib, A. S. (2013). Novel angiotensin I-converting enzyme inhibitory peptides derived from an edible mushroom, Pleurotus cystidiosus OK Miller identified by LC-MS/MS. BMC Complementary Altern. Med. 13 (1), 313–410. doi:10.1186/1472-6882-13-313
Liu, L., Chen, J., and Li, X. (2021). Novel peptides with α-glucosidase inhibitory activity from Changii Radix hydrolysates. Process Biochem. 111, 200–206. doi:10.1016/j.procbio.2021.08.019
Martínez-Maqueda, D., Miralles, B., Recio, I., and Hernández-Ledesma, B. (2012). Antihypertensive peptides from food proteins: a review. Food & Funct. 3 (4), 350–361. doi:10.1039/C2FO10192K
Meisel, H., and Schlimme, E. (1990). Milk proteins: precursors of bioactive peptides. Trends Food Sci. Technol. 1, 41–43. doi:10.1016/0924-2244(90)90029-X
Mirdita, M., Schütze, K., Moriwaki, Y., Heo, L., Ovchinnikov, S., and Steinegger, M. (2022). ColabFold: making protein folding accessible to all. Nat. Methods 19 (6), 679–682. doi:10.1038/s41592-022-01488-1
Mohapatra, A., Shinde, A. K., and Singh, R. (2019). Sheep milk: a pertinent functional food. Small Ruminant Res. 181, 6–11. doi:10.1016/j.smallrumres.2019.10.002
Mojica, L., Chen, K., and de Mejía, E. G. (2015). Impact of commercial precooking of common bean (Phaseolus vulgaris) on the generation of peptides, after pepsin– pancreatin hydrolysis, capable to inhibit dipeptidyl peptidase-IV. J. Food Sci. 80 (1), H188–H198. doi:10.1111/1750-3841.12726
Mu, X., Wang, R., Cheng, C., Ma, Y., Zhang, Y., and Lu, W. (2023). Preparation, structural properties, and in vitro and in vivo activities of peptides against dipeptidyl peptidase IV (DPP-IV) and α-glucosidase: a general review. Crit. Rev. Food Sci. Nutr., 1–13. doi:10.1080/10408398.2023.2217444
Mudgil, P., Alblooshi, M., Singh, B. P., Devarajan, A. R., and Maqsood, S. (2023a). Pearl millet protein hydrolysates exhibiting effective in-vitro antioxidant, antidiabetic and anti-lipidemic properties as potential functional food ingredient. Int. J. Food Sci. Technol. 58 (6), 3264–3272. doi:10.1111/ijfs.16151
Mudgil, P., Gan, C. Y., Baig, M. A., Hamdi, M., Mohteshamuddin, K., Aguilar-Toalá, J. E., et al. (2023b). In-depth peptidomic profile and molecular simulation studies on ACE-inhibitory peptides derived from probiotic fermented milk of different farm animals. Food Res. Int. 168, 112706. doi:10.1016/j.foodres.2023.112706
Nagpal, R., Behare, P., Rana, R., Kumar, A., Kumar, M., Arora, S., et al. (2011). Bioactive peptides derived from milk proteins and their health beneficial potentials: an update. Food & Funct. 2 (1), 18–27. doi:10.1039/C0FO00016G
Ngoh, Y. Y., and Gan, C. Y. (2018). Identification of Pinto bean peptides with inhibitory effects on α-amylase and angiotensin converting enzyme (ACE) activities using an integrated bioinformatics-assisted approach. Food Chem. 267, 124–131. doi:10.1016/j.foodchem.2017.04.166
Nguyen, H. T., Gomes Reis, M., Wa, Y., Alfante, R., Chanyi, R. M., Altermann, E., et al. (2023). Differences in aroma metabolite profile, microstructure, and rheological properties of fermented milk using different cultures. Foods 12 (9), 1875. doi:10.3390/foods12091875
Ningrum, S., Sutrisno, A., and Hsu, J. L. (2022). An exploration of angiotensinconverting enzyme (ACE) inhibitory peptides derived from gastrointestinal protease hydrolysate of milk using a modified bioassay-guided fractionation approach coupled with in silico analysis. J. Dairy Sci. 105 (3), 1913–1928. doi:10.3168/jds.2021-21112
Ningtyas, D. W., Hati, S., and Prakash, S. (2021). Bioconversion and bioaccessibility of isoflavones from sogurt during in vitro digestion. Food Chem. 343, 128553. doi:10.1016/j.foodchem.2020.128553
Nongonierma, A. B., and FitzGerald, R. J. (2013). Inhibition of dipeptidyl peptidase IV (DPP-IV) by proline containing casein-derived peptides. J. Funct. Foods 5 (4), 1909–1917. doi:10.1016/j.jff.2013.09.012
Nongonierma, A. B., Mooney, C., Shields, D. C., and FitzGerald, R. J. (2014). In silico approaches to predict the potential of milk protein-derived peptides as dipeptidyl peptidase IV (DPPIV) inhibitors. Peptides 57, 43–51. doi:10.1016/j.peptides.2014.04.018
Oshima, G., Shimabukuro, H., and Nagasawa, K. (1979). Peptide inhibitors of angiotensin I-converting enzyme in digests of gelatin by bacterial collagenase. Biochimica Biophysica Acta (BBA)-Enzymology. 566 (1), 128–137. doi:10.1016/0005-2744(79)90255-9
Padhi, S., Chourasia, R., Kumari, M., Singh, S. P., and Rai, A. K. (2022). Production and characterization of bioactive peptides from rice beans using Bacillus subtilis. Bioresour. Technol. 351, 126932. doi:10.1016/j.biortech.2022.126932
Panchal, G., Hati, S., and Sakure, A. (2020). Characterization and production of novel antioxidative peptides derived from fermented goat milk by L. fermentum. Food Sci. Technol. 119, 108887. doi:10.1016/j.lwt.2019.108887
Park, Y. W., and Nam, M. S. (2015). Bioactive peptides in milk and dairy products: a review. Korean J. Food Sci. Animal Resour. 35 (6), 831–840. doi:10.5851/kosfa.2015.35.6.831
Parmar, H. (2017). Isolation and purification of ACE-inhibitory peptides derived from fermented surti goat milk. Master’s thesis. AAU, Anand, Gujarat: Anand Agricultural University.
Parmar, H., Hati, S., Panchal, G., and Sakure, A. A. (2020). Purification and production of novel angiotensin I-converting enzyme (ACE) inhibitory bioactive peptides derived from fermented goat milk. Int. J. Peptide Res. Ther. 26, 997–1011. doi:10.1007/s10989-019-09902-7
Parmar, H., Hati, S., and Sakure, A. (2018). In vitro and in silico analysis of novel ACEinhibitory bioactive peptides derived from fermented goat milk. Int. J. Peptide Res. Ther. 24, 441–453. doi:10.1007/s10989-017-9630-4
Pei, J., Liu, Z., Pan, D., Zhao, Y., Dang, Y., and Gao, X. (2022). Transport, stability, and in vivo hypoglycemic effect of a broccoli-derived DPP-IV inhibitory peptide VPLVM. J. Agric. Food Chem. 70 (16), 4934–4941. doi:10.1021/acs.jafc.1c08191
Phelan, M., and Kerins, D. (2011). The potential role of milk-derived peptides in cardiovascular disease. Food & Funct. 2 (3-4), 153–167. doi:10.1039/C1FO10017C
Pihlanto, A., and Mäkinen, S. (2013). Antihypertensive properties of plant protein derived peptides. Bioact. Food Peptides Health Dis., 144–182. doi:10.5772/54565
Puchalska, P., Marina Alegre, M. L., and Garcia Lopez, M. C. (2015). Isolation and characterization of peptides with antihypertensive activity in foodstuffs. Crit. Rev. Food Sci. Nutr. 55 (4), 521–551. doi:10.1080/10408398.2012.664829
Qiu, L., Deng, Z., Zhao, C., Xiao, T., Weng, C., Li, J., et al. (2021). Nutritional composition and proteomic analysis of soft-shelled turtle (Pelodiscus sinensis) egg and identification of oligopeptides with alpha-glucosidase inhibitory activity. Food Res. Int. 145, 110414. doi:10.1016/j.foodres.2021.110414
Raveschot, C., Cudennec, B., Coutte, F., Flahaut, C., Fremont, M., Drider, D., et al. (2018). Production of bioactive peptides by lactobacillus species: from gene to application. Front. Microbiol. 9, 2354. doi:10.3389/fmicb.2018.02354
Razafindrakoto, O., Ravelomanana, N., Rasolofo, A., Rakotoarimanana, R. D., Gourgue, P., Coquin, P., et al. (1993). Le lait de chèvre peut-il remplacer le lait de vache chez l'enfant malnutri? Le. Lait. 73 (5-6), 601–611. doi:10.1051/lait:19935-658
Robert, M. C., Razaname, A., Mutter, M., and Juillerat, M. A. (2004). Identification of angiotensin-I-converting enzyme inhibitory peptides derived from sodium caseinate hydrolysates produced by Lactobacillus helveticus NCC 2765. J. Agric. Food Chem. 52 (23), 6923–6931. doi:10.1021/jf049510t
Rubak, Y. T., Nuraida, L., Iswantini, D., and Prangdimurti, E. (2020). Angiotensin-I-converting enzyme inhibitory peptides in milk fermented by indigenous lactic acid bacteria. Veterinary World 13 (2), 345–353. doi:10.14202/vetworld.2020.345-353
Rubak, Y. T., Nuraida, L., Iswantini, D., and Prangdimurti, E. (2022). Angiotensin-IConverting enzyme inhibitory peptides in goat milk fermented by lactic acid bacteria isolated from fermented food and breast milk. Food Sci. Animal Resour. 42 (1), 46–60. doi:10.5851/kosfa.2021.e55
Sagardia, I., Iloro, I., Elortza, F., and Bald, C. (2013). Quantitative structure–activity relationship-based screening of bioactive peptides identified in ripened cheese. Int. Dairy J. 33 (2), 184–190. doi:10.1016/j.idairyj.2012.12.006
Sansi, M. S., Iram, D., Vij, S., Kapila, S., and Meena, S. (2023). In vitro biosafety and bioactivity assessment of the goat milk protein derived hydrolysates peptides. J. Food Saf. 43, e13061. doi:10.1111/jfs.13061
Sarteshnizi, R. A., Sahari, M. A., Gavlighi, H. A., Regenstein, J. M., Nikoo, M., and Udenigwe, C. C. (2021). Influence of fish protein hydrolysate-pistachio green hull extract interactions on antioxidant activity and inhibition of α-glucosidase, α-amylase, and DPP-IV enzymes. Food Sci. Technol. 142, 111019. doi:10.1016/j.lwt.2021.111019
Selvaggi, M., Laudadio, V., Dario, C., and Tufarelli, V. (2014). Investigating the genetic polymorphism of sheep milk proteins: a useful tool for dairy production. J. Sci. Food Agric. 94 (15), 3090–3099. doi:10.1002/jsfa.6750
Sergent, T., Vanderstraeten, J., Winand, J., Beguin, P., and Schneider, Y. J. (2012). Phenolic compounds and plant extracts as potential natural antiobesity substances. Food Chem. 135 (1), 68–73. doi:10.1016/j.foodchem.2012.04.074
Shai, L. J., Magano, S. R., Lebelo, S. L., and Mogale, A. M. (2011). Inhibitory effects of five medicinal plants on rat alpha-glucosidase: comparison with their effects on yeast alphaglucosidase. J. Med. Plants Res. 5 (13), 2863–2867.
Shirkhan, F., Mirdamadi, S., Mirzaei, M., Akbari-adergani, B., and Nasoohi, N. (2023). The role of lactic acid bacteria in production of bioactive peptides in fermented milk with antioxidant and antidiabetic properties. J. Food Meas. Charact. 17, 4727–4738. doi:10.1007/s11694-023-01968-8
Shukla, P., Sakure, A., Pipaliya, R., Basaiawmoit, B., Maurya, R., Bishnoi, M., et al. (2022). Exploring the potential of Lacticaseibacillus paracasei M11 on antidiabetic, anti-inflammatory, and ACE inhibitory effects of fermented dromedary camel milk (Camelus dromedaries) and the release of antidiabetic and anti-hypertensive peptides. J. Food Biochem. 46 (12), e14449. doi:10.1111/jfbc.14449
Singh, B. P., Paul, S., and Goel, G. (2024a). Shotgun proteomics and molecular simulations on multifunctional bioactive peptides derived from the whey of unexplored “Gaddi” goat of Himalayas. Food Chem. 430, 137075. doi:10.1016/j.foodchem.2023.137075
Singh, B. P., Vij, S., and Hati, S. (2014b). Functional significance of bioactive peptides derived from soybean. Peptides 54, 171–179. doi:10.1016/j.peptides.2014.01.022
Singh, T., Biswas, D., and Jayaram, B. (2011). AADS-An automated active site identification, docking, and scoring protocol for protein targets based on physicochemical descriptors. J. Chem. Inf. Model. 51 (10), 2515–2527. doi:10.1021/ci200193z
Siow, H. L., and Gan, C. Y. (2016). Extraction, identification, and structure–activity relationship of antioxidative and α-amylase inhibitory peptides from cumin seeds (Cuminum cyminum). J. Funct. Foods 22, 1–12. doi:10.1016/j.jff.2016.01.011
Solanki, D. (2016). Purification and Characterization of ACE-inhibitory peptides derived from fermented camel milk. Master’s thesis. Anand, Gujarat: Anand Agricultural University, AAU.
Solanki, D., Hati, S., and Sakure, A. (2017). In silico and in vitro analysis of novel angiotensin I-converting enzyme (ACE) inhibitory bioactive peptides derived from fermented camel milk (Camelus dromedarius). Int. J. Peptide Res. Ther. 23, 441–459. doi:10.1007/s10989-017-9577-5
Steel, R. G. D., and Torrie, J. H. (1980). Principles and procedure of statistics-a biometrical approach. Japan: Mcgraw Hill Kogakusha Ltd., 137. doi:10.2307/2287561
Tavares, T. G., and Malcata, F. X. (2013). Whey proteins as source of bioactive peptides against hypertension. Bioact. Food Peptides Health Dis. 75. doi:10.5772/52680
Telagari, M., and Hullatti, K. (2015). In-vitro α-amylase and α-glucosidase inhibitory activity of Adiantum caudatum Linn. and Celosia argentea Linn. extracts and fractions. Indian J. Pharmacol. 47 (4), 425. doi:10.4103/0253-7613.161270
Theysgeur, S., Cudennec, B., Deracinois, B., Perrin, C., Guiller, I., Lepoudère, A., et al. (2020). New bioactive peptides identified from a Tilapia byproduct hydrolysate exerting effects on DPP-IV activity and intestinal hormones regulation after canine gastrointestinal simulated digestion. Molecules 26 (1), 136. doi:10.3390/molecules26010136
Trott, O., and Olson, A. J. (2010). AutoDock Vina: improving the speed and accuracy of docking with a new scoring function, efficient optimization, and multithreading. J. Comput. Chem. 31 (2), 455–461. doi:10.1002/jcc.21334
Uenishi, H., Kabuki, T., Seto, Y., Serizawa, A., and Nakajima, H. (2012). Isolation and identification of casein-derived dipeptidyl-peptidase 4 (DPP-4)-inhibitory peptide LPQNIPPL from gouda-type cheese and its effect on plasma glucose in rats. Int. Dairy J. 22 (1), 24–30. doi:10.1016/j.idairyj.2011.08.002
Vos, T., Abajobir, A. A., Abate, K. H., Abbafati, C., Abbas, K. M., Abd-Allah, F., et al. (2017). Global, regional, and national incidence, prevalence, and years lived with disability for 328 diseases and injuries for 195 countries, 1990–2016: a systematic analysis for the Global Burden of Disease Study 2016. Lancet 390 (10100), 1211–1259. doi:10.1016/S0140-6736(17)32154-2
Wakai, T., and Yamamoto, N. (2012). Antihypertensive peptides specific to Lactobacillus helveticus fermented milk. Biotechnology-Molecular Stud. Nov. Appl. Improv. Qual. Hum. Life, 159–172. doi:10.5772/28695
WHO (2021a). WHO. Available at: https://www.who.int/news-room/fact-sheets/detail/cardiovasculardiseases-(cvds).
WHO (2021b). WHO. Available at: https://www.who.int/news-room/factsheets/detail/hypertension#:∼:text=Hypertension%20is%20when%20blood%20pressure,the%20heart%20rests%20between%20beats.
Wu, J., Aluko, R. E., and Nakai, S. (2006). Structural requirements of angiotensin I- converting enzyme inhibitory peptides: quantitative structure-activity relationship modeling of peptides containing 4-10 amino acid residues. QSAR Comb. Sci. 25 (10), 873–880. doi:10.1002/qsar.200630005
Wu, N., Xu, W., Liu, K., Xia, Y., and Shuangquan, (2019). Angiotensin-converting enzyme inhibitory peptides from Lactobacillus delbrueckii QS306 fermented milk. J. Dairy Sci. 102 (7), 5913–5921. doi:10.3168/jds.2018-15901
Yamaki, K., and Mori, Y. (2006). Evaluation of alpha -glucosidase inhibitory activity in colored foods: a trial using slope factors of regression curves. J. Jpn. Soc. Food Sci. Technol. 53 (4), 229–231. doi:10.3136/nskkk.53.229
Yamamoto, N., Akino, A., and Takano, T. (1994). Antihypertensive effect of the peptides derived from casein by an extracellular proteinase from Lactobacillus helveticus CP790. J. Dairy Sci. 77 (4), 917–922. doi:10.3168/jds.S0022-0302(94)77026-0
Yang, Y., Zheng, N., Yang, J., Bu, D., Wang, J., Ma, L., et al. (2014). Animal species milk identification by comparison of two-dimensional gel map profile and mass spectrometry approach. Int. Dairy J. 35 (1), 15–20. doi:10.1016/j.idairyj.2013.09.008
Yu, Z., Yin, Y., Zhao, W., Yu, Y., Liu, B., Liu, J., et al. (2011). Novel peptides derived from egg white protein inhibiting alpha-glucosidase. Food Chem. 129 (4), 1376–1382. doi:10.1016/j.foodchem.2011.05.067
Zambrowicz, A., Pokora, M., Setner, B., Dąbrowska, A., Szołtysik, M., Babij, K., et al. (2015). Multifunctional peptides derived from an egg yolk protein hydrolysate: isolation and characterization. Amino Acids 47 (2), 369–380. doi:10.1007/s00726-014-1869-x
Zhang, Y., Chen, R., Zuo, F., Ma, H., Zhang, Y., and Chen, S. (2016). Comparison of dipeptidyl peptidase IV-inhibitory activity of peptides from bovine and caprine milk casein by in silico and in vitro analyses. Int. Dairy J. 53, 37–44. doi:10.1016/j.idairyj.2015.10.001
Zhao, B., Su, K., Mao, X., and Zhang, X. (2020). Separation and identification of enzyme inhibition peptides from dark tea protein. Bioorg. Chem. 99, 103772. doi:10.1016/j.bioorg.2020.103772
Zheng, L., Xu, Q., Lin, L., Zeng, X. A., Sun, B., and Zhao, M. (2019). In vitro metabolic stability of a casein-derived dipeptidyl peptidase-IV (DPP-IV) inhibitory peptide VPYPQ and its controlled release from casein by enzymatic hydrolysis. J. Agric. Food Chem. 67 (38), 10604–10613. doi:10.1021/acs.jafc.9b03164
Zhou, P., Jin, B., Li, H., and Huang, S. Y. (2018). HPEPDOCK: a web server for blind peptide–protein docking based on a hierarchical algorithm. Nucleic Acids Res. 46 (W1), W443–W450. doi:10.1093/nar/gky357
Złotek, U., Jakubczyk, A., Rybczyńska-Tkaczyk, K., Ćwiek, P., Baraniak, B., and Lewicki, S. (2020). Characteristics of new peptides GQLGEHGGAGMG, GEHGGAGMGGGQFQPV, EQGFLPGPEESGR, RLARAGLAQ, YGNPVGGVGH, and GNPVGGVGHGTTGT as inhibitors of enzymes involved in metabolic syndrome and antimicrobial potential. Molecules 25 (11), 2492. doi:10.3390/molecules25112492
Keywords: sheep milk, biofunctional properties, peptides, peptidomics, fermentation
Citation: Pipaliya R, Basaiawmoit B, Sakure AA, Maurya R, Bishnoi M, Kondepudi KK, Singh BP, Paul S, Liu Z, Sarkar P, Patel A and Hati S (2024) Peptidomics-based identification of antihypertensive and antidiabetic peptides from sheep milk fermented using Limosilactobacillus fermentum KGL4 MTCC 25515 with anti-inflammatory activity: in silico, in vitro, and molecular docking studies. Front. Chem. 12:1389846. doi: 10.3389/fchem.2024.1389846
Received: 22 February 2024; Accepted: 01 April 2024;
Published: 30 April 2024.
Edited by:
Peta Harvey, The University of Queensland, AustraliaCopyright © 2024 Pipaliya, Basaiawmoit, Sakure, Maurya, Bishnoi, Kondepudi, Singh, Paul, Liu, Sarkar, Patel and Hati. This is an open-access article distributed under the terms of the Creative Commons Attribution License (CC BY). The use, distribution or reproduction in other forums is permitted, provided the original author(s) and the copyright owner(s) are credited and that the original publication in this journal is cited, in accordance with accepted academic practice. No use, distribution or reproduction is permitted which does not comply with these terms.
*Correspondence: Subrota Hati, c3Vicm90YV9kdEB5YWhvby5jb20=