- Center for Supramolecular Chemistry and Catalysis and Department of Chemistry, College of Science, Shanghai University, Shanghai, China
Supramolecular containers have long been applied to regulate organic reactions with distinct selectivity, owing to their diverse functions such as the ability to pose a guest molecule(s) with a certain orientation and conformation. In this review, we try to illustrate how self-assembled coordination cages could achieve this goal. Two representative cage hosts, namely, self-assembled Pd(II)-ligand octahedral coordination cages ([Pd6L4]12+) and self-assembled Ga(III)-ligand tetrahedral coordination cages ([Ga4L6]12−) are selected as the pilot hosts that this mini review covers. Representative works in this area are presented here in brief.
1 Introduction
Ever since its establishment, supramolecular chemistry has received enormous attention and has rapidly become one of the most important fields in modern chemistry (Lehn, 1993; Lehn, 1995; Housecroft, 2021). Depending on various non-covalent interactions, supramolecular chemistry has been thoroughly studied and applied to various research areas, including molecular recognition, molecular devices, nanochemistry, and catalysis, etc. (Vriezema et al., 2005; Ariga and Kunitake, 2006; van Leeuwen, 2008; Kolesnichenko and Anslyn, 2017). Within the field of supramolecular chemistry, molecular container compounds are large hollow molecules with inner cavities that can accommodate various guest molecules. The inner cavity (inner phase) of a molecular container provides an elegantly isolated hydrophobic microenvironment, resembling active enzyme receptor sites, which can pose a guest molecule(s) with a certain fixed orientation and conformation. Logically, chemists have tried to simulate the function of natural enzymes by developing various synthetic supramolecular containers in recent decades to tackle problems in traditional organic chemistry, including improving reactivity and reaction rate, inducing new reaction selectivity, and even producing new reaction pathways (Purse and Rebek, 2005; Koblenz and WassenaarReek, 2008; Hooley and Rebek, 2009; Murase and Fujita, 2010; Yu and Rebek, 2018; Morimoto et al., 2020; Olivo et al., 2021). Selectivity is a crucial factor in conducting a certain organic reaction, but still remains one of the most significant challenges in organic synthetic chemistry (Ward, 1999). Poor reaction selectivity always results in complicated and even unachievable separation and purification procedures, which degrade the economy and efficiency of the synthetic methodology. It is rather difficult to regulate and control the reaction selectivity because the difference between transition state free energies regarding electronic, steric, and stereoelectronic influence of related reaction pathways, which give rise to isomeric products, is small (Chao et al., 1991; Balcells et al., 2016). Organic chemists have long made great efforts trying to regulate reaction selectivity (Neufeldt and Sanford, 2012; Davis and Phipps, 2017), and supramolecular chemistry and containers have come into their sights. Completely different from a bulk solution, the inner microenvironment of a supramolecular container can isolate and protect the guest substrates from the outside media, with certain fixed orientation and configuration achieved through various noncovalent interactions This certainly affects and controls the emergence of different or new selectivity as well as products that are not the major outcome or that even cannot be detected in regular conditions by altering the corresponding transition state free energies and reaction processes (Purse and Rebek, 2005; Koblenz and WassenaarReek, 2008; Hooley and Rebek, 2009; Murase and Fujita, 2010; Yu and Rebek, 2018; Morimoto et al., 2020; Olivo et al., 2021). Self-assembled coordination cage is a very important category of supramolecular containers, and, different from covalently constructed macrocycle hosts, they are readily self-assembled from ligands and metal ions through noncovalent coordination interactions (Murase and Fujita, 2010; García-Simón et al., 2014; García-Simón et al., 2015; Cullen et al., 2016; Howlader et al., 2016; Guo et al., 2017; Ueda et al., 2017; Hong et al., 2018; Martí-Centelles et al., 2018; Yu et al., 2018). The ionic property of self-assembled coordination cages provides them with two main advantages: (1) water solubility, which facilitates them in achieving molecular recognition and catalysis in water and (2) the ability to preferably accommodate and catalyze ionic guests and reactions. The Fujita group (Murase and Fujita, 2010) and the Toste, Raymond, and Bergman groups (García-Simón et al., 2014) have done a lot of pioneering and seminal works in this research area and have produced various fruitful achievements. In this mini review, we focus on summarizing representative examples of organic reactions inside self-assembled coordination cages that show distinct selectivity from that of the outside bulk solution conditions. Two representative types of self-assembled coordination cages were selected, namely, self-assembled Pd(II)-ligand octahedral coordination cages and self-assembled Ga(III)-ligand tetrahedral coordination cages, and this mini review is classified according to them.
2 Mini review
2.1 Self-assembled Pd(II)-ligand octahedral coordination cage ([Pd6L4]12+)
The self-assembled Pd(II)-ligand octahedral coordination cage was initially developed by the Fujita group (Fujita et al., 1995) and has been applied to various molecular recognition study and reaction catalysis research since then (Murase and Fujita, 2010). As shown in Figure 1A, it is a hollow octahedral framework self-assembled from six Pd(II) ions and four organic ligands, with a triazine core and three substituted 4-pyridyl groups. This cage host possesses 12 positive charges, which renders it very water soluble. The electron-deficient nature of the aromatic panel ligands endows it with a better ability to recognize electron-rich guest molecules and a significant role as a photosensitizer to facilitate guest-to-host photoinduced electron transfer (PET) (Yoshizawa et al., 2004; Furutani et al., 2009; Murase et al., 2011; Murase et al., 2012; Cullen et al., 2019). The Fujita group has reported plenty of organic reaction regulation works utilizing this distinguished supramolecular cage host (Murase and Fujita, 2010).
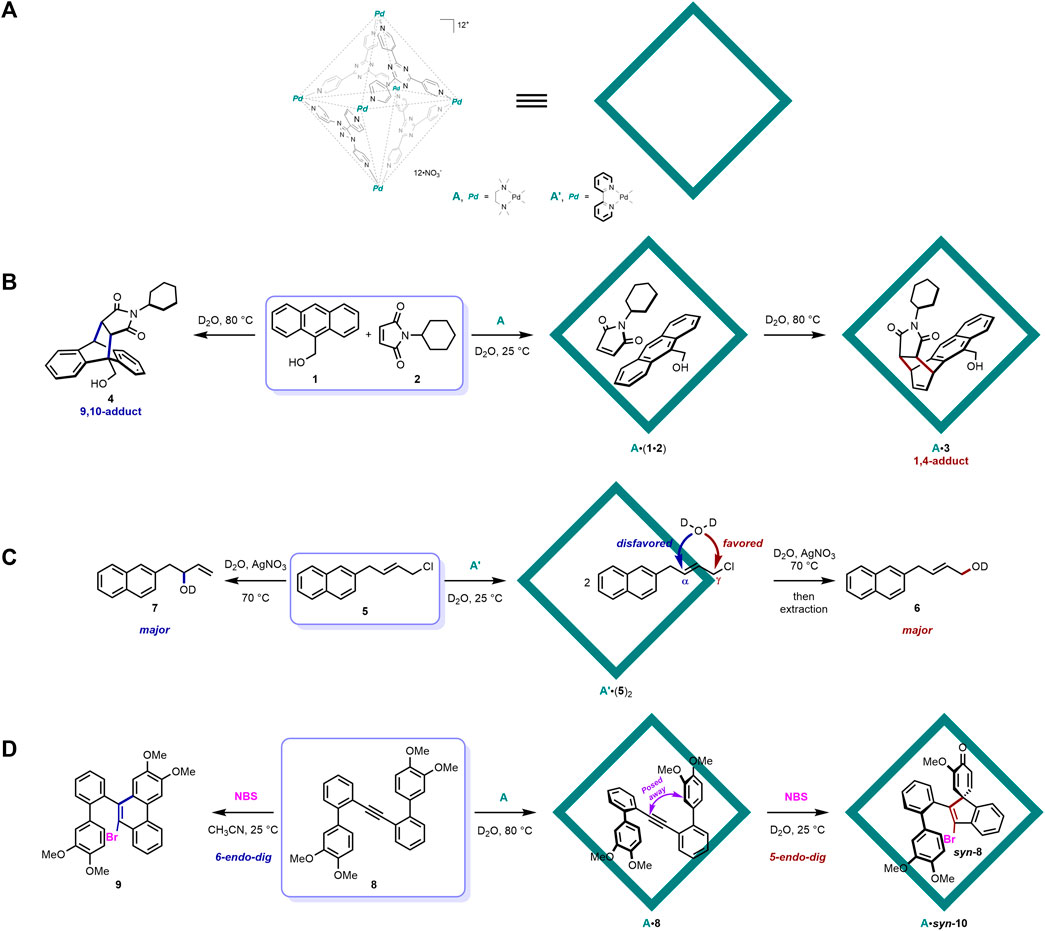
FIGURE 1. Examples of [Pd6L4]12+ cage mediated organic reactions with distinct selectivity: (A) structure of the representative self-assembled Pd(II)-ligand octahedral coordination cage; (B) cage host-mediated Diels-Alder reaction with distinct selectivity; (C) cage host-mediated nucleophilic substitution reaction of allylic chlorides with preferable terminal site selectivity; (D) cage host-mediated electrophilic spirocyclization of a 2-biphenylacetylene with distinct selectivity.
In 2006, Fujita and co-workers reported a seminal work on the Diels–Alder reaction between anthracene and phthalimide inside the [Pd6L4]12+ cage host with distinct site selectivity (Yoshizawa et al., 2006) (Figure 1B). Owing to its high localization of π-electron density at the center ring (Cheng and Li, 2003), the Diels–Alder cyclization reaction of anthracene generally occurs at this site and gives rise to a 9,10-adduct that bridges the center ring of the anthracene framework (Breslow, 1991; Fringuelli and Taticchi, 2002; Stuhlmann and Jäschke, 2002). However, when the corresponding reaction of 9-hydroxymethylanthrancen (1) and N-cyclohexylphthalimide (2) was moved into the cavity of the [Pd6L4]12+ cage host A in Fujita’s report, the site selectivity of this Diels–Alder reaction was altered to the 1,4-position of anthracene, producing a syn-isomer of 1,4-adduct 3. X-ray crystallographic analysis further confirmed the structure of the product together with its orientation and conformation inside the cage host, revealing that the naphthalene ring of the product interacts closely with one of the triazine ligand of A through π-π stacking. In the following control experiment without A, only the conventional 9,10-adduct 4 was detected without any 3. The fixed orientation of the substrates inside the cage host was responsible for this distinct site selectivity of the 1,4-position. In the force-field calculation study, 2 was shown to be parallel to 1, with its double bond in close contact with the 1,4- but not the 9,10-position of 1, owing to the steric effect inside the cage host. In another comparable example, when a less sterically demanding N-propylphthalimide substrate was applied, only the 9,10-adduct was formed, indicating the crucial role of the steric bulkiness of the N-substituent on the dienophile inside the cage host. This pioneering work perfectly validated the ability of supramolecular containers to accommodate guest substrates inside and to lend them certain fixed orientations and conformations, which give rise to a distinct reaction selectivity that is not seen in traditional bulk solution conditions. In following research, the Fujita group further provided a series of novel pericyclic reactions inside a similar cage host with distinct and controllable selectivity (Murase and Fujita, 2010).
In 2012, another nucleophilic substitution reaction of allylic chlorides inside a cage host with preferable terminal site selectivity was reported by Fujita and co-workers (Kohyama et al., 2012) (Figure 1C). Generally, the reaction of allylic chlorides with nucleophiles takes place at both the α- and γ-positions, and the steric and electronic effects of substrates together with the polarity of the solvent collectively influence the ratio of α- and γ-products (DeWolfe and Young, 1956). The cage host A′ also proved to be effective in recognizing the allylic chloride substrate (5) inside its hydrophobic cavity. In this mode, the α-position was buried inside the cavity, with the γ-position pointed out of the inner phase of A’. In this reaction, the solvent D2O acted as the nucleophile, and the hydrophobic pocket of A′ prevented its entering and protected the buried α-reactive position from contacting the incoming water nucleophile. On the other hand, the pointed-out terminal γ-position was exposed to the outside aqueous solution and was attacked by D2O, producing a terminal-induced product (6). In the control experiment without A’, the internal α-product (7) was major. Even though the ratio between terminal/internal products was not significant in this work, it represents an early example of the noncovalent protecting group function of supramolecular containers. Protecting groups are very famous in organic chemistry for their powerful function of preventing certain selected functional groups from reacting with other reagents (Schelhaas and Waldmann, 1996; Isidro-Llobet et al., 2009; Klán et al., 2013). Generally, they are covalently attached to the target moieties through pre-functionalization prior to the formal reaction and are deprotected after. However, just like the above example, supramolecular containers have offered a very promising alternative way of protecting and shielding certain reactive groups through the in situ recognition of the target moieties in the inner pocket via noncovalent interactions. The protecting manner of supramolecular containers has two main advantages: (1) the noncovalent mode of this concept requires no additional pre-functionalization procedures and it is weak enough to allow the substrate to easily dissociate from the protective host template, without further complicated deprotection procedures and (2) incompatible functional groups in traditional covalent protecting procedures can be tolerated in the noncovalent protecting system provided by supramolecular containers because no covalent bond formation is required. The [Pd6L4]12+ cage host was further applied to another reaction acting as this kind of noncovalent protecting groups to achieve site selective control (Takezawa et al., 2019).
Very recently, the same group have provided the electrophilic spirocyclization of a 2-biphenylacetylene in the presence of an electrophile through the fixing of conformation confined to the cavity of the cage host (Takezawa et al., 2022) (Figure 1D). When treated with electrophiles, 2-biphenylacetylene (8) underwent two different cyclization pathways: (1) one at the ortho position via 6-endo-dig cyclization to produce phenanthrene derivatives (9) (Goldfinger et al., 1997; Yamaguchi and Swager, 2001; Li et al., 2007; Dou et al., 2013) and (2) one at the ipso position via 5-endo-dig cyclization to produce benzospiro (Vriezema et al., 2005; Ariga and Kunitake, 2006)decane derivatives (10) (Appel et al., 2003; Zhang and Larock, 2005; Yu et al., 2008; Dohi et al., 2011a; Dohi et al., 2011b; Tang et al., 2012). In normal solution conditions, the former pathway and the corresponding phenanthrene products are often observed, and, only when the electron density at the ipso position is much higher than that at the ortho position, will the latter be major (Appel et al., 2003; Zhang and Larock, 2005; Yu et al., 2008; Dohi et al., 2011a; Dohi et al., 2011b; Tang et al., 2012). To achieve distinct selectivity of the latter, the authors naturally introduced the [Pd6L4]12+ cage host A into this reaction system. 8 was readily accommodated into the hollow pocket of A, and, according to 1H NMR, UV/Vis, and X-ray crystallographic analysis, the confined guest molecule was tightly packed with a folded conformation inside the host. The electron-rich aromatic rings of 8 interacted with the electron-deficient panel ligand of the host through π-π stacking. On the other hand, the ortho reactive positions were posed significantly away from the acetylene carbons, indicating the abovementioned 6-endo-dig cyclization may be suppressed. Indeed, treating the host-guest complex with electrophile NBS produced the spiro product 10 quantitatively, with single syn-diastereoselectivity. A control experiment revealed that 9 was formed through 6-endo-dig cyclization without the cage host. This recent example again showed how the [Pd6L4]12+ cage host could perfectly pose the guest molecule with a certain fixed orientation and conformation to induce distinct selectivity.
2.2 Self-assembled Ga(III)-ligand tetrahedral coordination cage ([Ga4L6]12−)
The self-assembled metal-ligand tetrahedral coordination cage (Figure 2A) was first reported by Raymond and co-workers (Caulder et al., 1998). It exhibits a tetrahedral shape and is spontaneously assembled from four metal ions, for example, Ga(III), Al(III), In(III), Fe(III), Ti(IV), Ge(IV), and six bis-bidentate catechol amide–containing ligands. Among them, the [Ga4L6]12− cage has been widely used for mediating organic reactions. Similar to the [Pd6L4]12+ cage host, the ionic property also generates good water solubility for the [Ga4L6]12− cage. On the other hand, the [Ga4L6]12− cage host is excellent at recognizing cationic guest molecules because of its negative charge nature. The electron-rich character also renders the [Ga4L6]12− cage host photosensitizer functionality and the ability to further induce host-to-guest photoinduced electron transfer (PET) (Dalton et al., 2015). Various seminal organic reaction regulation works involving this [Ga4L6]12− cage host have been provided by the collaboration between the Toste, Raymond, and Bergman groups (Hong et al., 2018).
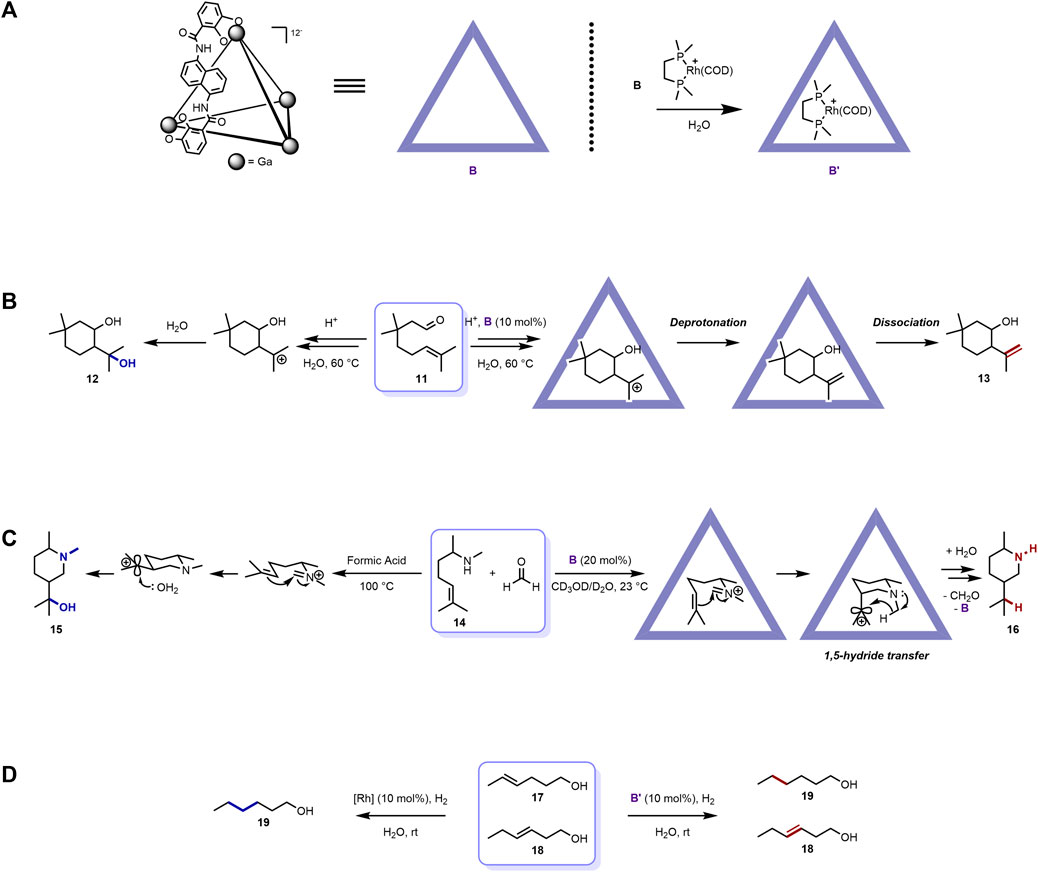
FIGURE 2. Examples of [Ga4L6]12- cage mediated organic reactions with distinct selectivity: (A) representative structures of [Ga4L6]12− cages; (B) cage mediated intramolecular Prins cyclization with distinct selectivity; (C) [Ga4L6]12− cage mediated aza-Prins cyclization with distinct selectivity; (D) [Ga4L6]12− cage mediated hydrogenation of olefins with distinct selectivity at the more terminal positions.
In 2012, Toste, Raymond, Bergman, and co-workers reported the catalytic intramolecular Prins cyclization of citronellal and its derivatives with distinct selectivity, which resembled the active sites of many terpene synthases (Hart-Cooper et al., 2012) (Figure 2B). When treated with buffered acidic solution, the citronellal derivative substrate (11) underwent cyclization to produce two main types of products, with the diol product (12) being major (Clark et al., 1984; Yuasa et al., 2000; Cheng et al., 2009). The authors then applied the [Ga4L6]12− cage host B to this reactive system to investigate the possibility of selectivity regulation. Not surprisingly, the product distribution was shifted, with the isopulegol-like product (13) being the major through deprotonation instead of nucleophilic capture by water of the cationic intermediate. As illustrated above, the negative charge–containing cage host [Ga4L6]12− can preferably recognize and stabilize cationic species on the one hand, while on the other, the inner space of B is hydrophobic and can prevent water from entering its interior to contact the encapsuled 11. The former factor stabilized and extended the lifetime of the cationic Prins cyclization intermediate; the latter hindered the nucleophilic attack of water that gave rise to the formation of the hydroxyl group. This work demonstrated the selectivity regulation ability of the [Ga4L6]12− cage host.
Later, the authors also reported another catalytic bimolecular aza-Prins cyclization inside the same cage host with new reactivity and selectivity (Kaphan et al., 2015) (Figure 2C). The reaction between the unsaturated amine substrate (14) and formaldehyde in formic acid bulk solution under reflux produced the alcohol product (15). In the reaction process, the amine group first condensed with formaldehyde to yield the iminium ion intermediate, which underwent aza-Prins cyclization and hydration. When B was incorporated into this system, however, a totally new pathway and product (16) arose under mild condition. The condensed iminium ion intermediate was subsequently encapsuled into the interior of the cage host with a constrictive spherical transition state, where the double bond was placed to an axial position owing to the steric inner microenvironment of the cavity. The iminium ion intermediate then cyclized to form the carbocation intermediate, followed by an unexpected transannular 1,5-hydride transfer process, which gave rise to 16 after hydrolysis. The subsequent systematic mechanism investigation revealed that the rate-limiting step of this special reaction was the encapsulation of the iminium ion intermediate and the unusual 1,5-hydride transfer process was supported through kinetic analysis and isotopic labeling studies. This unconventional reactivity and selectivity were due to the hydrophobic interior of the cage host, which prevents water from entering its inner space to attack the resulting carbocation, as well as the steric effect inside the cavity that poses the carbocation group to the axial position, close to the hydrogen of the carbon adjacent to the nitrogen, which favors the 1,5-hydride transfer process. This extraordinary example, in the authors’ words, “represents a rare example of such an extreme divergence of product selectivity observed within a catalytic metal-ligand supramolecular enzyme mimic” and “represents the most pronounced deviation in reactivity within a supramolecular catalyst to date.”
Recently, in 2019, the same collaboration groups reported the catalytic hydrogenation of olefins with distinct selectivity at the more terminal positions with the help of the [Ga4L6]12− cage host (Bender et al., 2019) (Figure 2D). In this work, host B was first mixed with a rhodium complex to produce the rhodium-encapsulated supramolecular catalyst B’. To illustrate the distinct selectivity of this catalyst, a mixture of methyl- and ethyl-substituted alkene substrates (17 and 18, respectively) were added to the catalyst aqueous solution under a H2 atmosphere at room temperature. After 20 h, only 17 was reduced, with nearly full retention of 18. However, with just the rhodium catalyst free in the bulk solution, both 17 and 18 were reduced to 19 within just 1 h. In this function mode, the reactive center of the catalyst was accommodated and buried inside the cavity, and only sterically accessible reactive sites (methyl substituted one) could enter the interior of the host to contact the rhodium catalyst center. In other words, the supramolecular container acted as a reaction flask for the catalyst and the reacting moiety, while the outside parts were “protected” from the reagent, which is in complete contrast to the abovementioned cases, where the supramolecular host functions as the noncovalent protecting groups. This beautiful example showed the diverse function of the promising supramolecular container for regulating distinct selectivity in various organic reactions.
2.3 Conclusion
To summarize, we have reviewed representative examples of how supramolecular containers, especially self-assembled coordination cages, could induce distinct selectivity in various organic reactions, different from that in the bulk solutions. The cage host is spontaneously assembled by metal ions and suitable ligands, with no need to form covalent bonds. It is water soluble, but its interior is highly hydrophobic and does not allow water into its cavity, which can generally alter the mechanism of water-associated processes and gives rise to distinct selectivity in these cases. On the other hand, the cage host can pose the encapsulated guest molecule(s) with certain fixed orientations and conformations to induce different site selectivity and even new reactivity. In some cases, the cage host acts as a noncovalent protecting group that shields certain parts of the substrate from the outside reagents, giving rise to distinct site selective products. In addition, the cage host could also function as a reaction vessel, and only the sterically accessible sites of the substrate could come inside the host to react with the catalyst center while the outside moieties could be “protected”. All the examples illustrated above demonstrate the powerful ability of self-assembled coordination cages in regulating reaction selectivity.
Author contributions
YL: Writing–original draft. XZ: Writing–original draft. SG: Writing–review and editing. RW: Conceptualization, Supervision, Writing–review and editing.
Funding
The author(s) declare financial support was received for the research, authorship, and/or publication of this article. This work was supported by the National Natural Science Foundation of China (No. 22101169).
Conflict of interest
The authors declare that the research was conducted in the absence of any commercial or financial relationships that could be construed as a potential conflict of interest.
The handling editor JH declared a past co-authorship with the author SG.
Publisher’s note
All claims expressed in this article are solely those of the authors and do not necessarily represent those of their affiliated organizations, or those of the publisher, the editors and the reviewers. Any product that may be evaluated in this article, or claim that may be made by its manufacturer, is not guaranteed or endorsed by the publisher.
References
Appel, T. R., Yehia, N. A. M., Baumeister, U., Hartung, H., Kluge, R., Ströhl, D., et al. (2003). Electrophilic cyclisation of bis(4-methoxybenzylthio)acetylene - competition between Ar2-6 and Ar1-5 routes, yielding 1H-2-benzothiopyrans or spiro derivatives of cyclohexadienone. Eur. J. Org. Chem. 1, 47–53. doi:10.1002/1099-0690(200301)2003:1<47:AID-EJOC47>3.0.CO;2-B
Ariga, K., and Kunitake, T. (2006). Supramolecular chemistry: Fundamentals and applications: Advanced textbook. Heidelberg: Springer.
Balcells, D., Clot, E., Eisenstein, O., Nova, A., and Perrin, L. (2016). Deciphering selectivity in organic reactions: A multifaceted problem. Acc. Chem. Res. 49, 1070–1078. doi:10.1021/acs.accounts.6b00099
Bender, T. A., Bergman, R. G., Raymond, K. N., and Toste, F. D. (2019). A supramolecular strategy for selective catalytic hydrogenation independent of remote chain length. J. Am. Chem. Soc. 141, 11806–11810. doi:10.1021/jacs.9b05604
Breslow, R. (1991). Hydrophobic effects on simple organic reactions in water. Acc. Chem. Res. 24, 159–164. doi:10.1021/ar00006a001
Caulder, D. L., Powers, R. E., Parac, T. N., and Raymond, K. N. (1998). The self-assembly of a predesigned tetrahedral M4L6 supramolecular cluster. Angew. Chem. Int. Ed. 37, 1840. doi:10.1002/(SICI)1521-3773(19980803)37:13/14<1840:AID-ANIE1840>3.0.CO;2-D
Chao, T-M., Baker, J., Hehre, W. J., and Kahn, S. D. (1991). Models for selectivity in organic reactions. Pure Appl Chem 63, 283–288. doi:10.1351/pac199163020283
Cheng, H., Meng, X., Liu, R., Hao, Y., Yu, Y., Cai, S., et al. (2009). Cyclization of citronellal to p-menthane-3,8-diols in water and carbon dioxide. Green Chem. 11, 1227. doi:10.1039/B823297K
Cheng, M-F., and Li, W-K. (2003). A computational study of the diels–alder reactions involving acenes: Reactivity and aromaticity. Chem. Phys. Lett. 368, 630–638. doi:10.1016/S0009-2614(02)01955-3
Clark, B. C., Chamblee, T. S., and Iacobucci, G. A. (1984). Acid-catalyzed cyclization of terpenoids in a micellar system. Selectivity and rate enhancement in the cyclization of citronellal. J. Org. Chem. 49, 4557–4559. doi:10.1021/jo00197a054
Cullen, W., Misuraca, M. C., Hunter, C. A., Williams, N. H., and Ward, M. D. (2016). Highly efficient catalysis of the Kemp elimination in the cavity of a cubic coordination cage. Nat. Chem. 8, 231–236. doi:10.1038/nchem.2452
Cullen, W., Takezawa, H., and Fujita, M. (2019). Demethylenation of cyclopropanes via photoinduced guest-to-host electron transfer in an M6L4 cage. Angew. Chem. Int. Ed. 58, 9171–9173. doi:10.1002/anie.201904752
Dalton, D. M., Ellis, S. R., Nichols, E. M., Mathies, R. A., Toste, F. D., Bergman, R. G., et al. (2015). Supramolecular Ga4L612– cage photosensitizes 1,3-rearrangement of encapsulated guest via photoinduced electron transfer. J. Am. Chem. Soc. 137, 10128–10131. doi:10.1021/jacs.5b06317
Davis, H. J., and Phipps, R. J. (2017). Harnessing non-covalent interactions to exert control over regioselectivity and site-selectivity in catalytic reactions. Chem. Sci. 8, 864–877. doi:10.1039/C6SC04157D
DeWolfe, R. H., and Young, W. G. (1956). Substitution and rearrangement reactions of allylic compounds. Chem. Rev. 56, 753–901. doi:10.1021/cr50010a002
Dohi, T., Kato, D., Hyodo, R., Yamashita, D., Shiro, M., and Kita, Y. (2011b). Discovery of stabilized bisiodonium salts as intermediates in the carbon-carbon bond formation of alkynes. Angew. Chem. Int. Ed. 50, 3784–3787. doi:10.1002/anie.201007640
Dohi, T., Nakae, T., Ishikado, Y., Kato, D., and Kita, Y. (2011a). New synthesis of spirocycles by utilizing in situ forming hypervalent iodine species. Org. Biomol. Chem. 9, 6899. doi:10.1039/C1OB06199B
Dou, C., Saito, S., Gao, L., Matsumoto, N., Karasawa, T., Zhang, H., et al. (2013). Sequential electrophilic and photochemical cyclizations from bis(bithienyl)acetylene to a tetrathienonaphthalene core. Org. Lett. 15, 80–83. doi:10.1021/ol303107y
Fringuelli, F., and Taticchi, A. (2002). The diels-alder reaction: Selected practical methods. Wiley.
Fujita, M., Oguro, D., Miyazawa, M., Oka, H., Yamaguchi, K., and Ogura, K. (1995). Self-assembly of ten molecules into nanometre-sized organic host frameworks. Nature 378, 469–471. doi:10.1038/378469a0
Furutani, Y., Kandori, H., Kawano, M., Nakabayashi, K., Yoshizawa, M., and Fujita, M. (2009). In situ spectroscopic, electrochemical, and theoretical studies of the photoinduced host−guest electron transfer that precedes unusual host-mediated alkane photooxidation. J. Am. Chem. Soc. 131, 4764–4768. doi:10.1021/ja8089075
García-Simón, C., Garcia-Borràs, M., Gómez, L., Parella, T., Osuna, S., Juanhuix, J., et al. (2014). Sponge-like molecular cage for purification of fullerenes. Nat. Commun. 5, 5557. doi:10.1038/ncomms6557
García-Simón, C., Gramage-Doria, R., Raoufmoghaddam, S., Parella, T., Costas, M., Ribas, X., et al. (2015). Enantioselective hydroformylation by a Rh-catalyst entrapped in a supramolecular metallocage. J. Am. Chem. Soc. 137, 2680–2687. doi:10.1021/ja512637k
Goldfinger, M. B., Crawford, K. B., and Swager, T. M. (1997). Directed electrophilic cyclizations: Efficient methodology for the synthesis of fused polycyclic aromatics. J. Am. Chem. Soc. 119, 4578–4593. doi:10.1021/ja9642673
Guo, J., Xu, Y-W., Li, K., Xiao, L-M., Chen, S., Wu, K., et al. (2017). Regio- and Enantioselective photodimerization within the confined space of a homochiral ruthenium/palladium heterometallic coordination cage. Angew. Chem. Int. Ed. 56, 3852–3856. doi:10.1002/anie.201611875
Hart-Cooper, W. M., Clary, K. N., Toste, F. D., Bergman, R. G., and Raymond, K. N. (2012). Selective monoterpene-like cyclization reactions achieved by water exclusion from reactive intermediates in a supramolecular catalyst. J. Am. Chem. Soc. 134, 17873–17876. doi:10.1021/ja308254k
Hong, C. M., Bergman, R. G., Raymond, K. N., and Toste, F. D. (2018). Self-assembled tetrahedral hosts as supramolecular catalysts. Acc. Chem. Res. 51, 2447–2455. doi:10.1021/acs.accounts.8b00328
Hooley, R. J., and Rebek, J. (2009). Chemistry and catalysis in functional cavitands. Chem. Biol. 16, 255–264. doi:10.1016/j.chembiol.2008.09.015
Housecroft, C. E. (2021). Supramolecular chemistry in the 3rd millennium. Chemistry 3, 509–510. doi:10.3390/chemistry3020035
Howlader, P., Das, P., Zangrando, E., and Mukherjee, P. S. (2016). Urea-functionalized self-assembled molecular prism for heterogeneous catalysis in water. J. Am. Chem. Soc. 138, 1668–1676. doi:10.1021/jacs.5b12237
Isidro-Llobet, A., Álvarez, M., and Albericio, F. (2009). Amino acid-protecting groups. Chem. Rev. 109, 2455–2504. doi:10.1021/cr800323s
Kaphan, D. M., Toste, F. D., Bergman, R. G., and Raymond, K. N. (2015). Enabling new modes of reactivity via constrictive binding in a supramolecular-assembly-catalyzed aza-Prins cyclization. J. Am. Chem. Soc. 137, 9202–9205. doi:10.1021/jacs.5b01261
Klán, P., Šolomek, T., Bochet, C. G., Blanc, A., Givens, R., Rubina, M., et al. (2013). Photoremovable protecting groups in chemistry and biology: Reaction mechanisms and efficacy. Chem. Rev. 113, 119–191. doi:10.1021/cr300177k
Koblenz, T. S., and WassenaarReek, J. J. N. H. (2008). Reactivity within a confined self-assembled nanospace. Chem. Soc. Rev. 37, 247–262. doi:10.1039/B614961H
Kohyama, Y., Murase, T., and Fujita, M. (2012). A self-assembled cage as a non-covalent protective group: Regioselectivity control in the nucleophilic substitution of aryl-substituted allylic chlorides. Chem. Commun. 48, 7811. doi:10.1039/c2cc33660j
Kolesnichenko, I. V., and Anslyn, E. V. (2017). Practical applications of supramolecular chemistry. Chem. Soc. Rev. 46, 2385–2390. doi:10.1039/C7CS00078B
Li, C-W., Wang, C-I., Liao, H-Y., Chaudhuri, R., and Liu, R-S. (2007). Synthesis of dibenzo[g,p]chrysenes from bis(biaryl)acetylenes via sequential ICl-induced cyclization and Mizoroki-Heck coupling. J. Org. Chem. 72, 9203–9207. doi:10.1021/jo701504m
Martí-Centelles, V., Lawrence, A. L., and Lusby, P. J. (2018). High activity and efficient turnover by a simple, self-assembled ‘artificial Diels-Alderase. J. Am. Chem. Soc. 140, 2862–2868. doi:10.1021/jacs.7b12146
Morimoto, M., Bierschenk, S. M., Xia, K. T., Bergman, R. G., Raymond, K. N., and Toste, F. D. (2020). Advances in supramolecular host-mediated reactivity. Nat. Catal. 3, 969–984. doi:10.1038/s41929-020-00528-3
Murase, T., and Fujita, M. (2010). Pericyclic reactions in an aqueous molecular flask. Chem. Rec. 10, 342–347. doi:10.1002/tcr.201000027
Murase, T., Nishijima, Y., and Fujita, M. (2012). Unusual photoreaction of triquinacene within self-assembled hosts. Chem. Asian J. 7, 826–829. doi:10.1002/asia.201101005
Murase, T., Takezawaa, H., and Fujita, M. (2011). Photo-driven anti-Markovnikov alkyne hydration in self-assembled hollow complexes. Chem. Commun. 47, 10960. doi:10.1039/C1CC14523A
Neufeldt, S. R., and Sanford, M. S. (2012). Controlling site selectivity in palladium-catalyzed C–H bond functionalization. Acc. Chem. Res. 45, 936–946. doi:10.1021/ar300014f
Olivo, G., Capocasa, G., Del Giudice, D., Lanzalunga, O., and Di Stefano, S. (2021). New horizons for catalysis disclosed by supramolecular chemistry. Chem. Soc. Rev. 50, 7681–7724. doi:10.1039/D1CS00175B
Purse, B. W., and Rebek, J. (2005). Functional cavitands: Chemical reactivity in structured environments. Proc. Natl. Acad. Sci. U. S. A. 102, 10777–10782. doi:10.1073/pnas.0501731102
Schelhaas, M., and Waldmann, H. (1996). Protecting group strategies in organic synthesis. Angew. Chem. Int. Ed. 35, 2056–2083. doi:10.1002/anie.199620561
Stuhlmann, F., and Jäschke, A. (2002). Characterization of an RNA active site: Interactions between a diels-alderase ribozyme and its substrates and products. J. Am. Chem. Soc. 124, 3238–3244. doi:10.1021/ja0167405
Takezawa, H., Fujii, Y., Murase, T., and Fujita, M. (2022). Electrophilic spirocyclization of a 2-biphenylacetylene via conformational fixing within a hollow-cage host. Angew. Chem. Int. Ed. 61, e202203970. doi:10.1002/anie.202203970
Takezawa, H., Kanda, T., Nanjo, H., and Fujita, M. (2019). Site-selective functionalization of linear diterpenoids through U-shaped folding in a confined artificial cavity. J. Am. Chem. Soc. 141, 5112–5115. doi:10.1021/jacs.9b00131
Tang, B-X., Zhang, Y-H., Song, R-J., Tang, D-J., Deng, G-B., Wang, Z-Q., et al. (2012). Intramolecular ipso-halocyclization of 4-(p-unsubstituted-aryl)-1-alkynes leading to spiro[4,5]trienones: Scope, application, and mechanistic investigations. J. Org. Chem. 77, 2837–2849. doi:10.1021/jo300037n
Ueda, Y., Ito, H., Fujita, D., and Fujita, M. (2017). Permeable self-assembled molecular containers for catalyst isolation enabling two-step cascade reactions. J. Am. Chem. Soc. 139, 6090–6093. doi:10.1021/jacs.7b02745
Vriezema, D. M., Comellas Aragonès, M., Elemans, J. A. A. W., Cornelissen, J. J. L. M., Rowan, A. E., and Nolte, R. J. M. (2005). Self-assembled nanoreactors. Chem. Rev. 105, 1445–1490. doi:10.1021/cr0300688
Yamaguchi, S., and Swager, T. M. (2001). Oxidative cyclization of bis(biaryl)acetylenes: Synthesis and photophysics of dibenzo[g,p]chrysene-based fluorescent polymers. J. Am. Chem. Soc. 123, 12087–12088. doi:10.1021/ja016692o
Yoshizawa, M., Miyagi, S., Kawano, M., Ishiguro, K., and Fujita, M. (2004). Alkane oxidation via photochemical excitation of a self-assembled molecular cage. J. Am. Chem. Soc. 126, 9172–9173. doi:10.1021/ja047612u
Yoshizawa, M., Tamura, M., and Fujita, M. (2006). Diels-alder in aqueous molecular hosts: Unusual regioselectivity and efficient catalysis. Science 312, 251–254. doi:10.1126/science.1124985
Yu, F., Poole, D., Mathew, S., Yan, N., Hessels, J., Orth, N., et al. (2018). Control over electrochemical water oxidation catalysis by preorganization of molecular ruthenium catalysts in self-assembled nanospheres. Angew. Chem. Int. Ed. 57, 11247–11251. doi:10.1002/anie.201805244
Yu, Q-F., Zhang, Y-H., Yin, Q., Tang, B-X., Tang, R-Y., Zhong, P., et al. (2008). Electrophilic ipso-iodocyclization of N-(4-methylphenyl)propiolamides: Selective synthesis of 8-methyleneazaspiro[4,5]trienes. J. Org. Chem. 73, 3658–3661. doi:10.1021/jo800328a
Yu, Y., and Rebek, J. (2018). Reactions of folded molecules in water. Acc. Chem. Res. 51, 3031–3040. doi:10.1021/acs.accounts.8b00269
Yuasa, Y., Tsuruta, H., and Yuasa, Y. (2000). A practical and efficient synthesis of p-menthane-3,8-diols. Org. Process Res. Dev. 4, 159–161. doi:10.1021/op9901036
Keywords: distinct selectivity, self-assembled coordination cages, constrained environment, confined spaces, noncovalent protecting group
Citation: Liang Y, Zhou X, Gopi S and Wang R (2023) Distinct selectivity inside self-assembled coordination cages. Front. Chem. 11:1269471. doi: 10.3389/fchem.2023.1269471
Received: 30 July 2023; Accepted: 21 August 2023;
Published: 05 September 2023.
Edited by:
Józef Haponiuk, Gdansk University of Technology, PolandReviewed by:
Arindam Mukhopadhyay, Idaho National Laboratory (DOE), United StatesCopyright © 2023 Liang, Zhou, Gopi and Wang. This is an open-access article distributed under the terms of the Creative Commons Attribution License (CC BY). The use, distribution or reproduction in other forums is permitted, provided the original author(s) and the copyright owner(s) are credited and that the original publication in this journal is cited, in accordance with accepted academic practice. No use, distribution or reproduction is permitted which does not comply with these terms.
*Correspondence: Rui Wang, d2FuZ3J1aTE5OTBAc2h1LmVkdS5jbg==; Sreeraj Gopi, ZHJnb3Bpc3JlZXJhakBnbWFpbC5jb20=
†These authors have contributed equally to this work and share first authorship