- 1Henan Key Laboratory of Advanced Nylon Materials and Application, School of Materials Science and Engineering, Zhengzhou University, Zhengzhou, China
- 2Zhengzhou Tobacco Research Institute of CNTC, Zhengzhou, China
- 3State Key Laboratory of High-efficiency Utilization of Coal and Green Chemical Engineering, Ningxia University, Yinchuan, China
Capturing and separating carbon dioxide, particularly using porous carbon adsorption separation technology, has received considerable research attention due to its advantages such as low cost and ease of regeneration. In this study, we successfully developed a one-step carbonization activation method using freeze-thaw pre-mix treatment to prepare high-nitrogen-content microporous nitrogen-doped carbon materials. These materials hold promise for capturing and separating CO2 from complex gas mixtures, such as biogas. The nitrogen content of the prepared carbon adsorbents reaches as high as 13.08 wt%, and they exhibit excellent CO2 adsorption performance under standard conditions (1 bar, 273 K/298 K), achieving 6.97 mmol/g and 3.77 mmol/g, respectively. Furthermore, according to Ideal Adsorption Solution Theory (IAST) analysis, these materials demonstrate material selectivity for CO2/CH4 (10 v:90 v) and CO2/CH4 (50 v:50 v) of 33.3 and 21.8, respectively, at 1 bar and 298 K. This study provides a promising CO2 adsorption and separation adsorbent that can be used in the efficient purification process for carbon dioxide, potentially reducing greenhouse gas emissions in industrial and energy production, thus offering robust support for addressing climate change and achieving more environmentally friendly energy production and carbon capture goals.
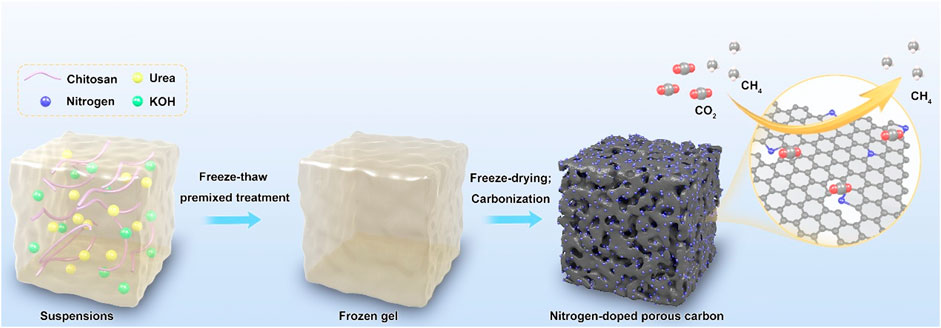
GRAPHICAL ABSTRACT | The nitrogen-doped porous carbon materials synthesized by the one-step carbonization activation method of freeze-thaw premixed treatment had extremely narrow pore size distribution and high nitrogen content, thus improving the adsorption and separation performance of the materials for CO2/CH4 gas mixtures.
1 Introduction
Over the past few decades, the extensive consumption of fossil fuels such as coal and oil has led to a significant increase in the concentration of carbon dioxide (CO2) in the atmosphere (Singh et al., 2019). These substantial CO2 emissions have adverse impacts on Earth’s climate, including accelerating sea-level rise and continuous glacier melting. With the rapid development of human society, the demand for energy continues to grow, while the reserves of fossil fuels like coal and oil are gradually depleting, further increasing the need for renewable alternative energy sources. Biogas was reliable renewable energy, generally produced by the anaerobic digestion of biomass. Biogas was mainly composed of CH4, CO2 and other components (Adnan et al., 2019). However, the existence of high concentration CO2 will reduce its calorific value and then hinder its practical application (Wang et al., 2015). To effectively utilize biogas and reduce air pollution, it was very urgent and vital to capture CO2 and recover CH4 from CO2/CH4 mixture gas. Carbon capture and CO2 separation technologies have thus become critical fields for reducing greenhouse gas emissions and achieving more environmentally sustainable energy production goals (Bernardo et al., 2021).
In this context, we place a particular emphasis on the capture and separation of CO2, especially through the use of porous carbon adsorption separation technology. Highly porous and nitrogen-doped porous carbon materials have demonstrated outstanding performance in CO2 adsorption and separation (Petrovic et al., 2021; Kielbasa, 2023; Li et al., 2023). Specifically, micropores smaller than 1 nm are crucial for enhancing CO2 adsorption and selectivity (Liu et al., 2020). Nitrogen-doped porous carbon materials are considered ideal candidates for CO2 capture and gas separation due to their enhanced interactions and the ability to selectively absorb acidic CO2 molecules (Yang et al., 2018; Wu et al., 2023).
However, current methods for preparing porous carbon materials often face two challenges when balancing high adsorption performance and high separation performance: first, they tend to produce materials with a wide pore size distribution, and second, they result in lower nitrogen content in the materials. These challenges make it difficult to obtain porous carbon materials that simultaneously exhibit high adsorption capacity and high selectivity. Therefore, the preparation of nitrogen-doped porous carbon adsorbents with high adsorption capacity and selectivity remains a challenging task.
In this study, we propose a one-step carbonization activation method using chitosan as a carbon source, urea as a nitrogen source, and potassium hydroxide as an activator with freeze-thaw pre-mix treatment to synthesize porous carbon materials with an extremely narrow pore size distribution (pore size <1 nm) and high nitrogen content (up to 13.08 wt%). Chitosan dissolves in alkaline conditions through freeze explosion, driven by its water absorption mechanism. After full water absorption, freezing at a low temperature alters the physical state, breaking weak hydrogen bonds between chitosan molecules. Addition of alkaline solvents like urea or ammonia disrupts hydrogen bonds further. Repeated freezing and thawing achieve dissolution, ensuring uniform distribution of nitrogen-doped agent and activator in the material. This enhances their adsorption and separation performance for gas mixtures. These porous carbon materials are expected to play a significant role in applications such as gas storage and gas purification, offering a potential solution for reducing greenhouse gas emissions and achieving more environmentally sustainable energy production goals.
2 Experiment section
2.1 Chemicals
Chitosan, urea, KOH and HCl were all analytically pure and purchased from China Pharmaceutical Group Co., Ltd. Deionized water was self-made in the laboratory.
2.2 Synthesis methods
9.6 g chitosan, 16 g urea, and KOH were dissolved in 80 mL of deionized water and stirred at room temperature for 30 min to obtain suspensions with the mass ratio of KOH/chitosan at 1 and 2, respectively. The suspensions were frozen at −34°C for 48 h, and the frozen solids were thawed at room temperature to obtain gelatinous substances. The solids obtained by direct freeze-drying were carbonized for 2 h at 600°C, 700°C, and 800°C, respectively. The heating rate was controlled at 10°C/min. After KOH was removed by dilute hydrochloric acid, the material was washed with deionized water to neutral and dried at 105°C for 12 h. The resulting material was denoted as FDCK-x-t (x was the KOH/chitosan mass ratio, t was the activation temperature).
9.6 g chitosan was put into a tubular furnace and carbonized at 700°C for 2 h. 9.6 g chitosan, 9.6 g KOH, and 16 g urea were ground in a mortar to a uniformly mixed powder, which was carbonized at 700°C for 2 h. The heating rate was controlled at 10°C/min. After washing with dilute hydrochloric acid and deionized water, it is dried in an oven at 105°C for 12 h. The obtained carbon materials were recorded as C-700 and CK-1-700 as the control group.
2.3 Characterization
Scanning electron microscope (SEM, Hitachi SU8010, Japan) and transmission electron microscopy (TEM, JEM-2100F, Japan) were used to characterize the microstructure and morphology of the samples. The material’s internal structure was further characterized by X-ray diffraction (XRD, SmartLab, Japan). The correlation analysis of C, N, and O elements in the materials was carried out by elemental analyzer (Elementar-UNICUBE, Germany) and X-ray photoelectron spectroscopy (XPS, Thermofisher Nexsa, America).
The Micromeritics ASAP 2460 adsorption apparatus measured the samples’ N2 adsorption-desorption isotherms (77 K). The Brunauer Emmett Teller (BET) method was used to calculate the specific surface area of the sample. The nonlocal density functional theory (NLDFT) method derived pore size distribution (PSD) from the N2 isotherm adsorption branch, assuming a slit pore model. Under the relative pressure of 0.99, the total pore volume of the sample was calculated by N2 adsorption capacity, and the area and volume of micropores were estimated by the t-plot method. The pore volume of narrow micropores (<1 nm) was obtained from the CO2 adsorption data at 273 K.
2.4 Related calculation
2.4.1 IAST
The ideal adsorption solution theory proposed by Myers and Prausnitz was one of the mainstream models widely used to evaluate the adsorption selectivity of binary gas mixtures in recent years (Li J. et al., 2022; Yun et al., 2022). The adsorption selectivity of binary mixture gas can be defined as:
where q1 and q2 were the adsorption amounts of component 1 and component 2 in the binary gas mixture under partial pressures p1 and p2, respectively.
2.4.2 The isosteric heat of adsorption (Qst)
The heat of adsorption referred to the heat released when the temperature was certain. The adsorbate was adsorbed to the adsorbent. It reflected the energy variation, the heterogeneity of the material surface, and the interaction between adsorbent and gas molecules during the adsorption process, which was an important index to evaluate the regeneration performance of adsorbent materials. Isothermal adsorption heat was difficult to obtain by direct measurement and was generally accepted by the Clausius-Clapeyron equation (Qin et al., 2019).
where Ti was the adsorption temperature; Pi was the pressure corresponding to Ti when the same adsorption capacity was reached; R was the ideal gas constant.
3 Results and discussion
3.1 Analysis of morphology, phase structure and surface chemical properties
SEM was used to observe morphologies of C-700 and representative nitrogen-doped porous carbon FDCK-1-700. As shown in Figure 1, C-700 had a smooth, flat surface. FDCK-1-700 modified by urea and KOH showed a prominent honeycomb structure. Microscopic morphologies of FDCK-1-700 were further characterized by TEM (Figure 2). FDCK-1-700 had abundant wormlike micropores with disordered distribution, indicating the amorphous structure of the sample. In addition, according to the EDS element mapping diagram (Figure 2C), the uniform distributions of N and O elements in FDCK-1-700 were confirmed.
XRD was used to study the crystal structure of FDCK-1-700, as shown in Supplementary Figure S1A. Wide and weak peaks were observed at 24.5° and 43.5°, respectively, corresponding to (002) and (100) planes of amorphous graphite carbon (Qin et al., 2019). The results showed that the carbon material was amorphous, consistent with TEM observation.
The doped nitrogen of the material was evaluated by elemental analysis. The results of the element analysis are shown in Table 1. By comparing the nitrogen content of FDCK-1-t, the nitrogen content of FDCK-1-600 was the highest, being 13.08 wt%. It was noteworthy that compared with CK-1-700 (3.97 wt%), FCDK-1-700 showed a higher nitrogen content (7.07 wt%), demonstrating the superiority of nitrogen content for the one-step carbonization activation method of freeze-thaw premixed treatment. Under the same KOH/chitosan mass ratio, carbon material’s nitrogen content decreasedwith the activation temperature increase mainly because the destroyed N-containing functional groups increased with temperature (Rao et al., 2019). For example, the nitrogen content decreased from 13.08 wt% on FDCK-1-600 to 4.45 wt% on FDCK-1-800. Nitrogen content decreased from 6.47 wt% on FDCK-2-600 to 0.38 wt% on FDCK-2-800. The shallow nitrogen content of FDCK-2-800 may be caused by the high proportion of activators and the increase in activation temperature.
XPS further analyzed the surface chemical properties of the materials. Supplementary Figure S1B was the XPS patterns of FDCK-x-t, we can observe three kinds of different peaks: C1s peaks at 283.5 eV, O1s peaks at 531.2 eV, and an N1s peaks at 398.8 eV. Figure 3 was the peak fitting results of the high-resolution C1s, N1s, and O1s atlas. FDCK-x-t high-resolution C1s spectrum (Figure 3A) could be fitted into four characteristic peaks at 285.2 eV, 286.2 eV, 288.0 eV, and 290.1 eV, corresponding to C-O, C-O, C-C, and O-C=O, respectively (Cai et al., 2018). FDCK-x-t high-resolution O1s spectrum (Figure 3B) could be fitted into three characteristic peaks at 530.0 eV, 532.6 eV, and 534.3 eV, corresponding to C-O, C-OH, and carboxyl groups, respectively (Rehman and Park, 2019). Oxygen-containing functional groups (especially C-OH) could increase the electron density on the surface of carbon materials and make more CO2 molecules enter the porous carbon materials through electrostatic adsorption, which further improved the CO2 adsorption performance of materials.
FDCK-x-t high-resolution N 1s spectrum (Figure 3C) could be fitted into three characteristic peaks at 398.4 eV, 399.9 eV, and 401.1 eV, corresponding to pyridinic-N, pyrrolic-N, and quaternary-N, respectively (Gao et al., 2016). Pyridinic-N and pyrrolic-N were components of six-membered and five-membered ring systems, respectively. Pyridinic-N could transfer one electron, and pyrrolic-N could provide two electrons to the π-system (Pels et al., 1995). Thus, these nitrogen functional groups exhibited the basic properties of lewis bases and played a role in captured lewis-acidic CO2 molecules. Supplementary Table S1 showed the relative nitrogen content of the porous carbon materials. It could be seen that the relative content of quaternary-N in the adsorbent increased gradually with the increase of activation temperature. This may be due to the rising temperature, which converted part of pyridinic-N and pyrrolic-N into the more stable quaternary-N.
3.2 Pore structure
The pore structure of the adsorbent was studied by an N2 adsorption-desorption isotherm at 77 K. As shown in Figure 4, FDCK-x-t showed sharp N2 absorption at low pressure, and then sharp “knees” appeared at P/P0 <0.2, followed by the formation of the adsorption platform. According to IUPAC classification, it belonged to the I type isotherm, indicating that the pore structure of FDCK-x-t was mainly microporous. As shown in Figure 4C, no obvious N2 absorption was observed on the N2 adsorption-desorption isotherm for C-700, indicating that the porosity of chitosan carbon was very low without being modified by urea and KOH. CK-1-700 showed rapid N2 absorption at low pressure, followed by slow N2 absorption and a certain hysteresis loop, indicating that it had a certain amount of mesopores. FDCK-1-700 showed a sharp N2 absorption under low pressure, followed by a sharp “knee” and an adsorption platform, and the adsorption curve and desorption curve basically coincide. Pore size distribution and cumulative pore volume of FDCK-x-t in Figure 5 also confirmed that they were mainly microporous, and there were a large number of extremely narrow micropores (pore size <1 nm) distribution. It was worth noting that FDCK-1-700 prepared by a one-step carbonization activation method of freeze-thaw premixed treatment had a high distribution of extremely narrow micropores, while CK-1-700 prepared by physical mixing under the same conditions had a more significant proportion of micropores (1 nm∼2 nm) and a certain amount of mesoporous pores (2 nm∼3 nm) (Figure 5C). The results indicated that the superiority of a one-step carbonization activation method of freeze-thaw premixed treatment for customizing extremely narrow microporous nitrogen-doped carbon materials. It could be seen that micropores of FDCK-x-t smaller than 1 nm were mainly concentrated in 0.5∼0.6 nm and 0.8∼0.9 nm (Figure 5D).
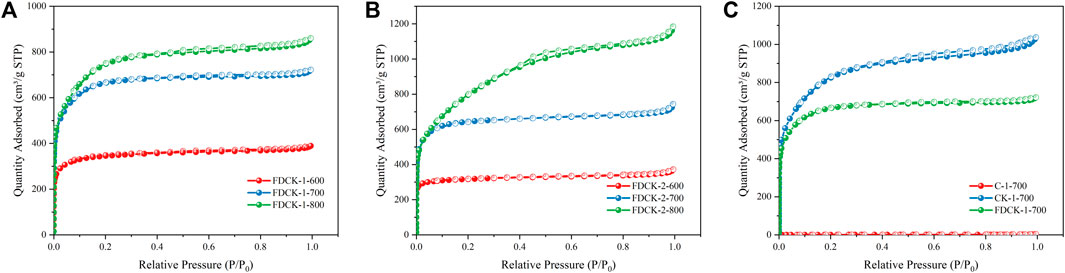
FIGURE 4. N2 adsorption-desorption isotherms at 77 K, FDCK-1-t (A), FDCK-2-t (B), and Contrast curve of different materials (C).
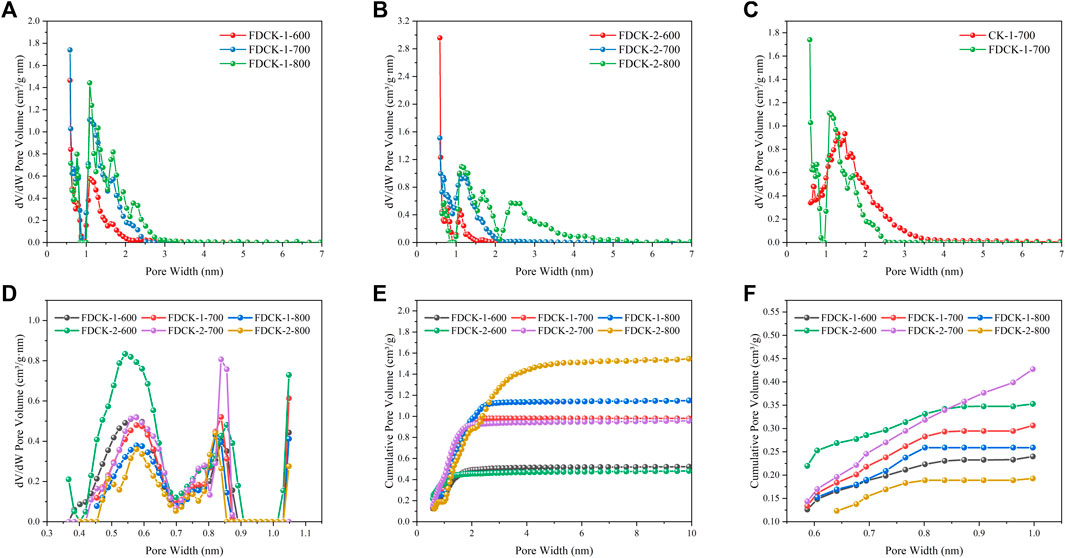
FIGURE 5. NLDFT pore size distribution curves (A–C), the extremely narrow micropore distribution curves (D), cumulative pore volume curves (E, F).
The specific surface area and pore volume parameters of C-700, CK-700, and FDCK-x-t are shown in Table 2. The specific surface area and total pore volume of the FDCK-x-t up to 2,897 m2/g and 1.83 cm3/g. The micropore area and micropore volume of FDCK-x-t reached the maximum at 700°C and decreased when the activation temperature increased to 800°C. This may be caused by the collapse of microporous channels due to high activation temperature. The results showed that 700°C was more suitable for carbonization activation of carbon precursors, which was consistent with previously reported results.
Chitosan could be dissolved in alkaline conditions by freeze explosion according to its water absorption mechanism. After chitosan fully absorbs water, the free water contained in it was frozen at a low temperature. Through the change in the physical state of water, the weak hydrogen bonds between chitosan molecules were dissociated. A small molecule of urea and hydrated metal ions could occupy the sites in chitosan molecules to form hydrogen bonds when alkaline solvents such as urea and KOH were added. Repeated freezing and thawing destroy the hydrogen bonds between chitosan molecules to achieve dissolution (Fan et al., 2009). In this study, the one-step carbonization activation method by freeze-thaw premixed treatment could make KOH and Urea more evenly dispersed into the carbon source to achieve uniform pore size distribution and higher N content, compared to the mechanical physical method.
3.3 Gas adsorption and separation performance
Figure 6 showed the CO2 adsorption isotherms of FDCK-x-t at 1 bar, 273 K, and 298 K. All adsorption and desorption curves coincide, showing good reversibility. There was no obvious hysteresis curve, indicating that the adsorbed gas could be well removed in the desorption process. The adsorbent was easy to regenerate under a vacuum without consuming excess energy. Supplementary Table S3 summarizes the CO2 adsorption capacity of the material under different conditions. The materials FDCK-1-700, FDCK-2-600, and FDCK-2-700 exhibit excellent CO2 adsorption performance. At 1 bar and 273 K, the CO2 adsorption capacities were as follows: FDCK-1-700 has a performance of 5.92 mmol/g, FDCK-2-600 shows a performance of 6.25 mmol/g, and FDCK-2-700 demonstrates a CO2 adsorption capacity of 6.97 mmol/g. At 1 bar and 298 K, the CO2 adsorption capacities were as follows: FDCK-1-700 has a performance of 3.11 mmol/g, FDCK-2-600 shows a performance of 3.77 mmol/g, and FDCK-2-700 demonstrates a CO2 adsorption capacity of 3.47 mmol/g.
This CO2 adsorption performance was superior to most porous carbon materials reported in recent years, comparison of CO2 adsorption performance in this work and recently reported data was shown in Table 3 and Figure 7. It was also superior to some other benchmark adsorbent materials, such as Zeolite 13X (3.5 mmol/g) (Jong-Seok Lee et al., 2002), Ni-4PYC (4.0 mmol/g) (Nandi S et al., 2015), CTF-TPC (4.2 mmol/g) (Dey et al., 2016), NJU-Bai (6.21 mmol/g) (Duan et al., 2012).
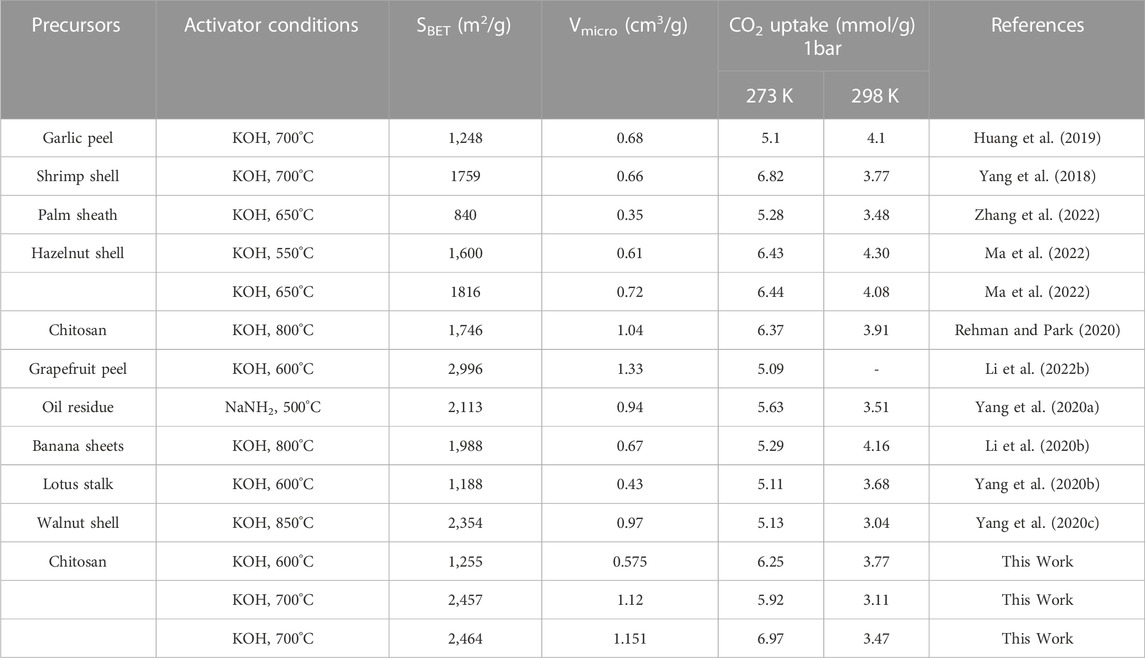
TABLE 3. Comparison of CO2 adsorption performance of biomass-derived porous carbon in recent 3 years.
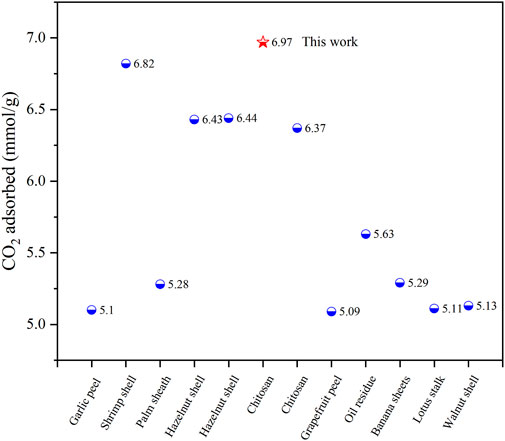
FIGURE 7. In recent 3 years, the CO2 performance of different biomass carbon materials was compared at 1 bar and 273 K.
It was worth noting that at the same KOH/chitosan ratio, FDCK-x-600 showed higher CO2 adsorption capacity at low pressure (<0.3 bar), which could be attributed to the high nitrogen content of FDCK-x-600. As shown in Supplementary Table S2, Under 273 K and 0.15 bar conditions, FDCK-2-600 had the maximum CO2 adsorption capacity of 2.56 mmol/g. This performance was superior to or comparable to some adsorbent materials, such as PSK-2-650 (2.0 mmol/g) (Zhang et al., 2022), SNMC-2-600 (2.21 mmol/g) (Zhang et al., 2019), NJU-Bai (1.5 mmol/g) (Duan et al., 2012).
To further study the effect of extremely narrow micropores and nitrogen content on CO2 absorption capacity. Figure 8 showed the regression model of extremely narrow micropore volume and CO2 absorption.
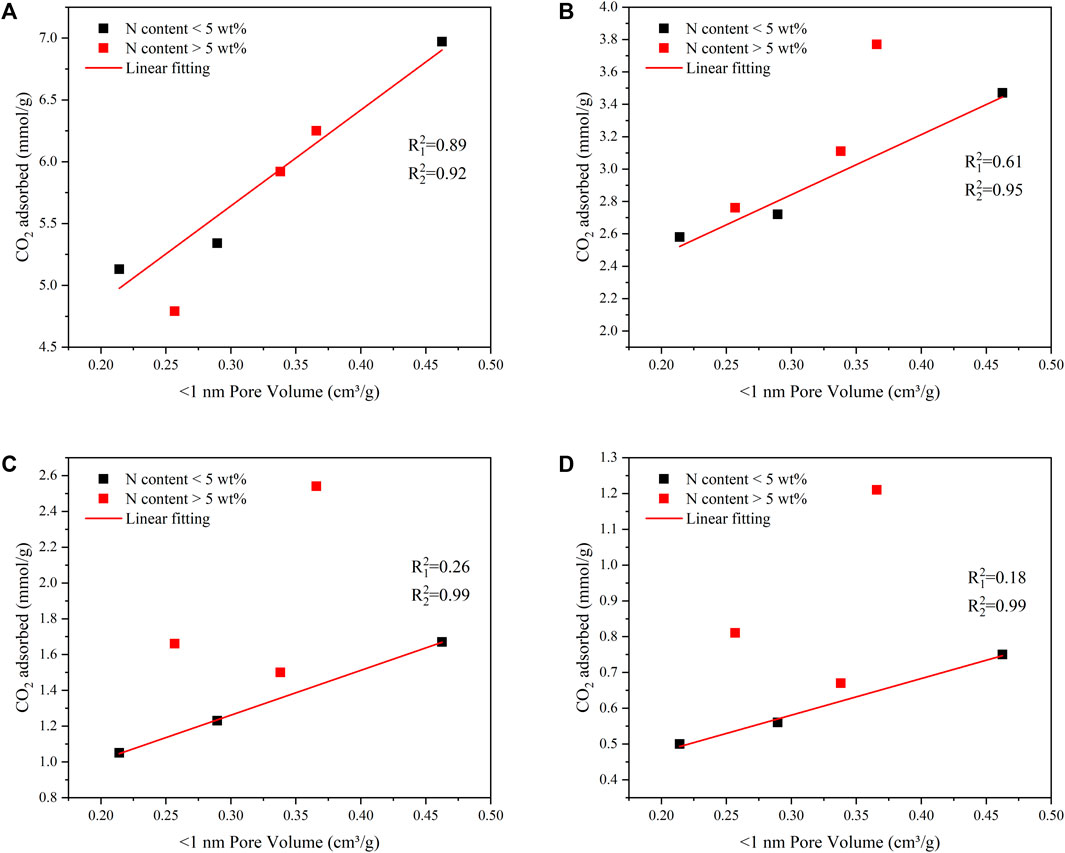
FIGURE 8. The linear relationship of the narrow pore volume and CO2 capture capacity at 1 bar and 273 K (A), 1 bar and 298 K (B), 0.15 bar and 273 K (C), 0.15 bar and 298 K (D).
The results showed that the adsorption capacity for CO2 gas was determined both by the extremely narrow pore volume and nitrogen content. When the pressure was 1 bar, the volume of extremely narrow micropores played a leading role in the CO2 adsorption performance of FDCK-x-t. However, under low pressure, the influence of the nitrogen content on the CO2 adsorption performance of materials was more significant.
To obtain the CO2/CH4 selectivity of FDCK-x-t, its CH4 adsorption capacity was measured under 1 bar, 273 K, and 298 K (the adsorption and desorption curves were shown in Supplementary Figure S3, and the adsorption capacity was demonstrated in Supplementary Table S3). The Langmuir-Freundlich model (Li Y. et al., 2020) was used to fit the CO2 and CH4 adsorption isotherms of FDCK-x-t to evaluate the relative performance of adsorbents through the adsorption isotherms of single-component gas (Figure 9 and Supplementary Figure S4). In consideration of the significant difference in gas concentration ratio (volume ratio) in natural biogas, the selectivity coefficient of CO2/CH4 at 10: 90 and 50: 50 was calculated in this study. Figure 10 and Table 4 showed and summarized the selectivity results calculated using IAST. It was noteworthy that FDCK-2-700 showed a relatively low IAST selectivity of 11.1 (7.5), although it showed a high CO2 adsorption performance of 6.97 mmol/g (3.47 mmol/g) at the conditions of 1 bar, 273 K (298 K) and CO2/CH4 (10: 90). FDCK-1-600 showed moderate CO2 adsorption performance at 1 bar, 273 K (298 K), but showed a surprising IAST selectivity of 52.7 (33.3) at this condition. This was mainly attributed to FDCK-1-600 having the highest nitrogen content (13.08 wt%). At the same time, compared with the preparation of nitrogen-doped porous carbon materials CK-1-700 by physical mixing under the same conditions, the IAST selectivity of FDCK-1-700 was improved by more than two times. The results showed that compared with the mechanical and physical mixing method, the one-step carbonization activation method of freeze-thaw premixed treatment had apparent advantages in gas adsorption and separation.
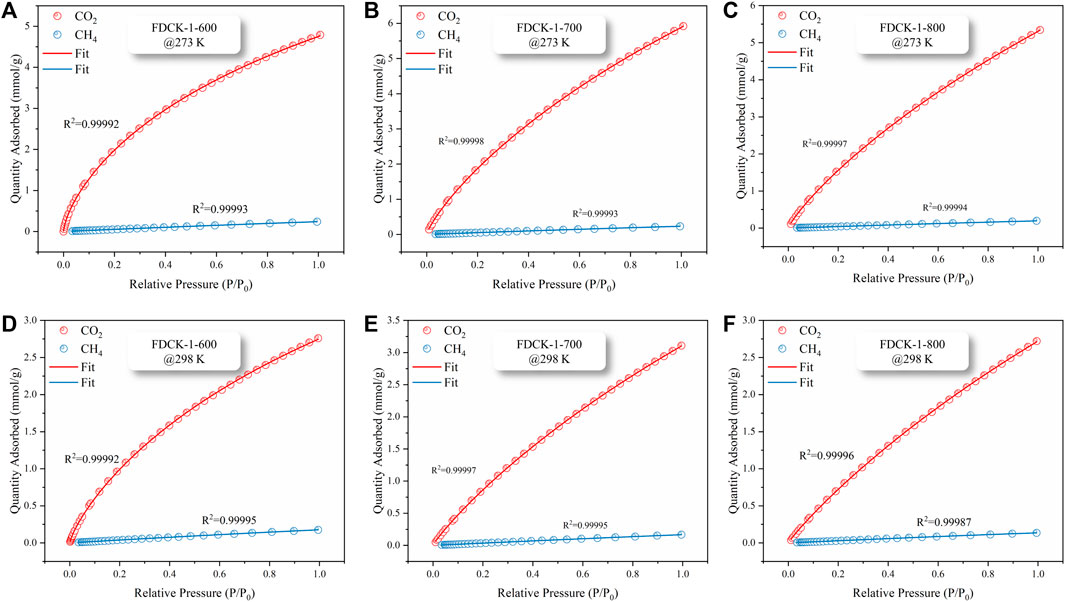
FIGURE 9. CO2 and CH4 isothermal adsorption fitting curves, FDCK-1-t at 273 K (A–C), FDCK-1-t at 298 K (D–F).
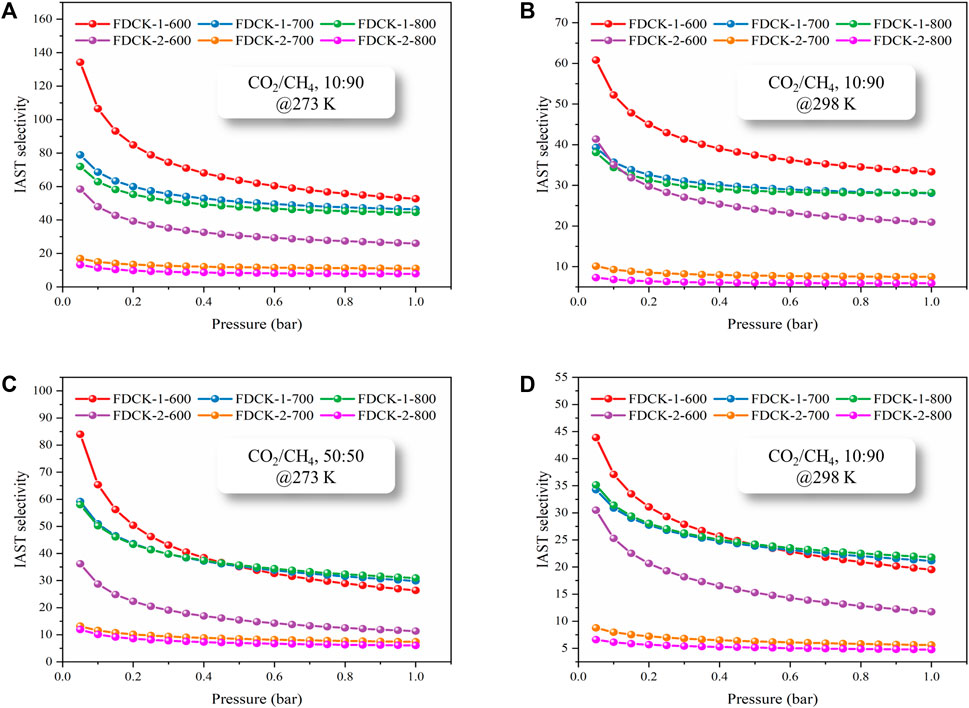
FIGURE 10. The IAST selectivity of FDCK-x-t at different conditions, CO2/CH4 10:90 and 273 K (A), CO2/CH4 10:90 and 298 K (B), CO2/CH4 50:50 and 273 K (C), CO2/CH4 50:50 and 298 K (D).
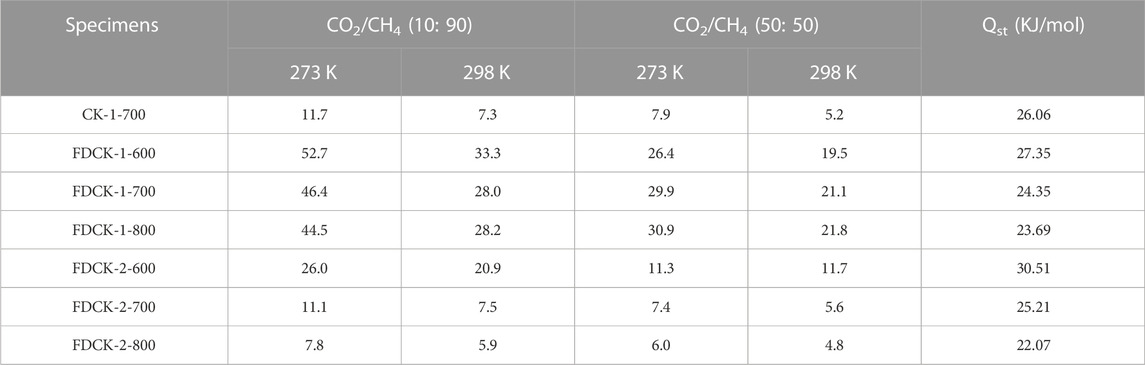
TABLE 4. IAST selectivity of the FDCK-x-t at different conditions and CO2 isosteric heat of adsorption.
The results showed that FDCK-x-t had good selective adsorption and separation performance for CO2/CH4, which was mainly attributed to the high nitrogen content in FDCK-x-t. The introduction of polar N-containing species in carbon materials enhanced the van der Waals force on CO2 intermolecular with quadrupole moment but had little effect on non-polar CH4 (Xiang et al., 2012). FDCK-x-t showed excellent IAST selectivity of 33.3 (52.7) and 21.8 (30.9) at 1 bar, 298 K (273 K) at two different gas mixtures (CO2/CH4, 10: 90; CO2/CH4, 50: 50). This property was superior to many porous carbon materials and some other reference adsorbent materials, as shown in Table 5.
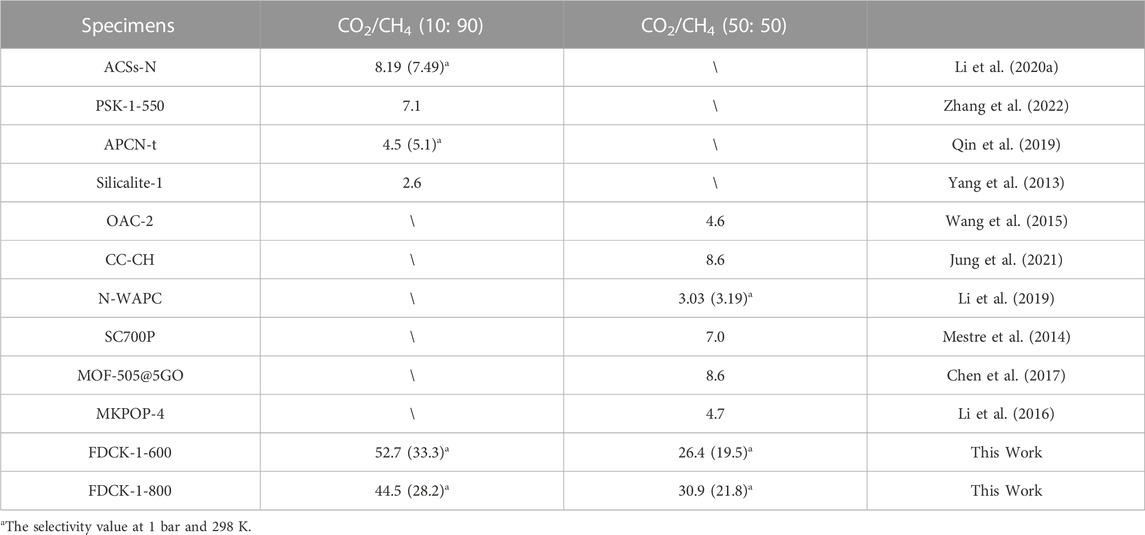
TABLE 5. The selectivity of the prepared porous carbon materials to different ratios of CO2/CH4 at 1 bar and 273 K was compared with that of reported adsorbent materials.
To verify the feasibility of FDCKs in practical application, a dynamic breakthrough experiment was carried out with FDCK-1-t in CO2/CH4 (10: 90) gas mixture. As shown in Figure 11, CH4 was detected earlier when the mixed gas passed through the column filled with adsorbent. For FDCK-1-600, the breakthrough point of CH4 was 3.7 min, the breakthrough point of CO2 was 13.2 min, and the penetration time was 9.5 min. For FDCK-1-700, the breakthrough point of CH4 was 5.2 min, the breakthrough point of CO2 was 10.9 min, and the penetration time was 5.7 min. For FDCK-1-800, the breakthrough point of CH4 was 5.5 min, the breakthrough point of CO2 was 9.4 min, and the penetration time was 3.9 min. It could be seen that FDCKs could selectively adsorb CO2 in the actual CO2 and CH4 mixture gas for biogas upgrading. The results showed that FDCKs had a broad application prospect in biogas selective adsorption.
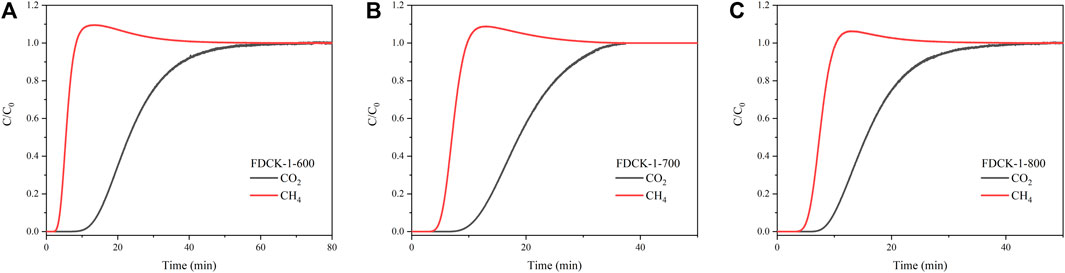
FIGURE 11. The breakthrough curves of CO2/CH4 (10:90) at 25°C and 1 bar, FDCK-1-600 (A), FDCK-1-700 (B), FDCK-1-800 (C).
Isosteric heat of adsorption (Qst) was a critical thermodynamic parameter to evaluate the interaction between adsorbent and adsorbed gas. According to the CO2 adsorption isotherms of adsorbent at 1 bar, 273 K, and 298 K, the relevant Qst values were calculated by the Clausius−Clapeyron equation. The Qst curve of FDCK-x-t was shown in Figure 12A. The initial Qst range of FDCK-x-t at low CO2 absorption capacity was 23.9∼39.3 kJ/mol, then gradually decreased to a certain value. It indicated that the surface of these adsorbents had heterogeneous adsorption. FDCK-x-t showed a high initial Qst value and then gradually decreased with the increase of CO2 loading, which may be because CO2 was first adsorbed in nitrogen-containing functional group adsorption sites and very narrow micropores, and then adsorbed in larger diameter micropores. The higher the Qst, the stronger the affinity of the adsorbent for CO2, which was conducive to the removal of CO2 from biogas. The overall Qst value varies from 21.2 to 39.3 kJ/mol, reflecting the physical adsorption characteristics of the adsorption process, indicating that the material was easy to regenerate.
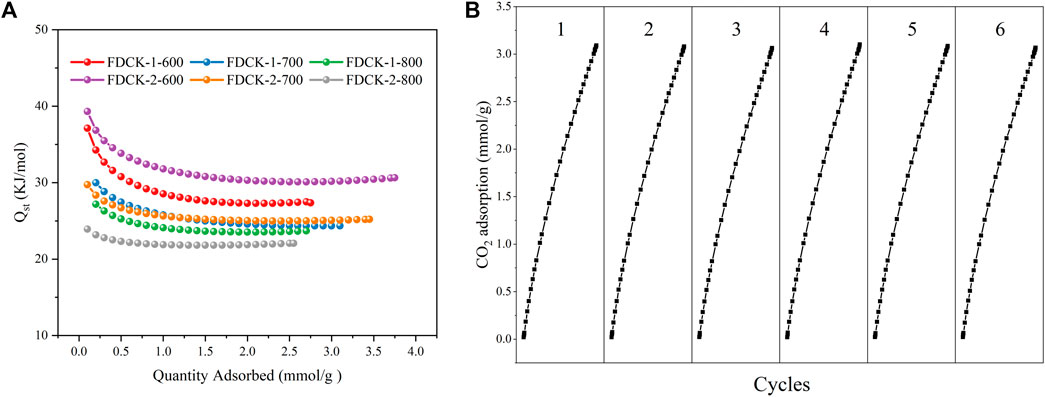
FIGURE 12. The CO2 isosteric heat of adsorption (A), recycling runs of CO2 adsorption-desorption on FDCK-1-700 at 1 bar and 298 K (B).
The regeneration performance was the key to the practical application of carbon dioxide adsorbents. Renewable and reused adsorbents not only save costs but also reduce the recovery and treatment of waste adsorbents. As shown in Figure 12B, six-time CO2 adsorption-desorption cycles were performed at 25°C and 1 bar on FDCK-1-700. In the cycle test, the adsorbent was degassed at 25°C for 1 h and then reused for adsorption measurement. The results showed that the adsorption capacity of FDCK-1-700 had little change after 6 times of continuous operation, indicating that the material had an excellent renewable performance.
4 Conclusion
In summary, we have successfully developed a one-step carbonization activation method with freeze-thaw pre-mix treatment to prepare extremely narrow microporous nitrogen-doped carbon materials, referred to as FDCKs. These FDCK materials exhibit outstanding performance in both CO2 adsorption and gas selective adsorption. They feature controllable nitrogen content, a high specific surface area, a large pore volume, and high CO2 adsorption capacity (6.97 mmol/g and 3.77 mmol/g at 1 bar, 273 K, and 298 K, respectively). Moreover, they demonstrate high selectivity at different CO2/CH4 ratios, offering significant potential for gas mixture separation. These materials maintain stability over multiple adsorption and desorption cycles, ensuring consistent performance through repeated use. In conclusion, FDCK materials hold great promise for various applications, particularly in gas separation and efficient purification, contributing to the reduction of greenhouse gas emissions and the achievement of more environmentally sustainable energy production goals.
Data availability statement
The original contributions presented in the study are included in the article/Supplementary Material, further inquiries can be directed to the corresponding authors.
Author contributions
HM: Data curation, Formal Analysis, Methodology, Validation, Writing–original draft, Writing–review and editing. KZ: Formal Analysis, Methodology, Supervision, Validation, Writing–original draft. ZG: Data curation, Formal Analysis, Resources, Validation, Writing–original draft. FC: Data curation, Supervision, Writing–original draft. LF: Formal Analysis, Supervision, Validation, Writing–original draft. BL: Methodology, Supervision, Writing–original draft. XQ: Resources, Writing–original draft. SW: Data curation, Validation, Writing–original draft. SC: Investigation, Methodology, Supervision, Validation, Writing–review and editing. BW: Resources, Supervision, Validation, Writing–review and editing. QM: Funding acquisition, Resources, Supervision, Writing–original draft.
Funding
The authors declare financial support was received for the research, authorship, and/or publication of this article. The authors would like to acknowledge the Opening Foundation of State Key Laboratory of High-efficiency Utilization of Coal and Green Chemical Engineering (Grant No. 2021-K68).
Conflict of interest
The authors declare that the research was conducted in the absence of any commercial or financial relationships that could be construed as a potential conflict of interest.
Publisher’s note
All claims expressed in this article are solely those of the authors and do not necessarily represent those of their affiliated organizations, or those of the publisher, the editors and the reviewers. Any product that may be evaluated in this article, or claim that may be made by its manufacturer, is not guaranteed or endorsed by the publisher.
Supplementary material
The Supplementary Material for this article can be found online at: https://www.frontiersin.org/articles/10.3389/fchem.2023.1333475/full#supplementary-material
References
Adnan, A. I., Ong, M. Y., Nomanbhay, S., Chew, K. W., and Show, P. L. (2019). Technologies for biogas upgrading to biomethane: a review. Bioengineering 6, 92–115. doi:10.3390/bioengineering6040092
Bernardo, M., Lapa, N., Fonseca, I., and Esteves, I. (2021). Biomass valorization to produce porous carbons: applications in CO2 capture and biogas upgrading to biomethane-A mini-review. Front. Energy Res. 9, 6. doi:10.3389/fenrg.2021.625188
Cai, W., Zhang, S., Hu, X., and Jaroniec, M. (2018). In situ synthesis of nitrogen-enriched activated carbons from Procambarus clarkii shells with enhanced CO2 adsorption performance. Energy & Fuels 32, 9701–9710. doi:10.1021/acs.energyfuels.8b02097
Chen, Y., Lv, D., Wu, J., Xiao, J., Xi, H., Xia, Q., et al. (2017). A new MOF-505@GO composite with high selectivity for CO2/CH4 and CO2/N2 separation. Chem. Eng. J. 308, 1065–1072. doi:10.1016/j.cej.2016.09.138
Dey, S., Bhunia, A., Esquivel, D., and Janiak, C. (2016). Covalent triazine-based frameworks (CTFs) from triptycene and fluorene motifs for CO2 adsorption. J. Mater. Chem. A 4, 6259–6263. doi:10.1039/c6ta00638h
Duan, J., Yang, Z., Bai, J., Zheng, B., Li, Y., and Li, S. (2012). Highly selective CO2 capture of an agw-type metal-organic framework with inserted amides: experimental and theoretical studies. Chem. Commun. (Camb) 48, 3058–3060. doi:10.1039/c2cc16231h
Fan, M., Hu, Q., and Shen, K. (2009). Preparation and structure of chitosan soluble in wide pH range. Carbohydr. Polym. 78, 66–71. doi:10.1016/j.carbpol.2009.03.031
Gao, F., Qu, J., Zhao, Z., Wang, Z., and Qiu, J. (2016). Nitrogen-doped activated carbon derived from prawn shells for high-performance supercapacitors. Electrochimica Acta 190, 1134–1141. doi:10.1016/j.electacta.2016.01.005
Huang, G., Wu, X., Hou, Y., and Cai, J. (2019). Sustainable porous carbons from garlic peel biowaste and KOH activation with an excellent CO2 adsorption performance. Biomass Convers. Biorefinery 10, 267–276. doi:10.1007/s13399-019-00412-6
Jong-Seok Lee, J.-H. K., Kim, J. H., Kim, J. T., Suh, J. K., Lee, J. M., and Lee, C. H. (2002). ‡ jin-tae kim,† jeong-kwon suh,† jung-min lee,† and chang-ha lee*,‡, adsorption equilibria of CO2 on zeolite 13X and zeolite X/activated carbon composite. J. Chem. Eng. Data 47, 1237–1242. doi:10.1021/je020050e
Jung, M., Park, J., Cho, S. Y., Elashery, S. E. A., Attia, N. F., and Oh, H. (2021). Flexible carbon sieve based on nanoporous carbon cloth for efficient CO2/CH4 separation. Surfaces Interfaces 23, 100960. doi:10.1016/j.surfin.2021.100960
Kielbasa, K. (2023). Activated biocarbons derived from molasses as new tailored CO2 adsorbents. Front. Chem. 11, 1184389. doi:10.3389/fchem.2023.1184389
Li, H., Ding, X., Zhao, Y.-C., and Han, B.-H. (2016). Preparation of mannitol-based ketal-linked porous organic polymers and their application for selective capture of carbon dioxide. Polymer 89, 112–118. doi:10.1016/j.polymer.2016.02.024
Li, J., Bao, A., Chen, J., and Bao, Y. (2022a). A green route to CO2 adsorption on biomass chitosan derived nitrogen-doped micropore-dominated carbon nanosheets by different activators. J. Environ. Chem. Eng. 10, 107021. doi:10.1016/j.jece.2021.107021
Li, J., Chen, X., Gong, J., Zhu, J., and Mijowska, E. (2020b). Deep insight into the pore size distribution of N-doped porous carbon materials on electrochemical energy storage and CO2 sorption. Diam. Relat. Mater. 105, 107802. doi:10.1016/j.diamond.2020.107802
Li, L. Y., Zhang, G. J., Wu, C. L., Liu, J., Li, G. Q., Wang, Y., et al. (2023). Novel nitrogen-enriched activated carbon with tunable microporosity from agricultural and plastic waste for CO2 adsorption. J. Environ. Chem. Eng. 11, 111257. doi:10.1016/j.jece.2023.111257
Li, W., Tu, W., Cheng, J., Yang, F., Wang, X., Li, L., et al. (2022b). Tuning N-doping thermal-process enables biomass-carbon surface modification for potential separation effect of CO2/CH4/N2. Sep. Purif. Technol. 282, 120001. doi:10.1016/j.seppur.2021.120001
Li, Y., Wang, S., Wang, B., Wang, Y., and Wei, J. (2020a). Sustainable biomass glucose-derived porous carbon spheres with high nitrogen doping: as a promising adsorbent for CO2/CH4/N2 adsorptive separation. Nanomaterials 10, 174. doi:10.3390/nano10010174
Li, Y., Xu, R., Wang, B., Wei, J., Wang, L., Shen, M., et al. (2019). Enhanced N-doped porous carbon derived from KOH-activated waste wool: a promising material for selective adsorption of CO2/CH4 and CH4/N2. Nanomater. (Basel) 9, 266. doi:10.3390/nano9020266
Liu, F., Zhang, Y., Zhang, P., Xu, M., Tan, T., Wang, J., et al. (2020). Facile preparation of N and O-rich porous carbon from palm sheath for highly selective separation of CO2/CH4/N2 gas-mixture. Chem. Eng. J. 399, 125812. doi:10.1016/j.cej.2020.125812
Ma, C., Lu, T., Shao, J., Huang, J., Hu, X., and Wang, L. (2022). Biomass derived nitrogen and sulfur co-doped porous carbons for efficient CO2 adsorption. Sep. Purif. Technol. 281, 119899. doi:10.1016/j.seppur.2021.119899
Mestre, A. S., Freire, C., Pires, J., Carvalho, A. P., and Pinto, M. L. (2014). High performance microspherical activated carbons for methane storage and landfill gas or biogas upgrade. J. Mater. Chem. A 2, 15337–15344. doi:10.1039/c4ta03242j
Nandi S, D. L. P., Daff, T. D., Rother, J., Liu, M., and Buchanan, W. (2015). A single-ligand ultra-microporous MOF for precombustion CO2 capture and hydrogen purification. Sci. Adv. 1, e1500421. doi:10.1126/sciadv.1500421
Pels, F. K. J., Moulijn, J., Zhu, Q., and Thomas, K. (1995). Evolution of nitrogen functionalities in carbonaceous materials during pyrolysis. Carbon 33, 1641–1653. doi:10.1016/0008-6223(95)00154-6
Petrovic, B., Gorbounov, M., and Masoudi Soltani, S. (2021). Influence of surface modification on selective CO2 adsorption: a technical review on mechanisms and methods. Microporous Mesoporous Mater. 312, 110751. doi:10.1016/j.micromeso.2020.110751
Qin, F., Guo, Z., Wang, J., Qu, S., Zuo, P., and Shen, W. (2019). Nitrogen-doped asphaltene-based porous carbon nanosheet for carbon dioxide capture. Appl. Surf. Sci. 491, 607–615. doi:10.1016/j.apsusc.2019.06.194
Rao, L., Liu, S., Wang, L., Ma, C., Wu, J., An, L., et al. (2019). N-doped porous carbons from low-temperature and single-step sodium amide activation of carbonized water chestnut shell with excellent CO2 capture performance. Chem. Eng. J. 359, 428–435. doi:10.1016/j.cej.2018.11.065
Rehman, A., and Park, S.-J. (2019). Environmental remediation by microporous carbon: an efficient contender for CO2 and methylene blue adsorption. J. CO2 Util. 34, 656–667. doi:10.1016/j.jcou.2019.08.015
Rehman, A., and Park, S.-J. (2020). From chitosan to urea-modified carbons: tailoring the ultra-microporosity for enhanced CO2 adsorption. Carbon 159, 625–637. doi:10.1016/j.carbon.2019.12.068
Singh, G., Lakhi, K. S., Sil, S., Bhosale, S. V., Kim, I., Albahily, K., et al. (2019). Biomass derived porous carbon for CO2 capture. Carbon 148, 164–186. doi:10.1016/j.carbon.2019.03.050
Wang, J., Krishna, R., Yang, J., and Deng, S. (2015). Hydroquinone and quinone-grafted porous carbons for highly selective CO2 capture from flue gases and natural gas upgrading. Environ. Sci. Technol. 49, 9364–9373. doi:10.1021/acs.est.5b01652
Wu, C. L., Zhang, G. J., Liu, J., Wang, Y., Zhao, Y. Q., and Li, G. Q. (2023). A green strategy to prepare nitrogen-oxygen co-doped porous carbons from macadamia nut shells for post-combustion CO2 capture and supercapacitors. J. Anal. Appl. Pyrolysis 171, 105952. doi:10.1016/j.jaap.2023.105952
Xiang, Z., Leng, S., and Cao, D. (2012). Functional group modification of metal–organic frameworks for CO2 capture. J. Phys. Chem. C 116, 10573–10579. doi:10.1021/jp3018875
Yang, F., Wang, J., Liu, L., Zhang, P., Yu, W., Deng, Q., et al. (2018). Synthesis of porous carbons with high N-content from shrimp shells for efficient CO2-capture and gas separation. ACS Sustain. Chem. Eng. 6, 15550–15559. doi:10.1021/acssuschemeng.8b03995
Yang, J., Li, J., Wang, W., Li, L., and Li, J. (2013). Adsorption of CO2, CH4, and N2 on 8-10-and 12-membered ring hydrophobic microporous high-silica Zeolites: DDR, silicalite-1, and beta. Industrial Eng. Chem. Res. 52, 17856–17864. doi:10.1021/ie403217n
Yang, P., Rao, L., Zhu, W., Wang, L., Ma, R., Chen, F., et al. (2020b). Porous carbons derived from sustainable biomass via a facile one-step synthesis strategy as efficient CO2 adsorbents. Industrial Eng. Chem. Res. 59, 6194–6201. doi:10.1021/acs.iecr.0c00073
Yang, Z., Guo, X., Zhang, G., and Xu, Y. (2020a). One-pot synthesis of high N-doped porous carbons derived from a N-rich oil palm biomass residue in low temperature for CO2 capture. Int. J. Energy Res. 44, 4875–4887. doi:10.1002/er.5287
Yang, Z., Zhang, G., Guo, X., and Xu, Y. (2020c). Designing a novel N-doped adsorbent with ultrahigh selectivity for CO2: waste biomass pyrolysis and two-step activation. Biomass Convers. Biorefinery 11, 2843–2854. doi:10.1007/s13399-020-00633-0
Yun, H., Kim, Y. J., Kim, S. B., Yoon, H. J., Kwak, S. K., and Lee, K. B. (2022). Preparation of copper-loaded porous carbons through hydrothermal carbonization and ZnCl2 activation and their application to selective CO adsorption: experimental and DFT calculation studies. J. Hazard Mater 426, 127816. doi:10.1016/j.jhazmat.2021.127816
Zhang, P., Zhong, Y., Ding, J., Wang, J., Xu, M., Deng, Q., et al. (2019). A new choice of polymer precursor for solvent-free method: preparation of N-enriched porous carbons for highly selective CO2 capture. Chem. Eng. J. 355, 963–973. doi:10.1016/j.cej.2018.08.219
Keywords: chitosan, nitrogen-doped porous carbon, CO2 adsorption, gas adsorption and separation, biomass
Citation: Min H, Zhang K, Guo Z, Chi F, Fu L, Li B, Qiao X, Wang S, Cao S, Wang B and Ma Q (2023) N-rich chitosan-derived porous carbon materials for efficient CO2 adsorption and gas separation. Front. Chem. 11:1333475. doi: 10.3389/fchem.2023.1333475
Received: 05 November 2023; Accepted: 01 December 2023;
Published: 14 December 2023.
Edited by:
Tamer S. Saleh, National Research Centre, EgyptReviewed by:
Jianbo Zhang, Northwest University, ChinaGuojie Zhang, Taiyuan University of Technology, China
Copyright © 2023 Min, Zhang, Guo, Chi, Fu, Li, Qiao, Wang, Cao, Wang and Ma. This is an open-access article distributed under the terms of the Creative Commons Attribution License (CC BY). The use, distribution or reproduction in other forums is permitted, provided the original author(s) and the copyright owner(s) are credited and that the original publication in this journal is cited, in accordance with accepted academic practice. No use, distribution or reproduction is permitted which does not comply with these terms.
*Correspondence: Shaokui Cao, Y2Fvc2hhb2t1aUB6enUuZWR1LmNu; Bing Wang, d2FuZ2Jpbmc2Njk2QDEyNi5jb20=