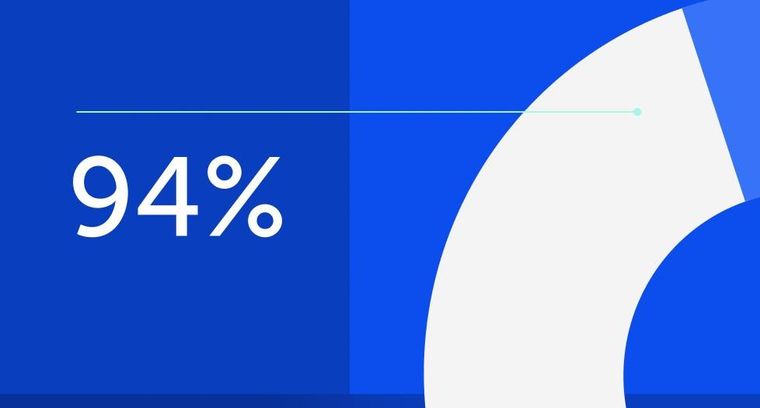
94% of researchers rate our articles as excellent or good
Learn more about the work of our research integrity team to safeguard the quality of each article we publish.
Find out more
MINI REVIEW article
Front. Chem., 17 October 2023
Sec. Medicinal and Pharmaceutical Chemistry
Volume 11 - 2023 | https://doi.org/10.3389/fchem.2023.1284292
Photodynamic Therapy (PDT) is an effective tumor treatment strategy that not only induces photocytotoxicity to kill tumor cells directly but also activates the immune system in the body to generate tumor-specific immunity, preventing cancer metastasis and recurrence. However, some limitations of PDT limit the therapeutic efficacy in deep tumors. Previous studies have used different types of nanoparticles (NPs) as drug carriers of photosensitizers (PSs) to overcome the shortcomings of PDT and improve therapeutic efficacy. Among them, bacterial outer membrane vesicles (OMVs) have natural advantages as carriers for PS delivery. In addition to the targeted delivery of PSs into tumor cells, their unique immunogenicity helps them to serve as immune adjuvants to enhance the PDT-induced immune effect, providing new ideas for photodynamic anticancer therapy. Therefore, in this review, we will introduce the biogenesis and anticancer functions of OMVs and the research on them as drug delivery carriers in PDT. Finally, we also discuss the challenges and prospects of OMVs as a versatile drug delivery carrier for photodynamic anticancer therapy.
Photodynamic Therapy (PDT) is a photochemical treatment triggered by light irradiation, and it has played a good role in the clinical treatment of tumors, precancerous lesions, infectious diseases, and other diseases as an alternative therapy in recent years. The principle of PDT is based on the excited photosensitizers (PSs) to produce reactive oxygen species (ROS) upon light irradiation at a specific wavelength, resulting in photocytotoxicity to damage the target tissue or cells (Kwiatkowski et al., 2018). Low-toxicity PSs, non-invasive light irradiation, and oxygen are the three core elements of PDT, so many scholars suggested that PDT is a promising treatment modality for cancer with better therapeutic effects and fewer invasiveness and side effects than conventional therapies (e.g., surgery, chemotherapy, and radiotherapy). PDT combined with endoscopic and fiberoptic light delivery techniques can expand the cancer treatment area, which may be inaccessible by surgery. The administration of light irradiation also exhibits high spatiotemporal control, reducing off-target toxicities (Overchuk et al., 2023). With the continuous deepening of research on PDT, researchers have found that in addition to killing tumor cells, PDT can also affect the body’s immune function and activate the immune system, resulting in tumor-specific immunity and preventing cancer metastasis and recurrence (Donohoe et al., 2019; Ji et al., 2022). In addition, the rare reports of drug resistance also make scholars believe that PDT will be a promising treatment modality for cancer (Lo et al., 2023). Some factors can affect the efficiency of PDT and its anti-tumor immune response, particularly the limitations of the inherent nature of PSs and light and complex tumor microenvironment (TME) (Hamblin and Abrahamse, 2021). First, most PSs are lipid-soluble drugs with poor stability, which need to be dissolved in organic solvents, making them unsuitable for intravenous administration. Second, although PSs will gather in tumor tissue, their low tumor selectivity and poor pharmacokinetics also cause photo-damage to normal tissue adjacent to tumor tissue and skin tissue (Kwiatkowski et al., 2018). Third, the excitation light of most existing PSs is short-wavelength ultraviolet-visible light (400–700 nm), and the strong absorption and scattering of light within this wavelength range by skin tissue make poor penetration depth, leading to PSs-induced PDT hard to achieve effective treatment of solid tumors in deep tissues. Although long-wavelength NIR light (700–1,100 nm) is used to activate some PSs with greater tissue penetration, the effect of NIR light on PDT in deeper tissues remains limited (Gao et al., 2022a). Fourth, solid tumors’ rapid growth with an underdeveloped vascular network causes a hypoxic state in TME that will limit the ROS level produced by PDT (Du et al., 2022; Li et al., 2023), and high concentrations of glutathione (GSH) in cancer cells can also consume ROS, leading to the inefficiency of PDT (Xiong et al., 2021; Wang et al., 2022b). Therefore, scholars have devoted themselves to seeking efficient and feasible methods to solve the above problems to promote the further development of PDT.
With the introduction of nanomaterials in PDT, based on their feature of the nanoscale structure, variable morphology, low biological toxicity, good biocompatibility, and easy modification, leading different types of nanoparticles as drug carriers of PSs provided more possibilities to overcome defects of PDT (Younus et al., 2023). For example, up-conversion nanoparticles (UCNPs) and persistent luminescence nanoparticles (PLNPs) can be used as drug delivery carriers to overcome the poor penetration depth of excitation light sources (Qiu et al., 2018; Fritzen et al., 2020). Gold nanoparticles (GNPs) as drug delivery carriers of PSs can generate additional photothermal effects under near-infrared (NIR) irradiation to improve the poor anti-cancer efficacy of PDT caused by hypoxia (Kim and Lee, 2018). Iron oxide nanoparticles (IONPs) can load PSs and then achieve MRI imaging guidance of tumors before or after PDT (Rajkumar and Prabaharan, 2017). Mesoporous silica nanoparticles (MSNs) have a large surface area and pore volume that can encapsulate PSs, small interfering RNA (siRNA), and chemotherapy drugs/prodrugs to achieve synergistic anti-tumor effects. Their surface can also be modified with functional groups such as folic acid (FA) and aptamers to provide the ability to target tumors and improve the distribution of PSs in the tumors (Prieto-Montero et al., 2023). Organic nanomaterials can be self-assembled into amphiphilic compounds, forming nanospheres or micelles to carry PSs and other drug formulations (Park et al., 2021). In addition, some modified nanomaterials can be responsive to the physiological characteristics of the tumor (e.g., enzyme and pH) and their microenvironment (e.g., hypoxia, GSH, hydrogen sulfide, H2O2, and H+) or externally applied physical stimuli (e.g., light, heat, ultrasound) to realize controlled release of PSs at specific sites (Zheng et al., 2020). However, scholars have raised concerns about biological toxicity, biodegradability, safety, immunogenicity, and tolerance of these nanomaterials after intravenous administration (Tong et al., 2022). These uncertainties limit the vast majority of research on the nanomaterials-based-delivery system of PSs to preclinical studies, which is a big challenge for them in achieving clinical conversion.
Extracellular vesicles (EVs) are nanosized lipid-bound vesicles with a size range of 30–150 nm in diameter released from cells. EVs have a lipid bilayer membrane that contributes to carrying exogenous anticancer drugs or therapeutic agents. In contrast to conventional synthetic nanomaterials, EVs have some advantages, such as low toxicity, low immunogenicity, high biocompatibility, and good stability in blood circulation (Elsharkasy et al., 2020; Meng et al., 2020). EVs also possess intrinsic cell-targeting properties and tissue-homing capabilities that liposomes do not have (Herrmann et al., 2021). In addition, through chemical methods, EVs can be gifted tumor-targeting ability by directly conjugating with targeting ligands (e.g., aptamers, antibodies, and peptides) (Jiang et al., 2022). Therefore, EVs as a natural drug delivery system have opened new frontiers for modern drug delivery, especially the delivery of PSs to the tumor tissues, representing a promising novel strategy of photodynamic anticancer therapy (Kusuzaki et al., 2017). More and more studies have confirmed that bacteria can secrete EVs similar to human cells, outer membrane vesicles (OMVs) (Sartorio et al., 2021; Liu et al., 2022). OMVs take part in many physiological processes of their parental bacteria, including nutrient acquisition, antibacterial defense, horizontal gene transfer, biofilm formation, virulence factor delivery, and intracellular/extracellular communication, and their biological function plays an essential role in the survival of the bacteria (Toyofuku et al., 2019). The application of OMVs as versatile tools for therapeutic approaches has attracted the attention of scholars because of their inherent ability to elicit an immune response in the body, especially in cancer immunotherapy (Zingl et al., 2021). At first, OMVs can be immunologic adjuvant or tumor vaccines for activating the body’s immune system in antitumor therapy (Chen et al., 2022; Hosseini-Giv et al., 2022; Long et al., 2022). Previous studies have confirmed that OMVs can specifically target tumor tissue and accumulate in tumor tissue after intravenous administration. OMVs cannot replicate and have higher safety than bacteria, indicating that OMVs have a natural advantage as a delivery carrier for antitumor drugs (Gujrati et al., 2014; Kim et al., 2017; Wang et al., 2019). Recent studies used OMVs as drug delivery carriers to enhance the PDT-induced immune effects and even achieve combinational photodynamic/chemo-/immunotherapy in tumor-bearing mice by simultaneously delivering chemotherapy drugs (Li et al., 2022; Zhuang et al., 2022). These works provide a novel idea for photodynamic anticancer therapy by developing OMVs-based drug delivery carriers.
Therefore, in this review, we will introduce the biogenesis and anticancer functions of OMVs and the research on them as drug delivery carriers in PDT. Finally, we also discuss the challenges and prospects of OMVs as a drug delivery carrier for photodynamic anticancer therapy.
OMVs are non-living spherical double-layer vesicle structures released by Gram-negative bacteria and some Gram-positive bacteria, mainly composed of the peptidoglycan layer and periplasm, containing bacterial bioactive proteins, lipids, nucleic acids, and metabolites (Jahromi and Fuhrmann, 2021; Ozkocak et al., 2022). Gram-negative and Gram-positive bacteria have obvious differences in cell structure, leading to different biogenesis processes and membrane components between their OMVs. Gram-negative bacteria have an outer membrane (OM) structure caused by the thin peptidoglycan membrane in the periplasm separating the OM from the inner membrane. OM is composed mainly of lipopolysaccharide (LPS), while the inner membrane riched phospholipid. OMVs mainly originate from the budding of the OM and explosive cell lysis (Zingl et al., 2021). The budding of OM will produce the classic OMVs after cell envelope disturbance caused by the following changes, as shown as Figure 1 (Kulp and Kuehn, 2010; Roier et al., 2016; Sartorio et al., 2021). a) the outer membrane-peptidoglycan protein (OM-PG) linkages are directly lost or by the movement of the linking protein; b) the local accumulation of peptidoglycan fragments or misfolded proteins in the periplasm; c) the accumulation of phospholipids in the outer leaflet of the OM via the downregulation of VacJ/Yrb ABC transporter; d) the local enrichment of LPS species with anionic charges; e) the hydrophobic molecules insert into the OM. These OMVs are characterized as having no cytoplasmic components as their content because the inner membrane remains intact (Wang et al., 2022a). Other types of OMVs of Gram-negative bacteria include outer-inner membrane vesicles (OIMVs) and explosive outer membrane vesicles (EOMVs) caused by explosive cell lysis (Pérez-Cruz et al., 2015; Delgado et al., 2019; Mandal et al., 2021). OIMV are the results of the vesicles containing cytoplasmic contents (such as DNA), which originated from the inner membrane protruding into the periplasm after endolysin degrading the peptidoglycan cell wall, are squeezed out from the cell surface with surrounding OM. EOMVs, randomly encapsulated released DNA vesicles, are formed by the re-circulation, aggregation, and self-assembly of the shattered membrane fragments induced by DNA damage-induced cell lysis. Gram-positive bacteria have a thick peptidoglycan layer outside but lack OM structure, and the released vesicles are derived from the cytoplasmic membrane (CM) (Toyofuku et al., 2023). Existing research suggests that endolysin-triggered “bubbling cell death” in Gram-positive bacteria forms the cytoplasmic membrane vesicles (CMVs), which can contain membrane and cytoplasmic components. Some studies have also observed specialized types of MVs with tube-shaped mechanical structures, which may be derived from the tube-like protrusions of the cytoplasmic membrane of Gram-positive bacteria or the tube-like outer membrane of Gram-negative bacteria. Thus, the biogenesis processes of OMVs and CMVs differ, indicating that they differ in origin, components, and function. Tian and others summarized the differences between OMVs and CMVs in their published review (see reference Tian et al., 2023). The production of OMVs is a spontaneous bacterial behavior in response to external stress. So the biogenesis processes of OMVs would be affected by temperature, growth environment, Quorum sensing (QS), bacterial growth stage, and hereditary factors, and even their contents will change accordingly, affecting their biological functions.
OMVs are derived from the OM and have the outer membrane proteins (OMP), LPS, lipoproteins (LPP), and peptidoglycan, which belong to pathogen-related molecular patterns (PAMPs). PAMPs can bind to pathogen recognition receptors on immune cells, activating the innate immune response. OMVs can passively target the tumor via the enhanced permeability and retention (EPR) effect (Suri et al., 2022). With these characteristics, OMVs have demonstrated unique advantages in antitumor therapy. To date, the application of OMVs’ antitumor action mainly includes tumor vaccines, cancer immunotherapeutic agents, and antitumor agent delivery carriers, as shown as Figure 2).
FIGURE 2. Schematic illustration of the application of OMVs’ antitumor action. The therapeutic strategy of OMVs mainly includes tumor vaccines, cancer immunotherapeutic agents, and antitumor agent delivery carriers.
Cancer immunotherapy activates the host immune system to attack and eliminate tumor cells and has become an important treatment strategy, including vaccines, cytokines, immune checkpoint inhibitors, etc. Among them, tumor vaccines based on their safety and reliability have also been a hot research field in recent years, mainly utilizing tumor-related antigens to overcome immune suppression caused by tumors, leading to enhancing immunogenicity and activating the immune system. OMVs possess intrinsic immunogenicity, and their tumor antigens can further stimulate the body’s immune response, which is beneficial for their development and application as tumor vaccines (Gao et al., 2022b). OMVs-based anti-tumor vaccines are mainly developed from functionally modified by genetic engineering of bacteria to cause a foreign protein to be expressed in the vesicle lumen or on its membrane surface (Wang et al., 2022b). For example, some antigens can be fused with the protein from the OMVs, such as cytolysinA (ClyA), hemoglobin protein (Hbp), and OMP, to form chimeric protein on the OMV membrane. Cheng and others used ClyA to fuse the catcher protein SpC/SnC, and the specific antigen protein binds to the tag protein SpT/SnT by a peptide bond, leading to various tumor antigens can be rapidly and simultaneously displayed on the OMVs surface via the binding to protein tags. This OMV-based vaccine platform elicits a specific anti-tumor immune response via specifically presenting antigens onto the OMV surface to inhibit metastasis and growth of tumors and induce long-term immune memory (Cheng et al., 2021). Huang and others used genetic recombination technology to fuse the thioredoxin (Trx) gene and the full-length mouse BFGF gene into the pThioHisA plasmid. The plasmids were transformed into E. coli DH5α competent cells to induce Trx-BFGF fusion protein expression and localized in the periplasmic space under the guidance of the Trx protein and loaded onto OMVs to form BFGF-modified OMVs (BFGF-OMVs). They found that BFGF-OMVs is a novel therapeutic tumor vaccine that can induce the body to produce antiangiogenesis autoantibodies to inhibit tumor angiogenesis through active immunization. The persistent anti-BFGF autoantibodies can be observed in tumor-bearing mice after only three times BFGF-OMVs intervention, which exerts tumor suppression effects (Huang et al., 2020).
OMVs have not only the immune regulatory ability but also have antitumor effects and can be used as cancer immunotherapeutic agents. Kim and others investigated the potential of OMVs as an immunotherapeutic agent for cancer. At first, They harvested the OMVs derived from lipid A acyltransferase (msbB) mutant E. coli, whose gene encoding the lipid component of lipopolysaccharide (lipid A acyltransferase) had been inactivated to avoid possible adverse effects by endotoxin lipopolysaccharide. After being injected intravenously via the tail vein, OMVs can target tumors and accumulate in tumor tissue without any targeted modification. OMVs induced long-term antitumor immune responses in tumor-bearing mice models and eradicated tumors by producing IFN-γ within the tumor microenvironment (Kim et al., 2017).
The double-layer membrane structure, tumor-targeting ability, gene or membrane modifiability, and the ability of long-distance delivery of active molecules of OMVs make them promising candidates as new types of antitumor agent delivery carriers. In particular, OMVs from certain bacteria can disrupt the mucosal and epithelial barrier integrity to facilitate OMVs to pass biological barriers (Jahromi and Fuhrmann, 2021). Doxorubicin (DOX) is a broad-spectrum antineoplastic agent, but it non-specifically targets all kinds of cells, resulting in adverse effects such as cardiotoxicity, myelosuppression, and immunosuppression. To alleviate the cytotoxicity of DOX to normal tissues, Kuerban and others utilized OMVs from attenuated Klebsiella pneumonia as the delivery carriers to load DOX. In their study, DOX and OMVs were gently mixed in PBS and then incubated at 37°C to form the DOX-loaded OMVs (DOX-OMV). DOX was efficiently transported into non-small-cell lung cancer cells by OMVs, leading to intensive cytotoxic effects and cell apoptosis. While OMVs elicit appropriate immune responses to enhance the antitumor effect of DOX with no evident toxic side effects and adverse reactions in tumor-bearing nude mice (Kuerban et al., 2020). Gujrati and others first demonstrated the possibility of loading siRNA into the OMVs to achieve tumor-targeted siRNA delivery. They employed the OMVs derived from the msbB mutant E. coli transformed with pGEX4T1-ClyA-affibody construct as the delivery carriers. These OMVs display a human epidermal growth factor receptor 2 (HER2)-specific affibody in the membrane as a targeting ligand, named AffiHER2OMVs. The TAMRA-labeled siRNA targeting kinesin spindle protein (KSP) as a model therapeutic agent was loaded into AffiHER2OMVs using electroporation. The siRNA-loaded OMVs can induce targeted gene silencing and significant inhibition of cell proliferation in HER2-overexpressing cell lines and tumor growth regression in tumor animal models rather than elicit their antitumor effects by overstimulating inflammatory or immunological pathways (Gujrati et al., 2014). Chen and others mixed E. coli-derived OMVs and AuNPs in a homogenizer to form stable complex Au-OMVs, indicating that OMVs can load nanoparticles on their surface. They found that Au-OMVs possess the ability to produce immuno-modulatory and radiosensitizing effects. Au-OMVs induce high intracellular ROS, chemotaxis of macrophages, and high levels of TNF- α, leading to a specific cytotoxic effect on glioma cells and reducing radiotherapy dosage in tumor-bearing mice (Chen et al., 2021). Shi and others selected the E. coli-derived OMVs to encapsulate 5-fluorouracil (5-FU)-loaded MSNs by high-pressure co-extrusion. OMVs as delivery carriers can improve the stability of the 5-FU-loaded MSNs, reduce the leakage of 5-FU, and enhance the accumulation of 5-FU-loaded MSNs in colon cancer cells that contribute to MSNs accurately releasing 5-FU in the lesion (Shi et al., 2020). Lately, Cui and others designed an efficient miRNA nano-delivery system for tumor gene therapy based on the PD1 displayed OMVs to encapsulate zeolitic imidazolate framework-8 (ZIF-8) containing miR-34a. The engineered OMVs exhibited high miRNA delivery efficiency, tumor targeting, immune activation, and checkpoint inhibition, representing promising biomimetic nano-delivery carriers for the intracellular delivery of miRNA to enhance tumor therapeutic efficacy (Cui et al., 2023).
The above research indicates that OMVs have shown multiple potential applications and research value in tumor therapy. Therefore, we wonder how to utilize the advantages of OMVs in tumor treatment to bring some new thinking to the research of photodynamic anticancer therapy.
In recent years, the stimulating effect of OMVs in tumor immune response and their ability to act as drug carriers have attracted scholars’ attention and have inspired their innovative attempts to apply OMVs to photodynamic anticancer therapy, as shown as Table 1. Zhang and others used attenuated Salmonella-derived OMVs as versatile drug carriers to overcome hypoxia in tumor tissue and improve PDT-induced immune response. They first synthesized a nano-complex (CAT-Ce6) from hydrophilic catalase (CAT) and hydrophobic PS (Chlorin e6, Ce6) via self-assembly. CAT-Ce6, as an amphiphilic complex, can avoid the aggregation of Ce6 in the aqueous solution, improving biocompatibility and distribution of Ce6 in tumor tissues. CAT-Ce6 has the catalytic activity of CAT, which can decompose H2O2 in tumor tissues to generate oxygen to improve the hypoxic state, thereby enhancing the photodynamic killing effect on tumors. Then they modified the programmed death-ligand 1 antibody (aPDL1) on the surface of OMVs by extrusion, forming OMV-aPDL1 as the delivery carriers for CAT-Ce6. After transferring CAT-Ce6 to tumor tissues, OMV-aPDL1 can activate anti-tumor immune responses and dendritic cells (DCs) to induce CD8 T cells to move to tumor tissues. While aPDL1 can blockade PD-1 to relieve the immunosuppressive effect, leading to eliminating tumor immune escape. Their experimental results prove that CAT-Ce6@OMV-aPDL1 can achieve the synergy of oxygenerated PDT and immunity for improving the efficiency of cancer treatment and even induced Immunological memory effect in mice receiving treatment twice (Zhang et al., 2022). Peng and others utilized OMV’s ability to penetrate the stratum corneum (SC) and designed OMV-based versatile delivery nanoplatforms for skin melanoma treatment, named I-P-OMVs. They harvest the attenuated OMVs derived from TRAIL gene-transformed E. coli and then modified αvβ3 integrin targeting peptide (RGP) on the surface of OMVs and load the indocyanine green (ICG) by simply co-incubation to form I-P-OMVs. Based on their design features, I-P-OMVs can penetrate SC via follicle routes and target skin melanoma through a specific binding with αvβ3 integrin at the surface of melanoma cells. The ICG loaded by I-P-OMVs can induce photodynamic/photothermal effects in melanoma spheroids under NIR irritation, leading to a high level of ROS and hyperthermia to kill melanoma cells. The photothermal effects can destroy OMVs to release TRAIL, which binds to death receptors in melanoma cells’ surface, activating the apoptosis in residual melanoma cells. These synergistic therapeutic effects can prevent cell proliferation and invasion of melanoma cells by interfering with the relevant genes and proteins and delaying the progression, relapse, and metastasis of melanoma with good biosafety in the mice model, indicating that I-P-OMVs represent a feasible OMVs-based versatile nano-platform with great potential in photo-treatment of melanoma (Peng et al., 2020). Li and others developed a macrophage-mediated OMV-based delivery carrier (OMVs@M) to transmit Ce6 and the chemotherapeutic drug doxorubicin (DOX) into triple-negative breast tumors, providing combinational photodynamic/chemo-/immunotherapy to eradicate and prevent tumor metastasis. In their research, E. coli -derived OMVs were uptaken by macrophages to form OMVs@M as delivery carriers, which can enhance OMVs’ tumor-targeting ability and safety via macrophage-mediated delivery, and OMVs can lead to M2-to-M1 polarization of macrophages and activate pyroptosis to improve antitumor immunity. Based on this bioengineering strategy, OMVs containing Ce6/DOX were uptaken by macrophages to form a versatile therapeutic platform (DOX/Ce6-OMVs@M) for laser-triggered photodynamic effect and synergic antitumor therapy (Li et al., 2022). Zhuang and others constructed versatile E.coli OMV-based hybrid nanovesicles with phytochemical features to enhance photodynamic effects-promoted immunotherapy. Interestingly, they chose the plant-derived thylakoid membranes (Tk) instead of conventional PSs because these special membranes contain various enzymes and photosystems (photosensitive chlorophyll) that can induce efficient photodynamic effects. OMVs are fused with thylakoid membranes by co-extrusion to form bacteria-plant hybrid vesicles (BPNs). BPNs can target tumor tissues, stimulate the immune response, and even generate ROS under 660 nm light irradiation, indicating that BPNs integrated the immune-modulatory functions of OMVs as cancer vaccines with the photodynamic effects of Tk as PS. After only one light irradiation, BPNs can eliminate tumor growth and prevent tumor metastasis without any evident side effects in colon cancer CT26 tumor xenografted mice, suggesting that phytochemical-engineered OMVs represent a novel versatile membrane-based hybrid system for highly efficient tumor treatment (Zhuang et al., 2022). The above-presented works demonstrate that OMVs could be the ideal candidates as drug delivery carriers in photodynamic anticancer therapy and combine with other strategies for synergic antitumor therapy, indicating that OMVs may be an efficient and feasible method to solve the shortcomings of PDT and will promote the further development of PDT.
Of course, we also realize that published studies of OMVs as drug delivery carriers in photodynamic anticancer therapy are still limited. This situation can be attributed to OMVs having many challenges in their preparation and application.
Although OMVs can not replicate like their parent bacteria and cause disease by themselves, they have bioactive proteins, lipids, nucleic acids, virulence factors, and metabolites from their parent bacteria. They may disrupt the microenvironment of target organs in complex human environments, leading to unexpected complications. For example, OMVs’ immunogenicity can activate the body’s immune response and cause immune storms, leading to adverse reactions and death (Matías et al., 2020). Qing and others observed that healthy Balb/c mice did not tolerate the OMVs treatments well after giving single-dose or multiple-dose intravenous (i.v.) injection regimes of the OMVs, and they speculated that the died mice died of some systemic inflammatory response (Qing et al., 2020). Hence, safety is the first challenge of OMVs as drug delivery carriers. How to attenuate the toxicity of OMVs with decreased levels of LPS is essential to OMV application (Li et al., 2020). However, using the detergents, genetic engineering, and physical or chemical methods to attenuate the toxicity may lead to the loss of bacterial antigens and lipoproteins and a decrease in the OMVs’ inherent immunogenicity, leading to OMVs will lose the immunoadjuvant effect (van de Waterbeemd et al., 2010; Li et al., 2020).
OMVs are naturally released from bacteria but in low quantities. Temperature, stress, low temperature, nutrient deficiency, antibiotics, and phages may increase OMV production during culture (Schwechheimer and Kuehn, 2015). But the large-scale production of OMVs still faces some difficulties and cannot meet the current clinical application standards. Some studies have shown that detergents (e.g., deoxycholate or sodium dodecyl sulfate) can stimulate bacteria to achieve higher production of OMVs but can cause unexpected changes in the properties of OMVs. Other methods have been reported to increase the production of OMVs, such as physical (e.g., sonication) or chemical (e.g., ethylenediaminetetraacetic acid, EDTA) treatment and genetic modifications. But scholars are also concerned that these methods could affect the size, stability, and composition of OMVs, resulting in the loss of the desired activity of OMVs (Naskar et al., 2021).
The biogenesis processes of OMVs would be affected by bacterial strains, growth conditions, and stages of bacterial growth, leading to the heterogeneity between bacterial strains and species. Bitto and others compared the OMVs produced by S. aureus, P. aeruginosa, and H. pylori, and they suggested that the production, size distribution, and protein cargo quantity of OMVs vary within and between bacterial species, as shown as Table 2 (Bitto et al., 2021). Take protein content as an example, the amount of OMP/periplasmic proteins packaged into E. coli-derived OMVs is 0.2%, whereas 12% of those proteins in Neisseria meningitidis -derived OMVs (Lieberman, 2022). So far, the protein assay is still the prioritized method of OMVs quantification, such as Bradford and bicinchoninic acid (BCA) (Bitto et al., 2021). The heterogeneity of OMVs may significantly affect their protein content, indicating that the protein concentration may not accurately reflect the OMVs’ quantity. In addition, OMVs are not uniformly sized vesicles. Their size can also influence their cargo content and the mechanisms of their entry into host cells (Turner et al., 2018).
TABLE 2. The quantitative comparison of size, number, protein, BMV-associated DNA, and RNA of S. aureus, P. aeruginosa, and H. pylori strains.
The existing isolation and purification technologies of OMVs include ultracentrifugation, ultrafiltration, protein precipitation, and affinity isolation. Finally, harvested OMVs need to be incubated on agar growth plates to ensure no bacteria are present in the OMVs (Collins et al., 2021; Michel and Gaborski, 2022). Li and others have a summary of methods used to isolate OMVs in their published review, including the isolation principle, advantages, and disadvantages (see reference Li et al., 2020). Different isolation and purification technologies have advantages and limitations, so they are hard to meet the production demand of pure and specific OMVs. In addition, there is still no standard for isolating OMVs, and researchers often choose the isolation and purification methods based on their research purposes and experimental conditions. But existing each technique may alter the properties of isolated OMVs, so new reliable methods are needed to be developed to yield OMVs with desired purity and quantity.
The drug loading methods determine the PSs loading efficiency and membrane integrity of OMVs. There are usually two methods for drug loading in OMVs. The first method is to load drugs during the biogenesis process of OMVs via genetic engineering to modify parent bacteria or add drugs in the bacterial culture medium, leading to bacteria releasing the drug-loaded OMVs. The detailed mechanisms of biogenesis and “cargo” selectivity of OMVs are still unclear. So the drug loading efficiency of this method still needs further research and evaluation. The second method is OMV engineering which is based on the lipid bilayer structure of OMVs to load hydrophilic or hydrophobic compounds. Hydrophobic PSs can be passively loaded into isolated OMVs through co-incubation without any active substances. Hydrophilic PSs can penetrate into isolated OMVs through ultrasound, electroporation, or extrusion. There are many methods to encapsulate drugs, but the drug encapsulation efficiency is unsatisfactory (Ding et al., 2022). And some drug-loading methods may affect the size, charge potential, membrane rigidity, and integrity of OMVs, resulting in the endocytosis of any delivered drugs by target cells will be potentially altered.
PDT has become an effective strategy for tumor treatment due to its unique action mechanism and low systemic toxicity. PDT has been clinically applied in treating superficial tumors and precancerous lesions with better therapeutic effects and application prospects. However, some limitations of PDT limit its therapeutic efficacy and widespread clinical application in deep-seated tumors. In recent years, researchers have made significant progress in the design of PSs, which has progressed from the first and second generations to the third generation, improving many shortcomings of traditional PSs. With the help of drug delivery carriers, PSs can achieve tumor targeting, TME/stimuli-response, and synergistic treatment of photodynamic/photothermal/chemo-/immuno-/gene therapy, providing new hope for precise and efficient tumor treatment. Existing research has confirmed that OMVs can also serve as delivery carriers like human EVs, and their unique immunogenicity contributes to they can be used as immune adjuvants to enhance the immune effect induced by PDT. OMVs can provide some positive benefits to photodynamic anticancer therapy and open a new avenue for designing versatile drug delivery carriers. We have realized that OMVs will have broad application prospects in photodynamic anticancer therapy. But many challenges need to be overcome, such as finding a way to balance detoxification and retaining enough efficacy of adjuvanticity, optimizing the purification method of OMVs, increasing production, and improving drug loading efficiency. Fortunately, some attempts have been made that may provide positive references. For instance, calcium phosphate (CaP) shells were employed to cover the surface of OMVs to attenuate toxicity (Qing et al., 2020). OMVs fused with cancer cell membranes to form hybrid OMVs have good homing and immune activation abilities (Wang et al., 2020). Therefore, we are confident that the deepening understanding of OMVs and the continuous progress of scientific research technology will contribute to the clinical transformation of OMVs as versatile drug delivery carriers in photodynamic anticancer therapy.
YJ: Writing–original draft, Writing–review and editing. ZZ: Writing–original draft. CiL: Writing–original draft. LW: Writing–original draft. CnL: Writing–review and editing.
The author(s) declare financial support was received for the research, authorship, and/or publication of this article. This work was supported by the grants from Research project of the Science and Technology Department of Sichuan province (2021YJ0217).
The authors express their sincere thanks to Prof. Hong Zhang for his helpful assistance.
The authors declare that the research was conducted in the absence of any commercial or financial relationships that could be construed as a potential conflict of interest.
All claims expressed in this article are solely those of the authors and do not necessarily represent those of their affiliated organizations, or those of the publisher, the editors and the reviewers. Any product that may be evaluated in this article, or claim that may be made by its manufacturer, is not guaranteed or endorsed by the publisher.
Bitto, N. J., Zavan, L., Johnston, E. L., Stinear, T. P., Hill, A. F., and Kaparakis-Liaskos, M. (2021). Considerations for the analysis of bacterial membrane vesicles: methods of vesicle production and quantification can influence biological and experimental outcomes. Microbiol. Spectr. 9, e0127321. doi:10.1128/Spectrum.01273-21
Chen, H., Zhou, M., Zeng, Y., Lv, Z., Wang, P., and Han, L. (2022). Recent advances in biomedical applications of bacterial outer membrane vesicles. J. Mater Chem. B 10, 7384–7396. doi:10.1039/d2tb00683a
Chen, M. H., Liu, T. Y., Chen, Y. C., and Chen, M. H. (2021). Combining augmented radiotherapy and immunotherapy through a nano-gold and bacterial outer-membrane vesicle complex for the treatment of glioblastoma. Nanomater. (Basel) 11, 1661. doi:10.3390/nano11071661
Cheng, K., Zhao, R., Li, Y., Qi, Y., Wang, Y., Zhang, Y., et al. (2021). Bioengineered bacteria-derived outer membrane vesicles as a versatile antigen display platform for tumor vaccination via Plug-and-Display technology. Nat. Commun. 12, 2041. doi:10.1038/s41467-021-22308-8
Collins, S. M., Nice, J. B., Chang, E. H., and Brown, A. C. (2021). Size exclusion chromatography to analyze bacterial outer membrane vesicle heterogeneity. J. Vis. Exp. 169, 62429. doi:10.3791/62429
Cui, C., Qian, H., Wang, J., Kang, J., Ma, W., Nian, Y., et al. (2023). Targeted miR-34a delivery with PD1 displayed bacterial outer membrane vesicles-coated zeolitic imidazolate framework nanoparticles for enhanced tumor therapy. Int. J. Biol. Macromol. 247, 125692. doi:10.1016/j.ijbiomac.2023.125692
Delgado, L., Baeza, N., Pérez-Cruz, C., López-Iglesias, C., and Mercadé, E. (2019). Cryo-transmission electron microscopy of outer-inner membrane vesicles naturally secreted by gram-negative pathogenic bacteria. Bio Protoc. 9, e3367. doi:10.21769/BioProtoc.3367
Ding, Y., Wang, L., Li, H., Miao, F., Zhang, Z., Hu, C., et al. (2022). Application of lipid nanovesicle drug delivery system in cancer immunotherapy. J. Nanobiotechnology 20, 214. doi:10.1186/s12951-022-01429-2
Donohoe, C., Senge, M. O., Arnaut, L. G., and Gomes-da-Silva, L. C. (2019). Cell death in photodynamic therapy: from oxidative stress to anti-tumor immunity. Biochim. Biophys. Acta Rev. Cancer 1872, 188308. doi:10.1016/j.bbcan.2019.07.003
Du, Y., Han, J., Jin, F., and Du, Y. (2022). Recent strategies to address hypoxic tumor environments in photodynamic therapy. Pharmaceutics 14, 1763. doi:10.3390/pharmaceutics14091763
Elsharkasy, O. M., Nordin, J. Z., Hagey, D. W., de Jong, O. G., Schiffelers, R. M., Andaloussi, S. E., et al. (2020). Extracellular vesicles as drug delivery systems: why and how? Adv. Drug Deliv. Rev. 159, 332–343. doi:10.1016/j.addr.2020.04.004
Fritzen, D. L., Giordano, L., Rodrigues, L. C. V., and Monteiro, J. H. S. K. (2020). Opportunities for persistent luminescent nanoparticles in luminescence imaging of biological systems and photodynamic therapy. Nanomater. (Basel) 10, 2015. doi:10.3390/nano10102015
Gao, J., Chen, Z., Li, X., Yang, M., Lv, J., Li, H., et al. (2022a). Chemiluminescence in combination with organic photosensitizers: beyond the light penetration depth limit of photodynamic therapy. Int. J. Mol. Sci. 23, 12556. doi:10.3390/ijms232012556
Gao, J., Feng, Q., Wang, J., and Zhao, X. (2022b). Bacterial outer membrane vesicle-based cancer nanovaccines. Cancer Biol. Med. 19, 1290–1300. doi:10.20892/j.issn.2095-3941.2022.0452
Gujrati, V., Kim, S., Kim, S. H., Min, J. J., Choy, H. E., Kim, S. C., et al. (2014). Bioengineered bacterial outer membrane vesicles as cell-specific drug-delivery vehicles for cancer therapy. ACS Nano 8, 1525–1537. doi:10.1021/nn405724x
Hamblin, M. R., and Abrahamse, H. (2021). Factors affecting photodynamic therapy and anti-tumor immune response. Anticancer Agents Med. Chem. 21, 123–136. doi:10.2174/1871520620666200318101037
Herrmann, I. K., Wood, M. J. A., and Fuhrmann, G. (2021). Extracellular vesicles as a next-generation drug delivery platform. Nat. Nanotechnol. 16, 748–759. doi:10.1038/s41565-021-00931-2
Hosseini-Giv, N., Basas, A., Hicks, C., El-Omar, E., El-Assaad, F., and Hosseini-Beheshti, E. (2022). Bacterial extracellular vesicles and their novel therapeutic applications in health and cancer. Front. Cell Infect. Microbiol. 12, 962216. doi:10.3389/fcimb.2022.962216
Huang, W., Shu, C., Hua, L., Zhao, Y., Xie, H., Qi, J., et al. (2020). Modified bacterial outer membrane vesicles induce autoantibodies for tumor therapy. Acta Biomater. 108, 300–312. doi:10.1016/j.actbio.2020.03.030
Jahromi, L. P., and Fuhrmann, G. (2021). Bacterial extracellular vesicles: understanding biology promotes applications as nanopharmaceuticals. Adv. Drug Deliv. Rev. 173, 125–140. doi:10.1016/j.addr.2021.03.012
Ji, B., Wei, M., and Yang, B. (2022). Recent advances in nanomedicines for photodynamic therapy (PDT)-driven cancer immunotherapy. Theranostics 12, 434–458. doi:10.7150/thno.67300
Jiang, Y., Wang, F., Wang, K., Zhong, Y., Wei, X., Wang, Q., et al. (2022). Engineered exosomes: a promising drug delivery strategy for brain diseases. Curr. Med. Chem. 29, 3111–3124. doi:10.2174/0929867328666210902142015
Kim, H. S., and Lee, D. Y. (2018). Near-infrared-responsive cancer photothermal and photodynamic therapy using gold nanoparticles. Polym. (Basel) 10, 961. doi:10.3390/polym10090961
Kim, O. Y., Park, H. T., Dinh, N. T. H., Choi, S. J., Lee, J., Kim, J. H., et al. (2017). Bacterial outer membrane vesicles suppress tumor by interferon-γ-mediated antitumor response. Nat. Commun. 8, 626. doi:10.1038/s41467-017-00729-8
Kuerban, K., Gao, X., Zhang, H., Liu, J., Dong, M., Wu, L., et al. (2020). Doxorubicin-loaded bacterial outer-membrane vesicles exert enhanced anti-tumor efficacy in non-small-cell lung cancer. Acta Pharm. Sin. B 10, 1534–1548. doi:10.1016/j.apsb.2020.02.002
Kulp, A., and Kuehn, M. J. (2010). Biological functions and biogenesis of secreted bacterial outer membrane vesicles. Annu. Rev. Microbiol. 64, 163–184. doi:10.1146/annurev.micro.091208.073413
Kusuzaki, K., Matsubara, T., Murata, H., Logozzi, M., Iessi, E., Di Raimo, R., et al. (2017). Natural extracellular nanovesicles and photodynamic molecules: is there a future for drug delivery? J. Enzyme Inhib. Med. Chem. 32, 908–916. doi:10.1080/14756366.2017.1335310
Kwiatkowski, S., Knap, B., Przystupski, D., Saczko, J., Kędzierska, E., Knap-Czop, K., et al. (2018). Photodynamic therapy - mechanisms, photosensitizers and combinations. Biomed. Pharmacother. 106, 1098–1107. doi:10.1016/j.biopha.2018.07.049
Li, M., Zhou, H., Yang, C., Wu, Y., Zhou, X., Liu, H., et al. (2020). Bacterial outer membrane vesicles as a platform for biomedical applications: an update. J. Control Release 323, 253–268. doi:10.1016/j.jconrel.2020.04.031
Li, X., Chen, L., Huang, M., Zeng, S., Zheng, J., Peng, S., et al. (2023). Innovative strategies for photodynamic therapy against hypoxic tumor. Asian J. Pharm. Sci. 18, 100775. doi:10.1016/j.ajps.2023.100775
Li, Y., Wu, J., Qiu, X., Dong, S., He, J., Liu, J., et al. (2022). Bacterial outer membrane vesicles-based therapeutic platform eradicates triple-negative breast tumor by combinational photodynamic/chemo-/immunotherapy. Bioact. Mater 20, 548–560. doi:10.1016/j.bioactmat.2022.05.037
Lieberman, L. A. (2022). Outer membrane vesicles: a bacterial-derived vaccination system. Front. Microbiol. 13, 1029146. doi:10.3389/fmicb.2022.1029146
Liu, S., Wu, X., Chandra, S., Lyon, C., Ning, B., Jiang, L., et al. (2022). Extracellular vesicles: emerging tools as therapeutic agent carriers. Acta Pharm. Sin. B 12, 3822–3842. doi:10.1016/j.apsb.2022.05.002
Lo, P. C., Ng, D. K. P., Pandey, R. K., and Zimcik, P. (2023). Photodynamic therapy: an innovative and versatile treatment modality triggered by light. Chempluschem 88, e202300159. doi:10.1002/cplu.202300159
Long, Q., Zheng, P., Zheng, X., Li, W., Hua, L., Yang, Z., et al. (2022). Engineered bacterial membrane vesicles are promising carriers for vaccine design and tumor immunotherapy. Adv. Drug Deliv. Rev. 186, 114321. doi:10.1016/j.addr.2022.114321
Mandal, P. K., Ballerin, G., Nolan, L. M., Petty, N. K., and Whitchurch, C. B. (2021). Bacteriophage infection of Escherichia coli leads to the formation of membrane vesicles via both explosive cell lysis and membrane blebbing. Microbiol. Read. 167, 001021. doi:10.1099/mic.0.001021
Matías, J., Pastor, Y., Irache, J. M., and Gamazo, C. (2020). Protective passive immunity in Escherichia coli ETEC-challenged neonatal mice conferred by orally immunized dams with nanoparticles containing homologous outer membrane vesicles. Vaccines (Basel). 8, 286. doi:10.3390/vaccines8020286
Meng, W., He, C., Hao, Y., Wang, L., Li, L., and Zhu, G. (2020). Prospects and challenges of extracellular vesicle-based drug delivery system: considering cell source. Drug Deliv. 27, 585–598. doi:10.1080/10717544.2020.1748758
Michel, L. V., and Gaborski, T. (2022). Outer membrane vesicles as molecular biomarkers for Gram-negative sepsis: taking advantage of nature's perfect packages. J. Biol. Chem. 298, 102483. doi:10.1016/j.jbc.2022.102483
Naskar, A., Cho, H., Lee, S., and Kim, K. S. (2021). Biomimetic nanoparticles coated with bacterial outer membrane vesicles as a new-generation platform for biomedical applications. Pharmaceutics 13, 1887. doi:10.3390/pharmaceutics13111887
Overchuk, M., Weersink, R. A., Wilson, B. C., and Zheng, G. (2023). Photodynamic and photothermal therapies: synergy opportunities for nanomedicine. ACS Nano 17, 7979–8003. doi:10.1021/acsnano.3c00891
Ozkocak, D. C., Phan, T. K., and Poon, I. K. H. (2022). Translating extracellular vesicle packaging into therapeutic applications. Front. Immunol. 13, 946422. doi:10.3389/fimmu.2022.946422
Park, J., Lee, Y. K., Park, I. K., and Hwang, S. R. (2021). Current limitations and recent progress in nanomedicine for clinically available photodynamic therapy. Biomedicines 9, 85. doi:10.3390/biomedicines9010085
Peng, L. H., Wang, M. Z., Chu, Y., Zhang, L., Niu, J., Shao, H. T., et al. (2020). Engineering bacterial outer membrane vesicles as transdermal nanoplatforms for photo-TRAIL-programmed therapy against melanoma. Sci. Adv. 6, eaba2735. doi:10.1126/sciadv.aba2735
Pérez-Cruz, C., Delgado, L., López-Iglesias, C., and Mercade, E. (2015). Outer-inner membrane vesicles naturally secreted by gram-negative pathogenic bacteria. PLoS One 10, e0116896. doi:10.1371/journal.pone.0116896
Prieto-Montero, R., Arbeloa, T., and Martínez-Martínez, V. (2023). Photosensitizer- mesoporous silica nanoparticles combination for enhanced photodynamic therapy. Photochem Photobiol. 99, 882–900. doi:10.1111/php.13802
Qing, S., Lyu, C., Zhu, L., Pan, C., Wang, S., Li, F., et al. (2020). Biomineralized bacterial outer membrane vesicles potentiate safe and efficient tumor microenvironment reprogramming for anticancer therapy. Adv. Mater 32, e2002085. doi:10.1002/adma.202002085
Qiu, H., Tan, M., Ohulchanskyy, T. Y., Lovell, J. F., and Chen, G. (2018). Recent progress in upconversion photodynamic therapy. Nanomater. (Basel). 8, 344. doi:10.3390/nano8050344
Rajkumar, S., and Prabaharan, M. (2017). Theranostics based on iron oxide and gold nanoparticles for imaging- guided photothermal and photodynamic therapy of cancer. Curr. Top. Med. Chem. 17, 1858–1871. doi:10.2174/1568026617666161122120537
Roier, S., Zingl, F. G., Cakar, F., Durakovic, S., Kohl, P., Eichmann, T. O., et al. (2016). A novel mechanism for the biogenesis of outer membrane vesicles in Gram-negative bacteria. Nat. Commun. 7, 10515. doi:10.1038/ncomms10515
Sartorio, M. G., Pardue, E. J., Feldman, M. F., and Haurat, M. F. (2021). Bacterial outer membrane vesicles: from discovery to applications. Annu. Rev. Microbiol. 75, 609–630. doi:10.1146/annurev-micro-052821-031444
Schwechheimer, C., and Kuehn, M. J. (2015). Outer-membrane vesicles from Gram-negative bacteria: biogenesis and functions. Nat. Rev. Microbiol. 13, 605–619. doi:10.1038/nrmicro3525
Shi, J., Ma, Z., Pan, H., Liu, Y., Chu, Y., Wang, J., et al. (2020). Biofilm-encapsulated nano drug delivery system for the treatment of colon cancer. J. Microencapsul. 37, 481–491. doi:10.1080/02652048.2020.1797914
Suri, K., D'Souza, A., Huang, D., Bhavsar, A., and Amiji, M. (2022). Bacterial extracellular vesicle applications in cancer immunotherapy. Bioact. Mater 22, 551–566. doi:10.1016/j.bioactmat.2022.10.024
Tian, C. M., Yang, M. F., Xu, H. M., Zhu, M. Z., Zhang, Y., Yao, J., et al. (2023). Emerging role of bacterial outer membrane vesicle in gastrointestinal tract. Gut Pathog. 15, 20. doi:10.1186/s13099-023-00543-2
Tong, L., Zhang, S., Huang, R., Yi, H., and Wang, J. W. (2022). Extracellular vesicles as a novel photosensitive drug delivery system for enhanced photodynamic therapy. Front. Bioeng. Biotechnol. 10, 1032318. doi:10.3389/fbioe.2022.1032318
Toyofuku, M., Nomura, N., and Eberl, L. (2019). Types and origins of bacterial membrane vesicles. Nat. Rev. Microbiol. 17, 13–24. doi:10.1038/s41579-018-0112-2
Toyofuku, M., Schild, S., Kaparakis-Liaskos, M., and Eberl, L. (2023). Composition and functions of bacterial membrane vesicles. Nat. Rev. Microbiol. 21, 415–430. doi:10.1038/s41579-023-00875-5
Turner, L., Bitto, N. J., Steer, D. L., Lo, C., D'Costa, K., Ramm, G., et al. (2018). Helicobacter pylori outer membrane vesicle size determines their mechanisms of host cell entry and protein content. Front. Immunol. 9, 1466. doi:10.3389/fimmu.2018.01466
van de Waterbeemd, B., Streefland, M., van der Ley, P., Zomer, B., van Dijken, H., Martens, D., et al. (2010). Improved OMV vaccine against Neisseria meningitidis using genetically engineered strains and a detergent-free purification process. Vaccine 28, 4810–4816. doi:10.1016/j.vaccine.2010.04.082
Wang, D., Liu, C., You, S., Zhang, K., Li, M., Cao, Y., et al. (2020). Bacterial vesicle-cancer cell hybrid membrane-coated nanoparticles for tumor specific immune activation and photothermal therapy. ACS Appl. Mater Interfaces 12, 41138–41147. doi:10.1021/acsami.0c13169
Wang, S., Gao, J., and Wang, Z. (2019). Outer membrane vesicles for vaccination and targeted drug delivery. Wiley Interdiscip. Rev. Nanomed Nanobiotechnol 11, e1523. doi:10.1002/wnan.1523
Wang, S. Y., Chen, G., Chen, J. F., Wang, J., Deng, S. H., and Cheng, D. (2022b). Glutathione-depleting polymer delivering chlorin e6 for enhancing photodynamic therapy. RSC Adv. 12, 21609–21620. doi:10.1039/d2ra01877b
Wang, S. Y., Guo, J., Bai, Y., Sun, C., Wu, Y., Liu, Z., et al. (2022a). Bacterial outer membrane vesicles as a candidate tumor vaccine platform. Front. Immunol. 13, 987419. doi:10.3389/fimmu.2022.987419
Xiong, Y., Xiao, C., Li, Z., and Yang, X. (2021). Engineering nanomedicine for glutathione depletion-augmented cancer therapy. Chem. Soc. Rev. 50, 6013–6041. doi:10.1039/d0cs00718h
Younus, L. A., Mahmoud, Z. H., Hamza, A. A., Alaziz, K. M. A., Ali, M. L., Yasin, Y., et al. (2023). Photodynamic therapy in cancer treatment: properties and applications in nanoparticles. Braz J. Biol. 84, e268892. doi:10.1590/1519-6984.268892
Zhang, J., Li, Z., Liu, L., Li, L., Zhang, L., Wang, Y., et al. (2022). Self-assembly catalase nanocomplex conveyed by bacterial vesicles for oxygenated photodynamic therapy and tumor immunotherapy. Int. J. Nanomedicine 17, 1971–1985. doi:10.2147/IJN.S353330
Zheng, Y., Li, Z., Chen, H., and Gao, Y. (2020). Nanoparticle-based drug delivery systems for controllable photodynamic cancer therapy. Eur. J. Pharm. Sci. 144, 105213. doi:10.1016/j.ejps.2020.105213
Zhuang, W. R., Wang, Y., Lei, Y., Zuo, L., Jiang, A., Wu, G., et al. (2022). Phytochemical engineered bacterial outer membrane vesicles for photodynamic effects promoted immunotherapy. Nano Lett. 22, 4491–4500. doi:10.1021/acs.nanolett.2c01280
Keywords: photodynamic therapy, photosensitizers, drug delivery carrier, nanoparticles, outer membrane vesicles
Citation: Jiang Y, Zhou Z, Liu C, Wang L and Li C (2023) Bacterial outer membrane vesicles as drug delivery carrier for photodynamic anticancer therapy. Front. Chem. 11:1284292. doi: 10.3389/fchem.2023.1284292
Received: 28 August 2023; Accepted: 09 October 2023;
Published: 17 October 2023.
Edited by:
Emre Güzel, Sakarya University of Applied Sciences, TürkiyeReviewed by:
Tomasz Koczorowski, Poznan University of Medical Sciences, PolandCopyright © 2023 Jiang, Zhou, Liu, Wang and Li. This is an open-access article distributed under the terms of the Creative Commons Attribution License (CC BY). The use, distribution or reproduction in other forums is permitted, provided the original author(s) and the copyright owner(s) are credited and that the original publication in this journal is cited, in accordance with accepted academic practice. No use, distribution or reproduction is permitted which does not comply with these terms.
*Correspondence: Chun Li, bXlzenh5eWtmeXhrbGNAeWVhaC5uZXQ=
Disclaimer: All claims expressed in this article are solely those of the authors and do not necessarily represent those of their affiliated organizations, or those of the publisher, the editors and the reviewers. Any product that may be evaluated in this article or claim that may be made by its manufacturer is not guaranteed or endorsed by the publisher.
Research integrity at Frontiers
Learn more about the work of our research integrity team to safeguard the quality of each article we publish.