- 1Inorganic Chemistry Department, Taras Shevchenko National University of Kyiv, Kyiv, Ukraine
- 2Faculty of Chemistry, University of Wroclaw, Wroclaw, Poland
- 3Physics Department, CICECO-Aveiro Institute of Materials, University of Aveiro, Aveiro, Portugal
- 4Departamento de Química Fundamental, Universidade Federal de Pernambuco, Recife, Brazil
Materials based on Eu3+ and Tb3+ coordination compounds are of great interest due to their strong red and green luminescence. Appropriate selection of ligands plays a huge role in optimizing their photophysical properties. Another very helpful instrument for such optimization is theoretical modelling, which permits the prediction of the emissive properties of materials through intramolecular energy transfer analysis. The ligands that allow for achieving high efficiency of Eu3+ and Tb3+ emissions include carbacylamidophosphates (CAPh, HL). In this brief review, we summarize recent research for lanthanides CAPh-based coordination compounds of general formulas Cat[LnL]4, [LnL3Q] and [Ln(HL)3(NO3)3], where Cat+ = Cs+, NEt4+, PPh4+ and Q = 1,10-phenanthroline, 2,2-bipyridine or triphenylphosphine oxide, involving the use of thermal gravimetric analysis, X-ray analysis, and absorption and luminescence spectroscopy. We carried out a comparison with selected Ln3+ β-diketonates. Possibilities and developments of theoretical calculations on energy transfer rates are also presented.
1 Introduction
During recent years, coordination compounds of lanthanides have been the focus of attention of many research groups around the world because of their practical application in modern technologies. Special attention is paid to research aimed at the search for luminescent materials. From this point of view, lanthanide coordination compounds attract attention primarily due to the specificity of the luminescence mechanism caused by f-f transitions, which allows for obtaining monochromatic radiation, which is uncharacteristic for phosphors of a purely organic nature. The luminescence of triply charged lanthanide ions (Ln3+) is the subject of research in many scientific fields from laser physics to molecular biology, which is due to interest not only from a fundamental point of view but also from the possibility of practical application in light generators, sensors, optical amplifiers, lasers, flat displays, fluorescent lighting, and medical diagnostics (Bünzli, 2017).
The problem of the low absorption capacity of 4f-4f transitions of Ln3+ (Bünzli, 2015) in coordination compounds is solved by the creation and selection of organic ligands capable of sensitizing the emission of lanthanides by transferring the energy absorbed by them to the metal, as well as by partially removing the parity ban on transitions inside the f-shell. Therefore, the search for new ligands, lanthanide emission sensitizers, and the synthesis and research of compounds based on them is a current task of modern coordination chemistry.
Chemists are constructing a great variety of new molecules that can be combined with lanthanide ions to give efficient lanthanide luminescence. A significant number of successful studies of luminescent lanthanides coordination compounds has been found among coordination compounds based on β-diketonate ligands. These compounds are the most popular and the most intensively investigated rare-earth coordination compounds partially because many different β-diketones are commercially available and the fact that the synthesis of the corresponding rare-earth coordination compounds is relatively easy. Other new promising ligands to design highly luminescent materials for practical use are carbacylamidophosphates (CAPhs), which can be considered as P,N-substituted analogues of β-diketones containing a chelating core C(O)N(H)P(O) (Amirkhanov et al., 2014). The phosphoryl group, present in these ligands, possess high affinity to lanthanides. Moreover, if compared with well-known β-diketones antennas, CAPhs contain up to three highly absorbing groups, and the high energy vibrations in the chelating fragment of CAPhs are reduced by replacing C with N and one C=O group with P=O.
In recent years, the field of lanthanide-based materials has significantly advanced with the introduction of various theoretical approaches and methods (Kushida, 1973; Malta et al., 1998; Maron and Eisenstein, 2001; Malta, 2008; Dutra et al., 2014; Wen et al., 2014; Tanner et al., 2018; Georgieva et al., 2020; Suta and Meijerink, 2020; Carneiro Neto et al., 2021; Moura et al., 2021a; Carneiro Neto et al., 2022; Moura et al., 2022a; Salerno et al., 2022). These procedures have proven successful in predicting and explaining the photophysical properties of these materials. For instance, the combination of density functional theory (DFT), intramolecular energy transfer (IET) theory, and rate equation modelling has enabled researchers to calculate the quantum yield, which is the ratio between absorbed and emitted photons (Kasprzycka et al., 2020; Carneiro Neto et al., 2022; Moura et al., 2022b; Moura et al., 2022c). These theoretical advancements have allowed for the design of lanthanide-based materials with tailored photophysical properties suitable for a wide range of applications, such as optical sensing (Almeida et al., 2022; Ramalho et al., 2022), optical thermometry (Lyubov et al., 2022; Salerno et al., 2022), bioimaging (Piñol et al., 2020; Yao et al., 2023), and light-emitting devices (Kido et al., 1993; Hernández-Rodríguez et al., 2023).
This review is about recent investigations on the luminescence of carbacylamidophosphates (CAPh) based on Eu3+ and Tb3+ coordination compounds. Herein we will focus on the Ln3+ coordination compounds based on phenyl-containing CAPhs, and on the features of their luminescence compared to Eu3+ and Tb3+ β-diketonates.
2 A brief history and main concepts of lanthanide photophysics
The unusually sharp absorption lines of rare earth compounds were first observed by Becquerel in 1906 when he measured the spectrum of the mineral xenotime (YPO4 containing traces of Er, Ce, and Th) (Becquerel, 1906). Around 1930, it was suggested that those lines could be due to electronic transitions within the Ln3+ 4f configuration - f-f transitions (Becquerel, 1929; Bethe, 1930). Thus, the key to understanding the spectral properties of lanthanides is their specific electronic configuration: the ground state of Ln3+ ions [Xe]4fn (n = 0–14) is energetically well separated from the [Xe]4fn−15d1 configuration (∆Е >32,000 cm−1). In addition, there is the shielding of the 4f-orbitals by the “xenon core” (54 electrons), in particular, by the 5s2 5p6 sublevels, which makes the valence 4f-orbitals “internal”. Therefore, electrons in the 4f shell do not play a role in the chemical bonding between the lanthanide ions and the ligands. As a consequence, the influence of the lanthanide coordination environment on the optical transitions within the 4f shell is small, resulting in sharp-line spectra.
If these transitions are indeed intraconfigurational, the question remained as to how these transitions acquire their strength: formally they are forbidden by the Laporte (or parity) selection rule. In 1937, van Vleck addressed this puzzle and showed that they become partially allowed as electronic dipole transitions by an admixture of configurations of opposite parity, such as the 4fn−15d1 configuration (Van Vleck, 1937). Some 4f-4f transitions are allowed as magnetic dipole transitions, and both schemes yield oscillator strengths of the same order of magnitude.
Dieke’s diagram (Figure 1) provides a comprehensive overview of the energy levels of the lanthanide series (Carnall et al., 1968a; Carnall et al., 1968b; Carnall et al., 1968c; Carnall et al., 1968d; Dieke, 1968; Carnall et al., 1979). It has been demonstrated that the position of Ln3+ energy levels is relatively insensitive to the chemical environment (Carnall et al., 1989; Peijzel et al., 2005). Therefore, this diagram can be applied to describe the energy levels of lanthanide ions in different compounds. However, changes in the intensity and width of spectral lines may occur depending on the local environment of the lanthanide ions (Judd, 1962; Ofelt, 1962; Mason et al., 1974; Judd, 1979; Malta, 1982; Moura et al., 2016). This highlights the importance of the local environment’s effects on the lanthanide ions, even though the energy level remains unchanged.
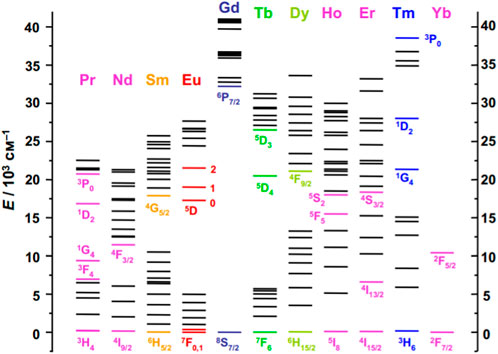
FIGURE 1. Energy level diagram for some Ln3+ ions (Dieke, 1968).
Most lanthanide ions are known to exhibit luminescent properties, but their intensity varies depending on the type of Ln3+. It is important to note that La3+ lacks 4f-electrons while Lu3+ has a filled 4f-shell, meaning that these ions do not exhibit 4f luminescence. However, the remaining lanthanides cover the entire spectral range from ultraviolet (Gd3+), visible light (Sm3+ - orange; Eu3+, Er3+ - red; Tb3+, Er3+ - green; Dy3+ - yellow; Tm3+ - blue) and near-infrared (Pr3+, Nd3+, Ho3+, Er3+, and Yb3+).
In 1942, it was discovered that certain organic ligands can enhance the luminescence of lanthanides through sensitization (Weissman, 1942; Yuster and Weissman, 1949). In the 1960s, systematic studies on luminescent lanthanide coordination compounds and their photophysics began to emerge (Crosby and Kasha, 1958; Crosby et al., 1961; Crosby et al., 1962; Bauer et al., 1964; Bhaumik, 1964; Melby et al., 1964; Bhaumik and El-Sayed, 1965). These studies mainly focused on Eu3+ and Tb3+ β-diketonates, and many of the photophysical concepts discovered at that time are still used today. Lanthanide β-diketonates remain among the most studied luminescent compounds due to their commercial availability, easy synthesis of the coordination compounds, and excellent luminescent properties.
In 1997, Malta introduced the formalism of the IET theory (Malta, 1997). This theory allowed for the calculation of energy transfer rates from organic excited states (e.g., S1 and T1) to the 4f excited states of Ln3+ using multipolar mechanisms such as dipole-dipole and dipole-quadrupole interactions. This was a significant advancement in the understanding of energy transfer mechanisms involving lanthanide ions. Later on, Gonçalves e Silva and Malta added an expression for the exchange mechanism (e Silva and Malta, 1997; Malta and Gonçalves e Silva, 1998), which further expanded the understanding of the energy transfer process. The addition of the exchange mechanism allowed for a more comprehensive understanding of the complex IET process involved in lanthanide-based compounds.
These works were particularly important because they filled a gap of 55 years in the quantitative approach to the IET theory since Weissman’s work in 1942 (Weissman, 1942). With these new developments, researchers had a more complete understanding of energy transfer mechanisms and could more accurately predict the rates of energy transfer between organic excited states and the 4f excited states of lanthanide ions. In recent years, modern computational programs such as LUMPAC (Dutra et al., 2014) and JOYSpectra (Moura et al., 2021a) have been developed and implemented with the IET equations to aid the lanthanide spectroscopy community in understanding the underlying photophysical processes of lanthanide-based compounds. These programs have greatly benefited researchers in predicting and understanding the complex energy transfer mechanisms that occur in lanthanide photophysics.
3 Sensitization, deactivation of lanthanide luminescence, and strategies to enhance the efficiency of Ln3+ luminescence in coordination compounds
Lanthanide ions have small 4f-4f absorption coefficients (Bünzli, 2015), making them difficult to excite directly. As a result, an indirect excitation approach known as sensitization or the “antenna effect” is commonly used in practical applications. In coordination compounds, a ligand is used as a sensitizer for lanthanide ion luminescence and also serves to protect the Ln3+ from solvent or other molecules, thus preventing nonradiative relaxations. As previously mentioned, the sensitization of lanthanides in coordination compounds was observed and proposed for the first time by Weissman (Yuster and Weissman, 1949), who suggested that a photon can be absorbed by an organic ligand with high absorption cross-section and transferred by the IET to the resonant levels of the Ln3+. Crosby and colleagues published the complete mechanism, introducing the role of the ligand triplet state in the IET process (Crosby et al., 1961; Crosby et al., 1962). Figure 2 shows a simplified Jablonski energy level scheme of the IET sensitization process.
The sensitization of the Ln3+ emission (Em.) occurs through several stages, including absorption of light by the ligand (Abs.), intersystem crossing (ISC), and intramolecular energy transfer (ligand-to-Ln3+). Additionally, direct energy transfer from the ligand’s excited singlet state S1 to the lanthanide can occur (Kasprzycka et al., 2017; Aquino et al., 2021; Moura et al., 2021b; Tanner et al., 2022). The excitation energy can be partially lost due to fluorescence emission (S1→S0), phosphorescence emission (T1→S0), and non-radiative relaxations in the middle of the sensitization process. Therefore, the efficiency of sensitization (ηsens) and the Ln3+ emission greatly affect the overall emission quantum yield (
The influence of the first component (ηsens) is regulated most often by selecting ligands with the optimal value of the singlet and triplet levels positions. The latter can be determined by the low-temperature phosphorescence spectra of La3+, Gd3+, and Lu3+ coordination compounds (Brito et al., 2010; Carneiro Neto et al., 2019). The optimal ranges of the energy gap between the ligand’s lowest triplet state and the emissive level of the lanthanide (ΔЕ) have been estimated as 2,500–3,500 cm−1 for an efficient ligand-to-metal energy transfer (Bünzli et al., 2010). When the energy gap is less than ∼1900 cm−1, the backward IET may take place (Sato and Wada, 1970), leading to a decrease in luminescence efficiency. On the other hand, if ΔЕ is too high, it increases the probability of non-radiative decay, making it difficult to efficiently transfer energy to the Ln3+. Although this rule is generally used in the design of efficient lanthanide-based phosphors, it does not always work in practice due to the more complicated nature of the IET process. Factors such as the influence of charge transfer states (Gérard et al., 1994; Petoud et al., 1999; Faustino et al., 2005; Kasprzycka et al., 2020; Kasprzycka et al., 2022), participation of several IET pathways between the ligand and the Ln3+ ion (Moura et al., 2021a; Moura et al., 2021b), donor-acceptor distances (Carneiro Neto et al., 2019; Kasprzycka et al., 2020; Moura et al., 2022c), and temperature (Carneiro Neto et al., 2021; Salerno et al., 2022) can also have an impact on the photophysical process.
The intrinsic quantum yield
Malta’s theory is used to describe the IET mechanisms between the donor (ligand) and acceptor (Ln3+) in the presence of a perturbation. This theory builds upon Fermi’s golden rule, which describes for a system the probability of making a transition from an initial state to a final state due to a perturbation. In this framework, three interactions or mechanisms are introduced: the dipole-dipole (
where,
Equations 2–4 provide key factors for understanding the interaction between the donor and acceptor in an energy transfer process.
Equations 7, 8 describe the “spin strengths” of the exchange interaction between the donor and acceptor molecules.
In these equations there are several important quantities to consider. These include
Last but not least, the
where the
To predict the population of each level (Figure 2) after estimating the IET rates theoretically, a rate equations model can be employed (Grant, 1971). This model consists of a set of ordinary differential equations which take into account all the rates (including decay and IET rates) involving different levels. Each level is described using the general form:
where
There are two approaches for solving the system of equations: analytical and numerical. The analytical method assumes that the system is in a steady-state regime (Carneiro Neto et al., 2019; Kasprzycka et al., 2020), which means that the derivatives of the populations are equal to zero. On the other hand, the numerical approach solves and propagates the equations through time. In this case, it is important to ensure that the system reaches the steady-state regime by the end of the numerical simulation (Carneiro Neto et al., 2019; Carneiro Neto et al., 2021). By solving these equations, we can obtain a quantitative understanding of the population of each energy level and the kinetics of the energy transfer process.
4 Synthesis, structural features, and thermal stability of coordination compounds Cat[LnL4], [LnL3Q], and [Ln(HL2)3(NO3)3] based on phenyl-containing carbacylamidophosphates
Carbacylamidophosphates (CAPhs) have been known since the 1960s when A. Kirsanov with his research group presented the so-called phosphazo reaction (Kirsanov and Makitra, 1956). Since the 1990s, these compounds have been used as ligands for different metal ions. And nowadays, the Cambridge Structural Database contains over two thousand structures with the –C(O)N(H)P(O)= system.
Herein we will limit ourselves to three representatives of CAPhs (Figure 3) with phenyl substitutes in different positions, which have been intensively studied recently as Eu3+ and Tb3+ luminescence sensitizers. The most typical CAPh based coordination compound types, Cat[LnL4], [LnL3Q], and [Ln(HL2)3(NO3)3] will be discussed (Figure 4).
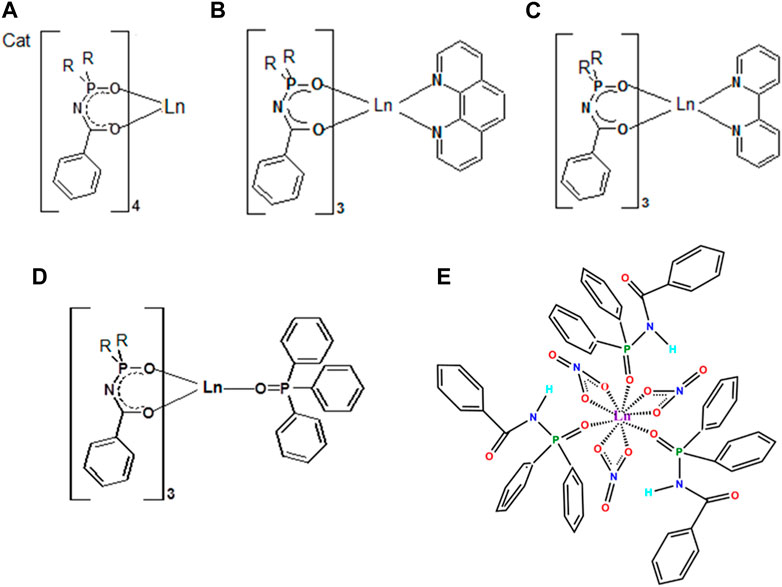
FIGURE 4. The structural formula of typical Ln3+ coordination compounds based on phenyl-containing CAPhs: Cat[LnL4] (A), [LnL3Q] (B-D) and [Ln(HL2)3(NO3)3] (E).
Generally, the two types of Ln3+ coordination compounds may be obtained, based on β-diketones or CAPhs, depending on the ratio of metal ions to the ligands anions in the product: ternary compounds with a ratio Ln:Ligand = 1:3 or tetrakis-compounds with a ratio Ln:Ligand = 1:4. Additionally, some CAPhs, being in neutral form, can bind metals and create ternary coordination compounds of general formula [Ln(HL)3A3] (where A is a nitrate or chloride anion) (Amirkhanov et al., 2014).
4.1 Tetrakis-coordination compounds Cat[LnL4]
Coordination compounds of general formula Cat[LnL4], where L = β-diketonate or CAPh and where the cationic counterparts are typically alkali metal ions or positively charged N, P-organic cation, have been extensively studied recently (see (Kariaka et al., 2022) and references therein). In the structure of tetrakis-compounds, a lanthanide ion is surrounded by four deprotonated ligands forming an LnO8 environment. Such compounds are formed by the self-assembly principle—the cationic counterparts should coincide by size and/or create short contact with the ligand to form the coordination compound of the mentioned type. By using such cations as sodium, caesium, tetraethylammonium, and tetraphenyl phosphonium, the following coordination compounds have been obtained based on HL1-3 ligands: Cs[LnL1]4, NEt4[LnL1]4, NEt4[LnL3]4, and PPh4[LnL3]4 (Kariaka et al., 2016a; Kariaka et al., 2017; Kariaka et al., 2018; Kariaka et al., 2022).
To obtain NEt4[LnL1]4, NEt4[LnL3]4, and PPh4[LnL3]4 the following synthetic route can be applied: a solution of LnCl3·nH2O (1 mmol) in 2-propanol, previously boiled with a dehydrating agent HC(OEt)3, is mixed with the solution of NaL (4 mmol) in acetone. Then the CatCl (1 mmol) solution in 5 mL of acetone is added. The resulting mixture is heated to boiling point and then cooled to room temperature. After filtering out the precipitated NaCl, the remaining liquid is left to slowly evaporate. In a day, well-faceted crystals of the target compounds appear. The crystals are filtered off, washed with cold 2-propanol, and dried in air. The crystals of NEt4[LnL1]4 coordination compounds can be obtained with a yield of around ∼70% for lanthanides with bigger radii (La–Dy) and ∼50% for Ho–Yb (Kariaka et al., 2022). These compounds are well soluble in DMSO, alcohols, acetone, acetonitrile, and dichloromethane, and under heating soluble in benzene, and insoluble in water. The melting points of the coordination compounds are in the range of 112–143°С. The NEt4[LnL3]4 and PPh4[LnL3]4 can be obtained with a yield of ∼80%. NEt4[LnL3]4 compounds are well soluble in DMSO, methanol, acetone, acetonitrile, dichloromethane, not very soluble in benzene and 2-propanol, and insoluble in water. The melting points of these compounds are in the range of 110–135°С. The PPh4[LnL3]4 compounds are soluble in DMSO, methanol, acetone, and CH2Cl2, and insoluble in nonpolar organic solvents and water. The melting points are in the range of 220–265°С.
To obtain coordination compounds Cs[LnL1]4 the following synthetic route can be applied: a solution of LnCl3·nH2O (1 mmol) in 2-propanol, previously boiled with a dehydrating agent HC(OEt)3, is mixed with a solution of NaL (3 mmol) in acetone. Then CsL (1 mmol) solution in 5 mL of acetone is added. The resulting mixture is heated to reflux and then cooled to room temperature. The NaCl that has precipitated is filtered out, and the resulting liquid is left in a vacuum desiccator over CaCl2 to dry out. The next day the obtained crystals of the compounds are filtered off, washed with cold 2-propanol, and dried in air. Yield: ∼70% for lanthanides with bigger radii (La–Dy) and ∼50% for Ho–Yb tetrakis-compounds. The coordination compounds are soluble in DMSO, alcohols, and acetone, and insoluble in non-polar organic solvents and H2O. The melting points of the compounds are in the range of 210–240°С.
Ligand HL3 does not form crystals of tetrakis-coordination compounds with an alkali ion in its outer-sphere, and HL1 does not form tetrakis-coordination compounds with the sodium cation, apparently due to steric complications during contact of oxygen atoms of phosphoryl groups with those metal cations. HL2 does not form tetrakis-compounds at all, presumably due to the too-close location of bulky phenyls to the chelating fragment.
The thermal decomposition of the phenyl containing CAPhs based Eu3+ tetrakis-compounds starts over 200°С, and after heating them to 600°С results in a weight loss of 51%—73%. The obtained residue is usually a mixture of europium phosphates. The total weight loss is less than for lanthanide β-diketonates, for which the total weight loss in this temperature range is usually over 80% (Bruno et al., 2009; Stilinović and Kaitner, 2009; Yi et al., 2012; Adati et al., 2019; Borges et al., 2019), due to their high volatility or formation of lanthanide oxides as residues. Tetrakis-compounds Cs[EuL1]4 and PPh4[EuL3]4 melt at a temperature of 240°С and decompose immediately after melting. The coordination compounds with tetraethylammonium cation have almost the same temperatures of decomposition; however, they have significantly lower melting points. Thus NEt4[EuL1]4 and NEt4[EuL3]4 exist in a liquid state in the temperature ranges 139–200 and 119–250°С, respectively.
The typical polyhedra of Ln3+ ions in the CAPh-based tetrakis-compounds are distorted square antiprism (D4d) and triangular dodecahedron (D2d). These types of polyhedra are also typical for Ln3+ tetrakis-β-diketonates (Bruno et al., 2009; Lunstroot et al., 2009; Stilinović and Kaitner, 2009). In the tetrakis-structure, the outer-sphere cations can influence the Ln3+ ions polyhedral geometry, due to forming short contacts with the CAPh ligands. Specifically, they interact with the phosphoryl and ester oxygen atoms of the CAPh ligands.
4.2 Ternary mixed ligand coordination compounds [LnL3Q]
In β-diketones or CAPhs-based Ln3+ ternary coordination compounds, the lanthanide ion coordination sphere cannot be saturated by three of these ligands. As a result, the ternary compounds contain solvents coordinated with the lanthanides. To remove the solvents and to improve the luminescence properties of the coordination compounds, ancillary ligands, such as 2,2-bipyridine (bipy), 1,10-phenanthroline (phen), triphenylphosphine oxide (TPPO), etc., are used.
The [LnL3Q] coordination compounds with CAPhs can be obtained according to the following synthetic route: a solution of Ln (NO3)3·nH2O (1 mmol) in 2-propanol, previously boiled with a dehydrating agent HC(OEt)3, is mixed with the solution of NaL (3 mmol) in acetone. Then 1 mmol of solid bipy or phen or TPPO is added to the mixture. The resulting mixture is boiled for some minutes to complete the dissolution of the ancillary ligand and then cooled down to room temperature. After 15 min, the precipitated NaNO3 is filtered off. The resulting clear solution is left to stand at ambient temperature for slow evaporation of the solvents. In a day, the coordination compounds precipitate as powders or crystals. The target precipitates are filtered off, washed with cold 2-propanol, and finally dried in air.
In the [LnL3bipy] and [LnL3phen], a lanthanide ion is bonded by six oxygen atoms of CAPhs and two nitrogen atoms of the ancillary ligand, forming an LnO6N2 environment. The typical polyhedra of lanthanide ions in ternary mixed ligand coordination compounds LnL3bipy and LnL3phen with phenyl-containing CAPhs are distorted square antiprisms (D4d) and triangular dodecahedra (D2d). In the LnL13TPPO, the central ion is surrounded by seven oxygen atoms, and the lanthanide ion polyhedron is interpreted as a distorted pentagonal bipyramid (D5h) (Kuzmina et al., 2020). As the CAPh ligands are bulkier compared with β-diketones, the mixed ligand compounds with CAPhs contain one TPPO ligand, while for β-diketones-based ones, both types [LnL3TPPO] and [LnL3 (TPPO)2] can be obtained (Nakajima et al., 2016; Zhao et al., 2018).
The mixed ligand coordination compounds are thermally stable up to 200°С and even beyond (Table 1). The LnL13TPPO has the lowest decomposition temperature (200°С). The highest decomposition temperature (280°С) is a characteristic of [EuL23bipy] and [EuL33phen] coordination compounds. The total compounds’ weight loss in the temperature range of 20–800°С does not exceed 70%, being smaller compared to mixed ligand β-diketonates. None of the mixed ligand compounds decompose immediately after melting and exist in fusion. The compound EuL33phen has the lowest melting point (82°С) and the biggest temperature interval of the coordination compound existence in the liquid state (almost 200°С). The melting points and temperature of decomposition do not vary significantly depending on the lanthanide ion. No tendency in melting points and temperature of decomposition exists depending on ancillary ligand type in the [LnL3bipy] and [LnL3phen] coordination compounds. Thus, [LnL13bipy] and [LnL13phen] have similar melting and decomposition temperatures, the compounds [LnL23bipy] and [LnL23phen] differ in their melting points, while [EuL33bipy] and [EuL33phen] differ significantly in both their melting points and temperatures of decomposition.
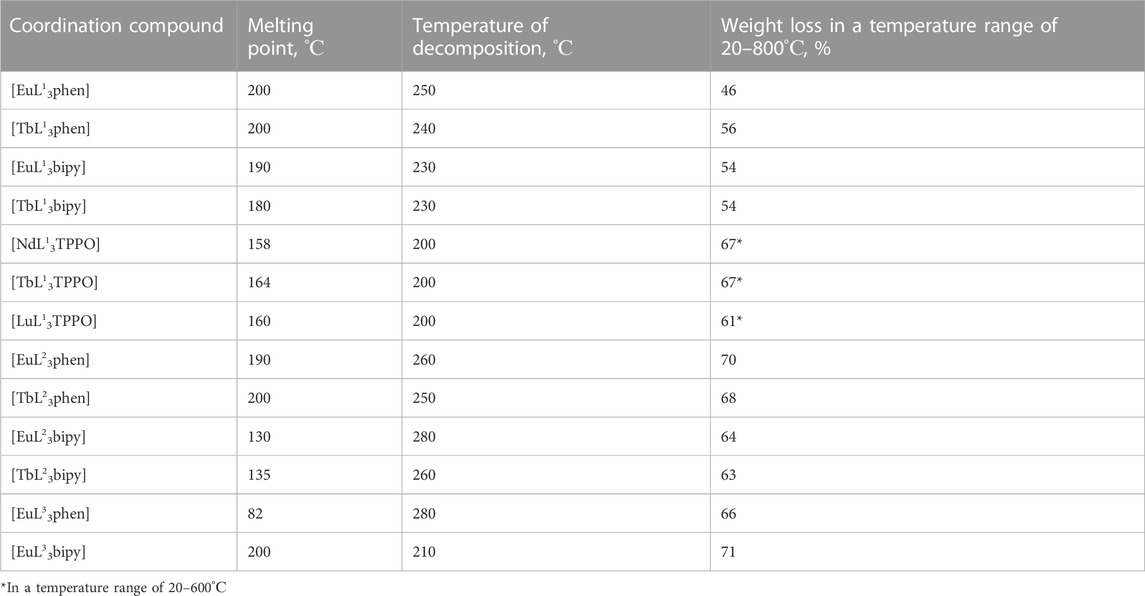
TABLE 1. Melting points, the temperatures of decomposition, and weight losses of ternary coordination compounds.
4.3 Coordination compounds [Ln(HL2)3(NO3)3]
Compounds [Ln(HL2)3(NO3)3] can be obtained as follows: a solution of Ln(NO3)3·nH2O (1 mmol) in acetone is mixed with the solution of HL2 (3 mmol) in acetone. The solvent is completely evaporated from the resulting mixture. Then hexane is added to the obtained oily residue, and this mixture is mixed for 2 hours to obtain a solid precipitate. The obtained precipitate of the target compounds is filtered off, washed with hexane, and dried in air. The coordination compounds are well soluble in acetone, CH2Cl2, and methanol, slightly soluble in 2-propanol and benzene, and insoluble in hexane and water. The melting points of the compounds are in the range of 122–140°С. By spectral studies and elemental analysis, it was established that the CAPh ligands are coordinated to lanthanides in a monodentate manner via the oxygen atom of the phosphoryl group, while the nitrate anions are coordinated in a bidentate manner (Legendziewicz et al., 2000). Thus, the lanthanides have an LnO9 coordination environment. Thermal gravimetric studies have shown that the compound [Eu(HL2)3(NO3)3] starts to decompose at 140°С and the total weight loss in the temperature range 20–800°С equals 71%.
5 Absorption and phosphorescence spectra of phenyl-containing CAPh based Ln3+ coordination compounds
In the UV region, the absorption spectra of the synthesized mixed ligand coordination compounds (Figure 5) are characterized by the presence of the two absorption bands with maxima at 235 and 265 nm for the compounds with phen, 238 and 275–280 nm for the compounds with bipy, and 240 and 265 nm for [LnL13TPPO].
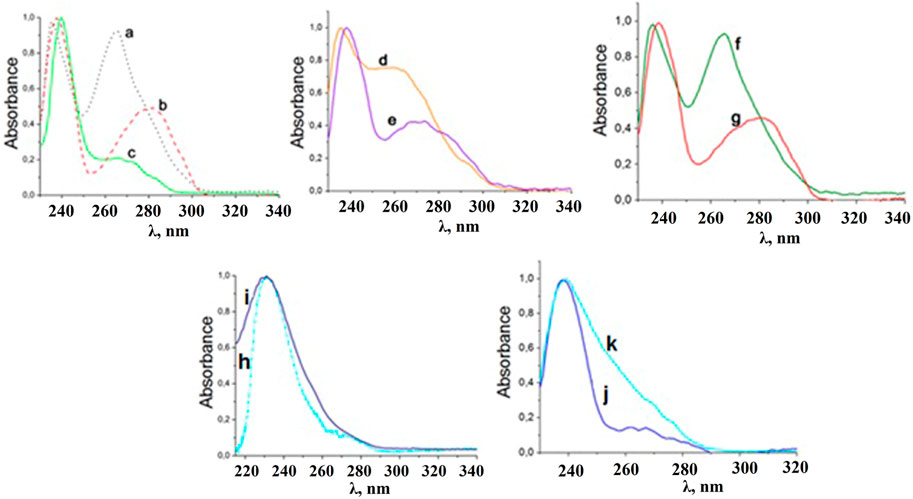
FIGURE 5. Normalized absorption spectra of [LnL13Phen] (a), [LnL13bipy] (b), [LnL13TPPO] (c), [LnL23Phen] (d), [LnL23bipy] (e), [LnL33Phen] (f), [LnL33bipy] (g), solutions in CH2Cl2, Cs[LnL14] (h), and NEt4[LnL14] (i), solutions in CH3CN and NEt4[LnL34] (j), PPh4[LnL34] (k) solutions in CH2Cl2 at 298 K.
The absorption spectra of the obtained tetrakis-coordination compounds contain one intense band with a maximum of 236–238 nm and a shoulder at 280 nm (Figure 5). The absorption bands in the spectra appear due to π→π* transitions of the ligands.
Considering the lowest energy edge of the absorption spectra of the coordination compounds, it can be assumed that the CAPhs lowest excited singlet state is located near 35 500 cm−1, the lowest excited singlet state of bipy and phen is near 33 100 cm−1, and for the TPPO ligand it is near 34 500 cm−1.
The lowest ligands triplet states positions Е(T1) in the CAPh-based compounds, estimated from the phosphorescence spectra of La3+ or Gd3+ compounds using the zero-phonon line, as well as for lanthanide coordination compounds based on phenyl containing β-diketonates, are given in Table 2. It is noteworthy that phenyl-containing CAPhs have higher Е(T1) compared with phenyl-containing β-diketones by nearly 6,000 cm−1. It is also worth noting that in mixed ligand coordination compounds, the Е(T1) is variable depending on the combination of the ligands. For example Е(T1) of phen in CAPh-based compounds [LnL3phen] varies from 21,552 to 22,371 cm−1 depending on the CAPh ligand. In compounds [Ln(bzac)3·2H2O] and [Ln(bzac)3phen], the Е(T1) of bzac ligand differs depending on the ancillary ligand, being equal to 21,550 and 20,202 cm−1, respectively. Comparing Е(T1) in the tetrakis-compounds, it can be concluded that the lowest ligands’ triplet states position also depends slightly on the nature of the cation.
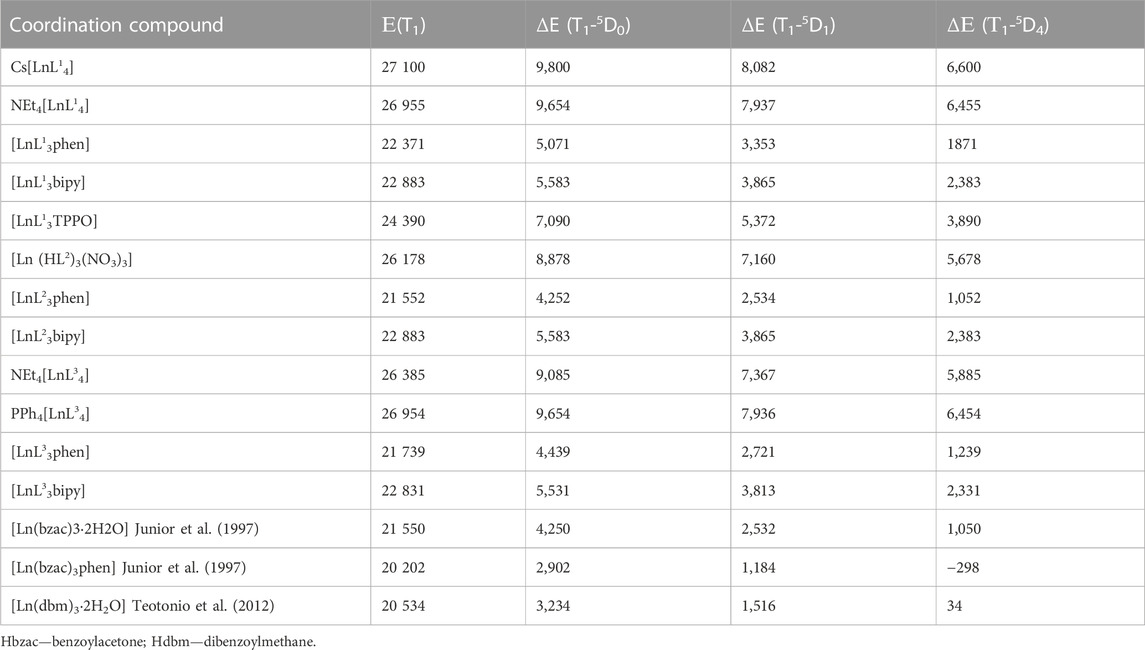
TABLE 2. The lowest ligands triplet state energies Е(T1) and the energy gaps ΔE (in cm−1) between T1 and 5DJ levels.
6 General interpretations of the Eu3+ and Tb3+ β-diketonates photoluminescence
β-diketones were among the first ligands discovered to be suitable sensitizers for the Eu3+ ion (Yuster and Weissman, 1949). These ligands form stable coordination compounds with lanthanide ions, allowing for efficient sensitization due to the small distance between the sensitizer and the ion. Furthermore, energy transfer from ligands to the metal in lanthanide β-diketonates occurs mainly through the triplet state, and the energy transfer from the excited singlet level of the ligand is often less relevant. As a result, many Eu3+ β-diketonate compounds exhibit intense luminescence. However, these ligands are often not the best sensitizers for Tb3+ ions since their triplet state has rather low energy, leading to an effective backward energy transfer from the Tb3+ (Yang et al., 1994a).
In search for effective luminescent lanthanide coordination compounds based on β-diketones, the main approach is to modify the β-diketone substituents. The luminescence properties of lanthanides can also be tuned by selecting suitable ancillary ligands in mixed ligand compounds or changing the outer-sphere cations in tetrakis-compounds. The luminescence of Eu3+ β-diketonate coordination compounds follows certain regularities based on their structure. Typically, tris- (or ternary) coordination compounds exhibit lower emission intensity, while the intensities of tetrakis-compounds are usually higher. Addition of an ancillary ligand to the coordination sphere of ternary coordination compounds generally increases their emission intensity. However, due to a significant energy gap between the resonance level of Eu3+ and the triplet of the ligands, the energy transfer is inefficient in coordination compounds with aliphatic β-diketones, such as acetylacetone (Hacac), trifluoroacetylacetone (Htfac), or hexafluoroacetylacetone (Hhfac), resulting in poor emission. This was discussed in the work by Filipescu and collaborators (Filipescu et al., 1964). Using a combination of both aromatic and aliphatic substituents in the β-diketonate ligands, such as benzoylacetone (Hbzac), benzoyltrifluoroacetone (Hbtfac), and thenoyltrifluoroacetone (Htta), can lead to compounds with more efficient emission than those containing symmetrical ligands like dibenzoylmethane (Hdbm), dithionylmethane (Hdtm), Hacac, or Hhfac. The increase in emission intensity in such systems is attributed to an increase in anisotropy around the Eu3+ ion (Filipescu et al., 1964; Lima et al., 2013).
The quantum yield of coordination compounds with fluorinated ligands is higher than non-fluorinated analogues. Among europium β-diketonates, a well-known coordination compound with good luminescent characteristics is [Eu (tta)3Phen]. S. B. Meshkova and co-authors have studied the photoemission of β-diketonates with perfluoroalkyl chains (Topilova et al., 1997; Meshkova et al., 2000). They have shown that the intensity and quantum yield of lanthanide luminescence, as well as the absorption capacity of the ligands, increase with the increasing number of carbon atoms in the chain. Such dependence was observed when the chain was increased to the size of C6F13 and was explained by the formation of a reliable hydrophobic shell around the metal ions.
Among Tb3+ coordination compounds, tris-compounds with 4,4′-difluorobenzoylmethane (Hfbm), di-4,4′-dimethoxybenzoylmethane (Hdmbm) (Filipescu et al., 1964), and acetylacetone (Yang et al., 1994a) demonstrated intensive emission. In order to obtain luminescent Tb3+ β-diketonates with aromatic substituents, compounds with 1-indoleacetylacetone and 3-indoleacetylacetone (Wu et al., 1992a; Wu et al., 1992b) were synthesized, since the energy of the triplet level of indole is higher than that of the phenyl group.
In the works (Yang et al., 1994a; Yang et al., 1994b), it was also noted that the rigid planar structure of chelating metallocycles contributes to the growth of metal-center emission sensitized by ligands since such a structure improves the energy transfer from the ligand to the metal.
Eu3+ and Tb3+ β-diketonate coordination compounds are usually excited by UV light. However, there is a need to find coordination compounds that can be excited by visible light for their use as luminescent labels. In this sense, several studies have focused on this search (Werts et al., 1999; Jiang et al., 2010; Ma and Wang, 2010; Reddy et al., 2013; Sun et al., 2015). Additionally, some Ln3+ β-diketonate have been reported to have low photostability when exposed to UV irradiation (Pagnot et al., 2000; Gameiro et al., 2001; Nockemann et al., 2005; Lima et al., 2006; Lima et al., 2009; Songzhu et al., 2010; Kai et al., 2011).
Another important indicator of the emission, which determines the practical interest in it, is the luminescence decay time (τexp). Unlike the quantum yield, which reflects emission quenching in the entire ligand-metal system, the luminescence lifetime characterizes non-radiative processes occurring only on the metal ion. Fluoride substituents in β-diketones have a positive effect on the value of the emission lifetime, increasing it almost twice compared to non-fluorinated analogues. The luminescence lifetime of Eu3+ ternary-compounds is shorter than that of tetrakis-compounds at 77 K, but the inverse relationship is observed at room temperature.
The luminescence characteristics of some Eu3+ and Tb3+ β-diketonate are presented in Table 3. As can be seen from the table, most β-diketonates of Eu3+ and Tb3+ show emission with a lifetime of up to a millisecond, and various quantum yields: from fractions of a percent to almost one hundred percent values (70%–85%). But as noted, the value of the quantum yield gives only a partial idea of the luminescence intensity of compounds, because the latter depends not only on the quantum yield but also on the amount of energy absorbed by the coordination compound (Binnemans et al., 2005).
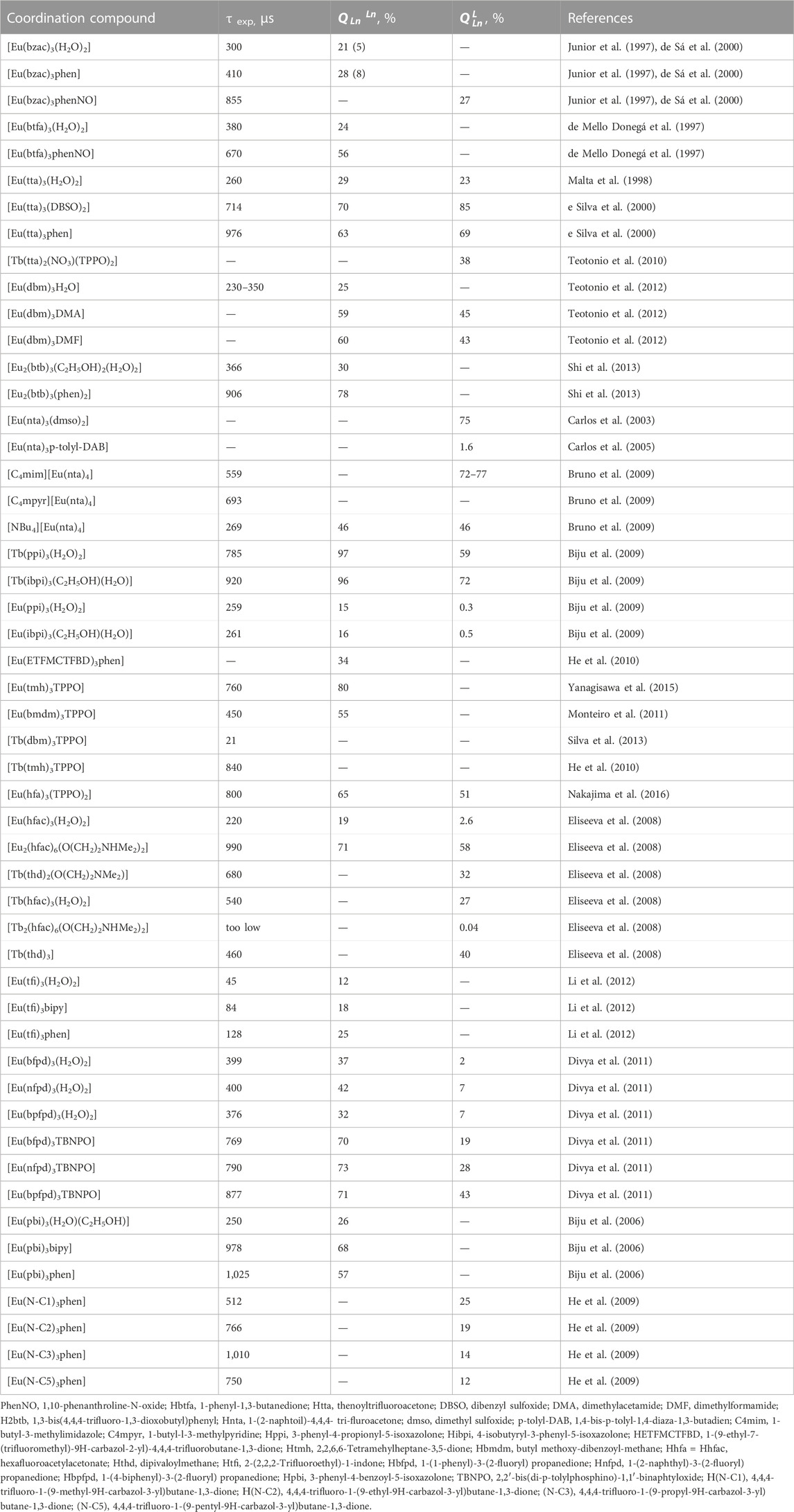
TABLE 3. Luminescence decay lifetime, intrinsic and overall quantum yields of some Eu3+ and Tb3+ β-diketonates.
Not only the emission efficiency of β-diketonates of lanthanides is of interest. In view of applications of the coordination compounds in OLEDs, their luminescent color is an important factor to be considered, which depends on the emission band ratio intensity. In the emission spectra of Eu3+ β-diketonates, the band of the hypersensitive 5D0→ 7F2 transition dominates. The ratio of intensities of hypersensitive and magnetic dipole transitions I (5D0→7F2)/I (5D0→7F1) is in the range of 8–27 (Klink et al., 2000; Binnemans et al., 2005).
From a theoretical perspective, lanthanide-base β-diketonates have been widely studied in the literature (Moura et al., 2016; Carlos et al., 2005; Divya et al., 2011; Petrov et al., 2015; Arrué et al., 2021; Malta et al., 1997; Singh et al., 2017; Santos et al., 2020; dos Santos et al., 2010; Batista et al., 1998; Lima et al., 2020; Fu et al., 2019; Nigoghossian et al., 2022). Some computational studies have helped in understanding the molecular and electronic structures of lanthanide β-diketonates (Fu et al., 2019; Lima et al., 2020; Arrué et al., 2021) as well as in exploring their stability (Petrov et al., 2015). In addition, the influence of the covalency of Ln–O bonds in the so-called Ωλ intensity parameters (sometimes referred to as Judd-Ofelt parameters) was also addressed using density functional theory (DFT) calculations (Moura et al., 2016). These kinds of studies have provided valuable insights into the design and development of new luminescent materials.
7 Photoluminescence of Eu3+ and Tb3+ complexes based on phenyl-containing carbacylamidophospates
In recent decades, coordination compounds based on hetero-substituted analogues of β-diketones, such as carbacylamidophosphates (CAPh) and sulfonylamidophosphates (SAPh), have been actively studied (Gawryszewska and Smolenski, 2014). From the point of view of obtaining luminescent materials, ligands of these types have some advantages over β-diketones. In particular, C=O vibrations (∼1,600 cm−1), which are present in β-diketones, are partially or completely replaced by less energetic P=O vibrations (∼1,250 cm−1) and S=O (∼1,350 cm−1); replacing the carbon atom with nitrogen in the chelating node of the ligands eliminates high-energy C–H oscillations; and the phosphoryl group, which is present in CAPhs and SAPhs, makes it possible to add one more “substitute-antenna”. Unlike many lanthanide β-diketonates, the amidophosphates form thermodynamically stable compounds which are not destroyed by UV, or even synchrotron irradiation (Gawryszewska and Smolenski, 2014).
The luminescence studies of coordination compounds based on CAPh ligands are currently limited. So far, only a few studies have investigated the luminescence properties of Eu3+ and Tb3+ coordination compounds with CAPh ligands. These studies include investigations on the luminescence of compounds with general compositions of Cat[LnL4]·xH2O (Amirkhanov et al., 1996; Sokolnicki et al., 1999; Tang et al., 2014; Kariaka et al., 2016a; Kariaka et al., 2017; Kariaka et al., 2018; Kariaka et al., 2022), [LnCl3(HL)3] and [Ln(NO3)3(HL)3] (Legendziewicz et al., 2000; Legendziewicz et al., 2001; Znovyak et al., 2009; Kariaka et al., 2016b), [LnL3Q] (Q = H2O, 2-propanol, 2,2-bipyridine, 1,10-phenanthroline, TPPO, 2-(1,3,4-oxadiazole-2-yl) pyridine) (Legendziewicz et al., 2001; Borzechowska et al., 2002; Oczko et al., 2003; Puchalska et al., 2008; Znovyak et al., 2009; Kariaka et al., 2014; Tang et al., 2014; Litsis et al., 2015; Kariaka et al., 2016b; Kariaka et al., 2016c; Nakajima et al., 2016; Yakovlev et al., 2018; Kariaka et al., 2020), [Na2LnL4(OTf)(DMF)] (Pham et al., 2020), Ln5L10(OH)5 (Kariaka et al., 2016d), binuclear Ln2L3phen2 (Horniichuk et al., 2021), and [Eu2(L)2(μ-Ph2POO)2(κ-OP(O)Ph2)2(CH3OH)2] (Pham et al., 2017). Some of these studies have also measured the luminescence decay lifetimes and emission quantum yields (overall and intrinsic), as shown in Table 4.
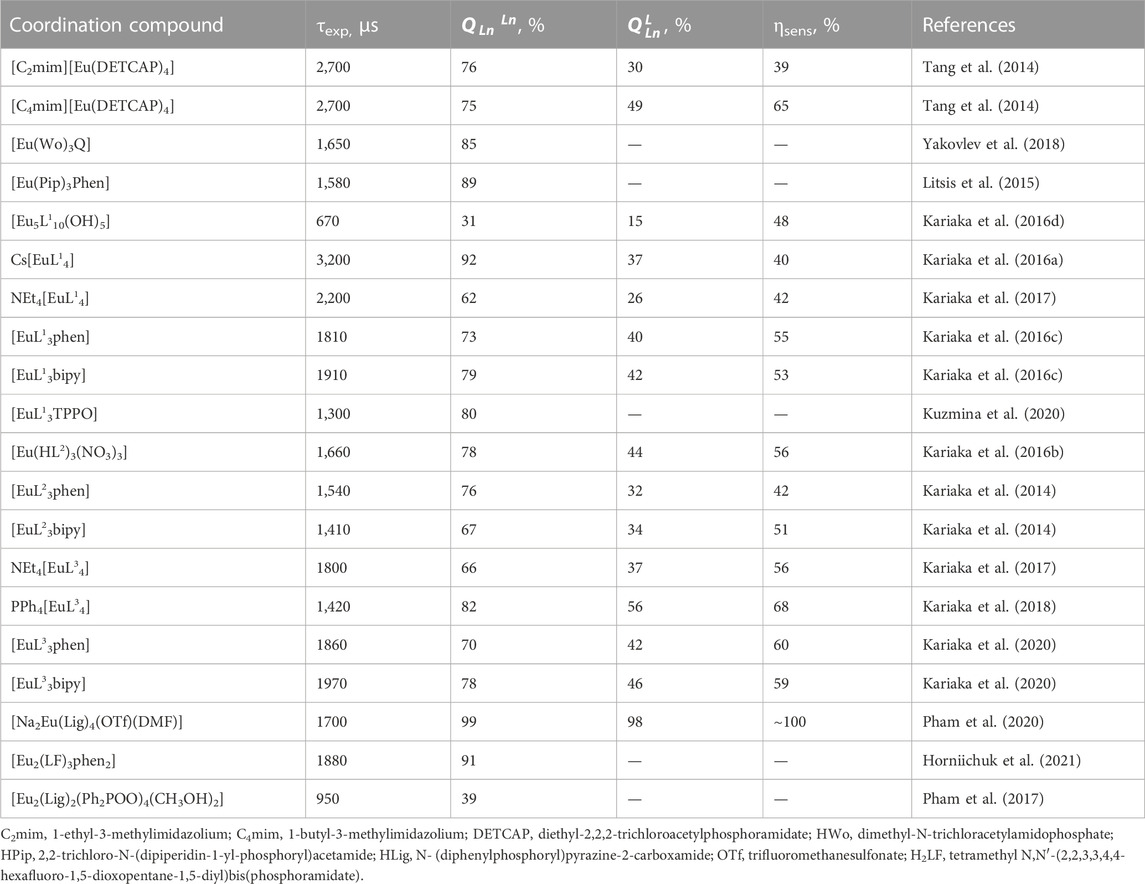
TABLE 4. Experimental luminescence characteristics of some phenyl-containing CAPh-based Eu3+ coordination compounds in crystalline state at room temperature.
Herein we will limit ourselves to a more detailed discussion of the luminescence properties of phenyl-containing CAPh-based Eu3+ and Tb3+ coordination compounds (Figure 6), obtained in the experiment and using theoretical calculations. Among these coordination compounds, the highest value of
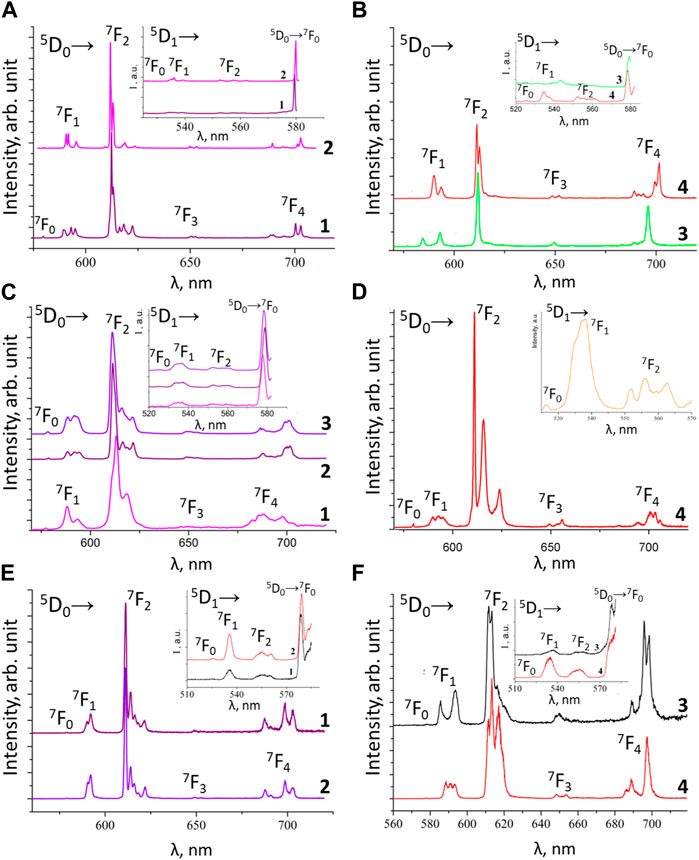
FIGURE 6. Low temperature (77 K) emission spectra of the europium compounds: (A) [EuL13Phen] (1) and [EuL13bipy] (2); (B) NEt4[EuL14] (3) and Cs[EuL14] (4); (C) [Eu(HL2)3(NO3)3] (1), [EuL23Phen] (2) and [EuL23bipy] (3); (D) [EuL13TPPO] (4); (E) [EuL33Phen] (1) and [EuL33bipy] (2); (F) NEt4[EuL34] (3) and PPh4[EuL34] (4).
The low temperature (77 K) luminescence spectra of the Eu3+ coordination compounds [EuL3bipy], [EuL3phen] (L = L1, L2, L3), Cat[EuL4] (L = L1, L3), [EuL13TPPO], and [Eu(HL2)3(NO3)3] are shown in Figure 6. The insets in these figures show 5D1 emission and the transition 5D0→7F0 at 77 K.
The 5D0 luminescence spectra of these coordination compounds are characterized by the presence of narrow bands, corresponding to transitions 5D0→7FJ (J = 0–4) and the absence of the ligand’s fluorescence band. For all these Eu3+ coordination compounds, except for NEt4[EuL34] and PPh4[EuL34], the 5D0→7F0 transition band at 77 K is a symmetrical singlet. For the compounds NEt4[EuL34] and PPh4[EuL34], this band is of low intensity, which makes reliable analysis of it impossible. The intensities distributions and the ratios between the hypersensitive and the magnetic dipole transitions 5D0→7F2/5D0→7F1 (red/orange ratio) are given in Table 5.
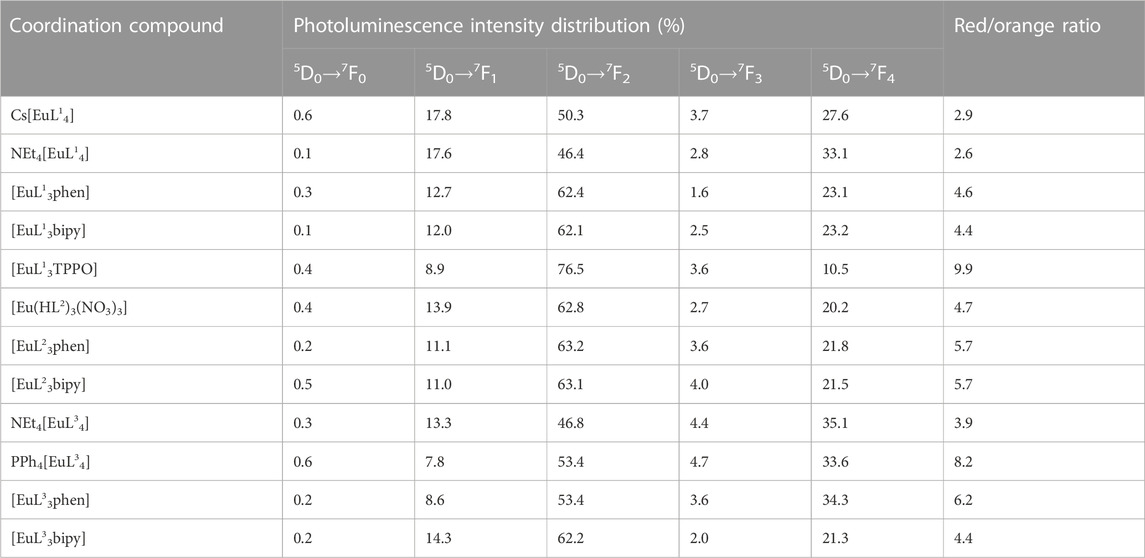
TABLE 5. Photoluminescence intensity distributions (%) for the europium compounds based on phenyl-containing CAPhs at 298 K.
The value of the red/orange ratio for Eu3+ compounds with CAPhs ligand (from 2.6 to 9.9) is smaller than for Eu3+ β-diketonates (from 7 to 27) (Klink et al., 2000; Binnemans et al., 2005). Unfortunately, based on these values it is not possible to draw conclusions about the comparison of the symmetry of the surroundings of the Eu3+ ion (Tanner, 2013; Binnemans, 2015) in compounds with CAPhs ligands and β-diketones. It should be remembered that the red/orange ratio is affected by various factors, including the polarizability of the ligands.
The quite high intensity of the 5D0→7F4 transition bands for tetrakis-compounds should be noted compared to tris-compounds with different ligands (Kariaka et al., 2022). Such an unusual behavior was earlier observed for some compounds and explained by the deviation of the EuO8 coordination polyhedron from the cubic geometry to D2 (Bettinelli et al., 2011) or by the highly polarized environment of Eu3+ with local symmetry corresponding to the D4d coordination geometry (Sá Ferreira et al., 2006).
The decay curves for all the considered CAPh-based europium coordination compounds have a monoexponential character. The luminescence lifetime does not depend on temperature (Table 6), which proves the absence of temperature-dependent non-radiative transitions in the compounds. Cs[EuL14] shows the highest value of the luminescence lifetime. The lowest luminescence lifetime is observed for the mixed-ligand compounds [EuL23phen], [EuL23bipy], and the tetrakis-compound PPh4[EuL34]. The 5D0 emission lifetime is dependent on the excitation wavelength, which is characteristic of many Eu3+ compounds with different ligands (Ferreira et al., 2012). This phenomenon is explained by the fact that the lifetime is related to the process of changing the occupancy levels of the lanthanide over time, and the latter depends on the presence or absence of energy transfer channels in the lanthanide itself and in its surroundings.
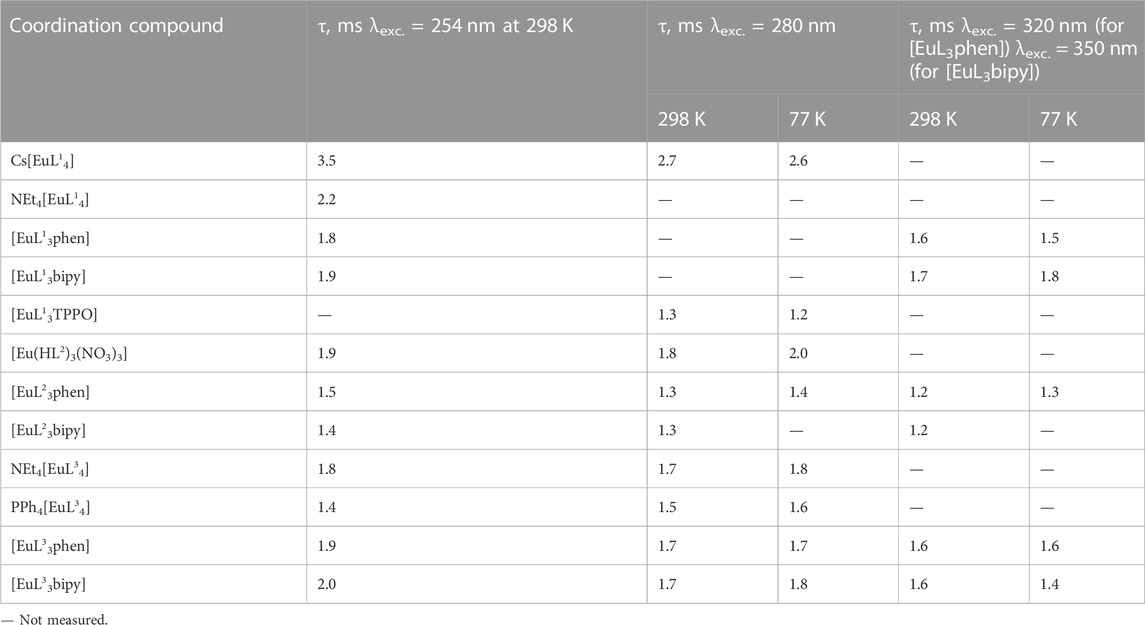
TABLE 6. Emission decay times of the Eu3+ coordination compounds at different wavelength excitations.
The luminescence spectra of the Tb3+ coordination compounds [TbL3bipy], [TbL3phen] (L = L1, L2, L3), Cat[TbL4] (L = L1, L3), [TbL13TPPO], and [Tb(HL2)3(NO3)3] are shown in Figure 7.
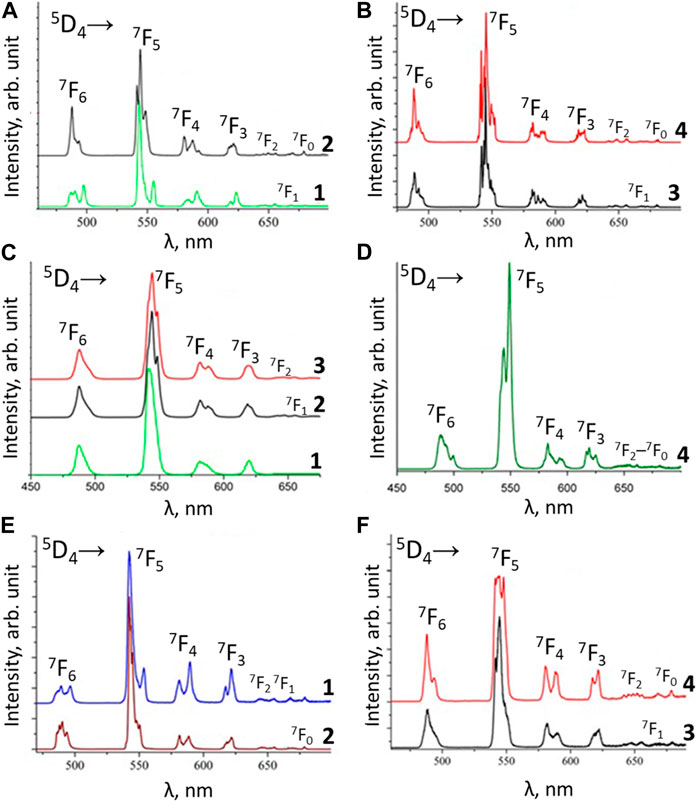
FIGURE 7. Low temperature (77 K) emission spectra of the terbium complexes: (A) NEt4[TbL14] (1) and Cs[TbL14] (2); (B)- [TbL13Phen] (3) and [TbL13bipy] (4); (C) [Tb(HL2)3(NO3)3] (1), [TbL23Phen] (2) and [TbL23bipy] (3); (D) [TbL13TPPO] (4); (E) NEt4[TbL34] (1) and PPh4 [TbL34] (2); (F) [TbL33Phen] (3) and [TbL33bipy] (4).
Similar to the Eu3+ coordination compounds, the luminescence spectra of the Tb3+ coordination compounds, obtained upon excitation into the ligand absorption bands, are characterized by the presence of narrow bands, corresponding to Tb3+ 4f-4f transitions 5D4→7FJ (J = 6–0) and absence of the ligand’s fluorescence band. This points to an effective excitation energy transfer from the ligands to the Eu3+ and Tb3+ ions. An effective excitation energy transfer is also confirmed by the excitation spectra, in which the intensities of the ligands’ absorption bands are significantly higher compared to f-f transitions of the Eu3+ and Tb3+ ions. The bands with maxima at ∼490, ∼545, ∼680, ∼620, ∼650, ∼670, and ∼680 nm in the luminescence spectra of the Tb3+ coordination compounds are assigned to 5D4-7FJ (J = 6–0) transitions, respectively. The band of 5D4→7F5 transition dominates in intensity in all the spectra. It is known that the luminescence spectra of terbium have a more complex Stark structure compared to the spectra of europium. In addition, in the case of terbium, even at 77 K, the Stark sublevels of the term 5D4 remain populated. These factors make it difficult to analyze the environment around the Tb3+ ion in compounds according to spectral criteria.
The Tb3+ luminescence intensity was measured for powdered samples. The coordination compounds’ crystals were ground thoroughly with a mortar and pestle for 2-3 min. The obtained powders were loaded in a solid sample cuvette (cell) supplied with Fluorolog FL 3–22. The front surface of each sample was also examined to ensure the absence of cracks and crevices in the powder. Such cracks and crevices were removed by gently tapping the cuvette or by gently pressing on the sample with a spatula and tracing paper. The [TbL23bipy] exhibited the highest emission intensity among the examined Tb3+ coordination compounds (Table 7). The lowest luminescence intensity was found for [Tb(HL2)3(NO3)3]. It should be noted that, in contrast to europium compounds, the terbium mixed ligand tris-compounds with 1,10-phenanthroline show lower emission intensity compared to coordination compounds with 2,2′-bipyridyne, which can be explained by better matching of the triplet level of 2,2′-bipyridyne with terbium 5D4 level, compared to 1,10-phenathroline. The [TbL3phen] luminescence intensity follows the trend of the ligands’ triplet state energy reduction (Figure 8). However, different emission intensities are observed for [TbL3bipy] compounds at the same ΔE value, which shows the importance of other factors in the sensitization process as well.
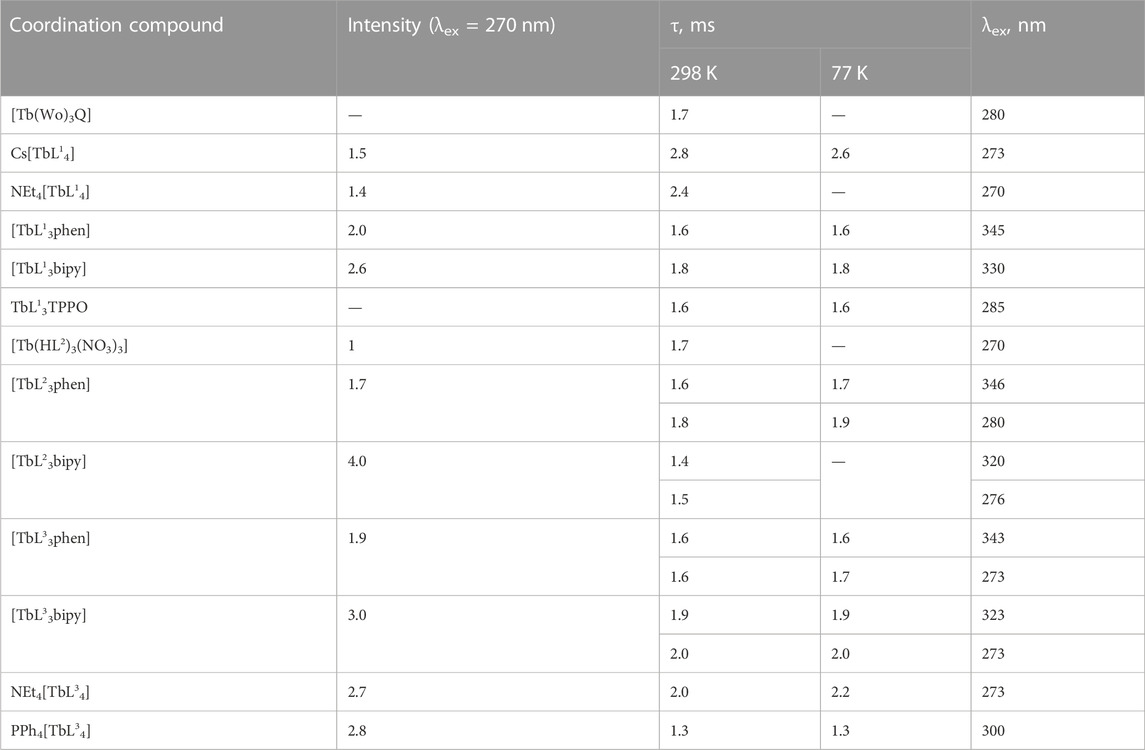
TABLE 7. Experimental luminescence characteristics of some phenyl-containing CAPh-based Tb3+ coordination compounds in crystal state at room temperature.
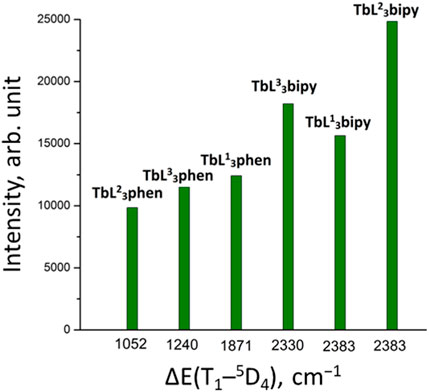
FIGURE 8. The luminescence intensity of [TbL3phen] and [TbL3bipy] coordination compounds for solid samples at room temperature.
8 Conclusions and perspectives
This review summarizes studies on luminescent lanthanide coordination compounds based on carbacylamidophosphates and focuses on the thermal behavior and luminescence of Eu3+ and Tb3+ coordination compounds. The nature of lanthanides luminescence and ways of its enhancement are described.
Comparing CAPh-based compounds to commonly used phosphors like β-diketonates can help showcase the distinctive thermal and spectral properties of CAPh-based compounds. Additionally, theoretical methods have proven to be effective in quantifying the energy transfer process between these classes of ligands and the Ln3+ ion.
Being close structural analogues of β-diketones, carbacylamidophosphates, however, differ from the former in their coordination behavior, spectral properties, and resistance to exposure to UV radiation, and thus are considered a promising new class of ligands for the design of highly luminescent materials for practical use. Phenyl-containing CAPh-based Eu3+ and Tb3+ coordination compounds possess intense, long-lasting luminescence. The rather high energy of the triplet state of the carbacylamidophosphates and the suppression of multiphonon emission quenching provides possibilities to use them for sensitization of a wider list of lanthanides, emitting in the visible region, including Dy3+ yellow/white emission and Tm3+ blue emission as well as in the NIR range - Nd3+, Er3+, and Yb3+.
Author contributions
NK: investigation, conceptualization, data curation, methodology, writing—original draft, writing—review and editing. AL: investigation, data curation, methodology, writing–original draft, writing—review and editing. AC: methodology, visualization, formal analysis, software, writing—review and editing. OM: methodology, writing—review and editing. PG: conceptualization, investigation, data curation, writing—original draft, visualization. VA: conceptualization, investigation, data curation, writing—original draft, writing—review and editing, visualization, supervision. All authors listed have made a substantial, direct, and intellectual contribution to the work and approved it for publication. All authors contributed to the article and approved the submitted version.
Funding
FCT (Fundação para a Ciência e Tecnologia, Portugal)—The Shape of Water Project—Grant number: PTDC/NAN-PRO/3881/2020. FCT (Fundação para a Ciência e Tecnologia, Portugal)—LogicALL project—Grant number: PTDC/CTM-CTM/0340/2021. Ministry of Education and Science of Ukraine (Grant 22BF037-04). This work was developed within the scope of the projects CICECO-Aveiro Institute of Materials, UIDB/50011/2020, UIDP/50011/2020, and LA/P/0006/2020, The Shape of Water (PTDC/NAN-PRO/3881/2020), and LogicALL (PTDC/CTM-CTM/0340/2021), financed by national funds through the FCT/MCTES (PIDDAC).
Conflict of interest
The authors declare that the research was conducted in the absence of any commercial or financial relationships that could be construed as a potential conflict of interest.
Publisher’s note
All claims expressed in this article are solely those of the authors and do not necessarily represent those of their affiliated organizations, or those of the publisher, the editors and the reviewers. Any product that may be evaluated in this article, or claim that may be made by its manufacturer, is not guaranteed or endorsed by the publisher.
References
Adati, R., Monteiro, J., Cardoso, L., de Oliveira, D., Jafelicci, M., and Davolos, M. (2019). The influence of different ammonium cations on the optical properties of tetrakis GdIII and EuIII complexes. J. Braz. Chem. Soc. 30, 1707–1716. doi:10.21577/0103-5053.20190073
Almeida, C. M. R., Magalhães, J. M. C. S., Barroso, M. F., and Durães, L. (2022). Latest advances in sensors for optical detection of relevant amines: Insights into lanthanide-based sensors. J. Mater. Chem. C 10, 15263–15276. doi:10.1039/D2TC03143D
Amirkhanov, V., Ovchinnikov, V., Legendziewicz, J., Graczyk, A., Hanuza, J., and Macalik, L. (1996). Spectroscopic studies of neodymium and europium phosphoro-azo β-diketonates. Acta Phys. Pol. A 90, 455–460. doi:10.12693/APhysPolA.90.455
Amirkhanov, V., Ovchinnikov, V., Trush, V., Gawryszewska, P., and Jerzykiewicz, L. B. (2014). “Powerful new ligand systems: Carbacylamidophosphates (CAPh) and sulfonylamidophosphates (SAPh),” in Ligands. Synthesis, characterization and role in biotechnology. Editors P. Gawryszewska, and P. Smoleński (New York: Nova Publishers), 199–248.
Aquino, L. E. do N., Barbosa, G. A., Ramos, J. de L., Giese, S. O. K., Santana, F. S., Hughes, D. L., et al. (2021). Seven-coordinate Tb3+ complexes with 90% quantum yields: High-performance examples of combined singlet- and triplet-to-Tb3+ energy-transfer pathways. Inorg. Chem. 60, 892–907. doi:10.1021/acs.inorgchem.0c03020
Arrué, L., Santoyo-Flores, J., Pizarro, N., Zarate, X., Páez-Hernández, D., and Schott, E. (2021). The role played by structural and energy parameters of β-Diketones derivatives as antenna ligands in Eu(III) complexes. Chem. Phys. Lett. 773, 138600. doi:10.1016/j.cplett.2021.138600
Batista, H. J., de Andrade, A. V. M., Longo, R. L., Simas, A. M., de Sá, G. F., Ito, N. K., et al. (1998). Synthesis, X-ray structure, spectroscopic characterization, and theoretical prediction of the structure and electronic spectrum of Eu(btfa)3·bipy and an assessment of the effect of fluorine as a β-diketone substituent on the Ligand−Metal energy. Inorg. Chem. 37, 3542–3547. doi:10.1021/ic971602v
Bauer, H., Blanc, J., and Ross, D. L. (1964). Octacoordinate chelates of lanthanides. Two series of compounds. J. Am. Chem. Soc. 86, 5125–5131. doi:10.1021/ja01077a016
Becquerel, J. (1906). Sur les variations des bandes d’absorption d’un cristal dans un champ magnétique. Compt. Rend. Hebd. Acad. Sci. 142, 775–779.
Becquerel, J. (1929). Einleitung in eine Theorie der magneto-optischen Erscheinungen in Kristallen. Z. Für Phys. 58, 205–216. doi:10.1007/BF01339043
Bethe, H. (1930). Zur Theorie des Zeemaneffektes an den Salzen der seltenen Erden. Z. Für Phys. 60, 218–233. doi:10.1007/BF01339827
Bettinelli, M., Speghini, A., Piccinelli, F., Neto, A. N. C., and Malta, O. L. (2011). Luminescence spectroscopy of Eu3+ in Ca3Sc2Si3O12. J. Lumin. 131, 1026–1028. doi:10.1016/j.jlumin.2011.01.016
Bhaumik, M. L., and El-Sayed, M. A. (1965). Mechanism and rate of the intramolecular energy transfer process in rare-earth chelates. J. Chem. Phys. 42, 787–788. doi:10.1063/1.1696007
Bhaumik, M. L. (1964). Quenching and temperature dependence of fluorescence in rare-earth chelates. J. Chem. Phys. 40, 3711–3715. doi:10.1063/1.1725078
Biju, S., Ambili Raj, D. B., Reddy, M. L. P., and Kariuki, B. M. (2006). Synthesis, crystal structure, and luminescent properties of novel Eu3+ heterocyclic β-diketonate complexes with bidentate nitrogen donors. Inorg. Chem. 45, 10651–10660. doi:10.1021/ic061425a
Biju, S., Reddy, M. L. P., Cowley, A. H., and Vasudevan, K. V. (2009). 3-Phenyl-4-acyl-5-isoxazolonate complex of Tb3+ doped into poly-β-hydroxybutyrate matrix as a promising light-conversion molecular device. J. Mater. Chem. 19, 5179. doi:10.1039/b905304b
Binnemans, K. (2005). “Rare-Earth beta-diketonates,” in Handbook of the physics and chemistry rare earths. Editors J. K. A. Gschneidner, J.-C. G. Bünzli, and V. K. Pecharsky (Elsevier), 107–272. doi:10.1016/S0168-1273(05)35003-3
Binnemans, K. (2015). Interpretation of europium(III) spectra. Coord. Chem. Rev. 295, 1–45. doi:10.1016/j.ccr.2015.02.015
Borges, A. S., Fulgêncio, F., Da Silva, J. G., Ribeiro-Santos, T. A., Diniz, R., Windmöller, D., et al. (2019). Luminescence and positron spectroscopies studies of tris(2,2,6,6-tetramethyl-3,5-heptanedionate) europium(III) and terbium(III) complexes containing 2-pyrrolidone as coligand. J. Lumin. 205, 72–81. doi:10.1016/j.jlumin.2018.07.031
Borzechowska, M., Trush, V., Turowska-Tyrk, I., Amirkhanov, W., and Legendziewicz, J. (2002). Spectroscopic and magnetic studies of mixed lanthanide complexes: LnL3α,α′Dipy in solution and in solid. J. Alloys Compd. 341, 98–106. doi:10.1016/S0925-8388(02)00075-0
Brito, H. F., Malta, O. M. L., Felinto, M. C. F. C., and Teotonio, E. E. S. (2010). “Luminescence phenomena involving metal enolates,” in PATAI'S chemistry of functional groups (Chichester, UK: John Wiley and Sons, Ltd). doi:10.1002/9780470682531.pat0419
Bruno, S. M., Ferreira, R. A. S., Almeida Paz, F. A., Carlos, L. D., Pillinger, M., Ribeiro-Claro, P., et al. (2009). Structural and photoluminescence studies of a europium(III) tetrakis(β-diketonate) complex with tetrabutylammonium, imidazolium, pyridinium and silica-supported imidazolium counterions. Inorg. Chem. 48, 4882–4895. doi:10.1021/ic900274a
Bünzli, J.-C. G., and V Eliseeva, S. (2010). “Basics of lanthanide photophysics,” in Lanthanide luminescence. Editors P. Hänninen, and H. Härmä (Berlin, Heidelberg: Springer), Vol. 7, 1–45. doi:10.1007/4243_2010_3
Bünzli, J.-C. G. (2015). On the design of highly luminescent lanthanide complexes. Coord. Chem. Rev. 293-294, 19–47. doi:10.1016/j.ccr.2014.10.013
Bünzli, J. C. G. (2017). Rising stars in science and Technology: Luminescent lanthanide materials. Eur. J. Inorg. Chem. 2017, 5058–5063. doi:10.1002/ejic.201701201
Carlos, L. D., De Mello Donegá, C., Albuquerque, R. Q., Alves, S., Menezes, J. F. S., and Malta, O. L. (2003). Highly luminescent europium(III) complexes with naphtoiltrifluoroacetone and dimethyl sulphoxide. Mol. Phys. 101, 1037–1045. doi:10.1080/0026897021000046898
Carlos, L. D., Fernandes, J. A., Ferreira, R. A. S., Malta, O. L., Gonçalves, I. S., and Ribeiro-Claro, P. (2005). Emission quantum yield of a europium(III) tris-β-diketonate complex bearing a 1,4-diaza-1,3-butadiene: Comparison with theoretical prediction. Chem. Phys. Lett. 413, 22–24. doi:10.1016/j.cplett.2005.07.033
Carnall, W. T., Fields, P. R., and Rajnak, K. (1968). Electronic energy levels of the trivalent lanthanide aquo ions. IV. Eu3+. J. Chem. Phys. 49, 4450–4455. doi:10.1063/1.1669896
Carnall, W. T., Fields, P. R., and Rajnak, K. (1968). Electronic energy levels in the trivalent lanthanide aquo ions. I. Pr3+, Nd3+, Pm3+, Sm3+, Dy3+, Ho3+, Er3+, and Tm3+. J. Chem. Phys. 49, 4424–4442. doi:10.1063/1.1669893
Carnall, W. T., Fields, P. R., and Rajnak, K. (1968). Electronic energy levels of the trivalent lanthanide aquo ions. II. Gd3+. J. Chem. Phys. 49, 4443–4446. doi:10.1063/1.1669894
Carnall, W. T., Fields, P. R., and Rajnak, K. (1968). Electronic energy levels of the trivalent lanthanide aquo ions. III. Tb3+. J. Chem. Phys. 49, 4447–4449. doi:10.1063/1.1669895
Carnall, W. T., Crosswhite, H., and Crosswhite, H. M. (1978). Energy level structure and transition probabilities in the spectra of the trivalent lanthanides in LaF₃. Argonne, IL, United States. doi:10.2172/6417825
Carnall, W. T. (1979). “Chapter 24 the absorption and fluorescence spectra of rare Earth ions in solution,” in Handbook of the physics and chemistry rare earths. Editors J. K. A. Gschneidner, and L. Eyring, 3, 171–208. doi:10.1016/S0168-1273(79)03007-5
Carnall, W. T., V Beitz, J., Crosswhite, H., Rajnak, K., and Mann, J. B. (1983). “Spectroscopic properties of the f-elements in compounds and solutions,” in Systematics and the properties of the lanthanides (Dordrecht: Springer Netherlands), 389–450. doi:10.1007/978-94-009-7175-2_9
Carnall, W. T., Goodman, G. L., Rajnak, K., and Rana, R. S. (1989). A systematic analysis of the spectra of the lanthanides doped into single crystal LaF3. J. Chem. Phys. 90, 3443–3457. doi:10.1063/1.455853
Carneiro Neto, A. N., and Moura, R. T. (2020). Overlap integrals and excitation energies calculations in trivalent lanthanides 4f orbitals in pairs Ln-L (L = Ln, N, O, F, P, S, Cl, Se, Br, and I). Chem. Phys. Lett. 757, 137884. doi:10.1016/j.cplett.2020.137884
Carneiro Neto, A. N., Teotonio, E. E. S., de Sá, G. F., Brito, H. F., Legendziewicz, J., Carlos, L. D., et al. (2019). “Modeling intramolecular energy transfer in lanthanide chelates: A critical review and recent advances,” in Handbook of the physics and chemistry rare earths. Editors J.-C. G. Bünzli, and V. K. Pecharsky (Elsevier), 56, 55–162. doi:10.1016/bs.hpcre.2019.08.001
Carneiro Neto, A. N., Mamontova, E., Botas, A. M. P., Brites, C. D. S., Ferreira, R. A. S., Rouquette, J., et al. (2021). Rationalizing the thermal response of dual-center molecular thermometers: The example of an Eu/Tb coordination complex. Adv. Opt. Mater. 10, 2101870. doi:10.1002/adom.202101870
Carneiro Neto, A. N., Moura, R. T., Carlos, L. D., Malta, O. L., Sanadar, M., Melchior, A., et al. (2022). Dynamics of the energy transfer process in Eu(III) complexes containing polydentate ligands based on pyridine, quinoline, and isoquinoline as chromophoric antennae. Inorg. Chem. 61, 16333–16346. doi:10.1021/acs.inorgchem.2c02330
Crosby, G. A., and Kasha, M. (1958). Intramolecular energy transfer in ytterbium organic chelates. Spectrochim. Acta. 10, 377–382. doi:10.1016/0371-1951(58)80105-8
Crosby, G. A., Whan, R. E., and Alire, R. M. (1961). Intramolecular energy transfer in rare earth chelates. Role of the triplet state. J. Chem. Phys. 34, 743–748. doi:10.1063/1.1731670
Crosby, G. A., Whan, R. E., and Freeman, J. J. (1962). Spectroscopic studies of rare earth chelates. J. Phys. Chem. 66, 2493–2499. doi:10.1021/j100818a041
de Mello Donegá, C., Junior, S. A., and de Sá, G. (1997). Synthesis, luminescence and quantum yields of Eu(III) mixed complexes with 4,4,4-trifluoro-1-phenyl-1,3-butanedione and 1,10-phenanthroline-N-oxide. J. Alloys Compd. 250, 422–426. doi:10.1016/S0925-8388(96)02562-5
de Sá, G. F., Malta, O. L., de Mello Donegá, C., Simas, A. M., Longo, R. L., Santa-Cruz, P. A., et al. (2000). Spectroscopic properties and design of highly luminescent lanthanide coordination complexes. Coord. Chem. Rev. 196, 165–195. doi:10.1016/S0010-8545(99)00054-5
Dieke, G. H. (1968). Spectra and energy levels of rare earth ions in crystals. New York: Wiley Interscience.
Divya, V., Freire, R. O., and Reddy, M. L. P. (2011). Tuning of the excitation wavelength from UV to visible region in Eu3+-β-diketonate complexes: Comparison of theoretical and experimental photophysical properties, Dalt. Trans 40, 3257. doi:10.1039/c0dt01652g
dos Santos, E. R., Freire, R. O., da Costa, N. B., Paz, F. A. A., de Simone, C. A., Júnior, S. A., et al. (2010). Theoretical and experimental spectroscopic approach of fluorinated ln3+−β-diketonate complexes. J. Phys. Chem. A 114, 7928–7936. doi:10.1021/jp104038r
Dutra, J. D. L., Bispo, T. D., and Freire, R. O. (2014). LUMPAC lanthanide luminescence software: Efficient and user friendly. J. Comput. Chem. 35, 772–775. doi:10.1002/jcc.23542
e Silva, F. R. G., and Malta, O. L. (1997). Calculation of the ligand–lanthanide ion energy transfer rate in coordination compounds: Contributions of exchange interactions. J. Alloys Compd. 250, 427–430. doi:10.1016/S0925-8388(96)02563-7
e Silva, F. R. G., Menezes, J. F. S., Rocha, G. B., Alves, S., Brito, H. F., Longo, R. L., et al. (2000). Emission quantum yield of europium (III) mixed complexes with thenoyltrifluoroacetonate and some aromatic ligands. J. Alloys Compd. 303–304, 364–370. doi:10.1016/S0925-8388(00)00642-3
Edvardsson, S., and Klintenberg, M. (1998). Role of the electrostatic model in calculating rare-Earth crystal-field parameters. J. Alloys Compd. 275–277, 230–233. doi:10.1016/S0925-8388(98)00309-0
Eliseeva, S. V., Kotova, O. V., Gumy, F., Semenov, S. N., Kessler, V. G., Lepnev, L. S., et al. (2008). Role of the ancillary ligand N,N-dimethylaminoethanol in the sensitization of EuIII and TbIII luminescence in dimeric β-diketonates. J. Phys. Chem. A 112, 3614–3626. doi:10.1021/jp711305u
Faustino, W. M., Malta, O. L., and de Sá, G. F. (2005). Intramolecular energy transfer through charge transfer state in lanthanide compounds: A theoretical approach. J. Chem. Phys. 122, 054109. doi:10.1063/1.1830452
Ferreira, R. A. S., Nolasco, M., Roma, A. C., Longo, R. L., Malta, O. L., and Carlos, L. D. (2012). Dependence of the lifetime upon the excitation energy and intramolecular energy transfer rates: The 5D0 EuIII emission case. Chem. - A Eur. J. 18, 12130–12139. doi:10.1002/chem.201201736
Filipescu, N., Sager, W. F., and Serafin, F. A. (1964). Substituent effects on intramolecular energy transfer. II. Fluorescence spectra of europium and terbium β-diketone chelates. J. Phys. Chem. 68, 3324–3346. doi:10.1021/j100793a039
Fu, C.-Y., Chen, L., Wang, X., and Lin, L.-R. (2019). Synthesis of bis-β-diketonate lanthanide complexes with an azobenzene bridge and studies of their reversible photo/thermal isomerization properties. ACS Omega 4, 15530–15538. doi:10.1021/acsomega.9b01817
Gameiro, C. G., da Silva, E. F., Alves, S., de Sá, G., and Santa-Cruz, P. (2001). Lanthanide complexes dispersed in enamel: A promising new material for photonic devices. J. Alloys Compd. 323–324, 820–823. doi:10.1016/S0925-8388(01)01152-5
Gawryszewska, P., and Smolenski, P. (2014). Ligands: Synthesis, characterization and role in biotechnology, ligands synth. Charact. Role Biotechnol., 1–295.
Gawryszewska, P., Sokolnicki, J., and Legendziewicz, J. (2005). Photophysics and structure of selected lanthanide compounds. Coord. Chem. Rev. 249, 2489–2509. doi:10.1016/j.ccr.2005.06.021
Georgieva, I., Zahariev, T., Aquino, A. J. A., Trendafilova, N., and Lischka, H. (2020). Energy transfer mechanism in luminescence Eu(III) and Tb(III) complexes of coumarin-3-carboxylic acid: A theoretical study. Spectrochim. Acta - Part A Mol. Biomol. Spectrosc. 240, 118591. doi:10.1016/j.saa.2020.118591
Gérard, I., Krupa, J., Simoni, E., and Martin, P. (1994). Investigation of charge transfer O2− → Ln3+ and F− → Ln3+ in LaF3:(ln3+, O2−) and YF3:(ln3+, O2−) systems. J. Alloys Compd. 207–208, 120–127. doi:10.1016/0925-8388(94)90191-0
Grant, W. J. C. (1971). Role of rate equations in the theory of luminescent energy transfer. Phys. Rev. B 4, 648–663. doi:10.1103/PhysRevB.4.648
Haas, Y., and Stein, G. (1971). Pathways of radiative and radiationless transitions in europium(III) solutions. The role of high energy vibrations. J. Phys. Chem. 75, 3677–3681. doi:10.1021/j100693a006
He, P., Wang, H., Liu, S., Shi, J., Wang, G., and Gong, M. (2009). Effect of different alkyl groups at the N-position on the luminescence of carbazole-based β-diketonate europium(III) complexes. J. Phys. Chem. A 113, 12885–12890. doi:10.1021/jp908416q
He, P., Wang, H. H., Liu, S. G., Shi, J. X., and Gong, M. L. (2010). An efficient 2-linked carbazolyl β-diketonate europium(III) complex as red phosphor applied in LED. Appl. Phys. B 99, 757–762. doi:10.1007/s00340-010-3972-3
Heller, A. (1966). formation of hot OH bonds in the radiationless relaxations of excited rare earth ions in aqueous solutions. J. Am. Chem. Soc. 88, 2058–2059. doi:10.1021/ja00961a046
Hernández-Rodríguez, M. A., Zanella, S., Fu, L., Neto, A. N. C., Carlos, L. D., and Brites, C. D. S. (2023). Designing all-photonic molecular analogs for electrical components: A reprogrammable luminescent filter based on Ln3+ ions. Laser Phot. Rev., 2200877. accepted. doi:10.1002/lpor.202200877
Horniichuk, O. Y., Kariaka, N. S., Smola, S. S., Rusakova, N. V., Trush, V. O., Sliva, T. Y., et al. (2021). Efficient sensitized luminescence of binuclear ln(III) complexes based on a chelating bis-carbacylamidophosphate. J. Fluoresc. 31, 1029–1039. doi:10.1007/s10895-021-02733-0
Jiang, L., Wu, J., Wang, G., Ye, Z., Zhang, W., Jin, D., et al. (2010). Development of a visible-light-sensitized europium complex for time-resolved fluorometric application. Anal. Chem. 82, 2529–2535. doi:10.1021/ac100021m
Judd, B. R. (1962). Optical absorption intensities of rare-Earth ions. Phys. Rev. 127, 750–761. doi:10.1103/PhysRev.127.750
Judd, B. R. (1979). Ionic transitions hypersensitive to environment. J. Chem. Phys. 70, 4830–4833. doi:10.1063/1.437372
Junior, S. A., de Almeida, F. V., de Sá, G. F., and de Mello Donegá, C. (1997). Luminescence and quantum yields of Eu3+ mixed complexes with 1-phenyl-1,3-butanedione and 1,10-phenanthroline or 1,10-phenanthroline-N-oxide. J. Lumin. 72–74, 478–480. doi:10.1016/S0022-2313(97)00025-2
Kai, J., Felinto, M. C. F. C., Nunes, L. A. O., Malta, O. L., and Brito, H. F. (2011). Intermolecular energy transfer and photostability of luminescence-tuneable multicolour PMMA films doped with lanthanide–β-diketonate complexes. J. Mater. Chem. 21, 3796. doi:10.1039/c0jm03474f
Kariaka, N. S., Trush, V. A., Sliva, T. Y., Dyakonenko, V. V., Shishkin, O. V., and Amirkhanov, V. M. (2014). Synthesis and spectral studies of lanthanides coordination compounds based on N-(diphenylphosphoryl)benzamide. The structure of N-(diphenylphosphoryl)benzamide. J. Mol. Struct. 1068, 71–76. doi:10.1016/j.molstruc.2014.03.069
Kariaka, N. S., Trush, V. A., Medviediev, V. V., Dyakonenko, V. V., Shishkin, O. V., Smola, S. S., et al. (2016). Coordination compounds based on CAPh type ligand: Synthesis, structural characteristics and luminescence properties of tetrakis-complexes CsLnL4 with dimethylbenzoylamidophosphate. J. Coord. Chem. 69, 123–134. doi:10.1080/00958972.2015.1115024
Kariaka, N. S., Trush, V. A., Smola, S. S., Fadeyev, E. M., Odynets, I. V., Sliva, T. Y., et al. (2016). Tris-(diphenylphosphoryl)benzamide lanthanides (III) trinitrates as a basis for creating the luminophore materials. Rep. Natl. Acad. Sci. Ukr., 85–94. doi:10.15407/dopovidi2016.05.085
Kariaka, N. S., Trush, V. A., Gawryszewska, P., Dyakonenko, V. V., Shishkina, S. V., Sliva, T. Y., et al. (2016). Spectroscopy and structure of [LnL3bipy] and [LnL3phen] complexes with CAPh type ligand dimethylbenzoylamidophosphate. J. Lumin. 178, 392–399. doi:10.1016/j.jlumin.2016.06.018
Kariaka, N. S., Rusanova, J. A., Smola, S. S., Kolotilov, S. V., Znovjyak, K. O., Weselski, M., et al. (2016). First examples of carbacylamidophosphate pentanuclear hydroxo-complexes: Synthesis, structure, luminescence and magnetic properties. Polyhedron 106, 44–50. doi:10.1016/j.poly.2015.12.052
Kariaka, N. S., Smola, S. S., Rusakova, N. V., Sliva, T. Y., and Amirkhanov, V. M. (2017). Luminescent lanthanide tetrakis-complexes with diphenyl-N-benzoylamidophosphate and tetraethylammonium cation. Odesa Natl. Univ. Her. Chem. 22, 32–41. doi:10.18524/2304-0947.2017.2(62).99915
Kariaka, N. S., Trush, V. A., Smola, S. S., Fadieiev, Y. M., Dyakonenko, V. V., Shishkina, S. V., et al. (2018). Highly luminescent diphenyl-N-benzoylamidophosphate based lanthanide tetrakis-complexes. J. Lumin. 194, 108–115. doi:10.1016/j.jlumin.2017.09.027
Kariaka, N. S., Smola, S. S., Rusakova, N. V., Dyakonenko, V. V., Shishkina, S. V., Sliva, T. Y., et al. (2020). Synthesis, crystal structure and luminescent properties of novel lanthanide complexes with CAPh type ligand diphenyl-N-benzoylamidophosphate and bidentate nitrogen donors. J. Lumin. 223, 117187. doi:10.1016/j.jlumin.2020.117187
Kariaka, N. S., Trush, V. A., Dyakonenko, V. V., Shishkina, S. V., Smola, S. S., Rusakova, N. V., et al. (2022). New luminescent lanthanide tetrakis-complexes NEt4[LnL4] based on dimethyl-N-benzoylamidophosphate. ChemPhysChem 23, e202200129. doi:10.1002/cphc.202200129
Kasprzycka, E., Trush, V. A., Amirkhanov, V. M., Jerzykiewicz, L., Malta, O. L., Legendziewicz, J., et al. (2017). Contribution of energy transfer from the singlet state to the sensitization of Eu3+ and Tb3+ luminescence by sulfonylamidophosphates. Chem. - A Eur. J. 23, 1318–1330. doi:10.1002/chem.201603767
Kasprzycka, E., Carneiro Neto, A. N., Trush, V. A., Jerzykiewicz, L., Amirkhanov, V. M., Malta, O. L., et al. (2020). How minor structural changes generate major consequences in photophysical properties of RE coordination compounds; resonance effect, LMCT state. J. Rare Earths. 38, 552–563. doi:10.1016/j.jre.2020.02.001
Kasprzycka, E., Carneiro Neto, A. N., Trush, V. A., Malta, O. L., Jerzykiewicz, L., Amirkhanov, V. M., et al. (2022). Spectroscopic aspects for the Yb3+ coordination compound with a large energy gap between the ligand and Yb3+ excited states. Spectrochim. Acta Part A Mol. Biomol. Spectrosc. 274, 121072. doi:10.1016/j.saa.2022.121072
Kido, J., Nagai, K., and Okamoto, Y. (1993). Organic electroluminescent devices using lanthanide complexes. J. Alloys Compd. 192, 30–33. doi:10.1016/0925-8388(93)90176-N
Kirsanov, A. V., and Makitra, R. G. (1956). Dichlorides of acylamidophosphoric acids of aromatic series. Zh Obs. Khim. 26, 905–907.
Klink, S. I., Grave, L., Reinhoudt, D. N., van Veggel, F. C. J. M., V Werts, M. H., Geurts, F. A. J., et al. (2000). A systematic study of the photophysical processes in polydentate triphenylene-functionalized Eu3+, Tb3+, Nd3+, Yb3+, and Er3+ complexes. J. Phys. Chem. A 104, 5457–5468. doi:10.1021/jp994286+
Kushida, T. (1973). Energy transfer and cooperative optical transitions in rare-earth doped inorganic materials. I. Transition probability calculation. J. Phys. Soc. Jpn. 34, 1318–1326. doi:10.1143/JPSJ.34.1318
Kuzmina, D. D., Trush, V. A., Kariaka, N. S., Domasevitch, K. V., Sliva, T. Y., Smola, S. S., et al. (2020). New lanthanide complexes with CAPh type ligand dimethyl-N-benzoylamidophosphate and an additional ligand triphenylphosphine oxide. Synthesis, crystal structure, luminescent and thermal properties. Opt. Mater. (Amst). 109, 110233. doi:10.1016/j.optmat.2020.110233
Legendziewicz, J., Oczko, G., Amirkhanov, V., Wiglusz, R., and Ovchynnikov, V. (2000). Synthesis and spectroscopic characteristics of a new class of lanthanide compounds of formulae Ln(HX)3(NO3)3 and Ln(HX)3Cl3. J. Alloys Compd. 300–301, 360–369. doi:10.1016/S0925-8388(99)00766-5
Legendziewicz, J., Oczko, G., Wiglusz, R., and Amirkhanov, V. (2001). Correlation between spectroscopic characteristics and structure of lanthanide phosphoro-azo derivatives of β-diketones. J. Alloys Compd. 323–324, 792–799. doi:10.1016/S0925-8388(01)01147-1
Li, J., Li, H., Yan, P., Chen, P., Hou, G., and Li, G. (2012). Synthesis, crystal structure, and luminescent properties of 2-(2,2,2-Trifluoroethyl)-1-indone lanthanide complexes. Inorg. Chem. 51, 5050–5057. doi:10.1021/ic202473b
Lima, P. P., Sá Ferreira, R. A., Freire, R. O., Almeida Paz, F. A., Fu, L., Alves, S., et al. (2006). Spectroscopic study of a UV-photostable organic-inorganic hybrids incorporating an Eu3+ β-diketonate complex. ChemPhysChem 7, 735–746. doi:10.1002/cphc.200500588
Lima, P. P., Almeida Paz, F. A., Ferreira, R. A. S., de Zea Bermudez, V., and Carlos, L. D. (2009). Ligand-assisted rational design and supramolecular tectonics toward highly luminescent Eu3+-containing Organic−Inorganic hybrids. Chem. Mater. 21, 5099–5111. doi:10.1021/cm901901j
Lima, N. B. D., Gonçalves, S. M. C., Júnior, S. A., and Simas, A. M. (2013). A comprehensive strategy to boost the quantum yield of luminescence of europium complexes. Sci. Rep. 3, 2395. doi:10.1038/srep02395
Lima, G. B. V., Bueno, J. C., da Silva, A. F., Carneiro Neto, A. N., Moura, R. T., Teotonio, E. E. S., et al. (2020). Novel trivalent europium β-diketonate complexes with N-(pyridine-2-yl)amides and N-(pyrimidine-2-yl)amides as ancillary ligands: Photophysical properties and theoretical structural modeling. J. Lumin. 219, 116884. doi:10.1016/j.jlumin.2019.116884
Litsis, O. O., Ovchynnikov, V. A., Scherbatskii, V. P., Nedilko, S. G., Sliva, T. Y., Dyakonenko, V. V., et al. (2015). Lanthanide mixed-ligand complexes of the [Ln(CAPh)3(Phen)] and [LaxEu1−x(CAPh)3(Phen)] (CAPh = carbacylamidophosphate) type. A comparative study of their spectral properties. Dalt. Trans. 44, 15508–15522. doi:10.1039/C5DT02557E
Lunstroot, K., Driesen, K., Nockemann, P., Van Hecke, K., Van Meervelt, L., Görller-Walrand, C., et al. (2009). Lanthanide-doped luminescent ionogels. Dalt. Trans., 298–306. doi:10.1039/B812292J
Lyubov, D. M., Carneiro Neto, A. N., Fayoumi, A., Lyssenko, K. A., Korshunov, V. M., Taydakov, I. V., et al. (2022). Employing three-blade propeller lanthanide complexes as molecular luminescent thermometers: Study of temperature sensing through a concerted experimental/theory approach. J. Mater. Chem. C 10, 7176–7188. doi:10.1039/D2TC01289H
Ma, Y., and Wang, Y. (2010). Recent advances in the sensitized luminescence of organic europium complexes. Coord. Chem. Rev. 254, 972–990. doi:10.1016/j.ccr.2010.02.013
Malta, O. L., and Gonçalves e Silva, F. R. (1998). A theoretical approach to intramolecular energy transfer and emission quantum yields in coordination compounds of rare Earth ions. Spectrochim. Acta Part A Mol. Biomol. Spectrosc. 54, 1593–1599. doi:10.1016/S1386-1425(98)00086-9
Malta, O. L., Brito, H. F., Menezes, J. F. S., e Silva, F. R. G., Alves, S., Farias, F. S., et al. (1997). Spectroscopic properties of a new light-converting device Eu(thenoyltrifluoroacetonate)3 2(dibenzyl sulfoxide). A theoretical analysis based on structural data obtained from a sparkle model. J. Lumin. 75, 255–268. doi:10.1016/S0022-2313(97)00107-5
Malta, O. L., Brito, H. F., Menezes, J. F. S., Silva, F. R. G. E., Donega, C. D., and Alves, S. (1998). Experimental and theoretical emission quantum yield in the compound Eu(thenoyltrifluoroacetonate)3.2(dibenzyl sulfoxide). Chem. Phys. Lett. 282, 233–238. doi:10.1016/S0009-2614(97)01283-9
Malta, O. L. (1982). A simple overlap model in lanthanide crystal-field theory. Chem. Phys. Lett. 87, 27–29. doi:10.1016/0009-2614(82)83546-X
Malta, O. L. (1997). Ligand—rare-Earth ion energy transfer in coordination compounds. A theoretical approach. J. Lumin. 71, 229–236. doi:10.1016/S0022-2313(96)00126-3
Malta, O. L. (2008). Mechanisms of non-radiative energy transfer involving lanthanide ions revisited. J. Non. Cryst. Solids. 354, 4770–4776. doi:10.1016/j.jnoncrysol.2008.04.023
Maron, L., and Eisenstein, O. (2001). DFT modeling of ligands in lanthanide chemistry: Is Ln[N(SiH3)2]3 a model for Ln[N(SiMe3)2]3? New J. Chem. 25, 255–258. doi:10.1039/b005266n
Mason, S. F., Peacock, R. D., and Stewart, B. (1974). Dynamic coupling contributions to the intensity of hypersensitive lanthanide transitions. Chem. Phys. Lett. 29, 149–153. doi:10.1016/0009-2614(74)85001-3
Melby, L. R., Rose, N. J., Abramson, E., and Caris, J. C. (1964). Synthesis and fluorescence of some trivalent lanthanide complexes. J. Am. Chem. Soc. 86, 5117–5125. doi:10.1021/ja01077a015
Meshkova, S. B., Kuz’min, V. E., Shapiro, Y. E., Topilova, Z. M., V Yudanova, I., V Bol’shoj, D., et al. (2000). Relationship between the analytical properties of lanthanide(3) β-diketonates and the nature of ligands. Zhurnal Anal. Khimii. 55, 118–1214. Available at: http://inis.iaea.org/search/search.aspx?orig_q=RN:32007755.
Monteiro, J. H. S. K., Adati, R. D., Davolos, M. R., Vicenti, J. R. M., and Burrow, R. A. (2011). Correlation between structural data and spectroscopic studies of a new β-diketonate complex with trivalent europium and gadolinium. New J. Chem. 35, 1234. doi:10.1039/c0nj00831a
Moura, R. T., Carneiro Neto, A. N., Longo, R. L., and Malta, O. L. (2016). On the calculation and interpretation of covalency in the intensity parameters of 4f-4f transitions in Eu3+ complexes based on the chemical bond overlap polarizability. J. Lumin. 170, 420–430. doi:10.1016/j.jlumin.2015.08.016
Moura, R. T., Carneiro Neto, A. N., Aguiar, E. C., Santos-, C. V., de Lima, E. M., Faustino, W. M., et al. (2021). (INVITED) JOYSpectra: A web platform for luminescence of lanthanides. Opt. Mater. X. 11, 100080. doi:10.1016/j.omx.2021.100080
Moura, R. T., Oliveira, J. A., Santos, I. A., Lima, E. M., Carlos, L. D., Aguiar, E. C., et al. (2021). Theoretical evidence of the singlet predominance in the intramolecular energy transfer in ruhemann’s purple Tb(III) complexes. Adv. Theory Simulations 4, 2000304. doi:10.1002/adts.202000304
Moura, R. T., Quintano, M., Santos-, C. V., Albuquerque, V. A. C. A., Aguiar, E. C., Kraka, E., et al. (2022). Featuring a new computational protocol for the estimation of intensity and overall quantum yield in lanthanide chelates with applications to Eu(III) mercapto-triazole Schiff base ligands. Opt. Mater. X. 16, 100216. doi:10.1016/j.omx.2022.100216
Moura, J. L., Costa, I. F., Santos, P. R. S., Silva, I. F., Moura, R. T., Carneiro Neto, A. N., et al. (2022). Enhancing the luminescence of Eu(III) complexes with the ruthenocene organometallic unit as ancillary ligand. Inorg. Chem. 61, 13510–13524. doi:10.1021/acs.inorgchem.2c02115
Nakajima, A., Nakanishi, T., Kitagawa, Y., Seki, T., Ito, H., Fushimi, K., et al. (2016). Hyper-stable organo-EuIII luminophore under high temperature for photo-industrial application. Sci. Rep. 6, 24458. doi:10.1038/srep24458
Nigoghossian, K., Bouvet, B., Félix, G., Sene, S., Costa, L., Milhet, P.-E., et al. (2022). Magneto-induced hyperthermia and temperature detection in single iron oxide core-silica/Tb3+/Eu3+(acac) shell nano-objects. Nanomaterials 12, 3109. doi:10.3390/nano12183109
Nockemann, P., Beurer, E., Driesen, K., Van Deun, R., Van Hecke, K., Van Meervelt, L., et al. (2005). Photostability of a highly luminescent europium β-diketonate complex in imidazolium ionic liquids. Chem. Commun., 4354–4356. doi:10.1039/b506915g
Oczko, G., Legendziewicz, J., Trush, V., and Amirkhanov, V. (2003). X-Ray analysis and excited state dynamics in a new class of lanthanide mixed chelates of the type LnPhβ3·Phen (Ln = Sm, Eu, Gd, Tb). New J. Chem. 27, 948–956. doi:10.1039/B211044J
Ofelt, G. S. (1962). Intensities of crystal spectra of rare-earth ions. J. Chem. Phys. 37, 511–520. doi:10.1063/1.1701366
Pagnot, T., Audebert, P., and Tribillon, G. (2000). Photostability study of europium dibenzolymethide embedded in polystyrene thin films with high concentration. Chem. Phys. Lett. 322, 572–578. doi:10.1016/S0009-2614(00)00478-4
Peijzel, P. S., Meijerink, A., Wegh, R. T., Reid, M. F., and Burdick, G. W. (2005). A complete energy level diagram for all trivalent lanthanide ions. J. Solid State Chem. 178, 448–453. doi:10.1016/j.jssc.2004.07.046
Petoud, S., Bünzli, J.-C. G., Glanzman, T., Piguet, C., Xiang, Q., and Thummel, R. P. (1999). Influence of charge-transfer states on the Eu(III) luminescence in mononuclear triple helical complexes with tridentate aromatic ligands. J. Lumin. 82, 69–79. doi:10.1016/S0022-2313(99)00015-0
Petrov, A. I., Lutoshkin, M. A., and Taydakov, I. V. (2015). Aqueous complexation of YIII, LaIII, NdIII, SmIII, EuIII, and YbIII with some heterocyclic substituted β-diketones. Eur. J. Inorg. Chem. 2015, 1074–1082. doi:10.1002/ejic.201403052
Pham, Y. H., Trush, V. A., Amirkhanov, V. M., and Gawryszewska, P. (2017). Structural and spectroscopic study of the europium complex with N -(diphenylphosphoryl)pyrazine-2-carboxamide. Opt. Mater. (Amst). 74, 197–200. doi:10.1016/j.optmat.2017.04.031
Pham, Y. H., Trush, V. A., Carneiro Neto, A. N., Korabik, M., Sokolnicki, J., Weselski, M., et al. (2020). Lanthanide complexes with N -phosphorylated carboxamide as UV converters with excellent emission quantum yield and single-ion magnet behavior. J. Mater. Chem. C 8, 9993–10009. doi:10.1039/D0TC01445A
Piguet, C., and Bünzli, J.-C. G. (1999). Mono- and polymetallic lanthanide-containing functional assemblies: A field between tradition and novelty. Chem. Soc. Rev. 28, 347–358. doi:10.1039/a804240c
Piñol, R., Zeler, J., Brites, C. D. S., Gu, Y., Téllez, P., Carneiro Neto, A. N., et al. (2020). Real-time intracellular temperature imaging using lanthanide-bearing polymeric micelles. Nano Lett. 20, 6466–6472. doi:10.1021/acs.nanolett.0c02163
Puchalska, M., Turowska-Tyrk, I., Trush, V., and Legendziewicz, J. (2008). Structural characteristic and luminescence properties of first known example of a pair of europium(III) complexes of phosphoroazo-derivative of β-diketone with inner and both inner and outer sphere 2,2′-bipyridine. J. Alloys Compd. 451, 264–269. doi:10.1016/j.jallcom.2007.04.183
Ramalho, J. F. C. B., Carneiro Neto, A. N., Carlos, L. D., André, P. S., and Ferreira, R. A. S. (2022). “Lanthanides for the new generation of optical sensing and Internet of Things,” in Handbook of the physics and chemistry rare earths. Editors J.-C. G. Bünzli, and V. K. Pecharsky (Elsevier B.V), 31–128. doi:10.1016/bs.hpcre.2021.12.001
Reddy, M. L. P., Divya, V., and Pavithran, R. (2013). Visible-light sensitized luminescent europium(III)-β-diketonate complexes: Bioprobes for cellular imaging. Dalt. Trans. 42, 15249. doi:10.1039/c3dt52238e
Sá Ferreira, R. A., Nobre, S. S., Granadeiro, C. M., Nogueira, H. I. S., Carlos, L. D., and Malta, O. L. (2006). A theoretical interpretation of the abnormal 5D0→7F4 intensity based on the Eu3+ local coordination in the Na9[EuW10O36]·14H2O polyoxometalate. J. Lumin. 121, 561–567. doi:10.1016/j.jlumin.2005.12.044
Salerno, E. V., Carneiro Neto, A. N., Eliseeva, S. V., Hernández-Rodríguez, M. A., Lutter, J. C., Lathion, T., et al. (2022). Tunable optical molecular thermometers based on metallacrowns. J. Am. Chem. Soc. 144, 18259–18271. doi:10.1021/jacs.2c04821
Santos, P. R. S., Pereira, D. K. S., Costa, I. F., Silva, I. F., Brito, H. F., Faustino, W. M., et al. (2020). Experimental and theoretical investigations of the [Ln(β-dik)(NO3)2(phen)2]⋅H2O luminescent complexes. J. Lumin. 226, 117455. doi:10.1016/j.jlumin.2020.117455
Sato, S., and Wada, M. (1970). Relations between intramolecular energy transfer efficiencies and triplet state energies in rare earth β-diketone chelates. Bull. Chem. Soc. Jpn. 43, 1955–1962. doi:10.1246/bcsj.43.1955
Shi, J., Hou, Y., Chu, W., Shi, X., Gu, H., Wang, B., et al. (2013). Crystal structure and highly luminescent properties studies of bis-β-diketonate lanthanide complexes. Inorg. Chem. 52, 5013–5022. doi:10.1021/ic302726z
Silva, F. A., Nascimento, H. A., Pereira, D. K. S., Teotonio, E. E. S., Brito, H. F., Felinto, M. C. F. C., et al. (2013). Energy transfer processes in Tb(III)-Dibenzoylmethanate complexes with phosphine oxide ligands. J. Braz. Chem. Soc. doi:10.5935/0103-5053.20130073
Singh, K., Boddula, R., and Vaidyanathan, S. (2017). Versatile luminescent europium(III)−β-Diketonate-imidazo-bipyridyl complexes intended for white LEDs: A detailed photophysical and theoretical study. Inorg. Chem. 56, 9376–9390. doi:10.1021/acs.inorgchem.7b01565
Smentek, L., and Kędziorski, A. (2010). Efficiency of the energy transfer in lanthanide-organic chelates; spectral overlap integral. J. Lumin. 130, 1154–1159. doi:10.1016/j.jlumin.2010.02.013
Smentek, L. (1998). Theoretical description of the spectroscopic properties of rare Earth ions in crystals. Phys. Rep. 297, 155–237. doi:10.1016/S0370-1573(97)00077-X
Sokolnicki, J., Legendziewicz, J., Amirkhanov, W., Ovchinnikov, V., Macalik, L., and Hanuza, J. (1999). Comparative optical studies of lanthanide complexes with three types of phosphoro-azo derivatives of β-diketones. Spectrochim. Acta Part A Mol. Biomol. Spectrosc. 55, 349–367. doi:10.1016/S1386-1425(98)00193-0
Songzhu, L., Xiangting, D., Jinxian, W., Guixia, L., Wenshen, Y., and Ruokun, J. (2010). Fabrication of Eu(III) complex doped nanofibrous membranes and their oxygen-sensing properties. Spectrochim. Acta Part A Mol. Biomol. Spectrosc. 77, 885–889. doi:10.1016/j.saa.2010.08.025
Stilinović, V., and Kaitner, B. (2009). Two tetrakis(benzoylacetonato)lanthanide species: Synthesis, characterization and structures of tetrakis(benzoylacetonato)cerium(IV) and triethylammonium tetrakis(benzoylacetonato)lanthanate(III) tetrahydrate. J. Coord. Chem. 62, 2698–2708. doi:10.1080/00958970902912365
Sun, L., Qiu, Y., Liu, T., Feng, J., Deng, W., and Shi, L. (2015). Visible-near-infrared luminescent lanthanide ternary complexes based on beta-diketonate using visible-light excitation. Luminescence 30, 1071–1076. doi:10.1002/bio.2860
Suta, M., and Meijerink, A. (2020). A theoretical framework for ratiometric single ion luminescent thermometers—thermodynamic and kinetic guidelines for optimized performance. Adv. Theory Simul. 3, 2000176. doi:10.1002/adts.202000176
Tang, S.-F., Lorbeer, C., Wang, X., Ghosh, P., and Mudring, A.-V. (2014). Highly luminescent salts containing well-shielded lanthanide-centered complex anions and bulky imidazolium countercations. Inorg. Chem. 53, 9027–9035. doi:10.1021/ic500979p
Tanner, P. A., Zhou, L., Duan, C., and Wong, K.-L. (2018). Misconceptions in electronic energy transfer: Bridging the gap between chemistry and physics. Chem. Soc. Rev. 47, 5234–5265. doi:10.1039/C8CS00002F
Tanner, P. A., Thor, W., Zhang, Y., and Wong, K.-L. (2022). Energy transfer mechanism and quantitative modeling of rate from an antenna to a lanthanide ion. J. Phys. Chem. A 126, 7418–7431. doi:10.1021/acs.jpca.2c03965
Tanner, P. A. (2013). Some misconceptions concerning the electronic spectra of tri-positive europium and cerium. Chem. Soc. Rev. 42, 5090–5101. doi:10.1039/c3cs60033e
Teotonio, E. E. S., Silva, F. A., Pereira, D. K. S., Santo, L. M., Brito, H. F., Faustino, W. M., et al. (2010). Luminescence enhancement of the Tb(III) ion with the thenoyltrifluoroacetonate ligand acting as an efficient sensitizer. Inorg. Chem. Commun. 13, 1391–1395. doi:10.1016/j.inoche.2010.07.043
Teotonio, E. E. S., Brito, H. F., de Sá, G. F., Felinto, M. C. F. C., Santos, R. H. A., Fuquen, R. M., et al. (2012). Structure and luminescent investigation of the Ln(III)–β-diketonate complexes containing tertiary amides. Polyhedron 38, 58–67. doi:10.1016/j.poly.2012.02.010
Topilova, Z. M., Meshkova, S. B., V Bol’shoj, D., Lozinskij, M. O., and Shapiro, Y. E. (1997). IR-luminescence of ytterbium ions in complexes with β-fluorodiketones. Zhurnal Neorg. Khimii. 42, 99–104. Available at: http://inis.iaea.org/search/search.aspx?orig_q=RN:28048261.
Van Vleck, J. H. (1937). The puzzle of rare-Earth spectra in solids. J. Phys. Chem. 41, 67–80. doi:10.1021/j150379a006
Weissman, S. I. (1942). Intramolecular energy transfer the fluorescence of complexes of Europium. J. Chem. Phys. 10, 214–217. doi:10.1063/1.1723709
Wen, J., Reid, M. F., Ning, L., Zhang, J., Zhang, Y., Duan, C. K., et al. (2014). Ab-initio calculations of Judd-Ofelt intensity parameters for transitions between crystal-field levels. J. Lumin. 152, 54–57. doi:10.1016/j.jlumin.2013.10.055
Werts, M. H. V., Duin, M. A., Hofstraat, J. W., and Verhoeven, J. W. (1999). Bathochromicity of Michler’s ketone upon coordination with lanthanide(III) β-diketonates enables efficient sensitisation of Eu3+ for luminescence under visible light excitation. Chem. Commun., 799–800. doi:10.1039/a902035g
Wu, S. L., Wu, Y. L., and Yang, Y. S. (1992). Rare Earth(iii) complexes with indole-derived acetylacetones I: Synthesis and characterization of rare Earth(iii) complexes. J. Alloys Compd. 180, 391–397. doi:10.1016/0925-8388(92)90407-Z
Wu, S. L., Wu, Y. L., and Yang, Y. S. (1992). Rare Earth(iii) complexes with indole-derived acetylacetones II. luminescent intensity for europium(iii) and terbium(iii) complexes. J. Alloys Compd. 180, 399–402. doi:10.1016/0925-8388(92)90408-2
Yakovlev, O. O., Kariaka, N. S., Trush, V. A., Smola, S. S., Siczek, M., and Amirkhanov, V. M. (2018). Luminescent properties and structure of new CAPh-based lanthanide complexes [LnL3Q], containing additional bis-heterocyclic aromatic ligand-antenna 2-(1,3,4-oxadiazole-2-yl) pyridine. Opt. Mater. (Amst). 75, 459–464. doi:10.1016/j.optmat.2017.10.044
Yanagisawa, K., Nakanishi, T., Kitagawa, Y., Seki, T., Akama, T., Kobayashi, M., et al. (2015). Seven-coordinate luminophores: Brilliant luminescence of lanthanide complexes with C3v geometrical structures. Eur. J. Inorg. Chem. 2015, 4769–4774. doi:10.1002/ejic.201500820
Yang, Y. S., Gong, M. L., Li, Y. Y., Lei, H. Y., and Wu, S. L. (1994). Effects of the structure of ligands and their Ln3+ complexes on the luminescence of the central Ln3+ ions. J. Alloys Compd. 207–208, 112–114. doi:10.1016/0925-8388(94)90189-9
Yang, X.-D., Chang, W.-B., and Ci, Y.-X. (1994). Time-resolved fluorescence immunoassay with measurement of a europium chelate in solution: Dissociation conditions and application for determination of cortisol. Anal. Chem. 66, 2590–2594. doi:10.1021/ac00087a026
Yao, J., López-Peña, G., Lifante, J., Iglesias-de la Cruz, M. C., Marin, R., Martín Rodríguez, E., et al. (2023). (INVITED)Adjustable near-infrared fluorescence lifetime emission of biocompatible rare-Earth-doped nanoparticles for in vivo multiplexing. Opt. Mater. X. 17, 100225. doi:10.1016/j.omx.2022.100225
Yi, X., Bernot, K., Pointillart, F., Poneti, G., Calvez, G., Daiguebonne, C., et al. (2012). A luminescent and sublimable DyIII-based single-molecule magnet. Chem. - A Eur. J. 18, 11379–11387. doi:10.1002/chem.201201167
Yuster, P., and Weissman, S. I. (1949). Effects of perturbations on phosphorescence: Luminescence of metal organic complexes. J. Chem. Phys. 17, 1182–1188. doi:10.1063/1.1747140
Zhao, W., Shao, H., Yu, G., Hou, Y., and Wang, S. (2018). The coordination and luminescence of the Eu(III) complexes with the polymers (PMMA, PVP). Polym. (Basel) 10, 508. doi:10.3390/polym10050508
Znovyak, K. O., Ovchinnikov, V. A., Moroz, O. V., Plum, T. Y., Shcherbatskyi, V. P., Polyakov, O. I., et al. (2009). Synthesis and luminescent properties of lanthanide coordination compounds based on N,N′-dipyrolidine-N′′-trichloroacetylphosphortriamide and dimetyl-N-trichloroacetylamidophosphate (in Ukr). Rep. Natl. Acad. Sci. Ukr. 6, 143–149. Available at: http://dspace.nbuv.gov.ua/xmlui/handle/123456789/8661.
Keywords: lanthanide, luminescence, carbacylamidophosphates, β-diketone, thermal gravimetric analysis, photosensitisation, energy transfer
Citation: Kariaka NS, Lipa A, Carneiro Neto AN, Malta OL, Gawryszewska P and Amirkhanov VM (2023) Eu3+ and Tb3+ coordination compounds with phenyl-containing carbacylamidophosphates: comparison with selected Ln3+ β-diketonates. Front. Chem. 11:1188314. doi: 10.3389/fchem.2023.1188314
Received: 17 March 2023; Accepted: 03 May 2023;
Published: 15 May 2023.
Edited by:
Feng Luo, East China University of Technology, ChinaReviewed by:
Yuichi Kitagawa, Hokkaido University, JapanUlrich Kynast, Muenster University of Applied Sciences, Germany
Copyright © 2023 Kariaka, Lipa, Carneiro Neto, Malta, Gawryszewska and Amirkhanov. This is an open-access article distributed under the terms of the Creative Commons Attribution License (CC BY). The use, distribution or reproduction in other forums is permitted, provided the original author(s) and the copyright owner(s) are credited and that the original publication in this journal is cited, in accordance with accepted academic practice. No use, distribution or reproduction is permitted which does not comply with these terms.
*Correspondence: Volodymyr M. Amirkhanov, dl9hbWlya2hhbm92QGtudS51YQ==