- 1Ningxia Key Laboratory of Intelligent Sensing for the Desert Information, School of Physics and Electronic-Electrical Engineering, Ningxia University, Yinchuan, China
- 2Basic Education Department, Guangdong Ocean University, Yangjiang, China
- 3Enviromental Monitoring Site of Ningxia Ningdong Energy and Chemical Industry Base, Yinchuan, China
In this study, the adsorption of gases (CH4, CO, H2, NH3, and NO) onto Al12Si12 nanocages was theoretically investigated using density functional theory. For each type of gas molecule, two different adsorption sites above the Al and Si atoms on the cluster surface were explored. We performed geometry optimization on both the pure nanocage and nanocages after gas adsorption and calculated their adsorption energies and electronic properties. The geometric structure of the complexes changed slightly following gas adsorption. We show that these adsorption processes were physical ones and observed that NO adsorbed onto Al12Si12 had the strongest adsorption stability. The Eg (energy band gap) value of the Al12Si12 nanocage was 1.38 eV, indicating that it possesses semiconductor properties. The Eg values of the complexes formed after gas adsorption were all lower than that of the pure nanocage, with the NH3–Si complex showing the greatest decrease in Eg. Additionally, the highest occupied molecular orbital and the lowest unoccupied molecular orbital were analyzed according to Mulliken charge transfer theory. Interaction with various gases was found to remarkably decrease the Eg of the pure nanocage. The electronic properties of the nanocage were strongly affected by interaction with various gases. The Eg value of the complexes decreased due to the electron transfer between the gas molecule and the nanocage. The density of states of the gas adsorption complexes were also analyzed, and the results showed that the Eg of the complexes decreased due to changes in the 3p orbital of the Si atom. This study theoretically devised novel multifunctional nanostructures through the adsorption of various gases onto pure nanocages, and the findings indicate the promise of these structures for use in electronic devices.
1 Introduction
Since Kroto et al. (1985) discovered (C60) fullerene, nanomaterials have gradually become a popular research topic due to their unique properties and wide range of applications (Goroff, 1996; Diederich and Gómez-López, 1999; Kataura et al., 2002; Martín, 2006). Instead of using only C to construct fullerenes, researchers have turned to fullerenes made from other elements, especially elements from group Ⅲ of the periodic table and the group V elements that are adjacent to C. These fullerenes include B12N12, Al12N12, B12P12, and Al12P12 (Beheshtian et al., 2012a; Beheshtian et al., 2012b; Beheshtian et al., 2012c; Rad and Ayub, 2016; Hussain et al., 2020a). In addition, researchers have used elements from groups Ⅱ and Ⅵ to construct similar fullerene structures, such as Be12O12, Mg12O12, Ca12O12, and all-boron fullerene (Kakemam and Peyghan, 2013; Zhai et al., 2014; Rezaei-Sameti and Abdoli, 2020; Ahsan et al., 2021). Other fullerenes are made from transition elements and oxygen element (de Oliveira et al., 2015). Nanocages with the general formula (XY)12, where n is the number of atoms, are more popular among researchers because they are more stable (Strout, 2000). Many types of nanostructures exist, among which inorganic nanostructures have attracted the attention of researchers due to their extremely high stability and asymmetric charge distribution (Dai, 2002; Tasis et al., 2006; Bindhu et al., 2016). The study of fullerene structures is a critical branch of nanotechnology because of their applications in electronic devices, special materials, and environmental processes. Shakerzdeh et al. (2015) studied the properties of alkali metals (Li, Na, and K) interacting with Be12O12 and Mg12O12 nanoclusters. Doping with alkali metals can significantly improve the non-linear optical response of nanocages. Beheshtian et al. (2012c) have studied the adsorption of NO and CO by Al12N12. Due to the different changes in the Eg (energy band gap) of NO and CO when adsorbed, resulting in different changes in electrical conductivity, Al12N12 clusters may selectively detect NO molecules when CO molecules are present. Vergara-Reyes et al. (2021) studied the adsorption of NO on the C36N24 fullerene, providing a possible carrier/protector of nitric oxide and thus fulfill its correct biological functions. Escobedo et al. (2019) conducted the effect of chemical order on the structural and physicochemical properties of B12N12 fullerene. Bautista Hernandez et al. (2022) found that the (TiO2)19 cluster is a good candidate for storing various gases, and can also be used as a hydrogen storage device.
For China in particular and the rest of the world in general, fossil fuel use must be scaled back, and hydrogen, which has the highest calorific value per unit mass of fuels and produces only water during combustion, is an attractive alternative (Yilanci et al., 2009; Züttel et al., 2010; Mazloomi and Gomes, 2012). Technologies for capturing pollutants and greenhouse gases from the atmosphere will also play a key role in our fight against climate change (Cazorla-Amorós et al., 1996; Zhang et al., 2008; Mastalerz et al., 2011).
Oku et al. (2004) synthesized the first inorganic nanocages in 2004 before the later emergence of B12N12, Al12N12, B12P12, and Al12P12 nanocages. Yarovsky and Goldberg, (2005) studied the adsorption of H2 by Al13 clusters using DFT (density functional theory). Thereafter, Felício-Sousa et al. (2021) examined the adsorption properties of H2, CO, CH4, and CH3OH on Fe13, Co12, Ni13, and Cu13 clusters using an ab initio investigation. Tan et al. (2022) studied clusters of different numbers of Al atoms. Yang et al. (2018) predicted the properties of electron redundant SinNn fullerenes. Metal oxide nanocages are also a popular area of research; Otaibi et al. conducted a theoretical study on the adsorption of glycoluril by Mg12O12 nanocages (Al-Otaibi et al., 2022). Some nanocages exhibit unique electronic properties after doping with alkali metals, and these have also been widely studied (Ahsin and Ayub, 2021). We replaced the N atoms in Al12N12 with Si atoms to investigate a more diverse range of materials than those in the literature. In this study, we adopted DFT to analyze the properties of Al12Si12, such as its stability, after the adsorption of CH4, CO, H2, NO, and NH3.
2 Computational methodology
An Al12Si12 nanocage was selected as the model adsorbent. Geometry optimization was performed using hybrid functional DFT (B3LYP) (Becke, 1993) implemented in Gaussian 09 (Frisch et al., 2004). B3LYP is a suitable and widely accepted functional for nanoclusters (Chen et al., 2009; Hussain et al., 2020b). The mixed basis set 6-31G (d, p) was used. Gas molecules CH4, CO, H2, NO, and NH3 were adsorbed onto Al12Si12 nanocages using the same method. Two adsorption sites on the Al12Si12 nanocage were considered. Vibration frequencies were also calculated at equivalent levels to verify that all stationary points corresponded to true minima on the potential energy surface. Geometry optimization was conducted, and Dads (distance of adsorption), Eads (adsorption energy), EHOMO (energy of the highest occupied molecular orbital), ELUMO (energy of lowest unoccupied molecular orbital), DOS (density of states), and QT (Mulliken charge transfer) were calculated to determine the adsorption mechanism.
The stability of the Al12Si12 nanocage was examined in terms of Ecoh (cohesive energy), which can be determined by calculating the average energy difference of each atom before and after bonding using the following equation:
where EAl and ESi are the energies of non-interacting Al and Si atoms, respectively, and Etotal is the energy of the Al12Si12 nanocage.
Eads is defined as follows:
Here, Eadsorbate@nanocage, Eadsorbate, and Enanocage are the total energies of an adsorbate adsorbed onto the pure nanocage, of the adsorbate, and of the pure nanocage, respectively. A negative value of Eads corresponds to exothermic adsorption. The more negative the adsorption value, the stronger the adsorption capacity. The DOS was generated in Multiwfn (O'Boyle et al., 2008).
3 Results and discussion
3.1 Geometrical characteristics
3.1.1 Al12Si12 structure
The optimized structures of the bare nanocage comprising eight hexagons and six tetragon rings are given in Figure 1. In this nanocage, two non-equivalent bonds exist: one between the tetragon and hexagon ring, and the other between the two hexagonal rings, represented as b64 and b66, respectively. The lengths of b64 and b66 are 2.47 and 2.43 Å, respectively. The calculated Ecoh of Al12Si12 is −3.11 eV with zero point energy (ZPE) correction (Buelna-García et al., 2022). The calculated Ecoh of each atom is closer to the reported Ecoh of the most stable inorganic analogue, B12N12. The experimental and theoretical reported Ecoh of B12N12 are −4.00 (Pokropivny et al., 2000) and −6.06 eV (Pokropivny and Ovsyannikova, 2007), respectively. As indicated by these results, the Al12Si12 nanocage is highly stable.
3.1.2 Al12Si12–gas molecule structure
CH4, CO, H2, NO, and NH3 were selected as the target adsorbates. We performed sufficient structural optimization of the Al12Si12 nanocage containing CH4, CO, H2, NO, and NH3 molecules. For each gas molecule, two different adsorption sites above the Al and Si atoms on the nanocage surface were considered. Each gas is discussed with respect to adsorption at each of the two nanocage positions. The bond length (b64 and b66), Dads value (defined as the center to center distance between the atoms of the nanocage and gas molecule that are closest to each other), and Eads value are listed in Table 1. According to previous studies, the threshold Eads at which physisorption becomes chemisorption is approximately 23.00 kcal/mol (1.00 eV) (Jouypazadeh and Farrokhpour, 2018; Nagarajan and Chandiramouli, 2019; Swetha et al., 2020). Dads must be < 2.00 Å for chemisorption to occur (Contreras et al., 2014). As shown in Table 1, the adsorption processes were all physical, and NO adsorbed above the Al atom of the nanocage had the strongest adsorption stability.
The calculated stable configurations (local minima) are summarized in Figure 1. When the gas was adsorbed onto the Al or Si atom of the Al12Si12 nanocage, slight local structural deformation of both the molecule and the nanocage occurred. The b66 and b64 bonds and angles remained almost unchanged. The adsorption that induced greatest change was that of NH3 onto the Si atom; it changed the lengths of b66 and b64 by 0.005 and 0.006 Å, respectively. The smallest change was caused by the adsorption of NO onto the Al atom; it changed the lengths of b66 and b64 by 0.0003 and 0.0204 Å, respectively.
In the Al atom adsorption group, the Eads of CH4–Al, CO–Al, NH3–Al, NO–Al, and H2–Al were −1.00, −1.05, −0.55, −15.35, and −12.37 kcal/mol, respectively. The adsorption stability of the NO and H2 analytes was much better than that of the others. The Dads values of NO–Al and H2–Al were 3.94 and 4.52 Å, respectively. For NO–Al, the N–O bond length increased from 1.06 Å in isolated NO to 1.16 Å in the adsorbed state. When Al12Si12 adsorbed H2, the H–H bond length increased from 0.6 Å in isolated H2 to 0.74 Å in the adsorbed state. The Dads between the gas molecule and the Al atom of the nanocage were 5.76 and 4.51 Å for CH4–Al and CO–Al, respectively. The C–H bonds of CH4–Al and the C–O bond of CO–Al also became slightly longer. The lengths of the four C–H bonds of CH4–Al increased by 0.02269, 0.02286, 0.02290, and 0.02313 Å. The length of the C–O bond of CO–Al increased by 0.02 Å. The Dads of NH3–Al was 6.11 Å. The length of the three N–H bonds in the NH3 molecule increased by 0.015636, 0.01599, and 0.01542 Å. Among these complexes, the three N–H bonds of NH3–Al had the smallest increase in length.
In the Si atom adsorption group, the Eads of CH4–Si, CO–Si, NH3–Si, NO–Si, and H2–Si were −1.02, −0.96, −1.23, −15.18, and −12.34 kcal/mol, respectively. The Dads values of CH4–Si, CO–Si, NH3–Si, NO–Si, and H2–Si were 7.36, 4.39, 3.48, 5.58, and 3.92 Å, respectively. In the Si atom adsorption group of complexes, both nanocages and gas molecules changed slightly. The length of the four C–H bonds increased by 0.02253, 0.02268, 0.02284, and 0.02315 Å. The length of the C–O bond increased by 0.02214 Å. The three N–H bonds increased in length by 0.01504, 0.01483, and 0.01485 Å. The lengths of the N–O bond and H–H bond increased by 0.09682, and 0.14372 Å, respectively. Among these complexes, the lengths of the three N–H bonds of NH3–Si increased by the least, but they increased by more than those of the three N–H bonds of NH3–Al in the Al group.
3.2 Electronic properties
3.2.1 Mulliken charge and energy band gap analysis
Detailed information including the EHOMO and ELUMO, QT, and the △Eg (change in, Eg of nanocage upon adsorption) values is listed in Table 2.
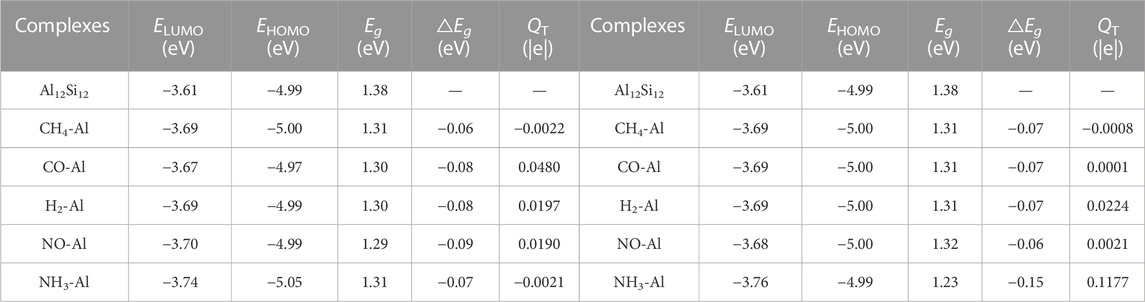
TABLE 2. The Energy of HOMO (EHOMO), energy of LUMO (ELUMO), energy band gap (Eg), change of Eg of nanocage upon the adsorption process (△Eg), Mulliken charge transfer (QT).
In the Al atom adsorption group, the Mulliken charge analysis shows that the number of transferred electrons in CH4–Al, CO–Al, NH3–Al, NO–Al, and H2–Al were −0.0020, −0.0480, −0.0021, 0.0190, and 0.0197 |e|, respectively. The Eg of CH4–Al, CO–Al, NH3–Al, NO–Al, and H2–Al are 1.31, 1.30, 1.31, 1.29, and 1.30 eV, respectively. The Eg of these complexes was lower than that of the pure nanocage, and this decrease was largest in the NO complex, at 0.09 eV, and smallest in the CH4 complex, at 0.06 eV.
In the Si atom adsorption group, the Mulliken charge analysis demonstrated that the number of transferred electrons in CH4–Si, CO–Si, NH3–Si, NO–Si, and H2–Si were −0.0008, 0.0001, 0.1177, 0.0021, and 0.0224 |e|, respectively. The Eg of CH4–Si, CO–Si, NH3–Si, NO–Si, and H2–Si was 1.31, 1.31, 1.23, 1.31, and 1.31 eV, respectively. The Eg values of these complexes were also lower than that of the pure nanocage, with the NH3 complex exhibiting the greatest decrease, at 0.15 eV, and the NO complex exhibiting is the smallest decrease at 0.06 eV.
As demonstrated in Table 2, the ELUMO values of these complexes were slightly lower than those of the pure nanocage, but their EHOMO values did not vary much from those of the pure nanocage. The adsorption stability of the Si adsorption group of complexes is similar to that of the Al adsorption group. However, the adsorbates in the Si adsorption group transferred fewer electrons than the corresponding adsorbates in the Al adsorption group, except for the H2 and NH3 analytes. The N atom gained electrons, and the three H atoms lost electrons in the NH3–Al adsorption. However, the N atom and the three H atoms all lost electrons in the NH3–Si adsorption due to the difference in adsorption position. The adsorption stability of NH3–Si was higher than that of NH3–Al due to the short adsorption distance and the large number of transferred electrons in the NH3–Si adsorption.
3.2.2 Frontier molecular orbitals and DOS
To further analyze the effect of gas molecules on the electronic properties of nanocages, the HOMO (highest occupied molecular orbital), LUMO (lowest unoccupied molecular orbital) and DOS of isolated and complexed nanocages were analyzed. The HOMO and LUMO distributions of Al12Si12 from a vertical perspective are shown in Figure 2. The HOMO primarily serves as an electron donor, and the LUMO primarily serves as an electron acceptor (Dai, 2002). For the HOMO of the pure Al12Si12 nanocage, the positive and negative areas alternate between Al and Si atoms (in Figure 2 the red area is negative, the green area is positive). However, for the LUMO of the pure Al12Si12 nanocage, the positive and negative areas alternated between the inside and outside of the nanocage and the HOMO and LUMO were distributed on the same plane. The obtained frontier molecular orbital energies (EHOMO and ELUMO) and the calculated, Eg value of the nanocages are shown in Table 2. The Eg of Al12Si12 was approximately 1.38 eV, demonstrating its semiconductor properties.
The HOMO and LUMO plots of the complexes are displayed in Figure 2. The lower the Eg value, the more easily a molecule was excited. In the Al adsorption group, the HOMO and LUMO of complexes were almost unchanged. After gas adsorption, the distribution positions of the HOMO and LUMO changed from being on the same plane to being perpendicular to each other. In the Si adsorption group, the HOMO and LUMO of CO–Si remained on the same plane after adsorption. This may be due to the low number of electron transfers. In NH3–Si, the LUMO distribution was in the NH3 molecule. This may be because NH3–Si has the shortest adsorption distance and the greatest charge transfer. In NO–Si, part of the HOMO is transferred to the quadrilateral ring opposite the top and bottom. This is most likely because the HOMO energy level of NO–Si is lower than that of NO–Al.
For further confirmation of the electronic behavior of these complexes, DOS analyses were performed (shown in Figures 3, 4). The orbitals in the lower energy region of Al12Si12 were mainly the 3p orbitals of the Si and Al atoms. At approximately −0.26 a.u., 3s orbitals of both the Al atoms and the Si atoms were maily present. The HOMO–LUMO energy level was composed mainly of the 3p orbitals of the Si and Al atoms, whereas the 3s orbitals of Al atoms were also present near the LUMO energy level.
The effect of the gas molecular orbital on the complex orbital when the nanocage adsorbed some gases was not significant (atomic orbitals that do not contribute to the total orbitals are not depicted in Figures 3, 4). We divided the complexes into two groups: The first including gas molecular orbitals that did not contribute to the total orbitals of the complexes, and the second including the gas molecular orbitals that contributed to the total orbitals of the complexes. In the first group, after gas adsorption, a new peak at 0.2 a.u. appeared, resulting in a slight decrease in the HOMO of the complexes (except for CO–Al). In addition, due to the adsorption of the gas molecule, the 3s orbital of the Al atom produced a small peak at −0.12 a.u. (shown by the blue curve), causing the peak at −0.11 a.u. to shift to the right slightly. This may be the reason for the decrease in LUMO energy level. In the second group, after gas adsorption, a new peak also appeared at 0.2 a.u.. The 3p orbital of the N atom in NH3–Al peaked at −0.27 a.u., whereas it peaked at −0.28 a.u. in NH3–Si (depicted by the brown curve). The 3p orbitals of the N and O atoms in NO–Al and NO–Si had peaks at −0.23 and −0.12 a.u. (the brown curve and the orange curve), respectively. Moreover, the peak value of the 3p orbital of the O atom was greater than that of the N atom. However, compared with the first group, the new peaks in the second group caused almost no decrease in HOMO and LUMO energy levels. The peak generated at 0.2 a.u. of the 3p orbital of the Si and Al atoms was the main reason for the reduction in HOMO energy level.
4 Conclusion
DFT calculations were performed to investigate the equilibrium geometries, stabilities, and electronic properties of gases adsorbed onto Al12Si12 nanocages. The Eg value of Al12Si12 was 1.38 eV, demonstrating the semiconductor properties of the nanocage. We performed structural optimization of Al12Si12 nanocages with adsorbed CH4, CO, H2, NO, and NH3 molecules to study their adsorption energies, equilibrium geometry, and electronic properties. Two adsorption positions on the nanocage were studied. The adsorption energy of these complexes demonstrated that the adsorption of these gases by Al12Si12 nanocages is physical. Our findings reveal that the stabilities of the complexes are as follows: Al12Si12–NO > Al12Si12–H2 > Al12Si12–NH3 >Al12Si12–CH4 >Al12Si12–CO. The geometric structure of the nanocage changed slightly following adsorption of molecules. The Eg of the complexes was lower than that of the pure nanocage due to the electron transfer between the gas molecules and the nanocage. The more electrons were transferred, the greater the decrease in Eg. Except for the 3p orbitals of the N and O atoms, the orbitals of the gas molecules did not contribute to the total orbitals of the complexes. The 3p orbitals of the Si atoms in the Al12Si12 nanocage are the main reason for the change in HOMO–LUMO energy levels detected.
Data availability statement
The original contributions presented in the study are included in the article/supplementary material, further inquiries can be directed to the corresponding author.
Author contributions
Conceptualization, L-KL and K-NL; methodology, K-NL; software, W-LX; validation, Y-QM; formal analysis, L-KL; investigation, BH; resources, BH; data curation, K-NL; writing original draft preparation, L-KL; writing—review and editing, K-NL; visualization, W-LX; supervision, K-NL; project administration, BH; funding acquisition, K-NL. All authors have read and agreed to the published version of the manuscript.
Funding
This work was supported by the National Natural Science Foundation of China, grant number 42167016. The Natural Science Foundation of Ningxia Province in China, grant number 2022AAC03123. The Key Research and Development Program of Ningxia Province in China, grant number 2020BEB04003, and the fifth batch of the Ningxia Youth Science and Technology Talents Project, grant number NXTJGC147.
Conflict of interest
The authors declare that the research was conducted in the absence of any commercial or financial relationships that could be construed as a potential conflict of interest.
Publisher’s note
All claims expressed in this article are solely those of the authors and do not necessarily represent those of their affiliated organizations, or those of the publisher, the editors and the reviewers. Any product that may be evaluated in this article, or claim that may be made by its manufacturer, is not guaranteed or endorsed by the publisher.
References
Ahsan, A., Khan, S., Gilani, M. A., and Ayub, K. (2021). Endohedral metallofullerene electrides of Ca12O12 with remarkable nonlinear optical response. RSC Adv. 11 (3), 1569–1580. doi:10.1039/d0ra08571e
Ahsin, A., and Ayub, K. (2021). Extremely large static and dynamic nonlinear optical response of small superalkali clusters NM3M’ (M, M’=Li, Na, K). J. Mol. Graph. Model. 109, 108031. doi:10.1016/j.jmgm.2021.108031
Al-Otaibi, J. S., Mary, Y. S., Mary, Y. S., Acharjee, N., and Churchill, D. G. (2022). Theoretical study of glycoluril by highly symmetrical magnesium oxide Mg12O12 nanostructure: Adsorption, detection, SERS enhancement, and electrical conductivity study. J. Mol. Model. 28 (10), 332. doi:10.1007/s00894-022-05332-3
Bautista Hernandez, A., Chigo Anota, E., Severiano Carrillo, F., Vázquez Cuchillo, O., and Salazar Villanueva, M. (2022). In-silico study of the adsorption of H2, CO and CO2 chemical species on (TiO2)n n=15–20 clusters: The (TiO2)19 case as candidate promising. J. Mol. Graph. Model. 117, 108316. doi:10.1016/j.jmgm.2022.108316
Becke, A. D. (1993). Density-functional thermochemistry. III. The role of exact exchange. J. Chem. Phys. 98 (7), 5648–5652. doi:10.1063/1.464913
Beheshtian, J., Bagheri, Z., Kamfiroozi, M., and Ahmadi, A. (2012). A comparative study on the B12N12, Al12N12, B12P12 and Al12P12 fullerene-like cages. J. Mol. Model. 18 (6), 2653–2658. doi:10.1007/s00894-011-1286-y
Beheshtian, J., Kamfiroozi, M., Bagheri, Z., and Peyghan, A. A. (2012). B12N12Nano-cage as potential sensor for NO2Detection. Chin. J. Chem. Phys. 25 (1), 60–64. doi:10.1088/1674-0068/25/01/60-64
Beheshtian, J., Peyghan, A. A., and Bagheri, Z. (2012). Selective function of Al12N12 nano-cage towards NO and CO molecules. Comput. Mat. Sci. 62, 71–74. doi:10.1016/j.commatsci.2012.05.041
Bindhu, M. R., Umadevi, M., Kavin Micheal, M., Arasu, M. V., and Abdullah Al-Dhabi, N. (2016). Structural, morphological and optical properties of MgO nanoparticles for antibacterial applications. Mat. Lett. 166, 19–22. doi:10.1016/j.matlet.2015.12.020
Buelna-García, C. E., Castillo-Quevedo, C., Quiroz-Castillo, J. M., Paredes-Sotelo, E., Cortez-Valadez, M., Martin-del-Campo-Solis, M. F., et al. (2022). Relative populations and IR spectra of Cu38 cluster at finite temperature based on DFT and statistical thermodynamics calculations. Front. Chem. 10, 841964. doi:10.3389/fchem.2022.841964
Cazorla-Amorós, D., Alcañiz-Monge, J., and Linares-Solano, A. (1996). Characterization of activated carbon fibers by CO2 adsorption. LANGMUIR 12 (11), 2820–2824. doi:10.1021/la960022s
Chen, L., Zhou, G.-Q., Xu, C., Zhou, T., and Huo, Y. (2009). Structural and electronic properties of hydrated MgO nanotube clusters. J. Mol. Struct-Theochem. 900 (1), 33–36. doi:10.1016/j.theochem.2008.12.019
Contreras, M. L., Cortés-Arriagada, D., Villarroel, I., Alvarez, J., and Rozas, R. (2014). Evaluating the hydrogen chemisorption and physisorption energies for nitrogen-containing single-walled carbon nanotubes with different chiralities: A density functional theory study. Struct. Chem. 25 (4), 1045–1056. doi:10.1007/s11224-013-0377-z
Dai, H. (2002). Carbon nanotubes: Opportunities and challenges. Surf. Sci. 500 (1), 218–241. doi:10.1016/s0039-6028(01)01558-8
de Oliveira, O. V., Pires, J. M., Neto, A. C., and Divino dos Santos, J. (2015). Computational studies of the Ca12O12, Ti12O12, Fe12O12 and Zn12O12 nanocage clusters. Chem. Phys. Lett. 634, 25–28. doi:10.1016/j.cplett.2015.05.069
Diederich, F., and Gómez-López, M. (1999). Supramolecular fullerene chemistry. Chem. Soc. Rev. 28 (5), 263–277. doi:10.1039/a804248i
Escobedo, A., Bautista-Hernandez, A., Camacho-Garcia, J. H., Cortes-Arriagada, D., and Chigo-Anota, E. (2019). Effect of chemical order in the structural stability and physicochemical properties of B12N12 fullerenes. Sci. Rep-UK. 9, 16521. doi:10.1038/s41598-019-52981-1
Felício-Sousa, P., Andriani, K. F., and Da Silva, J. L. F. (2021). Ab initio investigation of the role of the d-states occupation on the adsorption properties of H2, CO, CH4 and CH3OH on the Fe13, Co13, Ni13 and Cu13 clusters. Phy. Hys. Chem. Chem. Phys. 23 (14), 8739–8751. doi:10.1039/d0cp06091g
Frisch, M. J., Trucks, G. W., Schlegel, H. B., Scuseria, G. E., Robb, M. A., Cheeseman, J. R., et al. (2004). Gaussian 03, revision D.01. Wallingford, CT: Gaussian, Inc.
Goroff, N. S. (1996). Mechanism of fullerene formation. Acc. Chem. Res. 29 (2), 77–83. doi:10.1021/ar950162d
Hussain, S., Hussain, R., Mehboob, M. Y., Chatha, S. A. S., Hussain, A. I., Umar, A., et al. (2020). Adsorption of phosgene gas on pristine and copper-decorated B12N12 nanocages: A comparative DFT study. ACS Omega 5 (13), 7641–7650. doi:10.1021/acsomega.0c00507
Hussain, S., Shahid Chatha, S. A., Hussain, A. I., Hussain, R., Mehboob, M. Y., Gulzar, T., et al. (2020). Designing novel Zn-decorated inorganic B12P12 nanoclusters with promising electronic properties: A step forward toward efficient CO2 sensing materials. ACS Omega 5 (25), 15547–15556. doi:10.1021/acsomega.0c01686
Jouypazadeh, H., and Farrokhpour, H. (2018). DFT and TD-DFT study of the adsorption and detection of sulfur mustard chemical warfare agent by the C 24, C 12 Si 12, Al 12 N 12, Al 12 P 12, Be 12 O 12, B 12 N 12 and Mg 12 O 12 nanocages. J. Mol. Struct. 1164, 227–238. doi:10.1016/j.molstruc.2018.03.051
Kakemam, J., and Peyghan, A. A. (2013). Electronic, energetic, and structural properties of C- and Si-doped Mg12O12 nano-cages. Comput. Mat. Sci. 79, 352–355. doi:10.1016/j.commatsci.2013.06.036
Kataura, H., Maniwa, Y., Abe, M., Fujiwara, A., Kodama, T., Kikuchi, K., et al. (2002). Optical properties of fullerene and non-fullerene peapods. Appl. Phys. A 74 (3), 349–354. doi:10.1007/s003390201276
Kroto, H. W., Heath, J. R., O’Brien, S. C., Curl, R. F., and Smalley, R. E. (1985). C60: Buckminsterfullerene. Nature 318 (6042), 162–163. doi:10.1038/318162a0
Martín, N. (2006). New challenges in fullerene chemistry. Chem. Commun. (20), 2093–2104. doi:10.1039/b601582b
Mastalerz, M., Schneider, M. W., Oppel, I. M., and Presly, O. (2011). A salicylbisimine cage compound with high surface area and selective CO2/CH4 adsorption. Angew. Chem. Int. Ed. 50 (5), 1046–1051. doi:10.1002/anie.201005301
Mazloomi, K., and Gomes, C. (2012). Hydrogen as an energy carrier: Prospects and challenges. Renew. Sust. Energy Rev. 16 (5), 3024–3033. doi:10.1016/j.rser.2012.02.028
Nagarajan, V., and Chandiramouli, R. (2019). Acrylonitrile vapor adsorption studies on armchair arsenene nanoribbon based on DFT study. Appl. Surf. Sci. 494, 1148–1155. doi:10.1016/j.apsusc.2019.07.214
O'Boyle, N. M., Tenderholt, A. L., and Langner, K. M. (2008). cclib: A library for package-independent computational chemistry algorithms. J. Comput. Chem. 29 (5), 839–845. doi:10.1002/jcc.20823
Oku, T., Nishiwaki, A., and Narita, I. (2004). Formation and atomic structure of B12N12nanocage clusters studied by mass spectrometry and cluster calculation. Sci. Technol. Adv. Mat. 5 (5), 635–638. doi:10.1016/j.stam.2004.03.017
Pokropivny, V. V., and Ovsyannikova, L. I. (2007). Electronic structure and the infrared absorption and Raman spectra of the semiconductor clusters C24, B12N12, Si12C12, Zn12O12, and Ga12N12. Phys. Solid State 49 (3), 562–570. doi:10.1134/s1063783407030328
Pokropivny, V. V., Skorokhod, V. V., Oleinik, G. S., Kurdyumov, A. V., Bartnitskaya, T. S., Pokropivny, A. V., et al. (2000). J. Solid State Chem. 154 (1), 214.
Rad, A. S., and Ayub, K. (2016). Ni adsorption on Al12P12 nano-cage: A DFT study. J. Alloys Compd. 678, 317–324. doi:10.1016/j.jallcom.2016.03.175
Rezaei-Sameti, M., and Abdoli, S. K. (2020). The capability of the pristine and (Sc, Ti) doped Be12O12 nanocluster to detect and adsorb of mercaptopyridine molecule: A first principle study. J. Mol. Struct. 1205, 127593. doi:10.1016/j.molstruc.2019.127593
Shakerzdeh, E., Tahmasebi, E., and Shamlouei, H. R. (2015). The influence of alkali metals (Li, Na and K) interaction with Be12O12 and Mg12O12 nanoclusters on their structural, electronic and nonlinear optical properties: A theoretical study. Synth. Mater. 204, 17–24. doi:10.1016/j.synthmet.2015.03.008
Strout, D. L. (2000). Structure and stability of boron Nitrides: isomers of B12N12. J. Phys. Chem. A 104 (15), 3364–3366. doi:10.1021/jp994129a
Swetha, B., Nagarajan, V., Soltani, A., and Chandiramouli, R. (2020). Novel gamma arsenene nanosheets as sensing medium for vomiting agents: A first-principles research. Comput. Theor. Chem. 1185, 112876. doi:10.1016/j.comptc.2020.112876
Tan, L.-P., Die, D., and Zheng, B.-X. (2022). Growth mechanism, electronic properties and spectra of aluminum clusters. Spectrochim. Acta. A 267, 120545. doi:10.1016/j.saa.2021.120545
Tasis, D., Tagmatarchis, N., Bianco, A., and Prato, M. (2006). Chemistry of carbon nanotubes. Chem. Rev. 106 (3), 1105–1136. doi:10.1021/cr050569o
Vergara-Reyes, H. N., Acosta-Alejandro, M., and Chigo-Anota, E. (2021). Quantum-mechanical assessment of the adsorption of nitric oxide molecules on the magnetic carbon nitride (C36N24)− fullerene. Struct. Chem. 32, 1775–1786. doi:10.1007/s11224-021-01736-8
Yang, H., Song, Y., Zhang, Y., and Chen, H. (2018). Prediction of the electron redundant SinNn fullerenes. Phys. E 99, 208–214. doi:10.1016/j.physe.2018.02.010
Yarovsky, I., and Goldberg, A. (2005). DFT study of hydrogen adsorption on Al13clusters. Mol. Simul. 31 (6-7), 475–481. doi:10.1080/08927020412331337041
Yilanci, A., Dincer, I., and Ozturk, H. K. (2009). A review on solar-hydrogen/fuel cell hybrid energy systems for stationary applications. Prog. Energy Combust. Sci. 35 (3), 231–244. doi:10.1016/j.pecs.2008.07.004
Zhai, H.-J., Zhao, Y.-F., Li, W.-L., Chen, Q., Bai, H., Hu, H.-S., et al. (2014). Observation of an all-boron fullerene. Nat. Chem. 6, 727–731. doi:10.1038/nchem.1999
Zhang, W. J., Rabiei, S., Bagreev, A., Zhuang, M. S., and Rasouli, F. (2008). Study of NO adsorption on activated carbons. Appl. Catal. B-Environ. 83 (1), 63–71. doi:10.1016/j.apcatb.2008.02.003
Keywords: nanocage, Al12Si12, equilibrium geometries, stability, electronic properties
Citation: Li L-K, Ma Y-Q, Li K-N, Xie W-L and Huang B (2023) Structural and electronic properties of H2, CO, CH4, NO, and NH3 adsorbed onto Al12Si12 nanocages using density functional theory. Front. Chem. 11:1143951. doi: 10.3389/fchem.2023.1143951
Received: 13 January 2023; Accepted: 07 February 2023;
Published: 16 February 2023.
Edited by:
Renjith Thomas, Mahatma Gandhi University, IndiaReviewed by:
Ernesto Chigo, Meritorious Autonomous University of Puebla, MexicoJose Luis Cabellos, Polytechnic University of Tapachula, Mexico
Slavko Radenković, University of Kragujevac, Serbia
Copyright © 2023 Li, Ma, Li, Xie and Huang. This is an open-access article distributed under the terms of the Creative Commons Attribution License (CC BY). The use, distribution or reproduction in other forums is permitted, provided the original author(s) and the copyright owner(s) are credited and that the original publication in this journal is cited, in accordance with accepted academic practice. No use, distribution or reproduction is permitted which does not comply with these terms.
*Correspondence: Kang-Ning Li, a25saUBueHUuZWR1LmNu