- 1Key Laboratory Under Construction for Volatile Organic Compounds Controlling of Jiangsu Province, School of Environmental Science and Engineering, Yancheng Institute of Technology, Yancheng, China
- 2School of Chemistry and Chemical Engineering, Jiangsu University, Zhengjiang, China
Cu1In2Zr4-O-C catalysts with Cu2In alloy structure were prepared by using the sol–gel method. Cu1In2Zr4-O-PC and Cu1In2Zr4-O-CP catalysts were obtained from plasma-modified Cu1In2Zr4-O-C before and after calcination, respectively. Under the conditions of reaction temperature 270°C, reaction pressure 2 MPa, CO2/H2 = 1/3, and GHSV = 12,000 mL/(g h), Cu1In2Zr4-O-PC catalyst has a high CO2 conversion of 13.3%, methanol selectivity of 74.3%, and CH3OH space-time yield of 3.26 mmol/gcat/h. The characterization results of X-ray diffraction (XRD), scanning electron microscopy (SEM), and temperature-programmed reduction chemisorption (H2-TPR) showed that the plasma-modified catalyst had a low crystallinity, small particle size, good dispersion, and excellent reduction performance, leading to a better activity and selectivity. Through plasma modification, the strong interaction between Cu and In in Cu1In2Zr4-O-CP catalyst, the shift of Cu 2p orbital binding energy to a lower position, and the decrease in reduction temperature all indicate that the reduction ability of Cu1In2Zr4-O-CP catalyst is enhanced, and the CO2 hydrogenation activity is improved.
1 Introduction
The catalysts for CO2 hydrogenation to methanol mainly include Cu-based catalysts and other metal oxide catalysts (Bowker et al., 2022; Yan et al., 2022). Cu-based catalysts have been most widely studied. Among the other metal oxide catalysts, the In2O3 and ZrO2 catalysts show high activity and selectivity, due to their good CO2 adsorption and activation performance (Wu et al., 2021; Tada et al., 2022). Inspired by this, we prepared Cu1In2Zr4-O catalyst with Cu2In alloy structure by using the sol–gel method, which exhibited an excellent performance for CO2 hydrogenation to CH3OH. The CO2 conversion and methanol selectivity were 12.8% and 72.8%, respectively at 270°C, 2 MPa, and 12,000 mL·(g·h)−1. The special structure of Cu2In alloy strengthened the interaction between In and Cu species, further readjusted good dispersion, high surface area, and the adsorption and reduction properties of the catalyst. Briefly, Cu2In alloy is a key factor for improving catalytic performance (Gao et al., 2020).
On the basis of the Cu1In2Zr4-O catalyst, how can we further improve the catalytic performance? The preparation and modification methods of catalysts are worthy of special consideration. Different preparation and modification methods lead to changes in the particle size of metal particles, the dispersion of active components, the crystallinity of the catalyst, and the interaction between each component, which ultimately leads to differences in catalytic activity (Cg et al., 2021; Lasobras et al., 2021). Plasma modification of the catalyst results in better dispersion, larger specific surface area, and more lattice defects (Liu et al., 2016; Bagherzadeh and Haghighi, 2017).
Zeng et al. (2022) prepared NiMnAl-LDO (layered double oxides) catalysts for CO2 methanation. Solution plasma treatment was used to improve the dispersion, generate oxygen defects, and enhance the adsorption sites, improving the low-temperature activity and stability of the catalyst. Kierzkowska-Pawlak et al. (2017) investigated CO2 methanation on nanoscale metal oxides carried out on wire gauzes (FeCrAl). The catalysts were synthetized by plasma-assisted chemical vapor deposition. The plasma deposition promoted the generation of the specific nanostructure of metal oxides, which was responsible for ascendant catalytic activity. Han et al. (2020) prepared CuZnO-ZrO2 by using a co-precipitation method. The catalyst was pretreated by the glow discharge plasma before and after calcination. After treated with plasma, the catalyst showed a lower crystallinity and a better dispersion, and CO2 conversion increased by 38.9%.
In this work, the plasma-improved sol–gel method was used to prepare the Cu1In2Zr4-O catalyst with Cu2In alloy structure. Because the Cu1In2Zr4-O catalyst with Cu2In alloy structure prepared using the sol–gel method had exhibited good dispersion and excellent catalytic performance, the promotion of plasma modification may not be particularly significant. However, it still makes sense for the catalyst. The structure, chemical property, and catalytic activity of the catalysts before and after plasma treatment were systematically studied. In addition, the process parameters of the catalytic hydrogenation reaction were also optimized.
2 Experimental methods
2.1 Materials
Cu(NO3)2·3H2O, In(NO3)3·4.5H2O, Zr(NO3)4·5H2O, and C6H8O7·H2O were purchased from Sinopharm Chemical Reagent Co., Ltd.
2.2 Preparation of catalysts
Cu1In2Zr4-O-C catalysts with Cu2In alloy structure were prepared by using the sol–gel method, as described in our previous research (Gao et al., 2020). Cu1In2Zr4-O-CP and Cu-In/ZrO2-PC catalysts with Cu2In alloy structure were prepared by using the plasma-improved sol–gel method. The specific operation steps are as follows: first, the catalyst precursor was prepared by using the sol–gel method, which was divided into two parts after grinding (labeled as sample 1 and sample 2, respectively). Next, the No. 1 sample was roasted at 350°C for 4 h in a tubular furnace and then treated by plasma for 15 min. The obtained catalyst was recorded as Cu-In/ZrO2-CP. Finally, the No. 2 sample was first treated by plasma and then calcined, and other conditions remained the same to obtain the Cu-In/ZrO2-PC catalyst.
2.3 Characterization techniques
The XRD patterns were obtained on a PANalytical X’Pert3 Powder with Cu K α (λ = 0.154 nm). The working voltage, current, and scanning speed were 40 kV, 100 mA, and 8°/min, respectively. The specific surface area, pore volume, and pore size of catalytic materials were measured using Beckman Coulter SA3100. The composition of catalysts was measured using an inductively coupled plasma optical emission spectrometer (ICP-OES, Agilent 730). The morphology was observed using a Nova NanoSEM 450. The field emission operating voltage and current were 5 kV and 10 mA, respectively. The XPS atlas was analyzed using an ESCALAB 250Xi X-ray spectrometer. The H2-TPR and H2-TPD curves were collected using AutoChem II 2920, and the test temperature range was 50–800°C and 50–600°C, respectively. The plasma instrument used was the MVP-401 glow discharge plasma instrument produced by Kunshan Sokunlai Electromechanical Technology Co., Ltd.
2.4 Catalytic activity evaluation
The catalytic activity test was carried out on an HP-WF51 fixed bed reactor (stainless steel reactor with 10 mm inner diameter), and the catalyst loading amount was the mixture of 0.5 g of the catalyst (20–40 mesh) and 0.5 g of quartz sand (20–40 mesh). The reaction was carried out at 270°C, pressure 2 MPa, feed gas component ratio V (H2): V (CO2): V (N2) = 69:23:8, and space velocity 12,000 mL/(h · g). Before the reaction, the catalyst was reduced in a V (H2): V (N2) = 10:40 mixture in advance. The temperature was 350°C, and the pressure was 0.1 MPa. Afterward, when the temperature dropped to 270°C, the gas was switched to the feed gas component to start the reaction. The reaction products were analyzed by gas chromatography, and the concentration of CO2 and CO was detected using a TCD detector (TDX-01 was used for filling the column). The FID detector was used to detect hydrocarbon gases such as methanol (Porapak Q was used for the capillary column), and the corrected area normalization method was used to quantitatively analyze the gas concentration in tail gas.
3 Results and discussion
3.1 XRD analysis
Figure 1 shows the XRD spectra of Cu1In2Zr4-O-PC, Cu1In2Zr4-O-C, and Cu1In2Zr4-O-CP catalysts before and after reduction. The diffraction peaks at 2θ of 30.3°, 35.3°, 50.4°, and 60.2° belong to (011), (110), (112), and (121) crystal planes of t-ZrO2, respectively (Jcpds-050-1089) (Pei et al., 2022), and the diffraction peaks of In2O3 are located at 2θ of 21.5°, 35.5°, 51.0°, and 60.7° belong to the (211), (400), (440), and (622) crystal planes of the In2O3, respectively (Jcpds-06-0416) (Zafar et al., 2022). The diffraction peak intensity of Cu1In2Zr4-O-PC and Cu1In2Zr4-O-CP catalysts before reduction is significantly lower than that of Cu1In2Zr4-O-C catalysts, indicating that plasma modification reduces the crystallinity of the catalyst (Zhang et al., 2010). No CuO diffraction peak is found in all samples before reduction, indicating that CuO in all catalysts is mainly dispersed on the support surface in the highly dispersed or amorphous form (Azenha et al., 2017). In the reduced Cu1In2Zr4-O-PC, Cu1In2Zr4-O-C, and Cu1In2Zr4-O-CP catalysts, the t-ZrO2 diffraction peaks of the catalyst after plasma modification have no obvious change, while the diffraction peak intensity of Cu2In has decreased significantly, and the diffraction peak has widened. The reduced sample has no diffraction peak of metal Cu and In, indicating that the reduced Cu and In combine to form the Cu2In alloy phase. The XRD results show that the crystallinity of the catalyst after plasma modification is generally lower than that after direct calcination, and the particle size of the catalyst is reduced, thus improving the dispersion of the catalyst.
3.2 BET and ICP analysis
Figure 2 shows the N2 adsorption and desorption curves and pore size distribution of three catalyst samples. As shown in Figure 2A, according to the IUPAC classification, all catalysts show type IV isotherms with a H2 hysteresis loop, indicating that all catalysts have the characteristics of mesoporous materials (Witoon et al., 2018a). As shown in Figure 2B, all samples have a maximum pore size distribution of about 3.8 nm, indicating that the pore size of the catalyst sample is mainly mesoporous, with fewer micropores and macropores (Li et al., 2019). The N2 adsorption–desorption curves and the most probable pore size distribution of Cu1In2Zr4-O-PC, Cu1In2Zr4-O-C, and Cu1In2Zr4-O-CP catalysts are basically consistent, indicating that plasma modification has little effect on the physical properties of the catalysts. Table 1 shows the physicochemical properties of the catalysts. The specific surface area of the Cu1In2Zr4-O-CP catalyst (115.89 m2/g) is lower than that of the untreated catalyst (122.38 m2/g). The reason may be that the duration of plasma treatment is too long or the temperature is too high, which leads to the increase of the crystallinity of the catalyst, the aggregation of the catalyst, and the reduction of the specific surface area. The specific surface area of Cu1In2Zr4-O-PC catalyst (119.49 m2/g) has little change from that of the untreated catalyst (122.38 m2/g); that is, plasma modification has little effect on the specific surface area of the catalyst, and the pore volume of the catalyst after plasma modification and that of the untreated catalyst have no change basically. The molar percentage of Cu, In, and Zr in the three samples is in the range of 14.26%–14.29%, 28.49%–28.61%, and 57.11%–57.24%, respectively. The Cu/In/Zr molar ratio is very close to the theoretical value of 1:2:4.
3.3 XPS analysis
The XPS spectra of Cu 2p orbitals of different catalysts are shown in Figure 3A. The binding energies of Cu 2p3/2 and Cu 2p1/2 orbitals are approximately 932.8 and 952.8 eV, respectively, indicating that the Cu element in the three reduced catalysts exists in the Cu0 form, while the catalyst without plasma treatment has a shoulder peak at 934.5 eV, indicating that the Cu element in the untreated catalyst also exists in the Cu2+ form (Jiang et al., 2015). However, the catalyst after plasma treatment does not have a Cu2+ peak, and the binding energy at the Cu 2p orbit of the Cu1In2Zr4-O-CP catalyst is approximately 0.3 eV which is lower than that of the catalyst Cu 2p without plasma treatment, indicating that the density of the electron cloud around the Cu 2p orbit of the catalyst after plasma modification changed, thus causing chemical changes in elements and enhancing the interaction between Cu and In. Therefore, the Cu1In2Zr4-O-CP catalyst shows a good catalytic activity (Zhang et al., 2010). As shown in Figure 3B, the binding energies at 443.8 eV and 451.4 eV in the XPS spectrum of the In 3d orbit correspond to the binding energies at In 3d5/2 and In 3d3/2, respectively. The binding energies at the In 3d orbit of the Cu1In2Zr4-O-CP catalyst shift to the lower binding energies, indicating that the chemical environment and energy state around the In 3d orbit of the catalyst after plasma treatment have changed compared with those of the untreated catalyst sample. Figure 3C shows the XPS spectrum of the Zr element on the 3d orbit. The Zr 3d5/2 and Zr 3d3/2 orbital binding energies of the three catalysts are 181.6 and 184.0 eV, respectively. There is no significant difference in the binding energies of the three catalysts on the Zr 3d orbit, indicating that Zr elements of all the catalysts exist in the Zr4+ form, and the chemical environment and energy state around the Zr 3d orbit have not changed. Figure 4D shows the O1s spectra of the three catalysts. The fitted O1s spectra are composed of two asymmetric peaks, proving that there are two different O types on the catalyst surface. Among them, 529.5 eV at low binding energy belongs to lattice oxygen, and 531.0 eV at high binding energy belongs to adsorbed oxygen. As can be seen from the figure, the content of adsorbed oxygen is lower than that of lattice oxygen.
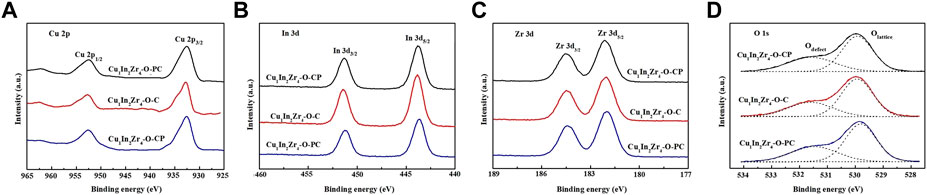
FIGURE 3. XPS patterns of the catalysts after reduction. (A) Cu 2p, (B) In 3d, (C) Zr 3d and (D) O1s.
3.4 SEM analysis
The SEM diagrams of Cu1In2Zr4-O-C and Cu1In2Zr4-O-CP catalysts after reduction are shown in Figure 4. Figures 4A, C show the catalyst morphology at 30 μm scale. The morphology of both catalysts belongs to an irregular blocky structure. Figures 4B, D correspond to the enlarged view of Figures 4A, C, respectively. From Figures 4A, C, it is observed that the Cu2In structure of the catalyst after reduction is composed of spherical particles of different sizes. The particle size of Cu2In particles in the Cu1In2Zr4-O-C catalyst after reduction is large, and Cu2In particles are unevenly dispersed on the support. After reduction, the particle size of Cu2In alloy in the Cu1In2Zr4-O-CP catalyst decreases, and the dispersion on the carrier is uniform. The catalyst modified by plasma can reduce the particle size of the active component, improve the dispersion of the active component, and therefore improve the catalytic activity of the catalyst (Sajjadi and Haghighi, 2018).
3.5 H2-TPR analysis
H2-TPR was used to explore the reduction ability of the catalyst after plasma modification, and the results are shown in Figure 5. All three catalysts have a strong H2 consumption peak in the range of 150°C–300°C, which is attributed to the consumption of CuO to H2. The multiple peaks in the range of 300°C–800°C correspond to the H2 consumption of dispersed and lattice In2O3, respectively (Li et al., 2022). It can be clearly observed that the H2 consumption peaks of the three catalysts CuO are asymmetric, which can be fitted into α and β peaks. The α peak belongs to the reduction of highly dispersed CuO on the surface of the catalyst carrier, while the β peak belongs to the reduction peak of CuO embedded in ZrO2 or In2O3 lattice (Ezeh et al., 2018). The calculated amount of H2 consumption of three catalysts listed in Table 2 is within the range of 76.4–76.6 mL, which slightly differs from each other. However, the peak shapes of the three samples are significantly different. There are two distinct peaks in the H2-TPR spectrum of Cu1In2Zr4-O-C, which overlap with each other but do not overlap at the top of the peaks. The top of the two peaks of Cu1In2Zr4-O-CP overlaps, while those of Cu1In2Zr4-O-PC almost completely overlap, appearing to have only one peak. The α peak difference of Cu1In2Zr4-O-PC and Cu1In2Zr4-O-CP is not significant (1°C), but there is a significant difference (5.3°C) in the β peaks. The reduction peak of bulk CuO has not been found, indicating that CuO in the three catalysts mainly exists in highly dispersed and lattice forms, which is consistent with the XRD results. Compared with the reduction temperature of Cu1In2Zr4-O-PC and Cu1In2Zr4-O-CP, the reduction temperature of Cu1In2Zr4-O-PC is the lowest, meaning that the catalyst treated by plasma reduces the reduction temperature of CuO, enhances the reduction ability of the catalyst, and shows good catalytic activity (Chen et al., 2019).
3.6 H2-TPD analysis
The H2-TPD spectra of the three catalysts are shown in Figure 6. There is an asymmetric H2 desorption peak composed of α and β peaks in the range of 50°C–600°C. The peak at lower temperature (α peak) belongs to highly dispersed metal copper, and the peak at higher temperature (β peak) is attributed to massive metal copper and lattice metal oxides (In2O3 and ZrO2) (Witoon et al., 2018b). After plasma treatment, both α and β peaks shift to lower temperature, and the α and β peak shift of Cu1In2Zr4-O-PC is the largest, indicating that the catalyst can desorb more H2 at lower temperature (Lu et al., 2020). As listed in Table 2, the amount of desorbed H2 of Cu1In2Zr4-O-PC is 114.7 mL, which is significantly higher than that of Cu1In2Zr4-O-C (91.5 mL). It further explains that plasma modification can produce more defect sites, increase the adsorption active sites of hydrogen on the surface of the catalyst, and enhance the adsorption capacity of H2, which can activate more H2 in the reactant and enhance the catalytic performance of CO2 hydrogenation to methanol. Therefore, the Cu1In2Zr4-O-PC catalyst with lower desorption temperature and higher hydrogen desorption peak area showed better CO2 hydrogenation performance.
3.7 Effect of plasma modification on catalytic performance
Table 3 shows the activity test results of Cu1In2Zr4-O-PC, Cu1In2Zr4-O-C, and Cu1In2Zr4-O-CP catalysts under the conditions of reaction temperature at 270°C, reaction pressure of 2 MPa, CO2/H2 = 1/3, and GHSV = 12,000 mL/(g·h). The Cu1In2Zr4-O-C catalyst has a CO2 conversion of 12.8%, CH3OH selectivity of 72.8%, and CH3OH yield of 9.3%. Compared with the unmodified catalyst, the conversion of CO2 and the selectivity of methanol on the catalyst modified by plasma have been improved, and the Cu1In2Zr4-O-PC catalyst shows the best performance of CO2 hydrogenation (a CO2 conversion of 13.3%, methanol selectivity of 74.3%, CH3OH yield of 9.8%, and a space-time yield of 3.26 mmol/gcat/h). The dispersion and reduction abilities of the catalyst modified by plasma are improved, thus improving the performance of CO2 hydrogenation to methanol. The carbon balance of Cu1In2Zr4-O-C was approximately 91.3%, while that of Cu1In2Zr4-O-CP and Cu1In2Zr4-O-PC achieved 94.1% and 96.7%, respectively. It is consistent with the changes in methanol selectivity and yield, indicating that the carbon balance is influenced by the dispersion of active components in the catalyst. The carbon balance of all three samples is below 100%, which may be attributed to carbon deposition on the catalyst, residues of products in chromatographic columns, and systematic errors in chromatographic analysis.
3.8 Effect of reaction temperature on catalytic performance
Under the conditions of reaction pressure 2 MPa, CO2/H2 = 1/3, and GHSV = 12,000 mL/(g · h), the effect of reaction temperature on CO2 conversion and methanol selectivity of the catalyst was investigated. The activity test results are shown in Figure7. Figures 7A, B, D show that the CO2 conversion, methanol selectivity, and methanol yield of Cu1In2Zr4-O-PC and Cu1In2Zr4-O-CP catalysts after plasma treatment are higher than those of Cu1In2Zr4-O-C catalysts, indicating that the catalysts after plasma treatment show good catalytic activity, and the CO2 conversion of Cu1In2Zr4-O-C, Cu1In2Zr4-O-PC, and Cu1In2Zr4-O-CP catalysts increases with the increase in reaction temperature. The selectivity of methanol decreased with the increase in reaction temperature. It is observed from Figure 7C that the selectivity of CO increases with the increase in temperature because the formation of CO is an endothermic reaction, and the increase in temperature causes the chemical equilibrium to move toward the positive reaction direction. The selectivity of methanol of Cu1In2Zr4-O-C and Cu1In2Zr4-O-PC catalysts decreased from 72.8% to 63.1% and 74.3% to 67.0%, respectively, at the reaction temperature of 270°C and 290°C. The Cu1In2Zr4-O-PC catalyst after plasma treatment has good methanol selectivity at higher temperatures.
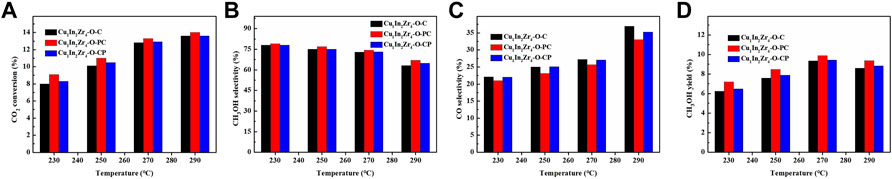
FIGURE 7. (A) CO2 conversion, methanol selectivity (B), CO selectivity (C), and methanol yield (D) of the catalysts at different temperatures. Reaction conditions: P = 2 MPa, CO2/H2 = 1/3, and GHSV = 12,000 mL/(g h).
3.9 Effect of reaction pressure on catalytic performance
Under the conditions of reaction temperature 270°C, CO2/H2 = 1/3, and GHSV = 12,000 mL/(g · h), the effect of reaction pressure on the CO2 conversion and methanol selectivity of the catalyst was investigated. The activity test results are shown in Figure 8. It is observed in Figures 8A, D that the CO2 conversion and methanol yield increase significantly with pressure. It is observed in Figure 8B that the selectivity of methanol slightly increases with pressure. In Figure 8C, the selectivity of CO continues to decrease with the increase in pressure. These results are consistent with the laws of thermodynamics. The CO2 conversion and methanol selectivity of Cu1In2Zr4-O-PC are higher than those of Cu1In2Zr4-O-CP under the same pressure, which corresponds to the better catalytic activity of the Cu1In2Zr4-O-PC catalyst. When the pressure increases to 4 MPa, the CO2 conversion rate of the Cu1In2Zr4-O-PC catalyst reaches 19.1%, the selectivity of methanol reaches 75.4%, and the yield of methanol reaches 14.4%. In other words, with the increase in pressure, the CO2 conversion rate, methanol selectivity, and methanol yield of the catalyst are significantly improved. Therefore, increasing the reaction pressure can effectively improve the catalytic activity and methanol selectivity of the catalyst.
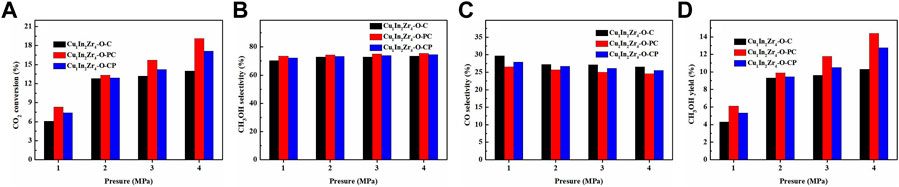
FIGURE 8. (A) CO2 conversion, methanol selectivity (B), CO selectivity (C), and methanol yield (D) of the catalysts at different pressures. Reaction conditions: T = 270°C, CO2/H2 = 1/3, and GHSV = 12,000 mL/(g h).
3.10 Catalytic performance comparisons
The recently reported catalysts closely related to our paper are listed in Table 4 for catalytic performance comparisons with our work. As shown in Table 4, the CO2 conversion, CH3OH selectivity, and yield of the reported catalysts are 10%–25%, 26%–86.2%, and 3.6%–18.3%, respectively, at 2–8 MPa, 170°C–350°C, and H2: CO2 molar ratio of 3. In our study, the CO2 conversion, CH3OH selectivity, and yield of CuInZr catalyst are 13.3%, 74.3%, and 9.8%, respectively, at 2 MPa, 270°C, and H2: CO2 molar ratio of 3, while those of CuInZr catalyst are 19.1%, 75.4%, and 14.4%, respectively, at 4 MPa, 270°C, and H2: CO2 molar ratio of 3. Therefore, the catalytic activity level in our work is upper middle above average under similar conditions. Considering that the testing conditions cannot be completely identical, this comparison of catalytic performance can only be used as a reference and cannot be blindly believed.
4 Conclusion
Cu1In2Zr4-O-C catalysts with Cu2In alloy structure were prepared for CO2 hydrogenation to methanol. Cu1In2Zr4-O-PC and Cu1In2Zr4-O-CP were obtained from plasma-modified Cu1In2Zr4-O-C before and after calcination, respectively. The characterization analysis showed that the precalcination plasma treatment can improve the dispersion, reduce the crystallinity, reduce the particle size, and enhance the reduction performance of the catalyst. Under the conditions of reaction temperature 270°C, reaction pressure 2 MPa, CO2/H2 = 1/3, and GHSV = 12,000 mL/(g·h), the Cu1In2Zr4-O-PC catalyst has a CO2 conversion of 13.3%, a methanol selectivity of 74.3%, and a CH3OH space-time yield of 3.26 mmol/gcat/h and also shows good stability. Compared with the Cu1In2Zr4-O-C catalyst, the CO2 conversion and methanol selectivity of the Cu1In2Zr4-O-PC catalyst were significantly improved.
Data availability statement
The original contributions presented in the study are included in the article/Supplementary Material, further inquiries can be directed to the corresponding author.
Author contributions
YC and JG prepared the catalysts; BY and HL performed the performance test and data analysis; FS and QX wrote the manuscript. All authors contributed to the article and approved the submitted version.
Funding
This work was supported by the Funding for School-level Research Projects of Yancheng Institute of Technology. This work was also supported by the Joint Open Fund of the Key Laboratory under Construction for Volatile Organic Compounds Controlling of Jiangsu Province and Natural Science Research Project of Jiangsu Universities (18KJB610020).
Acknowledgments
The authors also acknowledge support from the Analysis and Test Center, Yancheng Institute of Technology, and appreciate the help provided by D. Zheng.
Conflict of interest
The authors declare that the research was conducted in the absence of any commercial or financial relationships that could be construed as a potential conflict of interest.
Publisher’s note
All claims expressed in this article are solely those of the authors and do not necessarily represent those of their affiliated organizations, or those of the publisher, the editors, and the reviewers. Any product that may be evaluated in this article, or claim that may be made by its manufacturer, is not guaranteed or endorsed by the publisher.
Supplementary material
The Supplementary Material for this article can be found online at: https://www.frontiersin.org/articles/10.3389/fchem.2023.1187762/full#supplementary-material
References
Azenha, C. S. R., Mateos-Pedrero, C., Queirós, S., Concepcion, P., and Mendes, A. (2017). Innovative ZrO2-supported CuPd catalysts for the selective production of hydrogen from methanol steam reforming. Appl. Catal. B Environ. 203, 400–407. doi:10.1016/j.apcatb.2016.10.041
Bagherzadeh, S. B., and Haghighi, M. (2017). Plasma-enhanced comparative hydrothermal and coprecipitation preparation of CuO/ZnO/Al2O3 nanocatalyst used in hydrogenproduction via methanol steam reforming. Energy Convers. manage. 142, 452–465. doi:10.1016/j.enconman.2017.03.069
Bowker, M., Lawes, N., Gow, I., Hayward, J., Esquius, J. R., Richards, N., et al. (2022). The critical role of βPdZn alloy in Pd/ZnO catalysts for the hydrogenation of carbon dioxide to methanol. ACS Catal. 12, 5371–5379. doi:10.1021/acscatal.2c00552
Cg, A., Tra, B., Jr, A., Sb, A., Pl, C., Sl, C., et al. (2021). Effect of the preparation method on particle size and reaction selectivity on naphthalene hydrogenation over Ni/H-MOR catalysts. Catal. Today 360, 63–71. doi:10.1016/j.cattod.2019.08.044
Chen, K., Fang, H., Wu, S., Liu, X., Zheng, J., Zhou, S., et al. (2019). CO2 hydrogenation to methanol over Cu catalysts supported on La-modified SBA-15: The crucial role of Cu-LaOx interfaces. Appl. Catal. B Environ. 251, 119–129. doi:10.1016/j.apcatb.2019.03.059
Ezeh, C. I., Yang, X., He, J., Snape, C., and Cheng, X. (2018). Correlating ultrasonic impulse and addition of ZnO promoter with CO2 conversion and methanol selectivity of CuO/ZrO2 catalysts. Ultrason. Sonochem. 42, 48–56. doi:10.1016/j.ultsonch.2017.11.013
Gao, J., Song, F., Li, Y., Cheng, W., and Xu, Q. (2020). Cu2In nanoalloy enhanced performance of Cu/ZrO2 catalysts for the CO2 hydrogenation to methanol. Ind. Eng. Chem. Res. 59, 12331–12337. doi:10.1021/acs.iecr.9b06956
Han, F., Liu, H., Cheng, W., and Xu, Q. (2020). Highly selective conversion of CO2 to methanol on the CuZnO-ZrO2 solid solution with the assistance of plasma. RSC Adv. 10, 33620–33627. doi:10.1039/D0RA00961J
Jiang, X., Koizumi, N., Guo, X., and Song, C. (2015). Bimetallic Pd-Cu catalysts for selective CO2 hydrogenation to methanol. Appl. Catal. B Environ. 170, 173–185. doi:10.1016/j.apcatb.2015.01.010
Kierzkowska-Pawlak, H., Tracz, P., Redzynia, W., and Tyczkowski, J. (2017). Plasma deposited novel nanocatalysts for CO2 hydrogenation to methane. J. CO2Util. 17, 312–319. doi:10.1016/j.jcou.2016.12.013
Lasobras, J., Herguido, J., Menéndez, M., Soler, J, and Trifan, B. (2021). Modifications in the composition of CuO/ZnO/Al2O3 catalyst for the synthesis of methanol by CO2 hydrogenation. Catalysts 11, 774–786. doi:10.3390/catal11070774
Li, S., Wang, Y., Yang, B., and Guo, L. (2019). A highly active and selective mesostructured Cu/AlCeO catalyst for CO2 hydrogenation to methanol. Appl. Catal. A Gen. 571, 51–60. doi:10.1016/j.apcata.2018.12.008
Li, W., Zhang, J., Jiang, X., Mu, M., Zhang, A., Song, C., et al. (2022). Co-promoted In2O3/ZrO2 integrated with ultrathin nanosheet HZSM-5 as efficient catalysts for CO2 hydrogenation to gasoline. Ind. Eng. Chem. Res. 61, 6322–6332. doi:10.1021/acs.iecr.2c00460
LiangMaoGuoYu, Y. D. X. J., Ma, Z., Yu, J., and Wu, G. (2021). Solvothermal preparation of CuO-ZnO-ZrO2 catalysts for methanol synthesis via CO2 hydrogenation. J. Taiwan Inst. Chem. E. 121, 81–91. doi:10.1016/j.jtice.2021.03.049
Liu, C., Li, M., Wang, J., Zhou, X., Guo, Q., Yan, J., et al. (2016). Plasma methods for preparing green catalysts: Current status and perspective. Chin. J. Catal. 37, 340–348. doi:10.1016/S1872-2067(15)61020-8
Liu, Y., Zhang, Y., Wang, T., and Tsubaki, N. (2007). Efficient conversion of carbon dioxide to methanol using copper catalyst by a new low-temperature hydrogenation process. Chem. Lett. 36, 1182–1183. doi:10.1246/cl.2007.1182
Lu, Z., Sun, K., Wang, J., Zhang, Z., and Liu, C. (2020). A highly active Au/In2O3-ZrO2 catalyst for selective hydrogenation of CO2 to methanol. Catalysts 10, 1360. doi:10.3390/catal10111360
Pei, L., Cao, J., Liu, F., Yang, A., Yao, M., Zhao, T., et al. (2022). Thiolation behaviors of methanol catalyzed by bifimctional ZSM-5@t-ZrO2 catalyst. Catal. Today 397, 379–388. doi:10.1016/j.cattod.2021.08.011
Rui, N., Zhang, F., Sun, K., Liu, Z., Liu, C. J., Stavitski, E., et al. (2020). Hydrogenation of CO2 to methanol on a Auδ+- In2O3-x catalyst. ACS Catal. 10, 11307–11317. doi:10.1021/acscatal.0c02120
Sajjadi, S. M., and Haghighi, M. (2018). Impregnation vs. sol-gel and sol-gel-plasma dispersion of nickel nanoparticles over Al2O3 employed in combined dry reforming and partial oxidation of greenhouse gases to syngas. Int. J. Hydrogen Energy 43, 15014–15029. doi:10.1016/j.ijhydene.2018.06.073
Samson, K., Liwa, M., Socha, R. P., Góra-Marek, K, Mucha, D., Rutkowska-Zbik, D., et al. (2014). Influence of ZrO2 structure and copper electronic state on activity of Cu/ZrO2 catalysts in methanol synthesis from CO2. ACS Catal. 4, 3730–3741. doi:10.1021/cs500979c
Shi, Z., Pan, M., Wei, X., and Wu, D. (2021). Cu-In intermetallic compounds as highly active catalysts for CH3OH formation from CO2 hydrogenation. Int. J. Energy Res. 46, 1285–1298. doi:10.1002/er.7246
Shi, Z., Tan, Q., Tian, C., Pan, Y., Sun, X., Zhang, J., et al. (2019). CO2 hydrogenation to methanol over Cu-In intermetallic catalysts: Effect of reduction temperature. J. Catal. 379, 78–89. doi:10.1016/j.jcat.2019.09.024
Shi, Z., Tan, Q., and Wu, D. (2018). A novel core-shell structured CuIn@SiO2 catalyst for CO2 hydrogenation to methanol. AIChE J. 65, 1047–1058. doi:10.1002/aic.16490
Tada, S., Ochiai, N., Kinoshita, H., Yoshida, M., Shimada, N., Joutsuka, T., et al. (2022). Active sites on ZnxZr(1-x)O(2-x) solid solution catalysts for CO2-to-methanol hydrogenation. ACS Catal. 12, 7748–7759. doi:10.1021/acscatal.2c01996
Tan, Q., Shi, Z., and Wu, D. (2019). CO2 hydrogenation over differently morphological CeO2-supported Cu-Ni catalysts. Int. J. Energy Res. 43, 5392–5404. doi:10.1002/er.4636
Tan, Q., Shi, Z., and Wu, D. (2018). CO2 hydrogenation to methanol over a highly active Cu-Ni/CeO2-nanotube catalyst. Ind. Eng. Chem. Res. 57, 10148–10158. doi:10.1021/acs.iecr.8b01246
Wang, J., Li, G., Li, Z., Tang, C., Feng, Z., An, H., et al. (2017). A highly selective and stable ZnO-ZrO2 solid solution catalyst for CO2 hydrogenation to methanol. Sci. Adv. 3, 1701290–1701299. doi:10.1126/sciadv.1701290
Wang, W., Qu, Z., Song, L., and Fu, Q. (2020). CO2 hydrogenation to methanol over Cu/CeO2 and Cu/ZrO2 catalysts: Tuning methanol selectivity via metal-support interaction. J. Energy Chem. 40, 22–30. doi:10.1016/j.jechem.2019.03.001
Witoon, T., Kidkhunthod, P., Chareonpanich, M., and Limtrakul, J. (2018b). Direct synthesis of dimethyl ether from CO2 and H2 over novel bifunctional catalysts containing CuO-ZnO-ZrO2 catalyst admixed with WOx/ZrO2 catalysts. Chem. Eng. J. 348, 713–722. doi:10.1016/j.cej.2018.05.057
Witoon, T., Numpilai, T., Phongamwong, T., Donphai, W., Boonyuen, C., Warakulwit, C., et al. (2018a). Enhanced activity, selectivity and stability of a CuO-ZnO-ZrO2 catalyst by adding graphene oxide for CO2 hydrogenation to methanol. Chem. Eng. J. 334, 1781–1791. doi:10.1016/j.cej.2017.11.117
Wu, Q., Shen, C., Rui, N., Sun, K., and Liu, C. J. (2021). Experimental and theoretical studies of CO2 hydrogenation to methanol on Ru/In2O3. J. CO2Util. 53, 101720–101727. doi:10.1016/j.jcou.2021.101720
Yan, Y., Wong, R. J., Ma, Z., Donat, F., Xi, S., Saqline, S., et al. (2022). CO2 hydrogenation to methanol on tungsten-doped Cu/CeO2 catalysts. Appl. Catal. B Environ. 306, 121098–121107. doi:10.1016/j.apcatb.2022.121098
Zafar, F., Zhao, R., Ali, M., Park, Y. M., Roh, H. S., Gao, X., et al. (2022). Unprecedented contributions of In2O3 promoter on ordered mesoporous Cu/Al2O3 for CO2 hydrogenation to oxygenates. Chem. Eng. J. 439, 135649. doi:10.1016/j.cej.2022.135649
Zeng, J., Lu, K., Zhang, J., Sun, Y., Chang, Z., Li, J., et al. (2022). Solution plasma-assisted preparation of highly dispersed NiMnAl-LDO catalyst to enhance low-temperature activity of CO2 methanation. Int. J. Hydrogen Energy 47, 2234–2244. doi:10.1016/j.ijhydene.2021.10.183
Keywords: plasma modification, ZrO2, Cu2In alloy, CO2 hydrogenation, methanol
Citation: Song F, Gao J, Yang B, Cao Y, Liu H and Xu Q (2023) Cu2In alloy-embedded ZrO2 catalysts for efficient CO2 hydrogenation to methanol: promotion of plasma modification. Front. Chem. 11:1187762. doi: 10.3389/fchem.2023.1187762
Received: 16 March 2023; Accepted: 01 May 2023;
Published: 23 May 2023.
Edited by:
Yangang Wang, Jiaxing University, ChinaReviewed by:
Jun Ni, Zhejiang University of Technology, ChinaAndrii Kostyniuk, National Institute of Chemistry, Slovenia
Copyright © 2023 Song, Gao, Yang, Cao, Liu and Xu. This is an open-access article distributed under the terms of the Creative Commons Attribution License (CC BY). The use, distribution or reproduction in other forums is permitted, provided the original author(s) and the copyright owner(s) are credited and that the original publication in this journal is cited, in accordance with accepted academic practice. No use, distribution or reproduction is permitted which does not comply with these terms.
*Correspondence: Qi Xu, eWN4cXN0ZXZlQDE2My5jb20=