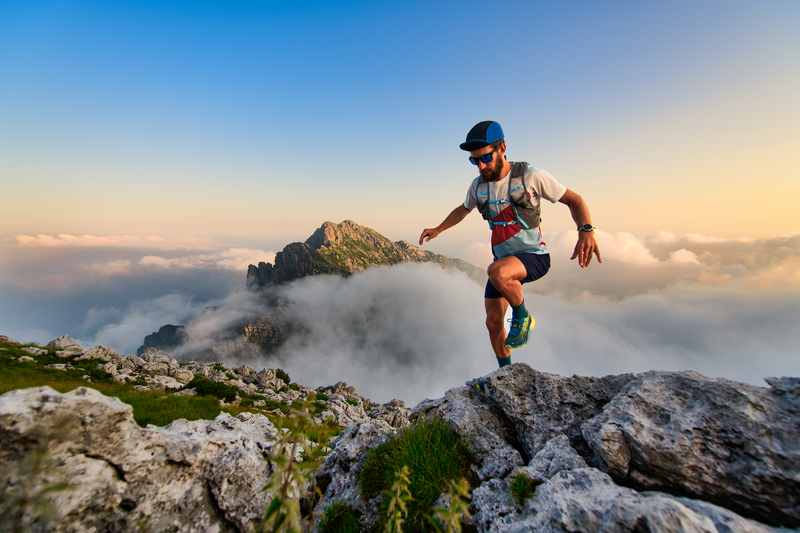
95% of researchers rate our articles as excellent or good
Learn more about the work of our research integrity team to safeguard the quality of each article we publish.
Find out more
ORIGINAL RESEARCH article
Front. Chem. , 01 September 2023
Sec. Electrochemistry
Volume 11 - 2023 | https://doi.org/10.3389/fchem.2023.1264593
This article is part of the Research Topic Semiconducting Materials for Photoelectrochemical Water Splitting View all 4 articles
Li–O2 batteries are a promising technology for the upcoming energy storage requirements because of their high theoretical specific energy density of 11,680 Wh kg−1. Currently, the actual capacity of Li–O2 batteries is much lower than this theoretical value. In many studies, perovskites have been applied as catalysts to improve the air electrode reactions in Li–O2 batteries. The effects of structure and doping on the catalytic activity of perovskites are still unclear. La1-xSrxCoO3-δ (x = 0.1, 0.3, and 0.5) and La0.9Sr0.1YbO3-δ mixed with carbon black (Vulcan XC500 or Super P) were used as air electrode catalysts. Electrochemical characterizations were conducted using a Swagelok-type cell. The charge–discharge capacity and cyclic voltammetry (CV) performance were investigated in this study. The La1-xSrxCoO3-δ (x = 0.1, 0.3, and 0.5) is a suitable cathode catalyst for Li–O2 batteries. In this study, the La0.5Sr0.5CoO3-δ/Super P cathode demonstrated the highest discharge capacity (6,032 mAh g−1). This excellent performance was attributed to the large reaction area and enhanced Li2CO3 generation.
• La-based perovskites mixed with carbon black were used as cathode catalysts in Li–O2 batteries.
• The cathode with La0.5Sr0.5CoO3-δ/Super P demonstrated the highest charge–discharge capacity.
• Production of Li2CO3 increased discharge capacity.
Lithium-ion batteries (LIBs) are widely utilized in laptops, smartphones, power banks, renewable energy systems, and electric vehicles worldwide (Lu et al., 2013; Li et al., 2018; Dunn et al., 2021; Muruganantham et al., 2022; Zhu et al., 2022; Anirudha et al., 2023). However, the density of energy storage in LIBs is still insufficient to fulfill the increasing energy requirements for advanced electric transportation (Wei et al., 2021; Luo et al., 2022). The recently developed metal–air batteries, including Zn–oxygen, Na–oxygen, and Li–oxygen (Li–O2) batteries, have attracted considerable interest because of their advantages such as low cost, high flexibility, and high theoretical energy density (Hardin et al., 2013; Rahman et al., 2013; Kang et al., 2022; Li et al., 2022; Salado and Lizundia, 2022; Yang et al., 2022). In addition, in comparison with LIBs, Li–O2 batteries offer much higher gravimetric energy density, which could reach the theoretical value of 11,680 Wh kg−1 because oxygen electrodes can directly use oxygen from the surrounding environment while discharging; therefore, oxygen does not need to be stored within the battery (Girishkumar et al., 2010). However, Li–O2 batteries have been facing several serious challenges, including high overvoltage, poor rate capacity, and short cycle life, which are mainly caused by the sluggish dynamics of the air electrode during Li2O2 formation (2Li+ + 2e− + O2 → Li2O2, oxygen reduction reaction (ORR)) and Li2O2 decomposition [Li2O2 → 2Li+ + 2e− + O2, oxygen evolution reaction (OER)] (Thapa et al., 2010; Thapa and Ishihara, 2011; Pan et al., 2019; Cui et al., 2021; Yin et al., 2021; Zhan et al., 2021). Furthermore, battery performance is also affected by the components present in ambient air, resulting in significantly lower practical specific energy (Zhang et al., 2018).
Recently, Li–O2/CO2 batteries have attracted considerable attention because they capture and utilize carbon (Takechi et al., 2011; Lim et al., 2013; Yin et al., 2017; Zou et al., 2019; Chen et al., 2020; Savunthari et al., 2021; Iputera et al., 2022; Wang et al., 2023; Wu et al., 2023). In addition, during discharge, Li+ ions react with O2 and CO2 to produce Li2O2 and Li2CO3. These products can improve the battery capacity by 289% compared to batteries operating on 100% O2 (Takechi et al., 2011). Therefore, the generation of Li2CO3 is beneficial for battery capacity. At the same time, Li2O2 and Li2CO3 that precipitate on the air electrode surface during discharge are difficult to completely decompose during charge (Gallant et al., 2012). These discharge products (Li2O2 and Li2CO3) block the pores of the air electrode and thus cause performance degradation because they hinder air supply and liquid electrolyte diffusion (Liu et al., 2017; Zhao et al., 2018). Hence, it is necessary to promote Li2CO3 generation on oxygen electrodes during discharge and decomposition of discharge products during charge.
Because reactions on the air electrode significantly affect battery performance, many studies have focused on enhancing the electrocatalytic activity of the oxygen electrode. Therefore, catalyst addition to oxygen electrodes in Li–O2 batteries is necessary to improve the electrochemical activities of ORR during discharge and OER during charging (Thapa et al., 2010; Thapa and Ishihara, 2011; Pan et al., 2019; Yin et al., 2021). Currently, Pt-based catalysts are considered excellent catalysts for the ORR and OER (Wu and Yang, 2013; Liu et al., 2019), and IrO2 is known to be the best catalyst for the OER (Lee et al., 2012). Although these materials show excellent performance, their high cost limits their application in Li–O2 batteries. Recently, some studies have demonstrated that perovskite catalysts are beneficial for oxygen reduction during discharge and the decomposition of discharge products during charge (Xu et al., 2013; Du et al., 2014; Jin et al., 2014; Zhang et al., 2014; Ma et al., 2020; Du et al., 2021). Oxygen vacancies in the perovskite LaCoO3 have been reported to enhance the bifunctional catalytic activity (ORR and OER) because of the valence electron transformation of the Co ions (Du et al., 2021). In addition, the battery discharge capacity and long-term cycling stability were also remarkably increased. Zhang et al. used porous LaNiO3 as a catalyst for an air electrode and improved the discharge capacity from 2,545 to 3,407 mAh g−1 (Zhang et al., 2014). However, it is unclear how the catalysts affect the discharge products and battery capacity.
In this study, La1-xSrxCoO3-δ (x = 0.1, 0.3, and 0.5) and La0.9Sr0.1YbO3-δ were used as catalysts for oxygen electrode to determine their effects on Li–O2 battery capacity. Additionally, the performances of two types of carbon black as cathode substrates were tested. Finally, the discharge products were analyzed to explain the differences in battery performance for different cathode materials. The findings of this study are expected to facilitate the development of catalysts for air electrodes in Li–O2 batteries.
The sol–gel method was used to synthesize La1-xSrxCoO3-δ (x = 0.1 (L9SC), 0.3 (L7SC), and 0.5 (L5SC)) and La0.9Sr0.1YbO3-δ (L9SYb) catalysts. La(NO3)2 · 6H2O (purity 99.9%, Alfa Aesar, United States), Sr(NO3)2 (purity 99%, Alfa Aesar, United States), Co(NO3)2 · 6H2O (purity 98%, Acros Organics, United States), Yb(NO3)3⋅6H2O (purity 99.9%, Strem Chemicals Inc., United States), citric acid (J. T. Baker, United States), and ethylenediaminetetraacetic acid (Alfa Aesar, Spain) were separately dissolved in deionized water. The precursor solution was heated under stirring at 200°C until a gel-like phase was obtained. The dry gel was completely burned at 300°C to form a powder, which was then ground and calcination in air at 1,100°C for 5 h to obtain the L9SC, L7SC, L5SC, and L9SYb powders.
The phase compositions of the synthesized materials were identified by X-ray diffraction (XRD; Malvern Panalytical Empyrean, Cu Kɑ). All the as-prepared materials were carefully sieved, and the sub-25-µm fraction was used in the XRD analysis. In addition, the compositions of cathode materials were determined using XRD before and after one charge–discharge cycle.
Electrochemical characterization was conducted using a Swagelok-type cell. The cathode was formed by casting a mixture of La-based perovskites, carbon black (Vulcan XC500 or Super P), and polytetrafluoroethylene (wt. ratio of 4.25:4.25:1.5) and then pressing the mixture onto a carbon paper (GD210, CeTech Co., Ltd., Taiwan). Lithium foil was used as the anode and was separated with a porous polypropylene film (FinTech Co., Ltd. Taiwan). Electrochemical measurements were performed using gastight Swagelok-type cells, with the exception of a stainless-steel window that enabled exposure to O2 gas. Lithium bis(trifluoromethanesulfonyl) (1 M in tetraethylene glycol dimethyl ether) was used as the electrolyte. The charge–discharge performance was determined in the voltage range of 2.4–4.3 V at a constant current of 0.1 mA cm−2 in O2 atmosphere.
CV was performed using the same Swagelok cell at a scan rate of 0.1 mV s−1 in the voltage range of 2.0–4.5 V on the Princeton V3.
The phase purity of L9SC, L7SC, L5SC, and L9SYb powders was verified by analyzing their crystal structures using XRD (Figure 1). The diffraction peaks of L9SC, L7SC, L5SC, and L9SYb phases match well with the corresponding reference patterns thus confirming that each as-prepared material is composed of a single major phase. In the case of La1-xSrxCoO3-δ, the formation of impurity phases with increasing Sr doping is not observed, as shown in Figure 1. Therefore, the as-prepared materials have sufficiently high purities and crystallinities for use as catalysts.
Figure 2 shows the charge–discharge curves for Li–O2 in the voltage range of 4.3–2.4 V at a constant current of 0.1 mA cm−2 at room temperature. First, only carbon black (Vulcan XC500 and Super P) electrodes without catalysts were tested in one-cycle charge-discharge tests (Figure 2A). The charge–discharge capacity results are listed in Table 1. These results indicate that the performance of Super P is superior to that of Vulcan XC500. In particular, the discharge capacity of Super P is almost twice that of Vulcan XC500. Super P, with a large specific surface area, could provide more reaction sites for Li+ ions from the anode (Wang et al., 2018). Subsequently, as shown in Figure 2B, L5SC was used as a catalyst and mixed with Vulcan XC500 and Super P, and the obtained cathode was tested. The results show that the addition of L5SC improved both the charge and discharge capacities. In the case of Vulcan XC500, the charge capacity increased from 926 to 2,247 mAh g−1, and the discharge capacity increased from 1,394 to 2,108 mAh g−1. This indicates that L5SC can promote the OER and ORR in Li−O2 batteries. Furthermore, in the case of Super P, the charge and discharge capacities were significantly enhanced from 955 to 5,500 mAh g−1 and from 2,253 to 6,032 mAh g−1, respectively. Super P has a large specific surface area (62 m2/g) for mixing with L5SC; therefore, the addition of L5SC produced a greater effect on OER and ORR of the Li−O2 battery, resulting in excellent Li−O2 battery performance.
FIGURE 2. Charge–discharge curves for Li–O2 with different air electrodes in the voltage range of 4.3–2.4 V at a constant current of 0.1 mA cm−2 at room temperature. (A) Carbon black (Vulcan XC500 or Super P) without catalysts used as the air electrode. (B) Carbon black (Vulcan XC500 or Super P) with L5SC catalyst used as the air electrode.
TABLE 1. Capacities obtained in the charge–discharge test for carbon black (XC500 or Super P) air electrodes without and with L5SC.
The capacities of cathodes made of Vulcan XC500 mixed with different catalysts (L5SC, L7SC, and L9SC) were measured using one-cycle charge–discharge tests (Figure 3; Table 2). According to the results, the capacities show the same tendencies during charge and discharge. The L5SC/XC500 electrode shows the best performance, and the L7SC/XC500 electrode shows a higher capacity than the L9SC/XC500 electrode. These results suggest that catalyst activity could be promoted by increasing the number of oxygen vacancies. It has been previously shown that the catalytic activity of Sr-doped LaCoO3 in the OER could increase with the number of oxygen vacancies (Mefford et al., 2016; Lu et al., 2019). Furthermore, the number of oxygen vacancies in perovskites has also been reported to be related to catalytic performance for ORR (Gayen et al., 2020; Ji et al., 2020). Therefore, it was considered that the charge and discharge capacities increased because the oxygen vacancies in Sr-doped LaCoO3 promoted the OER and ORR of the Li–O2 batteries.
FIGURE 3. One-cycle charge–discharge test results for Vulcan XC500 cathode with different catalysts (L5SC, L7SC, and L9SC).
TABLE 2. Charge–discharge capacity of the Vulcan XC500 cathode with different catalysts (L5SC, L7SC, and L9SC).
The capacities of cathodes made of Super P mixed with different catalysts (L5SC, L7SC, L9SC, and L9SYb) were measured using one-cycle charge–discharge tests (Figure 4; Table 3). According to the results, the capacities show the same tendencies during charge and discharge. The L5SC/Super P demonstrated the highest capacity in this study. This is because L5SC has the highest catalytic activity in the OER and ORR, and Super P has a larger specific surface area than Vulcan XC500. In addition, compared with Sr-doped LaCoO3, L9SYb shows relatively low catalyst activity in OER and ORR. In fact, the OER and ORR performances of L9SYb were previously investigated in only a few studies because of its low catalytic activity. Therefore, the effects of the catalysts can be easily elucidated by comparing the differences between the performances of L5SC and L9Yb.
FIGURE 4. Capacity of Super P cathode with different catalysts (L5SC, L7SC, L9SC, and L9SYb) in one-cycle charge–discharge test.
TABLE 3. Capacities of Super P cathodes with different catalysts (L5SC, L7SC, L9SC, and L9SYb) in charge–discharge tests.
Figure 5 shows XRD results for L5SC/Super P and L9SYb/Super P before and after one-cycle charge–discharge test. In Figure 5, L5SC and L9SYb are labeled with blue triangle and red cross, respectively, and Li2CO3 and Li2O2 are labeled with blue circle and orange diamond, respectively. The comparison of Figures 5A, B shows that more Li2CO3 was generated on the L5SC/Super P cathode than on the L9SYb/Super P cathode. This indicates that L5SC promoted the reaction between C (from electrolyte solvent or cathode material), O2, and Li+ to generate Li2CO3 during discharge. According to the literature, the generation of Li2CO3 can increase the capacity of Li/CO2–O2 batteries (Takechi et al., 2011; Zou et al., 2019). Yin et al. suggested that two electrons are involved in the generation of Li2CO3 (Yin et al., 2017). At the same time, only one electron is involved in the formation of Li2O2. Therefore, the generation of Li2CO3 could significantly increase the discharge capacity of L5SC/Super P catalysts. We could not determine the amount of Li2CO3 produced through XRD measurements; therefore, we conducted CV experiments on Li–O2 batteries with L5SC/Super P and L9SYb/Super P cathodes (Figure 6).
FIGURE 5. XRD results before and after one-cycle charge–discharge test for (A) L5SC/Super P and (B) L9SYb/Super P.
CV curves for Li–O2 batteries with L5SC/Super P and L9SYb/Super P were obtained in the voltage range of 2.0–4.5 V at a constant current of 0.1 mA cm−2 (Figure 6). To observe complete reduction and oxidation peaks in CV curves, they were obtained in the potential range of 4.5–2.0 V. The ORR peak in CV curves corresponds to the generation of Li2O2 and Li2CO3, while the OER peak corresponds to the evolution of O2 and CO2 from Li2O2 and Li2CO3. In addition, according to previous studies, the theoretical voltage for Li2O2 oxidation is 2.90 V for the reaction Li2O2 → 2Li+ + 2e− + O2 (Lim et al., 2013). At the same time, the theoretical voltage for the oxidation of Li2CO3 is 3.82 V through the reaction Li2CO3 → 2Li+ + 2e− + 1/2O2 + CO2 (Lim et al., 2013). Therefore, Li2CO3 is more chemically stable than Li2O2. The mentioned voltage levels (2.9 and 3.82 V) are indicated in Figure 6. In Figure 6, for both cathodes, the current density during oxidation increased at 2.9 V and reached a maximum at 4.5 V. In particular, for L5SC/Super P, the current density significantly increases in the voltage range of 3.82–4.5 V. In addition, the CV curve area of the L5SC/Super P battery is larger than that of the L9SYb/Super P battery. This indicates that a greater amount of generators in the L5SC/Super P cathode was oxidized and decomposed to Li ions and gases during oxidation. Above 3.82 V this phenomenon is more pronounced. This suggests that more Li2CO3 was produced in the L5SC/Super P cathode. Therefore, the L5SC/Super P cathode demonstrated the best discharge capacity in this study because of promoted Li2CO3 generation.
The electrochemical impedance spectroscopy (EIS) results during charge and after discharge are shown in Figure 7. For L5SC/Super P, the ohmic and polarization resistances significantly increase after discharge. This indicates that a greater amount of non-conductive Li2O2 and Li2CO3 was generated in L5SC/Super P, which blocked electronic conduction and O2 diffusion. As shown in Figures 7A, B, the polarization resistance of L9SYb/Super P is larger than that of L5SC/Super P, which also indicates that L9SYb has lower catalytic activity than L5SC.
In this study, the charge–discharge performances of L9SC, L7SC, L5SC, and L9SYb catalysts for Li-O2 batteries were investigated. In addition, the charge–discharge performances of two types of carbon black (XC500 and Super P) were determined. According to the literature, La1-xSrxCoO3-δ is as a superior OER and ORR catalyst compared to La0.9Sr0.1YbO3-δ. Additionally, an increase in the number of oxygen vacancies and an increase in the surface area by blending with Super P carbon have been previously reported to improve the catalytic activity in cathodic reactions in Li–O2 batteries. In this study, the L5SC/Super P air electrode showed the best charge and discharge capacities corroborating the abovementioned findings from the literature. In addition, the type of generator (Li2O2 or Li2CO3) could be considered a factor that affects the discharge capacity. The results of this study show that the battery capacity increases with the amount of Li2CO3 generated. The L5SC/Super P cathode material promoted the production of Li2CO3 and thus showed excellent performance. At the same time, based on XRD and CV results, L9SYb/Super P showed low Li2CO3 yields, which also indicated that it did not promote the reaction of Li+ ions with oxygen.
The raw data supporting the conclusion of this article will be made available by the authors, without undue reservation.
B-ZH: Investigation, Methodology, Writing–original draft. J-KL: Investigation, Software, Writing–review and editing. Y-HL: Conceptualization, Funding acquisition, Project administration, Supervision, Visualization, Writing–review and editing.
The author(s) declare financial support was received for the research, authorship, and/or publication of this article. This study was supported by the Ministry of Science and Technology of Taiwan [Grant No. MOST 110-2221-E-027 -058 -].
The authors declare that the research was conducted in the absence of any commercial or financial relationships that could be construed as a potential conflict of interest.
The handling editor DW declared a shared affiliation with the author BH, JL, and YL at the time of review.
All claims expressed in this article are solely those of the authors and do not necessarily represent those of their affiliated organizations, or those of the publisher, the editors and the reviewers. Any product that may be evaluated in this article, or claim that may be made by its manufacturer, is not guaranteed or endorsed by the publisher.
Anirudha, J., Bazri, B., Tong, Z., Iputera, K., Huang, J. Y., Wei, D. H., et al. (2023). Controlling cell components to design high-voltage all-solid-state lithium-ion batteries. ChemSusChem 7, e202202151. doi:10.1002/cssc.202202151
Chen, C. J., Yang, J. J., Chen, C. H., Wei, D. H., Hu, S. F., and Liu, R. S. (2020). Improvement of lithium anode deterioration for ameliorating cyclabilities of non-aqueous Li–CO2 batteries. Nanoscale 12, 8385–8396. doi:10.1039/d0nr00971g
Cui, X., Luo, Y., Zhou, Y., Dong, W., and Chen, W. (2021). Application of functionalized graphene in Li-O2 batteries. Nanotechnology 32, 132003. doi:10.1088/1361-6528/abd1a7
Du, D., Zheng, R., Chen, X., Xiang, W., Zhao, C., et al. (2021). Adjusting the covalency of metal-oxygen bonds in LaCoO3 by Sr and Fe cation codoping to achieve highly efficient electrocatalysts for aprotic lithium-oxygen batteries. ACS Appl. Mater Interfaces 13 (28), 33133–33146. doi:10.1021/acsami.1c08586
Du, Z., Yang, P., Wang, L., Lu, Y., Goodenough, J. B., Zhang, J., et al. (2014). Electrocatalytic performances of LaNi1-xMgxO3 perovskite oxides as bi-functional catalysts for lithium air batteries. J. Power Sources 265, 91–96. doi:10.1016/j.jpowsour.2014.04.096
Dunn, J., Slattery, M., Kendall, A., and AmbrosevH, S. S. (2021). Circularity of lithium-ion battery materials in electric vehicles. Environ. Sci. Technol. 55 (8), 5189–5198. doi:10.1021/acs.est.0c07030
Gallant, B. M., Mitchell, R. R., Kwabi, D. G., Zhou, J., Zuin, L., Thompson, C. V., et al. (2012). Chemical and morphological changes of Li–O2 battery electrodes upon cycling. J. Phys. Chem. C 116, 20800–20805. doi:10.1021/jp308093b
Gayen, P., Saha, S., Bhattacharyya, K., and Ramani, V. K. (2020). Oxidation state and oxygen-vacancy-induced work function controls bifunctional oxygen electrocatalytic activity. ACS Catal. 10 (14), 7734–7746. doi:10.1021/acscatal.0c01541
Girishkumar, G., McCloskey, B., Luntz, A. C., Swanson, S., and Wilcke, W. (2010). Lithium-air battery: Promise and challenges. J. Phys. Chem. Lett. 1 (14), 2193–2203. doi:10.1021/jz1005384
Hardin, W. G., Slanac, D. A., Wang, X., Dai, S., Johnston, K. P., and Stevenson, K. J. (2013). Highly active, nonprecious metal perovskite electrocatalysts for bifunctional metal–air battery electrodes. J. Phys. Chem. Lett. 4, 1254–1259. doi:10.1021/jz400595z
Iputera, K., Fu, Y. L., Li, L., Hu, S. F., Wei, D. H., and Liu, R. S. (2022). H2O wash: A simple method toward eliminating discharge products and regenerating cathodes of Li–O2 batteries. Green Chem. 24, 9755–9762. doi:10.1039/d2gc01817a
Ji, Q., Bi, L., Zhang, J., Cao, H., and Zhao, X. S. (2020). The role of oxygen vacancies of ABO3 perovskite oxides in the oxygen reduction reaction. Energy Environ. Sci. 13 (5), 1408–1428. doi:10.1039/d0ee00092b
Jin, C., Yang, Z., Cao, X., Lu, F., and Yang, R. (2014). A novel bifunctional catalyst of Ba0.9Co0.5Fe0.4Nb0.1O3-δ perovskite for lithium-air battery. Int. J. Hydrog. Energy 39 (6), 2526–2530. doi:10.1016/j.ijhydene.2013.12.003
Kang, H., Peng, H., Kang, Y., Hao, Y., Yan, X., Li, L., et al. (2022). Porous rare earth-transition metal bimetallic oxide nanoparticles oxygen electrocatalyst for rechargeable Zinc-air battery. J. Taiwan Inst. Chem. E 134, 104280. doi:10.1016/j.jtice.2022.104280
Lee, Y., Suntivich, J., May, K. J., Perry, E. E., and Shao-Horn, Y. (2012). Synthesis and activities of rutile IrO2 and RuO2 nanoparticles for oxygen evolution in acid and alkaline solutions. J. Phys. Chem. Lett. 3 (3), 399–404. doi:10.1021/jz2016507
Li, M., Lu, J., Chen, Z., and Amine, K. (2018). 30 years of lithium-ion batteries. Adv. Mater 30, 1800561. doi:10.1002/adma.201800561
Li, S., Guo, H., He, S., Yang, H., Liu, K., Duan, G., et al. (2022). Advanced electrospun nanofibers as bifunctional electrocatalysts for flexible metal-air (O2) batteries: Opportunities and challenges. Mater Des. 214, 110406. doi:10.1016/j.matdes.2022.110406
Lim, H. K., Lim, H. D., Park, K. Y., Seo, D. H., Gwon, H., Hong, J., et al. (2013). Toward a lithium–“air” battery: The effect of CO2 on the chemistry of a lithium–oxygen cell. J. Am. Chem. Soc. 135 (26), 9733–9742. doi:10.1021/ja4016765
Liu, M., Zhao, Z., Duan, X., and Huang, Y. (2019). Nanoscale structure design for high-performance Pt-based ORR catalysts. Adv. Mater 31 (6), 1802234. doi:10.1002/adma.201802234
Liu, Y., Wang, L., Cao, L., Shang, C., Wang, Z., Wang, H., et al. (2017). Understanding and suppressing side reactions in Li–air batteries. Mater Chem. Front. 1, 2495–2510. doi:10.1039/c7qm00353f
Lu, L., Han, X., Li, J., Hua, J., and Ouyang, M. (2013). A review on the key issues for lithium-ion battery management in electric vehicles. J. Power Sources 226, 272–288. doi:10.1016/j.jpowsour.2012.10.060
Lu, Y., Ma, A., Yu, Y., Tan, R., Liu, C., Zhang, P., et al. (2019). Engineering oxygen vacancies into LaCoO3 perovskite for efficient electrocatalytic oxygen evolution. ACS Sustain. Chem. Eng. 7 (3), 2906–2910. doi:10.1021/acssuschemeng.8b05717
Luo, J., Li, H., Yu, G., Xu, W., Yin, H., and Hou, Z. (2022). Multifunctional sandwich-structured double-carbon-layer modified SnS nanotubes with high capacity and stability for Li-ion batteries. Mater Adv. 3, 3631–3641. doi:10.1039/d2ma00017b
Ma, S. B., Kwon, H. J., Kim, M., Bak, S. M., Lee, H., Ehrlich, S. N., et al. (2020). Mixed ionic-electronic conductor of perovskite LixLayMO3-δ toward carbon-free cathode for reversible lithium-air batteries. Adv. Energy Mater 10, 2001767. doi:10.1002/aenm.202001767
Mefford, J. T., Rong, X., Abakumov, A. M., Hardin, W. G., Dai, S., Kolpak, A. M., et al. (2016). Water electrolysis on La1-xSrxCoO3-δ perovskite electrocatalysts. Nat. Commun. 7, 11053. doi:10.1038/ncomms11053
Muruganantham, R., Lin, M. C., Wang, P. K., Chang, B. K., and Liu, W. R. (2022). Highly effective Al-doped titanium niobate porous anode material for rechargeable high-rate Li-ion storage performance. J. Taiwan Inst. Chem. E 131, 104187. doi:10.1016/j.jtice.2021.104187
Pan, J., Tian, X. L., Zaman, S., Dong, Z., Liu, H., Park, H. S., et al. (2019). Recent progress on transition metal oxides as bifunctional catalysts for lithium-air and zinc-air batteries. Batter Supercaps 2, 336–347. doi:10.1002/batt.201800082
Rahman, M. A., Wang, X., and Wen, C. (2013). High energy density metal-air batteries: A review. J. Electrochem Soc. 160 (10), A1759–A1771. doi:10.1149/2.062310jes
Salado, M., and Lizundia, E. (2022). Advances, challenges and environmental impacts in metal-air battery electrolytes. Mater Today Energy 28, 101064. doi:10.1016/j.mtener.2022.101064
Savunthari, K., Savunthari, V., Chen, C. H., Chen, Y. R., Tong, Z., Iputera, K., et al. (2021). Effective Ru/CNT cathode for rechargeable solid-state Li–CO2 batteries. ACS Appl. Mater. Interfaces 13, 44266–44273. doi:10.1021/acsami.1c11000
Takechi, K., Shiga, T., and Asaoka, T. (2011). A Li-O2/CO2 battery. Chem. Commun. 47, 3463–3465. doi:10.1039/c0cc05176d
Thapa, A. K., and Ishihara, T. (2011). Mesoporous α-MnO2/Pd catalyst air electrode for rechargeable lithium–air battery. J. Power Sources 196 (16), 7016–7020. doi:10.1016/j.jpowsour.2010.09.112
Thapa, A. K., Saimen, K., and Ishihara, T. (2010). Pd/MnO2 air electrode catalyst for rechargeable lithium/air battery. Electrochem Solid-State Lett. 13, A165. doi:10.1149/1.3481762
Wang, J., Liu, J., Cai, Y., Cheng, F., Niu, Z., and Chen, J. (2018). Super P carbon modified lithium anode for high-performance Li-O2 batteries. ChemElectroChem 5, 1702–1707. doi:10.1002/celc.201800289
Wang, L., Lu, Y., Xie, M., Zhao, S., Li, Z., and Liu, Q. (2023). Interfacially engineered induced nickel-based heterostructures as efficient catalysts for Li-O2 batteries. Electrochim. Acta 437, 141476. doi:10.1016/j.electacta.2022.141476
Wei, W., Wang, Q., Li, J., Liu, D., Niu, J., and Liu, P. (2021). Clusters of ultra-fine tin dioxide nanoparticles anchored polypyrrole nanotubes as anode for high electrochemical capacity lithium ion batteries. J. Taiwan Inst. Chem. E 129, 135–143. doi:10.1016/j.jtice.2021.09.030
Wu, J., and Yang, H. (2013). Platinum-based oxygen reduction electrocatalysts. Acc. Chem. Res. 46 (8), 1848–1857. doi:10.1021/ar300359w
Wu, X., Niu, B., Zhang, H., Li, Z., Luo, H., Tang, Y., et al. (2023). Enhancing the reaction kinetics and reversibility of Li–O2 batteries by multifunctional polymer additive. Adv. Energy Mater 13 (3), 2203089. doi:10.1002/aenm.202203089
Xu, J. J., Xu, D., Wang, Z. L., Wang, H. G., Zhang, L. L., and Zhang, X. B. (2013). Synthesis of perovskite-based porous La0.75Sr0.25MnO3 nanotubes as a highly efficient electrocatalyst for rechargeable lithium-oxygen batteries. Angew. Chem. Int. Ed. 52 (14), 3979–3982. doi:10.1002/ange.201210057
Yang, J., Zhang, D., Lin, T., Zhang, W., Li, C., and Gao, L. (2022). Effect of quinoline-8-sulfonic acid and CaO as hybrid electrolyte additives on microstructure and property of AA5052 alloy anode for aluminum-air battery. J. Taiwan Inst. Chem. E 131, 104150. doi:10.1016/j.jtice.2021.11.017
Yin, H., Li, D., Chi, Z., Zhang, Q., Liu, X., Ding, L., et al. (2021). Iridium coated Co nanoparticles embedded into highly porous N-doped carbon nanocubes grafted with carbon nanotubes as a catalytic cathode for high-performance Li–O2 batteries. J. Mater Chem. A 9, 17865–17875. doi:10.1039/d1ta04830a
Yin, W., Grimaud, A., Lepoivre, F., Yang, C., and Tarascon, J. M. (2017). Chemical vs electrochemical formation of Li2CO3 as a discharge product in Li–O2/CO2 batteries by controlling the superoxide intermediate. J. Phys. Chem. Lett. 8 (1), 214–222. doi:10.1021/acs.jpclett.6b02610
Zhan, Y., Yu, S. Z., Luo, S. H., Feng, J., and Wang, Q. (2021). Nitrogen-coordinated CoS2@ NC yolk–shell polyhedrons catalysts derived from a metal-organic framework for a highly reversible Li-O2 battery. ACS Appl. Mater Interfaces 13 (15), 17658–17667. doi:10.1021/acsami.1c02564
Zhang, J., Zhao, Y., Zhao, X., Liu, Z., and Chen, W. (2014). Porous perovskite LaNiO3 nanocubes as cathode catalysts for Li-O2 batteries with low charge potential. Sci. Rep. 4, 6005. doi:10.1038/srep06005
Zhang, X., Mu, X., Yang, S., Wang, P., Guo, S., Han, M., et al. (2018). Research progress for the development of Li-air batteries: Addressing parasitic reactions arising from air composition. Energy Environ. Mater 12, 61–74. doi:10.1002/eem2.12008
Zhao, Z., Huang, J., and Peng, Z. (2018). Achilles’ Heel of lithium–air batteries: Lithium carbonate. Angew. Chem. Int. Ed. 57 (15), 3874–3886. doi:10.1002/anie.201710156
Zhu, X., Schülli, T. U., Yang, X., Lin, T., Hu, Y., Chen, N., et al. (2022). Epitaxial growth of an atom-thin layer on a LiNi0.5Mn1.5O4 cathode for stable Li-ion battery cycling. Nat. Commun. 13, 1565. doi:10.1038/s41467-022-28963-9
Keywords: Li-O2 battery, perovskite catalysts, battery capacity enhancement, carbon black, Li2O2 and Li2CO3
Citation: Hsu B-Z, Lai J-K and Lee Y-H (2023) La-based perovskites for capacity enhancement of Li–O2 batteries. Front. Chem. 11:1264593. doi: 10.3389/fchem.2023.1264593
Received: 21 July 2023; Accepted: 23 August 2023;
Published: 01 September 2023.
Edited by:
Da-Hua Wei, National Taipei University of Technology, TaiwanReviewed by:
Chia-Yun Chen, National Cheng Kung University, TaiwanCopyright © 2023 Hsu, Lai and Lee. This is an open-access article distributed under the terms of the Creative Commons Attribution License (CC BY). The use, distribution or reproduction in other forums is permitted, provided the original author(s) and the copyright owner(s) are credited and that the original publication in this journal is cited, in accordance with accepted academic practice. No use, distribution or reproduction is permitted which does not comply with these terms.
*Correspondence: Yi-Hsuan Lee, eWhsZWVAbWFpbC5udHV0LmVkdS50dw==
Disclaimer: All claims expressed in this article are solely those of the authors and do not necessarily represent those of their affiliated organizations, or those of the publisher, the editors and the reviewers. Any product that may be evaluated in this article or claim that may be made by its manufacturer is not guaranteed or endorsed by the publisher.
Research integrity at Frontiers
Learn more about the work of our research integrity team to safeguard the quality of each article we publish.