- 1Department of Pharmacy, The Third People’s Hospital of Chengdu, Chengdu, China
- 2Sichuan Engineering Research Center for Biomimetic Synthesis of Natural Drugs, School of Life Science and Engineering, Southwest Jiaotong University, Chengdu, China
- 3Sichuan Research Center for Drug Precision Industrial Technology, West China School of Pharmacy, Sichuan University, Chengdu, China
A series of novel N-aryl-debenzeyldonepezil derivatives (1–26) were designed and synthesized as cholinesterase inhibitors by the modification of anti-Alzheimer’s disease drug donepezil, using Palladium catalyzed Buchwald-Hartwig cross-coupling reaction as a key chemical synthesis strategy. In vitro cholinesterase inhibition studies demonstrated that the majority of synthesized compounds exhibited high selective inhibition of AChE. Among them, analogue 13 possessing a quinoline functional group showed the most potent AChE inhibition effect and significant neuroprotective effect against H2O2-induced injury in SH-SY5Y cells. Furthermore, Compound 13 did not show significant cytotoxicity on SH-SY5Y. These results suggest that 13 is a potential multifunctional active molecule for treating Alzheimer’s disease.
1 Introduction
Alzheimer’s disease (AD) is a common age-related neurodegenerative brain disorder characterized by progressive cognitive dysfunction, memory loss, and restrictions in daily activities (Ross and Poirier, 2004). According to the World Health Organization (WHO), approximately 50 million people suffer from AD disease around the world, and the number of patients will reach more than 150 million in 2050 as the population ages (Du et al., 2022). As one of the neurodegenerative disorders, the exact pathogenesis of AD is not fully known. Many studies have revealed that AD might involve abnormality of multiple neurochemical factors, such as low levels of acetylcholine (ACh), the amplitude of unusual deposits of β-amyloid (Aβ) peptide, hyperphosphorylated tau protein, neuronal apoptosis, and so on (Li et al., 2020; Perry et al., 2002; Lee et al., 2018; Mozaffarnia et al., 2020). Many hypotheses have been proposed based on these pathogenic factors to treat AD. The typical cholinergic hypothesis suggests that the selective loss of cholinergic neurons in the brain results in cognitive impairment in AD, whereas improvement of the central cholinergic transmission is an effective treatment strategy (Du et al., 2018). Among the several approaches attempted to enhance ACh levels in the brain, acetylcholinesterase inhibitors (AChEIs) have been proven in patients with mild or moderate AD (Anand and Singh, 2013). Up to now, donepezil, galantamine, rivastigmine, and tacrine as AChEIs have been approved by FDA used in AD treatment (Figure 1) (Pardo-Moreno et al., 2022). Although tacrine regrettably had to be withdrawn from the market in 2005 due to its hepatotoxicity (Summers et al., 1989), all four approved AChEIs possess a basic nitrogen atom. Notably, the basic nitrogen is protonated at physiological pH to provide a temporary positive charge and interacts with the active site of AChE, generating an impact on the overall affinity of AChE for the ligands (Peauger et al., 2017). Therefore, the incorporation of a nitrogen atom in bioactive compounds to recreate their unique properties has been regarded as an effective AChEIs medicinal development route.
Donepezil, approved by the FDA in 1996, is a second-generation specific reversible AChEI for the treatment of mild and moderate AD, showing good patient tolerance and mild adverse effects (Seltzer, 2007). The crystal structure of donepezil-rhAChE complex showed benzyl moiety (Unit A) in donepezil as an important site forms π-π interactions with residues of the active site (Akhoon et al., 2020). Furthermore, the piperidine ring (Unit B) and indanone (Unit C) reach out to π-π stacking interactions with peripheral anionic and catalytic active sites of AChE (Cheung et al., 2012). These findings contribute to the cognition of potential pharmacophores interacting with the residues of the active site. With increased research enthusiasm in terms of functional AChEI molecule design, using donepezil’s key structural moieties to develop novel AChEIs has drawn immense attention. For instance, fluorine-containing donepezil analogues fluoropezil possessing a longer drug-target residence time has been developed, which is preparing to enter phase II trial (Zhou et al., 2021; Qian et al., 2023). In our previous study, various nitrogen-containing functional groups were introduced into Unit C to provide a series of α-aryl donepezil analogues owning high AChE inhibition (Wan et al., 2021a). In order to find better anti-AD compounds, considering that the aromatic ring in Unit A is a vital binding site, we envisaged using aromatic nitrogen heterocycles in place of the phenyl group might form the additional bonding force to strengthen the AChE inhibitory activity. Furthermore, the Palladium-catalyzed Buchwald-Hartwig cross-coupling reaction is one of the powerful approaches for constructing abundant structures libraries via the formation of C-N bonds and provides an important chemical ligation strategy for the present study (Dorel et al., 2019).
Therefore, as part of our ongoing research program toward exploiting novel anti-AD bioactive molecules (Wan et al., 2021a; Wan et al., 2021b; Wan et al., 2021c; Miao et al., 2021; Xu et al., 2023; Zhang et al., 2023), the present work pay attention to the AChE inhibitory activity affected by the benzyl moiety in donepezil, and proposed the structural modification of donepezil for efficiently introducing aromatic nitrogen heterocycles or aryl functional groups located at N position via Palladium-catalyzed Buchwald-Hartwig reaction, hoping to find the potential bioactive molecules. Herein, the synthesis and pharmacological evaluation of donepezil analogues are reported.
2 Results and discussion
2.1 Chemistry
To conduct the biological activity evaluation of a new class of N-aryl-debenzeyldonepezil derivates, a set of target compound 1–26 were prepared by employing debenzeyldonepezil as a key intermediate and introducing an aryl functional group attached to the nitrogens of piperidine ring via Buchwald-Hartwig C-N cross-coupling. At the beginning of this study, donepezil was reacted with 10% Pd/C in methanol under a hydrogen atmosphere at room temperature for 4 h resulting in the generation of debenzeyldonepezil in 90% yield (Scheme 1). Next, debenzeyldonepezil and 2-bromopyridine were chosen as model substrates to explore the optimum Buchwald-Hartwig reaction conditions for the preparation of aiming N-aryl-debenzeyldonepezil. According to previous reports (Heravi et al., 2018; Dorel et al., 2019), after examination of ligands, bases, and solvents, we found that the ligand played a vital role in this C-N cross-coupling process. As shown in Table 1, the monophosphine ligands PPh3 and PCy3 afforded little product 1 (entries 1 and 2). The yield of the derived product was dramatically improved when using Mephos as a ligand. Therefore, the N-arylated product 1 could be recieved in as high as 89% yield in the presence of Pd(OAc)2 (10 mol%), Mephos (20 mol%), and t-BuOK (3 equiv.) in 1,4-dioxane at 90°C for 17 h (entry 3). Then, the influence of different bases and solvents on the reaction process was investigated. When other bases such as t-BuONa, K2CO3, and KF were used instead of t-BuOK, the desired cross-coupling product 1 was obtained in unsatisfactory yields (entries 4–6). Similarly, replacing 1,4-dioxane with DME and THF provided a lower yield of 37% and 23%, respectively (entries 7 and 8).
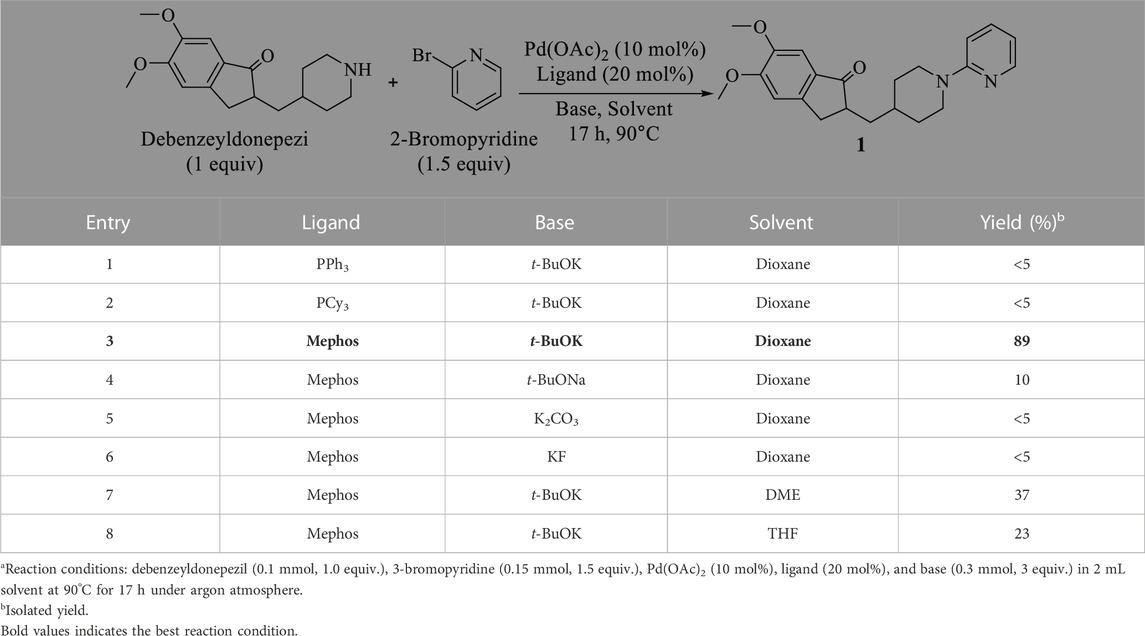
TABLE 1. Optimization of the N-arylation reaction conditionsa.
Subsequently, Palladium-catalyzed Buchwald-Hartwig reactions between debenzeyldonepezil and various aryl bromides were conducted under standard reaction conditions. As exemplified in Scheme 2, the investigated N-heteroaryl bromides smoothly provided the corresponding N-arylated debenzeyldonepezil products 1–13 in excellent yields (65%–89%). Both electron-donating groups (methyl and methoxy) and halogen fluorine at meta-, para- or ortho-position of 2-bromopyridine were well tolerated. The bromide substrates bearing a multisubstituted ring were tested and afforded the expected products 3 and 5 in 77% and 69% yields, respectively. Meanwhile, quinoline groups were suitable as well in this process to furnish corresponding products in yields of 72% (11), 75% (12), and 68% (13). In addition to pyridine and quinoline bromides, the substrates carrying phenyl rings were examined and provided the conjugate products in 50–92% yield (14–26). The unsubstituted benzene-bromide furnished the derivative 14 in 89% yield. Similarly, the phenyl bromides bearing electron-donating groups (methyl, methoxy, and tertiary butyl) (15–18) worked efficiently. The substrates carrying electron-withdrawing groups (fluorine and trifluoromethyl) (19–23) were in lower yields in comparison to that of 15–18. Compounds 24 and 25 equipped biphenyl groups were successfully prepared in yields of 79% and 69%, respectively. Furthermore, the reaction of debenzeyldonepezil with 1-bromo-4-methoxynaphthalene gave compound 26 an 80% yield. Structures of these N-aryl-debenzeyldonepezil analogues were characterized by NMR and HRESIMS spectra.
2.2 Biological activities
2.2.1 In vitro cholinesterase inhibition studies
The inhibitory activity of N-aryl-debenzeyldonepezil analogues (1–26) against AChE (from electric eel) was initially evaluated by means of Ellman’s spectrophotometric method (Ellman et al., 1961), employing donepezil as the positive control. The screening test was conducted at the concentrations of 100 and 50 μM, and the ability of these molecules to inhibit the enzymes is reported in Table 2. When introducing methylpyridine-provided compounds 2–5, the AChE inhibition activity of them was obviously lower than that of pyridine-substituted analogue 1. For the position of the methoxyl substituent group, compounds 8 with 4-methoxy pyridine and 9 with 3-methoxypyridine obtained maintenance in activity, while the activity of 6 (6-methoxypyridine) and 7 (5-methoxypyridine) decreased. The only compound 10 (EeAChE inhibitory rate = 44.1 ± 3.0% at 50 μM) in the series bearing a strong electron-withdrawing group also resulted in a larger decrease of AChE inhibitory activity than analogue 1. The above results implied that the steric effects of these substituent groups possibly combining with electronic effects led to an adverse impact on the inhibition potency for this set of analogues. In short, the N-aryl-debenzeyldonepezils 1–10 equipping pyridine ring showed slightly decreased AChE inhibitory capacity, compared to that of donepezil, which revealed that the introduction of pyridine is at a significant disadvantage.
On the other hand, for the analogues possessing the quinoline fragments, compounds 11–13 exhibited favorable inhibitory activities, implying that the presence of this basic atomic group could increase interactions with the active sites of the enzymes. Compound 13 showed the best inhibitory potency, which needs an in-depth investigation. Many studies of AChE crystal structures have confirmed that the active site gorge is located in the deep and narrow “canyon” (Cheung et al., 2012; Liu et al., 2022), which implied that the larger group 2-methylquinoline might contribute to occupying the active site and obtaining compound 13 with high activity against AChE. Moreover, most compounds of the phenyl series (14–23) showed moderate inhibition effect against AChE. Introducing electron-withdrawing groups into the benzene ring, as shown in compounds 19–23, improved activity more than that of analogues with electron-donating groups (15–18), probably due to electronic effects. However, two diphenyl analogues (24 and 25) among this subset of compounds seem to be noneffective, showing an EeAChE inhibitory rate of only about 50% at 50 µM. Then, the EqBuChE inhibition effects of these N-aryl-debenzeyldonepezil derivatives were also explored. As expected, all of them showed low inhibitory activity against eqBuChE.
2.2.2 Molecular modelling studies
To explore the binding mechanisms of compound 13 on AChE (PDB ID code: 1acj), a docking study to reveal the sites of binding and relative binging energy was performed. The highest-scored pose of 13 with a binding energy of −6.9 kcal/mol is depicted in Figure 2. Molecular docking results indicated that analogue 13 was suitably filled in the active site of AChE. Two π-alkyl hydrophobic interactions were formed by the quinoline fragment with Tyr 70 and Tyr 121, respectively. The benzene ring from quinoline established a π-anion bond with Asp 72. Moreover, attempt 334 was in a favorable position of stacking with the piperidine ring and quinoline’s benzene ring, forming a π–π and a π–alkyl interactions. Comparatively, in the active cavity of BuChE (PDB ID code: 6ASM), only the benzene ring from indanone interacted with Val 348 by π–alkyl interaction, and the carbonyl showed a conventional hydrogen bond with residues Arg 132. The highest-scored pose of 13 presented a binding energy of −5.6 kcal/mol (Figure 3). The above results could explain the compound 13 possessed the selective AChE inhibitory activity, which verified the in vitro enzyme inhibitory assay.
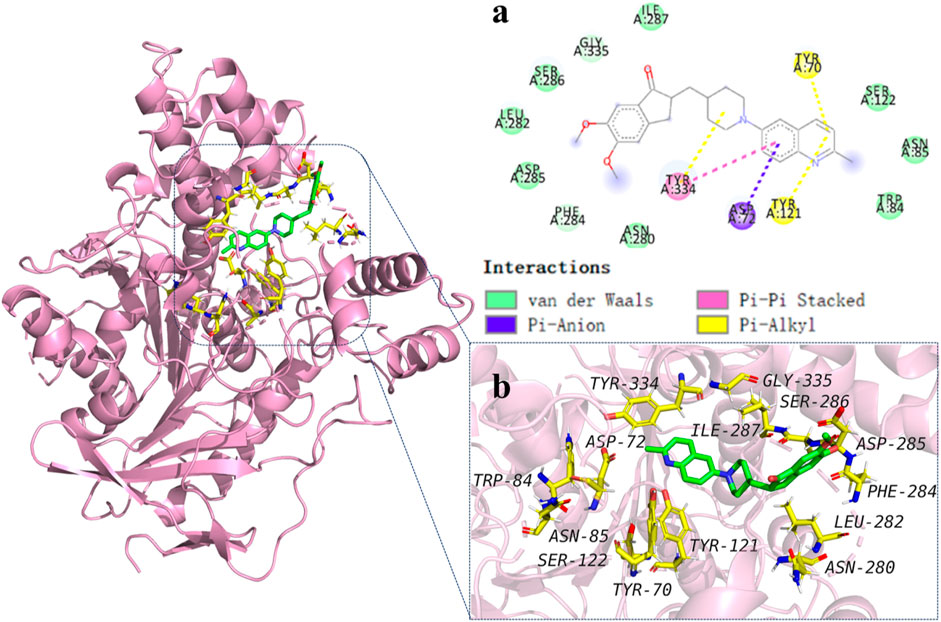
FIGURE 2. Molecular modeling of 13 in the active cavity of AChE (PDB ID: 1acj). (A) Residues of the active site involved in docking, and types of bonds involved in docking: π–π bond is shown as pick dashed lines, π–Alkyl bonds are shown as yellow dashed lines, and π–Anion bonds are shown as purple dashed lines; (B) Best docking positioning of compound 13 with AChE.
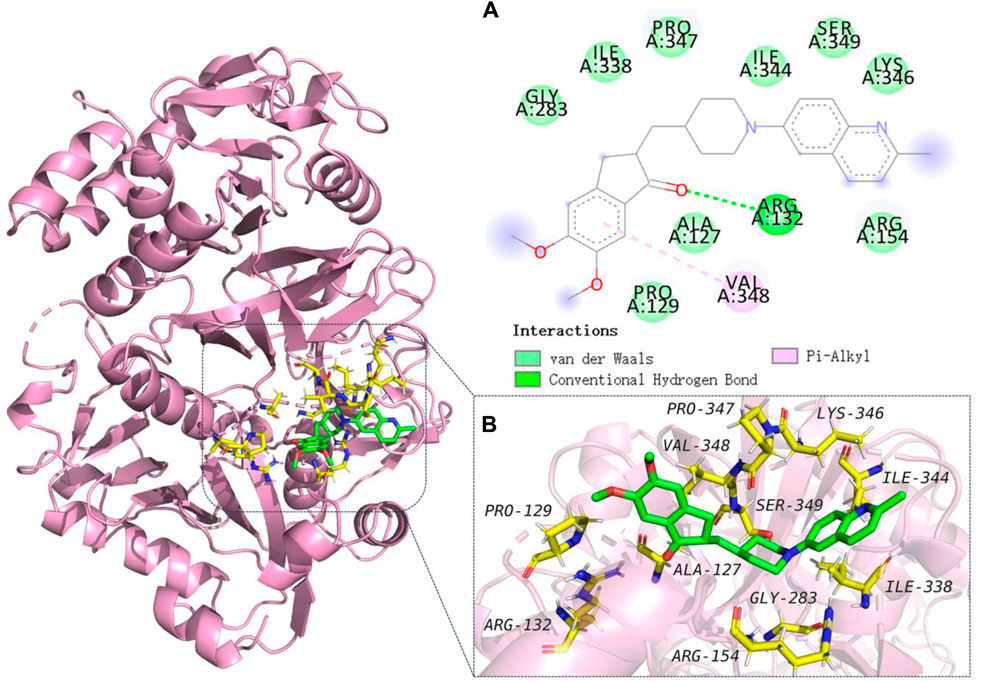
FIGURE 3. Molecular modeling of 13 in the active cavity of BuChE (PDB ID: 6ASM). (A) Residues of the active site involved in docking, and types of bonds involved in docking: π–π bond is shown as a pink dashed line and conventional hydrogen bonds are shown as green dashed lines; (B) Best docking positioning of compound 13 with BuChE.
2.2.3 Neuroprotection effects against H2O2-induced injury in SH-SY5Y cells
The death of neuronal cells is the central abnormality occurring in brains suffering from AD (Niikura et al., 2002). Neuroprotection is a useful way to protect the neuronal cells from damage, which potentially slows down or prevents the progression of AD (Coleman et al., 2004). To explore the therapeutic potential of synthesized analogues 1–26, the neuroprotective effects of 1–26 against H2O2-induced injury in SH-SY5Y cells were performed. Firstly, the neurotoxicity of compounds 1–26 on SH-SY5Y cell viability was determined using the MTT assay method. As shown in Table 3, N-aryl-debenzeyldonepezils 6–8, 17–22, and 26 did not affect the cell viability of SH-SY5Y cells even at concentrations up to 50 μM, indicating that they were not neurotoxic. Then, non-cytotoxic compounds (6–8, 17–22, and 26) were examined for their potential neuroprotective activity on damaged SH-SY5Y cellular models induced by 700 μM H2O2. In the model group, 700 μM H2O2 significantly reduced cell viability and decreased to 61% compared to the control group. The protective effect of analogues 6–8, 17–22, and 26 against H2O2 are shown in Table 4. The survival rate of damaged SH-SY5Y cells increased to 115% after the following treatment of compound 13, suggesting that synthetic compound 13 can protect cells against H2O2-induced cell death.
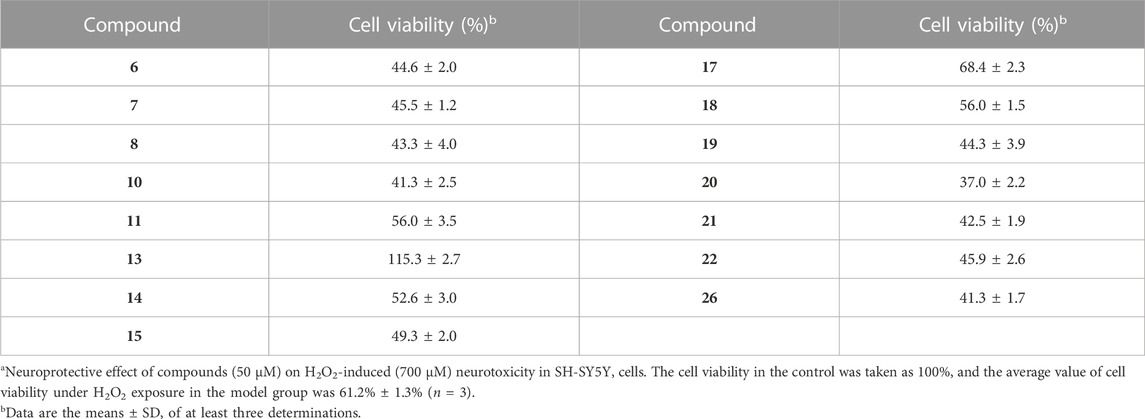
TABLE 4. Neuroprotective effect of non-cytotoxic compounds against H2O2-induced injury in SH-SY5Y cellsa.
2.2.4 BBB prediction
Penetrating the blood-brain barrier (BBB) is extremely significant for anti-AD medicines, so the BBB penetration of compounds 1–26 was predicted by using Calculate Molecular Properties (Discovery Studio 2020 Client). The predicted data are listed in Table 5. The level “0” shows that the Brain-Blood ratio is greater than 5:1, the value “1” expresses that the Brain-Blood ratio is between 1:1 and 5:1, and the value “2” expresses that the Brain-Blood ratio is between 0.3:1 and 1:1. Besides compound 11, most of the synthesized compounds are BBB permeable.
3 Conclusion
In summary, a series of new N-aryl-debenzeyldonepezil analogues (1–26) were efficiently synthesized in modification of donepezil by using Palladium-catalyzed Suzuki-Miyaura cross-coupling reaction as a key synthetic method. The cholinesterase inhibition and neuroprotective effects of these analogues were evaluated to develop potential candidate molecules for the treatment of AD. Among them, compound 13 exhibited good selective inhibitory activities against AChE, which might be attributed to the introduction of nitrogen-containing aromatic heterocycle. Molecular modeling was performed to explain the possible interaction between compound 13 and AChE. The prediction of bioavailability disclosed that analogue 13 had a favorable capacity to pass through the blood-brain barrier (BBB). Furthermore, further study showed that active compound 13 displayed non-neurotoxicity and good neuroprotective potency against H2O2-induced injury in SH-SY5Y cells. These results showed that compound 13 could be developed as an efficient multifunctional potential active molecule for the treatment of AD.
4 Experimental section
4.1 Chemistry
4.1.1 Materials and methods
All reactions were conducted under an inert atmosphere of dry argon. Anhydrous THF was purchased from Aladdin and used without further purification. Unless otherwise noted, the reagents and solvents used in this article were all commercially available analytical or chemical grades and used directly without any purification. Reactions were monitored by thin layer chromatography (TLC) on silica gel plates (GF 254) using UV light to visualize the course of the reactions. Silica gel H (Qingdao Sea Chemical Factory, Qingdao, PR China) was used for column chromatography. NMR spectra were recorded by using a Bruker AV 400 or 600 nuclear magnetic resonance instrument. Chemical shifts (δ) were recorded in ppm relative to TMS as the internal standard. HRESIMS spectra were recorded on a Waters Acquity UPLC/Q–TOF micro mass spectrometer.
4.1.2 Synthesis of debenzeyldonepezil
Donepezil (2.635 mmol) was suspended in 40 mL of a MeOH solution. To the reaction was added 10% Pd/C (0.2635 mmol), stirred at 25°C under an argon atmosphere for 12 h. Then, the mixture was filtered and evaporated under a vacuum. The reaction crude product was purified by flash column chromatography using PE-EA (3:1 to 1:1) to obtain debenzeyldonepezil as amorphous powder (90% yield); 1H NMR (400 MHz, CDCl3) δ 7.16 (s, 1H), 6.85 (s, 1H), 3.95 (s, 3H), 3.89 (s, 3H), 3.23 (dd, J = 17.4, 8.0 Hz, 1H), 3.09 (t, J = 9.6 Hz, 2H), 2.74−2.67 (m, 2H), 2.63−2.57 (m, 2H), 1.92−1.86 (m, 1H), 1.77 (d, J = 13.2 Hz, 1H), 1.69 (d, J = 13.2 Hz, 1H), 1.69 (d, J = 13.2 Hz, 1H), 1.34−1.24 (m, 2H), 1.22−1.11 (m, 2H); 13C NMR (100 MHz, CDCl3) δ 208.0, 155.6, 149.6, 148.9, 129.4, 107.5, 104.5, 56.3, 56.2, 46.9, 46.8, 45.3, 39.5, 35.0, 34.4, 33.4, 33.1; HRESIMS m/z 290.1740 [M + H]+ (calcd for C17H24NO3, 290.1756).
4.1.3 Synthesis of N-aryl-debenzeyldonepezil analogues 1–26
The suspension of Pd(OAc)2 (5 mol%) and Mephos (10 mol%) in anhydrous 1,4-dioxane (3 mL) was stirred at 30°C under an argon atmosphere for 2 h to obtain a brown solution. The brown solution was added to a 10 mL sealed dry reaction vial containing debenzeyldonepezil (1 mmol), naphthalenyl, bromides (1.5 mmol), and t-BuOK (3 mmol) via a syringe. The reaction mixture was stirred for 17 h at 90°C before quenching with two drops of H2O. Then, the mixture was diluted with ethyl acetate (3 mL), and filtered over a pad of MgSO4. The pad was rinsed with ethyl acetate, and the mixture was concentrated in vacuo. The reaction crude product was purified by flash column chromatography using PE-EA (2:1–1:2) to obtain the N-aryl-debenzeyldonepezil analogues 1–26 (50%–89% yields).
Compound 1, amorphous powder, 89% yield; 1H NMR (400 MHz, CDCl3) δ 8.18−8.16 (m, 1H), 7.44−7.42 (m, 1H), 7.17 (s, 1H), 6.86 (s, 1H), 6.66 (d, J = 8.4 Hz, 1H), 6.57−6.55 (m, 1H), 4.33 – 4.28 (m, 2H), 3.96 (s, 3H), 3.90 (s, 3H), 3.31 (q, J = 8.4 Hz, 1H), 2.87−2.79 (m, 2H), 2.77−2.70 (m, 2H), 1.97−1.90 (m, 1H), 1.87−1.78 (m, 2H), 1.40−1.25 (m, 4H); 13C NMR (100 MHz, CDCl3) δ 207.8, 159.8, 155.7, 149.7, 148.9, 148.2, 137.6, 129.5, 112.9, 107.6, 107.4, 104.6, 56.4, 56.3, 45.9, 45.9, 45.5, 39.0, 35.0, 33.6, 32.8, 31.6; HRESIMS m/z 367.2012 [M + H]+ (calcd for C22H27N2O3, 367.2022).
Compound 2, amorphous powder, 85% yield; 1H NMR (400 MHz, CDCl3) δ 8.15−8.14 (m, 1H), 7.39−7.37 (m, 1H), 7.18 (s, 1H), 6.87 (s, 1H), 6.82 (dd, J = 7.2, 4.8 Hz, 1H), 3.96 (s, 3H), 3.91 (s, 3H), 3.49−3.42 (m, 2H), 3.28 (q, J = 8.4 Hz, 1H), 2.83−2.73 (m, 4H), 2.26 (s, 3H), 2.02−1.96 (m, 1H), 1.91−1.77 (m, 2H), 1.53−1.36 (m, 4H); 13C NMR (100 MHz, CDCl3) δ 207.9, 162.6, 155.7, 149.6, 148.9, 145.3, 139.2, 129.5, 125.3, 117.7, 107.5, 104.6, 56.4, 56.2, 50.39, 50.38, 45.6, 39.0, 34.8, 33.7, 33.4, 32.3, 18.4; HRESIMS m/z 381.2162 [M + H]+ (calcd for C23H29N2O3, 381.2178).
Compound 3, amorphous powder, 77% yield; 1H NMR (400 MHz, CDCl3) δ 7.34 (t, J = 8.4 Hz, 1H), 7.18 (s, 1H), 6.87 (s, 1H), 6.45 (d, J = 2.7 Hz, 1H), 6.43 (s, 1H), 4.35−4.30 (m, 2H), 3.96 (s, 3H), 3.91 (s, 3H), 3.29 (q, J = 8.0 Hz, 1H), 2.83−2.79 (m, 1H), 2.78−2.71 (m, 3H), 2.39 (s, 3H), 1.97−1.90 (m, 1H), 1.86−1.77 (m, 2H), 1.40−1.30 (m, 4H); 13C NMR (100 MHz, CDCl3) δ 207.8, 159.5, 156.9, 155.7, 149.6, 148.9, 137.8, 129.4, 112.2, 107.5, 104.6, 104.0, 56.3, 56.2, 46.0, 45.9, 45.4, 38.9, 35.0, 33.5, 32.8, 31.6, 24.7; HRESIMS m/z 381.2165 [M + H]+ (calcd for C23H29N2O3, 381.2178).
Compound 4, amorphous powder, 82% yield; 1H NMR (400 MHz, CDCl3) δ 8.04 (d, J = 5.2 Hz, 1H), 7.18 (s, 1H), 6.87 (s, 1H), 6.48 (s, 1H), 6.43 (d, J = 5.2 Hz, 1H), 4.31−4.27 (m, 2H), 3.96 (s, 3H), 3.91 (s, 3H), 3.31 (q, J = 9.2 Hz, 1H), 2.85−2.79 (m, 2H), 2.78−2.70 (m, 2H), 2.25 (s, 3H), 1.97−1.90 (m, 1H), 1.87−1.77 (m, 2H), 1.40−1.29 (m, 4H); 13C NMR (100 MHz, CDCl3) δ 207.8, 160.0, 155.7, 149.6, 148.9, 148.4, 147.7, 129.4, 114.4, 107.8, 107.5, 104.6, 56.4, 56.3, 46.1, 46.0, 45.4, 39.0, 35.0, 33.5, 32.7, 31.6, 21.6; HRESIMS m/z 381.2163 [M + H]+ (calcd for C23H29N2O3, 381.2178).
Compound 5, amorphous powder, 69% yield; 1H NMR (400 MHz, CDCl3) δ 7.96 (d, J = 2.0 Hz, 1H), 7.22 (d, J = 2.0 Hz, 1H), 7.19 (s, 1H), 6.88 (s, 1H), 3.97 (s, 3H), 3.91 (s, 3H), 3.40−3.35 (m, 2H), 3.29 (q, J = 8.0 Hz, 1H), 2.79−2.73 (m, 4H), 2.24 (s, 3H), 2.21 (s, 3H), 2.02−1.96 (m, 1H), 1.90−1.76 (m, 2H), 1.53−1.37 (m, 4H); 13C NMR (100 MHz, CDCl3) δ 208.0, 160.7, 155.6, 149.6, 149.0, 145.2, 140.2, 129.5, 127.0, 125.0, 107.5, 104.6, 56.4, 56.3, 50.8, 50.7, 45.7, 39.0, 34.8, 33.7, 33.4, 32.3, 18.1, 17.6; HRESIMS m/z 395.2321 [M + H]+ (calcd for C24H31N2O3, 395.2335).
Compound 6, amorphous powder, 80% yield; 1H NMR (400 MHz, CDCl3) δ 7.37 (t, J = 8.0 Hz, 1H), 7.17 (s, 1H), 6.86 (s, 1H), 6.16 (d, J = 8.0 Hz, 1H), 6.02 (d, J = 8.0 Hz, 1H), 4.33−4.27 (m, 2H), 3.96 (s, 3H), 3.90 (s, 3H), 3.85 (s, 3H), 3.28 (q, J = 9.2 Hz, 1H), 2.83−2.71 (m, 4H), 1.96−1.89 (m, 1H), 1.85−1.76 (m, 2H), 1.40−1.24 (m, 4H); 13C NMR (100 MHz, CDCl3) δ 207.7, 163.2, 158.5, 155.6, 149.6, 148.8, 140.1, 129.4, 107.5, 104.5, 98.3, 97.4, 56.3, 56.2, 53.0, 45.8, 45.7, 45.4, 38.9, 34.9, 33.5, 32.6, 31.5; HRESIMS m/z 397.2116 [M + H]+ (calcd for C23H29N2O4, 397.2127).
Compound 7, amorphous powder, 77% yield; 1H NMR (400 MHz, CDCl3) δ 7.93 (d, J = 3.2 Hz, 1H), 7.18 (s, 1H), 7.13 (dd, J = 6.0, 2.8 Hz, 1H), 6.87 (s, 1H), 6.66 (d, J = 8.8 Hz, 1H), 4.16−4.10 (m, 2H), 3.96 (s, 3H), 3.91 (s, 3H), 3.79 (s, 3H), 3.31 (q, J = 9.2 Hz, 1H), 2.81−2.77 (m, 2H), 2.76−2.71 (m, 2H), 1.97−1.91 (m, 1H), 1.88−1.78 (m, 2H), 1.44−1.30 (m, 4H); 13C NMR (100 MHz, CDCl3) δ 207.9, 155.7, 155.6, 149.7, 149.0, 148.9, 133.8, 129.5, 125.2, 108.7, 107.6, 104.6, 56.5, 56.4, 56.3, 47.2, 47.1, 45.5, 39.0, 34.9, 33.6, 32.8, 31.6; HRESIMS m/z 397.2110 [M + H]+ (calcd for C23H29N2O4, 397.2127).
Compound 8, amorphous powder, 68% yield; 1H NMR (400 MHz, CDCl3) δ 8.03 (d, J = 5.8 Hz, 1H), 7.17 (s, 1H), 6.86 (s, 1H), 6.21 (dd, J = 5.8, 2.2 Hz, 1H), 6.12 (d, J = 2.2 Hz, 1H), 4.30−4.24 (m, 2H), 3.96 (s, 3H), 3.90 (s, 3H), 3.80 (s, 3H), 3.31 (q, J = 9.2 Hz, 1H), 2.86−2.78 (m, 2H), 2.75−2.71 (m, 2H), 1.96−1.90 (m, 1H), 1.86−1.77 (m, 2H), 1.40−1.29 (m, 4H); 13C NMR (100 MHz, CDCl3) δ 207.8, 167.4, 161.6, 155.7, 149.6, 149.2, 148.8, 129.4, 107.5, 104.6, 100.7, 92.1, 56.4, 56.2, 55.0, 46.1, 46.0, 45.4, 38.9, 35.0, 33.5, 32.7, 31.5; HRESIMS m/z 397.2117 [M + H]+ (calcd for C23H29N2O4, 397.2127).
Compound 9, amorphous powder, 70% yield; 1H NMR (400 MHz, CDCl3) δ 7.86 (dd, J = 3.6, 1.2 Hz, 1H), 7.18 (s, 1H), 7.01 (dd, J = 6.4, 1.6 Hz, 1H), 6.87 (s, 1H), 6.81 (dd, J = 8.0, 4.8 Hz, 1H), 3.96 (s, 3H), 3.94−3.92 (m, 2H), 3.90 (s, 3H), 3.84 (s, 3H), 3.28 (q, J = 9.2 Hz, 1H), 2.80−2.72 (m, 4H), 2.01−1.94 (m, 1H), 1.88−1.76 (m, 2H), 1.57−1.34 (m, 4H); 13C NMR (100 MHz, CDCl3) δ 207.9, 155.6, 153.0, 149.6, 148.9, 147.2, 138.9, 129.5, 117.5, 116.8, 107.5, 104.6, 56.3, 56.2, 55.4, 49.1, 49.0, 45.6, 39.0, 35.0, 33.5, 33.3, 32.0; HRESIMS m/z 397.2114 [M + H]+ (calcd for C23H29N2O4, 397.2127).
Compound 10, amorphous powder, 65% yield; 1H NMR (400 MHz, CDCl3) δ 7.97−7.94 (m, 1H), 7.24−7.10 (m, 2H), 6.85 (d, J = 2.0 Hz, 1H), 6.73−6.66 (m, 1H), 4.14−4.02 (m, 2H), 3.94 (s, 3H), 3.89 (s, 3H), 3.32−3.17 (m, 1H), 2.94−2.66 (m, 4H), 1.98−1.92 (m, 1H), 1.87−1.73 (m, 2H), 1.49−1.37 (m, 4H); 13C NMR (100 MHz, CDCl3) δ 208.0, 158.2, 149.6 (d, J = 30 Hz), 142.8 (d, J = 5 Hz), 142.6 (d, J = 60 Hz), 131.4, 129.4, 123.1 (d, J = 19 Hz), 115.8, 107.5, 104.5, 56.3, 56.2, 48.8, 48.7, 45.5, 39.1, 34.9, 33.5, 33.3, 31.9, 28.8; HRESIMS m/z 385.1911 [M + H]+ (calcd for C22H26FN2O3, 385.1927).
Compound 11, amorphous powder, 72% yield; 1H NMR (400 MHz, CDCl3) δ 7.86 (d, J = 10.0 Hz, 1H), 7.69 (d, J = 8.4 Hz, 1H), 7.58 (dd, J = 6.4, 1.2 Hz, 1H), 7.51−7.49 (m, 1H), 7.20−7.20 (m, 1H), 7.18 (s, 1H), 7.01 (d, J = 9.2 Hz, 1H), 6.87 (s, 1H), 4.59−4.56 (m, 2H), 3.97 (s, 3H), 3.91 (s, 3H), 3.32−3.24 (q, J = 8.4 Hz, 1H), 3.00−2.93 (m, 2H), 2.79−2.72 (m, 2H), 1.99−1.92 (m, 1H), 1.90−1.83 (m, 2H), 1.42−1.31 (m, 4H); 13C NMR (100 MHz, CDCl3) δ 207.7, 157.7, 155.7, 149.7, 148.8, 137.4, 129.5, 129.4, 127.3, 127.2, 126.7, 123.0, 122.2, 110.0, 107.5, 104.6, 56.4, 56.3, 45.8, 45.4, 45.3, 38.9, 35.1, 33.6, 32.9, 31.8; HRESIMS m/z 417.2166 [M + H]+ (calcd for C26H29N2O3, 417.2178).
Compound 12, amorphous powder, 75% yield; 1H NMR (400 MHz, CDCl3) δ 8.88 (dd, J = 4.2, 1.8 Hz, 1H), 8.50 (d, J = 7.6 Hz, 1H), 7.79 (d, J = 8.4 Hz, 1H), 7.61 (t, J = 7.6 Hz, 1H), 7.38 (dd, J = 8.6, 4.0 Hz, 1H), 7.20 (s, 1H), 7.12 (d, J = 6.4 Hz, 1H), 6.88 (s, 1H), 3.97 (s, 3H), 3.92 (s, 3H), 3.41−3.35 (m, 2H), 3.34−3.27 (m, 1H), 2.83−2.75 (m, 4H), 2.08−2.03 (m, 1H), 1.97−1.87 (m, 2H), 1.71−1.62 (m, 4H); 13C NMR (100 MHz, CDCl3) δ 207.8, 155.7, 150.9, 150.2, 149.7, 149.6, 148.9, 132.6, 129.5, 129.4, 124.4, 120.3, 115.2, 115.1, 107.5, 104.6, 56.4, 56.3, 54.2, 45.6, 39.0, 34.7, 33.8, 33.5, 32.6, 29.8; HRESIMS m/z 417.2161 [M + H]+ (calcd for C26H29N2O3, 417.2178).
Compound 13, amorphous powder, 68% yield; 1H NMR (400 MHz, CDCl3) δ 7.89 (s, 1H), 7.87 (s, 1H), 7.48 (dd, J = 6.8, 2.4 Hz, 1H), 7.19−7.17 (m, 2H), 7.02 (d, J = 2.8 Hz, 1H), 6.87 (s, 1H), 3.96 (s, 3H), 3.91 (s, 3H), 3.84−3.78 (m, 2H), 3.32 (q, J = 7.6 Hz, 1H), 2.84−2.72 (m, 4H), 2.68 (s, 3H), 2.00−1.97 (m, 1H), 1.94−1.90 (m, 2H), 1.57−1.37 (m, 4H); 13C NMR (100 MHz, CDCl3) δ 207.8, 155.9, 155.7, 149.7, 149.4, 148.9, 143.3, 135.1, 129.4, 129.1, 127.7, 123.3, 122.3, 109.6, 107.5, 104.6, 56.4, 56.2, 50.3, 50.2, 45.4, 38.8, 34.5, 33.5, 32.9, 31.8, 25.0; HRESIMS m/z 431.2322 [M + H]+ (calcd for C27H31N2O3, 431.2335).
Compound 14, amorphous powder, 89% yield; 1H NMR (400 MHz, CDCl3) δ 7.35−7.31 (m, 3H), 7.04 (d, J = 8.0 Hz, 2H), 6.95 (s, 1H), 6.90 (t, J = 7.6 Hz, 1H), 4.04 (s, 3H), 3.99 (s, 3H), 3.81−3.74 (m, 1H), 3.39−3.32 (m, 1H), 2.85−2.81 (m, 2H), 2.79−2.75 (m, 2H), 2.09−2.00 (m, 1H), 1.97−1.88 (m, 2H), 1.60−1.44 (m, 4H); 13C NMR (100 MHz, CDCl3) δ 207.8, 155.7, 152.0, 149.6, 148.8, 129.4, 129.2, 119.5, 116.7, 107.5, 104.6, 56.3, 56.2, 50.1, 50.0 45.4, 38.8, 34.5, 33.5, 33.0, 31.9; HRESIMS m/z 366.2050 [M + H]+ (calcd for C23H28NO3, 366.2069).
Compound 15, amorphous powder, 90% yield; 1H NMR (400 MHz, CDCl3) δ 7.18 (s, 1H), 7.06 (d, J = 8.4 Hz, 2H), 6.86 (s, 1H), 6.87 (d, J = 8.4 Hz, 2H), 3.97 (s, 3H), 3.91 (s, 3H), 3.29 (q, J = 8.0 Hz, 1H), 2.78−2.62 (m, 4H), 2.26 (s, 3H), 1.99−1.93 (m, 1H), 1.89−1.79 (m, 2H), 1.50−1.42 (m, 2H), 1.53−1.34 (m, 4H); 13C NMR (100 MHz, CDCl3) δ 207.9, 155.7, 150.0, 149.7, 148.9, 129.8, 129.5, 129.1, 117.2, 107.6, 104.6, 56.4, 56.3, 50.9, 50.8, 45.6, 38.9, 34.6, 33.6, 33.2, 32.0, 20.5; HRESIMS m/z 380.2210 [M + H]+ (calcd for C24H30NO3, 380.2226).
Compound 16, amorphous powder, 88% yield; 1H NMR (400 MHz, CDCl3) δ 7.18 (s, 1H), 7.14 (t, J = 7.6 Hz, 1H), 6.87 (s, 1H), 6.76 (d, J = 8.6 Hz, 2H), 6.66 (d, J = 7.6 Hz, 1H), 3.97 (s, 3H), 3.91 (s, 3H), 3.71−3.66 (m, 2H), 3.31 (q, J = 8.0 Hz, 1H), 2.78−2.67 (m, 4H), 2.31 (s, 3H), 1.99−1.94 (m, 1H), 1.89−1.79 (m, 2H), 1.52−1.34 (m, 4H); 13C NMR (100 MHz, CDCl3) δ 207.8, 155.7, 152.1, 149.6, 148.9, 138.9, 129.5, 129.1, 120.5, 117.7, 113.9, 107.6, 104.7, 56.4, 56.4, 56.3, 50.3, 45.5, 39.0, 34.6, 33.6, 33.2, 32.0, 22.0; HRESIMS m/z 380.2213 [M + H]+ (calcd for C24H30NO3, 380.2226).
Compound 17, amorphous powder, 92% yield; 1H NMR (400 MHz, CDCl3) δ 7.29−7.26 (m, 2H), 7.19 (s, 1H), 6.91−6.89 (m, 2H), 6.87 (s, 1H), 3.97 (s, 3H), 3.91 (s, 3H), 3.69−3.63 (m, 2H), 3.31 (q, J = 8.0 Hz, 1H), 2.79−2.73 (m, 2H), 2.72−2.64 (m, 2H), 1.99−1.93 (m, 1H), 1.89−1.79 (m, 2H), 1.52−1.41 (m, 4H), 1.29 (s, 9H); 13C NMR (100 MHz, CDCl3) δ 207.8, 155.7, 149.6, 148.9, 142.2, 129.5, 126.0, 125.9, 116.4, 107.5, 104.6, 56.4, 56.3, 50.4, 50.3, 45.5, 38.9, 34.5, 34.1, 33.5, 33.2, 32.0, 31.6; HRESIMS m/z 422.2681 [M + H]+ (calcd for C27H36NO3, 422.2695).
Compound 18, amorphous powder, 77% yield; 1H NMR (400 MHz, CDCl3) δ 7.18 (s, 1H), 6.94 (d, J = 9.2 Hz, 2H), 6.87 (s, 1H), 6.84 (d, J = 9.2 Hz, 2H), 3.97 (s, 3H), 3.91 (s, 3H), 3.77 (s, 3H), 3.55−3.50 (m, 2H), 3.26 (q, J = 8.0 Hz, 1H), 2.78−2.71 (m, 2H), 2.68−2.60 (m, 2H), 2.00−1.93 (m, 1H), 1.90−1.80 (m, 2H), 1.54−1.35 (m, 4H); 13C NMR (100 MHz, CDCl3) δ 207.9, 155.7, 153.9, 149.7, 148.9, 146.5, 129.5, 119.0, 114.6, 107.6, 104.7, 56.4, 56.3, 55.7, 51.8, 45.5, 38.9, 34.4, 33.5, 33.3, 32.1; HRESIMS m/z 396.2160 [M + H]+ (calcd for C24H30NO4, 396.2175).
Compound 19, amorphous powder, 75% yield; 1H NMR (400 MHz, CDCl3) δ 7.18 (s, 1H), 6.98−6.95 (m, 1H), 6.94−6.90 (m, 2H), 6.90−6.86 (m, 2H), 3.97 (s, 3H), 3.91 (s, 3H), 3.59−3.54 (m, 2H), 3.27 (q, J = 9.2 Hz, 1H), 2.80−2.72 (m, 2H), 2.70−2.63 (m, 2H), 2.02−1.87 (m, 2H), 1.88−1.74 (m, 2H), 1.60 (s, 1H), 1.50 (m, 2H); 13C NMR (100 MHz, CDCl3) δ 207.8, 155.8, 149.7, 148.9, 148.8 (d, J = 2.4 Hz), 129.5, 118.6 (d, J = 10 Hz), 115.7 (d, J = 20 Hz), 107.6, 104.7, 56.4, 56.3, 51.3, 45.5, 38.9, 34.4, 33.6, 33.2, 32.1; HRESIMS m/z 384.1961 [M + H]+ (calcd for C23H27FNO3, 384.1975).
Compound 20, amorphous powder, 68% yield; 1H NMR (400 MHz, CDCl3) δ 7.19 (d, J = 5.2 Hz, 1H), 7.15 (dd, J = 8.3, 7.1 Hz, 1H), 6.87 (s, 1H), 6.69 (dd, J = 6.0, 2.4 Hz, 1H), 6.61 (dt, J = 12.8, 2.4 Hz, 1H), 6.51−6.46 (m, 1H), 3.97 (s, 3H), 3.91 (s, 3H), 3.73−3.68 (m, 2H), 3.29 (q, J = 8.0 Hz, 1H), 2.78−2.71 (m, 4H), 1.98−1.91 (m, 1H), 1.89−1.79 (m, 2H), 1.60−1.36 (m, 4H); 13C NMR (100 MHz, CDCl3) δ 207.8, 164.1 (d, J =240 MHz), 155.8, 153.5 (d, J =10 MHz), 149.7, 148.9, 130.2 (d, J =10 MHz), 129.5, 111.7, 107.6, 105.5 (d, J =20 MHz), 104.7, 103.1 (d, J =20 MHz), 56.4, 56.3, 49.6, 49.6, 45.5, 38.9, 34.5, 33.6, 32.8, 31.7; HRESIMS m/z 384.1967 [M + H]+ (calcd for C23H27FNO3, 384.1975).
Compound 21, amorphous powder, 77% yield; 1H NMR (400 MHz, CDCl3) δ 7.47 (d, J = 8.4 Hz, 2H), 7.18 (s, 1H), 6.94 (d, J = 8.4 Hz, 2H), 6.87 (s, 1H), 3.97 (s, 3H), 3.91 (s, 3H), 3.84−3.79 (m, 2H), 3.30 (q, J = 8.0, 1H), 2.86−2.81 (m, 2H), 2.80−2.71 (m, 2H), 1.98−1.91 (m, 1H), 1.90−1.69 (m, 2H), 1.60−1.35 (m, 4H); 13C NMR (100 MHz, CDCl3) δ 207.7, 155.8, 153.7, 149.8, 148.9, 129.5, 126.6 (q, J = 4 Hz), 114.9, 111.5, 107.6, 104.7, 100.6, 56.5, 56.3, 48.9, 48.9, 45.4, 38.9, 34.5, 33.6, 32.6, 31.6; HRESIMS m/z 434.1930 [M + H]+ (calcd for C24H27F3NO3, 434.1943).
Compound 22, amorphous powder, 72% yield; 1H NMR (400 MHz, CDCl3) δ 7.32 (t, J = 8.0 Hz, 1H), 7.18 (s, 1H), 7.12 (t, J = 2.0 Hz, 1H), 7.08−7.01 (m, 2H), 6.87 (s, 1H), 3.96 (s, 3H), 3.91 (s, 3H), 3.76−3.70 (m, 2H), 3.27 (q, J = 7.6 Hz, 1H), 2.81−2.71 (m, 4H), 1.98−1.93 (m, 1H), 1.91−1.82 (m, 2H), 1.50−1.35 (m, 4H); 13C NMR (100 MHz, CDCl3) δ 207.7, 155.8, 152.0, 149.7, 148.9, 131.5 (q, J = 30 MHz), 129.7, 129.5, 124.6 (q, J = 280 MHz) 119.4, 115.5 (q, J = 10 MHz), 112.6 (q, J = 10 MHz), 107.6, 104.6, 56.4, 56.3, 49.7, 49.6, 45.4, 38.8, 34.4, 33.6, 32.8, 31.7; HRESIMS m/z 434.1935 [M + H]+ (calcd for C24H27F3NO3, 434.1943).
Compound 23, amorphous powder, 50% yield; 1H NMR (400 MHz, CDCl3) δ 7.24 (d, J = 3.5 Hz, 3H), 7.18 (s, 1H), 6.88 (s, 1H), 3.97 (s, 3H), 3.92 (s, 3H), 3.85−3.76 (m, 2H), 3.34−3.21 (m, 1H), 2.86 (m, 2H), 2.81−2.70 (m, 2H), 1.99−1.93 (m, 1H), 1.92−1.85 (m, 2H), 1.49−1.36 (m, 4H); 13C NMR (100 MHz, CDCl3) δ 207.6, 155.8, 152.1, 149.8, 148.8, 132.4 (q, J = 30 MHz), 129.5, 124.9, 121.8, 115.1, 110.6, 107.6, 104.7, 56.5, 56.3, 49.1, 49.0, 45.3, 38.8, 34.3, 33.6, 32.5, 31.5; HRESIMS m/z 502.1801 [M + H]+ (calcd for C25H26F6NO3, 502.1817).
Compound 24, amorphous powder, 79% yield; 1H NMR (400 MHz, CDCl3) δ 7.60−7.58 (m, 2H), 7.42 (t, J = 7.6 Hz, 2H), 7.35−7.30 (m, 2H), 7.19 (s, 1H), 7.16 (t, J = 2.0 Hz, 1H), 7.07 (d, J = 7.6 Hz, 1H), 6.95 (dd, J = 8.2, 2.4 Hz, 1H), 6.87 (s, 1H), 3.96 (s, 3H), 3.91 (s, 3H), 3.80−3.75 (m, 2H), 3.27 (q, J = 7.6 Hz, 1H), 2.80−2.73 (m, 4H), 2.08−2.04 (m, 1H), 1.95−1.85 (m, 2H), 1.60−1.42 (m, 4H); 13C NMR (100 MHz, CDCl3) δ 207.8, 155.7, 152.4, 149.7, 148.9, 142.4, 142.1, 129.6, 129.5, 128.8, 127.4, 127.3, 118.6, 115.8, 115.7, 107.6, 104.6, 56.4, 56.3, 50.3, 50.3, 45.5, 38.9, 34.6, 33.6, 33.1, 31.9; HRESIMS m/z 442.2370 [M + H]+ (calcd for C29H32NO3, 442.2382).
Compound 25, amorphous powder, 69% yield; 1H NMR (400 MHz, CDCl3) δ 7.55 (d, J = 7.2 Hz, 2H), 7.52 (d, J = 8.8 Hz, 2H), 7.40 (t, J = 8.8 Hz, 2H), 7.40 (t, J = 7.6 Hz, 2H), 7.29−7.27 (m, 1H), 7.19 (s, 1H), 7.02 (d, J = 8.8 Hz, 1H), 6.88 (s, 1H), 3.97 (s, 3H), 3.92 (s, 3H), 3.79−3.74 (m, 1H), 3.28 (q, J = 8.4 Hz, 1H), 2.81−2.72 (m, 4H), 2.01−1.94 (m, 1H), 1.92−1.82 (m, 2H), 1.52−1.37 (m, 4H); 13C NMR (100 MHz, CDCl3) δ 207.9, 155.8, 151.2, 149.7, 148.9, 141.2, 132.1, 129.5, 128.9, 127.9, 126.7, 126.5, 116.7, 107.6, 104.7, 56.4, 56.3, 50.0, 45.5, 38.9, 34.6, 33.6, 33.0, 31.9, 29.9; HRESIMS m/z 442.2391 [M + H]+ (calcd for C29H32NO3, 442.2382).
Compound 26, amorphous powder, 80% yield; 1H NMR (400 MHz, CDCl3) δ 8.24 (d, J = 8.0 Hz, 1H), 8.20 (d, J = 8.0 Hz, 1H), 7.53−7.45 (m, 2H), 7.21 (s, 1H), 7.02 (d, J = 8.0 Hz, 1H), 6.89 (s, 1H), 6.74 (d, J = 7.6 Hz, 1H), 3.97 (s, 6H), 3.92 (s, 3H), 3.34−3.28 (m, 2H), 2.82−2.78 (m, 2H), 2.09−2.04 (m, 1H), 1.95 – 1.85 (m, 2H), 1.68−1.60 (m, 6H), 1.50−1.42 (m, 1H); 13C NMR (100 MHz, CDCl3) δ 208.0, 155.7, 151.6, 149.7, 149.0, 144.5, 130.3, 129.6, 129.0, 126.7, 126.2, 125.4, 123.6, 122.5, 114.6, 107.6, 104.7, 103.6, 56.4, 56.3, 55.8, 54.0, 45.8, 33.6, 32.9, 29.9; HRESIMS m/z 446.2319 [M + H]+ (calcd for C28H32NO4, 446.2331).
4.2 Biological methods
4.2.1 AChE and BuChE inhibition
All reagents, AChE (from electric eel) and BuChE (from equine serum) were purchased from Sigma-Aldrich. Ellman’s method was used to measure the ChE inhibitory effects of synthesized N-aryl-debenzeyldonepezils (Ellman et al., 1961). The tested compounds were dissolved in DMSO and diluted with phosphate buffer to final concentrations. The enzyme solution was prepared by dissolving 2.5 mg (0.5 U/mL) AChE in 1 mL pH 8.0 phosphate buffer. In 96-well plates, 140 μL phosphate buffer (pH 6.7) and 10 μL AChE were incubated with 10 μL of various concentrations of test compounds at 25°C for 20 min. Then, 10 μL DTNB (0.75 mM) and 10 μL ATCI (1.5 mM) were added for incubation at 37°C for 20 min. The absorbance was monitored at 405 nm using a microplate reader (TECAN SPECTRA, Wetzlar, Germany). Each concentration was assayed in triplicate. BuChE assays were present using a similar method to that described above. Enzyme inhibitory activity (%) = [1 – (Asample/Acontrol)] × 100.
4.2.2 Cell culture and treatment
The neuroprotective activity was evaluated in human neuroblastoma SH-SY5Y cells which were treated with H2O2. Human SH-SY5Y neuroblastoma cells were obtained from ATCC (Manassas, VA, United States) and were cultured at 37°C in a humidified atmosphere of 5% CO2, in Gibcotm Dulbecco’s Modified Eagle Medium (DMEM) supplemented with 10% heat-inactivated fetal bovine serum. The viability of the cells was determined by MTT assay. MTT was purchased from Sigma-Aldrich (St. Louis, MO, United States). SH-SY5Y cells were seeded in 96-well plates at a density of 1 × 105 cells/well for 12 h. Cells were pretreated with 50 μM of each test compound. After 24 h of incubation, hydrogen peroxide solution (H2O2, 700 μM) was added and incubated for 4 h. Then, MTT (5 mg/mL) was added to each well. After a 4 h treatment, the supernatant was removed, and DMSO (150 μL/well) was added to dissolve the insoluble formazan crystals. Then, the plate was vibrated, and the absorbance was measured at 490 nm using a microplate reader.
4.2.3 Molecular docking analysis
The ligand-protein docking was guided by Discovery Studio 2020 Client and PyRx software to predict the binding poses of the ligand in the active site of AChE. The 3D models of AChE (PDB ID:1acj) and BuChE (PDB ID: 6ASM) were obtained from Protein Data Bank (http://www.rcsb.org/). The 3D models of the protein of AchE and BuChE were further optimized by Discovery Studio 2020 Client to minimize energy. The AutoDock Vina was employed for docking calculations using the default parameters. The blood-brain barrier (BBB) penetration was predicted by the Discovery Studio 2020 Client.
Data availability statement
The raw data supporting the conclusion of this article will be made available by the authors, without undue reservation.
Author contributions
J-JX: Formal Analysis, Funding acquisition, Writing–review and editing. JL: Methodology, Software, Writing–review and editing. HX: Formal Analysis, Writing–review and editing. J-BX: Methodology, Writing–original draft, Writing–review and editing. L-XW: Conceptualization, Funding acquisition, Supervision, Writing–original draft, Writing–review and editing.
Funding
The author(s) declare financial support was received for the research, authorship, and/or publication of this article. This research was financially supported by grants from the National Natural Science Foundation of China (82204244), the Natural Science Foundation of Sichuan Province (23NSFSC2370), and the Young Pharmacist Foundation of Sichuan Hospital Association (22031).
Conflict of interest
The authors declare that the research was conducted in the absence of any commercial or financial relationships that could be construed as a potential conflict of interest.
Publisher’s note
All claims expressed in this article are solely those of the authors and do not necessarily represent those of their affiliated organizations, or those of the publisher, the editors and the reviewers. Any product that may be evaluated in this article, or claim that may be made by its manufacturer, is not guaranteed or endorsed by the publisher.
Supplementary material
The Supplementary Material for this article can be found online at: https://www.frontiersin.org/articles/10.3389/fchem.2023.1282978/full#supplementary-material
References
Akhoon, B. A., Choudhary, S., Tiwari, H., Kumar, A., Barik, M. R., Rathor, L., et al. (2020). Discovery of a new donepezil-like acetylcholinesterase inhibitor for targeting Alzheimer's disease: computational studies with biological validation. J. Chem. Inf. Model. 60, 4717–4729. doi:10.1021/acs.jcim.0c00496
Anand, P., and Singh, B. (2013). A review on cholinesterase inhibitors for Alzheimer's disease. Arch. Pharm. Res. 36, 375–399. doi:10.1007/s12272-013-0036-3
Cheung, J., Rudolph, M. J., Burshteyn, F., Cassidy, M. S., Gary, E. N., Love, J., et al. (2012). Structures of human acetylcholinesterase in complex with pharmacologically important ligands. J. Med. Chem. 55, 10282–10286. doi:10.1021/jm300871x
Coleman, P., Federoff, H., and Kurlan, R. (2004). A focus on the synapse for neuroprotection in Alzheimer disease and other dementias. Neurology 63, 1155–1162. doi:10.1212/01.wnl.0000140626.48118.0a
Dorel, R., Grugel, C. P., and Haydl, A. M. (2019). The Buchwald-Hartwig amination after 25 years. Angew. Chem. Int. Ed. 58, 17118–17129. doi:10.1002/anie.201904795
Du, C. X., Wang, L., Guan, Q. W., Yang, H. Y., Chen, T. K., Liu, Y. J., et al. (2022). N-benzyl benzamide derivatives as selective sub-nanomolar butyrylcholinesterase inhibitors for possible treatment in advanced Alzheimer's disease. J. Med. Chem. 65, 11365–11387. doi:10.1021/acs.jmedchem.2c00944
Du, X. G., Wang, X. Y., and Geng, M. Y. (2018). Alzheimer's disease hypothesis and related therapies. Transl. Neurodegener. 7, 2. doi:10.1186/s40035-018-0107-y
Ellman, G. L., Courtney, K. D., Andres, V., and Featherstone, R. M. (1961). A new and rapid colorimetric determination of acetylcholinesterase activity. Biochem. Pharmacol. 7, 88–95. doi:10.1016/0006-2952(61)90145-9
Heravi, M. M., Kheilkordi, Z., Zadsirjan, V., Heydari, M., and Malmir, M. (2018). Buchwald- Hartwig reaction: an overview. J. Organomet. Chem. 861, 17–104. doi:10.1016/j.jorganchem.2018.02.023
Lee, S. Y., Chiu, Y. J., Yang, S. M., Chen, C. M., Huang, C. C., Lee-Chen, G. J., et al. (2018). Novel synthetic chalcone-coumarin hybrid for A beta aggregation reduction, antioxidation, and neuroprotection. CNS Neurosci. Ther. 24, 1286–1298. doi:10.1111/cns.13058
Li, Q., Xing, S. S., Chen, Y., Liao, Q. H., Xiong, B. C., He, S. Y., et al. (2020). Discovery and biological evaluation of a novel highly potent selective butyrylcholinsterase inhibitor. J. Med. Chem. 63, 10030–10044. doi:10.1021/acs.jmedchem.0c01129
Liu, J., Liu, L., Zheng, L., Feng, K. W., Wang, H. T., Xu, J. P., et al. (2022). Discovery of novel 2,3-dihydro-1H-inden-1-ones as dual PDE4/AChE inhibitors with more potency against neuroinflammation for the treatment of Alzheimer's disease. J. Med. Chem. 238, 114503. doi:10.1016/j.ejmech.2022.114503
Miao, S. X., Wan, L. X., He, Z. X., Zhou, X. L., Li, X. H., and Gao, F. (2021). Pd-catalyzed direct diversification of natural anti-Alzheimer’s disease drug: synthesis and biological evaluation of N-aryl Huperzine A analogueues. J. Nat. Prod. 84, 2374–2379. doi:10.1021/acs.jnatprod.1c00600
Mozaffarnia, S., Teimuri-Mofrad, R., and Rashidi, M. R. (2020). Design, synthesis and biological evaluation of 2,3-dihydro-5,6-dimethoxy-1H-inden-1-one and piperazinium salt hybrid derivatives as hAChE and hBuChE enzyme inhibitors. Eur. J. Med. Chem. 191, 112140. doi:10.1016/j.ejmech.2020.112140
Niikura, T., Hashimoto, Y., Tajima, H., and Nishimoto, I. (2002). Death and survival of neuronal cells exposed to Alzheimer's insults. J. Neurosci. Res. 70, 380–391. doi:10.1002/jnr.10354
Pardo-Moreno, T., Gonzalez-Acedo, A., Rivas-Dominguez, A., Garcia-Morales, V., Garcia-Cozar, F. J., Ramos-Rodriguez, J. J., et al. (2022). Therapeutic approach to Alzheimer's disease: current treatments and new perspectives. Pharmaceutics 14, 1117. doi:10.3390/pharmaceutics14061117
Peauger, L., Azzouz, R., Gembus, V., Tintas, M. L., Santos, J. S. D., Bohn, P., et al. (2017). Donepezil-based central acetylcholinesterase inhibitors by means of a "bio-oxidizable" prodrug strategy: design, synthesis, and in vitro biological evaluation. J. Med. Chem. 60, 5909–5926. doi:10.1021/acs.jmedchem.7b00702
Perry, G., Cash, A. D., and Smith, M. A. (2002). Alzheimer disease and oxidative stress. J. Biomed. 2, 120–123. doi:10.1155/S1110724302203010
Qian, H. J., Yu, C. Y., Zhu, H. J., Ding, Q. C., Cai, Y. T., Jing, J., et al. (2023). Safety, tolerability, and pharmacokinetics of fluoropezil (DC20), a novel acetylcholinesterase inhibitor: A phase I study in healthy young and elderly Chinese subjects. CTS-Clin. Transl. Sci. 16, 810–822. doi:10.1111/cts.13490
Ross, C. A., and Poirier, M. A. (2004). Protein aggregation and neurodegenerative disease. Nat. Med. 10, 10–17. doi:10.1038/nm1066
Seltzer, B. (2007). Donepezil: an update. Expert Opin. Pharmacother. 8, 1011–1023. doi:10.1517/14656566.8.7.1011
Summers, W. K., Koehler, A. L., Marsh, G. M., Tachiki, K., and Kling, A. (1989). Long-term hepatotoxicity of tacrine. Lancet 1, 729. doi:10.1016/s0140-6736(89)92246-0
Wan, L. X., Miao, S. X., He, Z. X., Li, X. H., Zhou, X. L., and Gao, F. (2021a). Pd-catalyzed direct modification of an anti-Alzheimer’s disease drug: synthesis and biological evaluation of α-aryl donepezil analogueues. ACS Omega 6, 23347–23354. doi:10.1021/acsomega.1c03103
Wan, L. X., Zhang, J. F., Zhen, Y. Q., Zhang, L., Li, X. H., Gao, F., et al. (2021b). Isolation, structure elucidation, semi-synthesis, and structural modification of C19-diterpenoid alkaloids from Aconitum apetalum and their neuroprotective activities. J. Nat. Prod. 84, 1067–1077. doi:10.1021/acs.jnatprod.0c01111
Wan, L. X., Zhen, Y. Q., He, Z. X., Zhang, Y., Zhang, L., Li, X. H., et al. (2021c). Late-stage modification of medicine: Pd-catalyzed direct synthesis and biological evaluation of N-aryltacrine derivatives modification of medicine: Pd-catalyzed direct synthesis and biological evaluation of N-aryltacrine derivatives. ACS Omega 6, 9960–9972. doi:10.1021/acsomega.1c01404
Xu, J. B., Miao, S. X., Gao, F., and Wan, L. X. (2023). Palladium-catalyzed synthesis and acetylcholinesterase inhibitory activity evaluation of 1-arylhuperzine A derivatives acetylcholinesterase inhibitory activity evaluation of 1-arylhuperzine A derivatives. J. Asian Nat. 25, 1097–1109. doi:10.1080/10286020.2023.2196619
Zhang, Y., Xu, J. B., Xiao, Y., Ji, W. S., Shan, L. H., Wan, L. X., et al. (2023). Palladium- Catalyzed synthesis, acetylcholinesterase inhibition and neuroprotective activities of N-aryl galantamine analogueues. J. Nat. Prod. 86, 939–946. doi:10.1021/acs.jnatprod.2c01150
Keywords: donepezil, Alzheimer’s disease, Buchwald-Hartwig reaction, AChE, neuroprotection
Citation: Xu J-J, Luo J, Xi H, Xu J-B and Wan L-X (2023) Palladium-catalyzed synthesis and anti-AD biological activity evaluation of N-aryl-debenzeyldonepezil analogues. Front. Chem. 11:1282978. doi: 10.3389/fchem.2023.1282978
Received: 25 August 2023; Accepted: 27 November 2023;
Published: 08 December 2023.
Edited by:
Gokhan Zengin, Selcuk University, TürkiyeReviewed by:
Prashant Murumkar, Maharaja Sayajirao University of Baroda, IndiaSyed H. Mehdi, University of Arkansas for Medical Sciences, United States
Copyright © 2023 Xu, Luo, Xi, Xu and Wan. This is an open-access article distributed under the terms of the Creative Commons Attribution License (CC BY). The use, distribution or reproduction in other forums is permitted, provided the original author(s) and the copyright owner(s) are credited and that the original publication in this journal is cited, in accordance with accepted academic practice. No use, distribution or reproduction is permitted which does not comply with these terms.
*Correspondence: Jing-Jing Xu, eHVqaW5namluZzAyMjRAMTYzLmNvbQ==; Lin-Xi Wan, d2FubHhAc2N1LmVkdS5jbg==