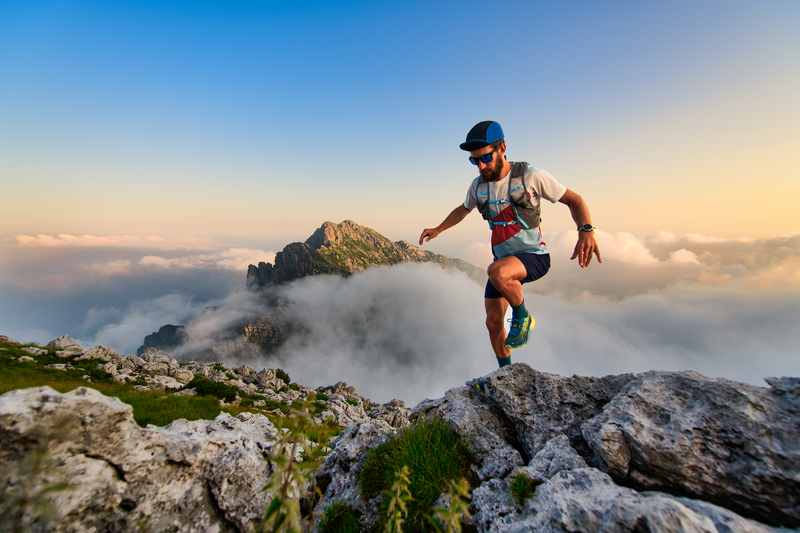
95% of researchers rate our articles as excellent or good
Learn more about the work of our research integrity team to safeguard the quality of each article we publish.
Find out more
ORIGINAL RESEARCH article
Front. Chem. , 23 February 2023
Sec. Electrochemistry
Volume 11 - 2023 | https://doi.org/10.3389/fchem.2023.1129133
This article is part of the Research Topic Advanced Electrocatalytic Materials for Water Electrolysis and Fuel Cells View all 5 articles
Attractive technology for producing sustainable hydrogen with water electrolyzers was foreseen as one of the most promising ways to meet the increasing demands of renewable resources and electricity storage. Mainly used for the efficient generation of H2, water electrolysis involving hydrogen evolution reactions (HERs) depends on efficient and affordable electrocatalysts. Hydrogen is an effective fuel that can be produced by splitting water. Hence, the search for highly efficient HER catalysts is a major challenge as efficient hydrogen evolution catalysts are sought to replace catalysts such as platinum. Here, we describe a low-cost and highly effective electrocatalyst for the proper incorporation of the HER electrocatalyst with low overpotential, effective charge transfer kinetics, low Tafel slope, and good durability. By using a simple hydrothermal approach to produce Co3(PO4)2.8H2O/CNF, it is possible to attach Co3(PO4)2.8H2O to the surface of carbon nanofibers (CNFs), which also exhibit remarkable HER activity at an overpotential of 133 mV and produce a current density of 10 mA/cm2 and a 48 mV/decade for the Tafel slope. Large electrochemical surface areas and easy charge transfer from Co3(PO4)2.8H2O to the electrode through conductive Co3(PO4)2.8H2O/CNF composites are the reasons for the improved performance of Co3(PO4)2.8H2O/CNF.
The energy crisis, environmental degradation, and global warming—all driven by the extensive use of fossil fuels—have motivated the production of clean and renewable energy. Currently, due to the accelerating spread of the global energy crisis and the ongoing decay of traditional fossil fuels, a clean, high-energy-density hydrogen economy derived from renewable sources is being extensively studied and developed (Zou and Zhang, 2015). The H2 evolution reaction (HER) critically depends on appropriate electrocatalysts such as platinum (Pt) and its alloys, which catalyze the conversion of pairs of protons and electrons to H2 at high reaction rates with low overpotentials. However, the high price and relative scarcity of Pt severely limits its widespread use. Therefore, finding reliable and effective alternative catalysts that are geologically abundant is imperative for the future of the hydrogen economy (Gao et al., 2015). The development of an inexpensive HER electrocatalyst, using base elements with superior activity and high stability as a replacement for expensive platinum, has been one of the most pressing goals in recent years. In recent decades, several studies have been conducted to replace noble-metal-based electrocatalysts (Bui et al., 2020).
Because of its ability to catalyze the splitting of water, cobalt (Co) has become an intriguing base metal. The preparation of Co-based composites and complexes using homogeneous molecular catalysts has received much research attention (Anjum et al., 2018; Ahmed et al., 2021; Hu et al., 2021a; Singh et al., 2022a; Singh et al., 2022b). In addition, cobalt-based electrocatalysts (CoP, Co3O4, CoOOH, CoSe2, etc.) have attracted significant attention for a variety of applications, including sensors (Hu et al., 2021b; Sari et al., 2022), supercapacitors (Rovetta et al., 2017; Li et al., 2018), lithium-ion batteries (Khan et al., 2016), and OER (Sun et al., 2021; Biswas et al., 2022; Kubba et al., 2022). Because of their strong electrochemical activity, cobalt phosphates have attracted much attention in recent decades and have been used extensively in electrochemical energy storage and as electrocatalysts for water splitting (Shu et al., 2018; Majhi and Yadav, 2021a). An inductive influence due to the presence of (PO4)3 groups means that the redox coupling of the transition metal is significantly higher than that of the comparable oxide. In addition, the excellent ionic conductivity of the large (PO4)3 units creates open pathways that can facilitate rapid ionic migration (Samal et al., 2016). Carbon-based materials (carbon nanofibers and carbon nanotubes) have attracted significant interest in an attempt to improve the long-term stability of the catalysts, while transition metal oxides (Zhao et al., 2018; Zhu et al., 2019; Ahmed et al., 2021; Majhi and Yadav, 2021b), sulfides (Biswas et al., 2021; Majhi and Yadav, 2021c; Sun et al., 2021), and phosphates (Ahmed et al., 2022; Majhi and Yadav, 2022a; Majhi and Yadav, 2022b) of other transition metals have been used as binding and support materials due to their excellent corrosion resistance, good conductivity, and adjustable chemical surface properties. Carbon-based materials have received significant attention due to their adaptable surface chemistry, strong conductivity, and excellent corrosion resistance. Therefore, to achieve highly efficient overall water splitting to produce clean H2, a rational design of electrocatalysts is required. This must account for processing costs, catalytic activity, and long-term stability (Woo et al., 2020).
In this work, we synthesized a hydrated phosphate-based carbon-nanofiber-supported material (Co3(PO4)2.8H2O/CNFs) to study the HER performance of the catalyst in acidic media. Hydrated cobalt phosphate with carbon nanofibers has shown excellent stability over 24 h, implying its superior stability during the HER reaction. The composite helps increase the highly electroactive surface area, high conductivity, and vertical growth relative conducting CNFs, exposing a high density of edge phosphate. Other factors that increase the activity of Co3(PO4)2.8H2O/CNFs are high current density, low Tafel slope, and low charge transfer resistance, which are 133 mV, 48 dec/cm1, and 43.04, respectively. The developed catalyst showed electrocatalytic performance comparable to commercial Pt/C in the acidic HER medium.
Cobalt (II) chloride (CoCl2), diammonium hydrogen phosphate (NH4)2HPO4, commercially available CNF, ethanol (99.9% AR grade), and potassium hydroxide (KOH) were purchased from Loba Chemie, and platinum carbon (Pt/C) and 5% Nafion™ 117 solution were purchased from Sigma-Aldrich. All chemicals were stored in a dry place and used without any further purification.
Co3(PO4)2.8H2O/CNFs were prepared using a facile hydrothermal method. In a typical synthesis, 259.6 mg of CoCl2 was dispersed in DIH2O and then 45 mg of CNF (commercial) was added, followed by stirring for 10 min. The reaction mixture was transferred to a Teflon-lined autoclave for hydrothermal treatment at 180°C for 24 h. The sample was collected by centrifugation and washed several times with both distilled water and ethanol to remove undesired species, and the product was dried in an oven at 70°C for 12 h.
To identify the crystallographic phases and the formation of synthesized Co3(PO4)2.8H2O/CNF, along with Co3(PO4)2.8H2O and CNF, an X-ray diffraction (XRD) analysis was conducted; the results are shown in Figure 1 and Figure 2. The XRD pattern of the hydrothermal synthesized (Co3(PO4)2.8H2O/CNF) catalyst is intact and well-matched with the ICSD card no: 00-033-0432 of hydrated cobalt phosphate, and most prominent broad diffraction peaks assigned to the (002) plane of CNF at the 2θ position of 26.35° are present (Figure 1A). This outcome primarily suggests the presence of hydrate molecules in the hydrothermally synthesized Co3(PO4)2.8H2O/CNF catalyst. The XRD pattern exhibited dominant peaks at 2θ with values of 11.26°, 13.26°, 18.28°, 19.62°, 21.97°, 23.19°, 27.97°, 30.31°, 33.17°, 33.36°, 35.67°, 37.31°, 40.79°, and 41.53°, corresponding to the lattice planes of (110), (0 2 0), (2 0 0), (−1 0 1), (1 3 0), (1 0 1), (0 3 1), (2 1 1), (−3 2 1), (−1 4 1), (1 4 1), (3 0 1), (−3 4 1), and (−2 5 1), respectively. The diffraction patterns of Co3(PO4)2.8H2O (Figure 1B) indexed well with the monoclinic phase 12/m space group standard patterns, and no other peaks were detected in the XRD patterns. However, the XRD pattern of Co3(PO4)2.8H2O/CNF (Figure 1C) showed peaks corresponding to both Co3(PO4)2.8H2O and CNF, indicating the formation of a Co3(PO4)2.8H2O/CNF hybrid structure. The observed peaks had high intensities, clearly showing that the as-prepared samples were highly crystalline. No other impurities were found. The levels of the high-intensity peaks are highlighted in the composite spectra.
FIGURE 1. (A) XRD pattern of CNF, (B) Co3(PO4)2.8H2O, and (C) Co3(PO4)2.8H2O/CNF composite structure.
FIGURE 2. (A) XRD pattern of the Co3(PO4)2.8H2O/CNF catalyst, (B) Rietveld-refined XRD pattern of the Co3(PO4)2.8H2O/CNF catalyst, and (C) VESTA-generated polyhedral ball–stick model crystal structure of the monoclinic crystal lattice of the Co3(PO4)2.8H2O phase with unit cell volume 603.095581 Å3 (drawn with solid orange lines), where a = 10.02100 Å, b = 13.32390 Å, c = 4.67245 Å, α = γ = 90°, and β = 104.8240°.
Furthermore, for comprehensive conclusiveness, we performed the Rietveld refinement of the PXRD pattern of Co3(PO4)2.8H2O/CNF (Figure 2) by FullProf Suite software. The crystal structure was generated using a refined CIF file in VESTA software. As shown by the atomic positions given in Supplementary Table S2, we clearly identified the presence of hydrogen atoms along with the copper, phosphorus, and oxygen atoms. These hydrogen atoms came from the H2O molecules conjugated with the Co3(PO4)2 lattice. Now, the Co3(PO4)2.8H2O lattice crystallizes into a 2D monoclinic structure with the space group C12/m 1. It is composed of two Co3P2(OH)16 sheets with preferential orientation in the (0 1 0) direction. Among the two inequivalent Co2⁺ sites, the first site (corresponding to the Co1 atom at the 2a Wyckoff site) is bonded to six O2⁻ atoms in the CoO₆ octahedron, which shares corners with the two equivalent PO₄ tetrahedra. There are two shorter (2.0624 Å) and four longer (2.1646 Å) Co–O bond lengths. At the second Co2⁺ site (corresponding to the Co2 atom at the 4g Wyckoff site), Co2⁺ is bonded to six O2⁻ atoms to form a CoO₆ octahedron that shares corners with four equivalent PO₄ tetrahedra and an edge with one CoO₆ octahedron. The Co–O bond distances range between 2.0755 and 2.1822 Å. P⁵⁺ is bonded to four O2⁻ atoms to occupy the PO₄ tetrahedral site that shares corners with five CoO₆ octahedra. The P–O bond distances range from 1.5529 to 1.5910 Å. There are five inequivalent O2⁻ sites; among them, at the first O2⁻ site (corresponding to the O1 atom), O2⁻ is bonded in a nine-coordinate geometry to one Co2⁺ and eight H1⁺ atoms (3 H1, 3 H2, H3, and H4). At the second O2⁻ site (corresponding to the O2 atom), O2⁻ is bonded in a six-coordinate geometry to one Co2⁺ atom and five H1⁺ atoms (2 H1, 2 H3, and H4). At the third O2⁻ site (corresponding to the O3 atom), O2⁻ is bonded in a four-coordinate geometry to one Co2⁺, one P⁵⁺, and two equivalent H1⁺ atoms (H3 and H4). At the fourth O2⁻ site (corresponding to the O4 atom), O2⁻ is bonded in a three-coordinate geometry to two equivalent Co2⁺ atoms and one P⁵⁺ atom. At the fifth O2⁻ site (corresponding to the O5 atom), O2⁻ is bonded in a six-coordinate geometry to one Co2⁺, one P⁵⁺, and four equivalent H1⁺ atoms (H2). Among the four inequivalent H1⁺ sites, at the first H1⁺ site (corresponding to the H1 atom), H1⁺ is bonded in a five-coordinate single-bond geometry to five O2⁻ atoms (3 O1 and 2 O2), with a shorter H–O bond length of 0.8835 Å (O2) and a longer H–O bond length of 2.8099 Å (O1). At the second H1⁺ site (corresponding to the H2 atom), H1⁺ is bonded in a five-coordinate single-bond geometry to five O2⁻ atoms (3 O1 and 2 O5), with a shorter H–O bond length of 0.9470 Å (O1) and a longer H–O bond length of 2.6647 Å (O5). At the third H1⁺ site (corresponding to the H3 atom), H1⁺ is bonded in a four-coordinate single-bond geometry to four O2⁻ atoms (O1, 2 O2, and 2 O3), with a shorter H–O bond length of 0.8675 Å (O2) and a longer H–O bond length of 2.8224 Å (O1). At the fourth H1⁺ site (corresponding to the H4 atom), H1⁺ is bonded in a three-coordinate single-bond geometry to three inequivalent O2⁻ atoms (O1, O2, and O3) with H–O bond lengths of 0.6671 Å (O1), 2.7845 Å (O1), and 2.1287 Å (O5). Thus, we can conclude from the crystal structure symmetry that the Co3(PO4)2 phase contains water molecules in the Co3(PO4)2.8H2O/CNF composite system as we synthesized it in hydrothermal treatment and because at low temperature, the Co3(PO4)2 phase is stable in its ortho-hydrate due to its thermodynamical preferences.
Fourier-transform infrared spectroscopy (FTIR), thermogravimetric analysis (TGA), and derivative thermogravimetric (DTG) analysis are presented in Figure 3. The FTIR spectrum (Figure 3A) demonstrates that the stretching of the P–O–P linkages is attributed to the peaks at 714 cm−1 and 855 cm−1 and shows the vibrational mode of PO43-. The strong absorption asymmetric vibration mode of the PO4 group was observed at 1027 cm−1. The characteristic band at 1611 cm−1 represents the bending vibration mode of a water molecule (H–O–H). Adsorbed water is represented by a broad absorption band from 3051 to 3448 cm−1 and can be assigned to the vibrational bond of O–H. These results indicate that the deposited material contains structural water and formation of hydrous cobalt phosphate (Co3(PO4)2.8H2O)/CNF.
FIGURE 3. (A) FTIR analysis, and (B) TGA (blue, left axis) and DTG (red, right axis) analysis of Co3(PO4)2·8H2O.
At higher temperatures and treatment in an inert atmosphere, the cobalt cation and the phosphate anion reinstall a crystal structure. The removal of coordinated water molecules is also confirmed by thermal derivative thermogravimetric (DTG) and gravimetric analysis (TGA), as shown in Figure 3B. The thermogram of the Co3(PO4)2.8H2O/CNF material exhibits two distinct loss stages. The first step shows the initial 4.8% weight loss in the temperature range of 105 °C; in the second stage, a 19.3% weight loss is observed in the temperature range of 106°C–153 C due to the loss of water. The total weight loss of the sample was found to be 46.2% when the temperature was up to 700°C.
The surface morphology of the Co3(PO4)2.8H2O/CNF material was observed by FE-SEM. Typical FE-SEM images of a synthesized sample are shown in Figure 4. The FE-SEM image of Co3(PO4)2.8H2O shows a rod-shaped flower (Figure 4A) and resembles CNF with a thread-like morphology (Figure 4B). The Co3(PO4)2.8H2O/CNF composite shows that cobalt phosphate flowers are stacked with a uniform and regular structure on the surface of carbon nanofibers; images of the composite are shown in Figures 4C, D. It can be observed that the surface morphology of cobalt phosphate is a tightly packed flower, which may suggest support for effective electron transport. In addition, the elemental color map of the Co3(PO4)2.8H2O/CNF composite is shown in Figures 5A–E, where it can be observed that the distribution of Co, O, P, and C is suboptimal, resulting in the exposed substrate observed in these pictures. Energy-dispersive X-ray spectroscopy (EDX) was performed to confirm the elemental composition and is shown in Figure 5F, where all elements are presented while their ratios in weight percentage and atomic percentage are included in Figure 5F. The FE-SEM image of Co3(PO4)2.8H2O distribution over CNF, where a large percentage of cobalt phosphate is distributed over CNF, could indicate the increase in conductivity essential for enhancing the electrocatalytic water-splitting reaction.
FIGURE 5. (A) Mapping selected image. (B) Mapping of Co, (C) P, (D) O, (E) C, and (F) EDX of the Co3(PO4)2.8H2O/CNF composite flower.
XPS measurements were performed to validate the chemical composition and oxidation state of Co3(PO4)2.8H2O/CNF. The XPS survey spectra of the composite are presented in Supplementary Figure S4, and the high-resolution XPS spectra of Co 2p, P 2p, O 1s, and C 1s are shown in Figure 6. The high-resolution Co 2p orbital consists of two spin-orbit components of 2p3/2 and 2p1/2 for the Co2+ and Co3+ states. The two peaks at the binding energies 780.87 and 796.82 eV are assigned to the Co3+ state, and 782.71 and 798.34 eV are assigned to the Co2+ state for the Co 2p3/2 and 2p1/2 nuclear levels, respectively. Co 2p shows two satellite peaks of Co 2p3/2 and Co 2p1/2 core levels (Figure 6A) (Song et al., 2020). The P 2p region of Co3(PO4)2.8H2O/CNF shows two characteristic peaks at a binding energy of 132.97 eV and 133.95 eV, corresponding to the 2p3/2 nuclear levels and 2p1/2, which can be assigned to the phosphate group (Figure 4B). O1s signals are centered at a binding energy of 530.78, and 531.68 eV corresponds to the phosphate oxygen and OH group of H2O molecules present in the lattice (Figure 6C). In the C1s spectrum (Figure 6D), the peaks centered at about 284.30, 285.63, and 287.77 eV are indicated on sp2-hybridized C-C, C-N, and C-O, respectively (Yuan et al., 2016).
FIGURE 6. High-resolution deconvoluted XPS spectra of (A) Co 2p, (B) P 2p, (C) O 1s, and (D) C 1s energy levels in the Co3(PO4)2.8H2O/CNF composite structure.
The linear sweep voltammetry (LSV) curves were measured in the potential window range from 0 V to -1 V versus Ag/AgCl for the HER process, with a sampling rate of 10 mV s−1 and 0.5 M H2SO4 solution as the electrolyte. The long-term HER stability test of the catalyst was performed using an Ag/AgCl electrode in the acidic medium. Electrochemical impedance spectroscopy (EIS) was performed in 0.5 M H2SO4 solution over the frequency range of 100 kHz to 0.1 Hz, at an overpotential of 400 mV. The HER electrocatalytic activity of Co3(PO4)2.8H2O/CNF, Co3(PO4)2.8H2O, and carbon nanofiber (CNF) was assessed by linear sweep voltammetry (LSV) and compared to data obtained for commercial Pt/C as a reference (Figure 7). Co3(PO4)2.8H2O/CNF had the lowest overpotential among all the catalysts, indicating its superior HER activity. Overpotentials of the catalysts are given in Table 1, with the composites Co3(PO4)2.8H2O/CNF, Co3(PO4)2.8H2O, CNF, and Pt/C having overpotential values of 133 mV, 188 mV, 275 mV, and 32 mV, respectively. Record a current density of 10 mA/cm2 (Figure 7A). Using the Volmer and Heyrovsky equation (η = a + b log j, where η is over potential, j is current density, b is Tafel slope and a is constant), we calculated the Tafel slopes of the linear domains to determine the kinetics of the catalysts. We found the Tafel slope values for composites Co3(PO4)2.8H2O/CNF, Co3(PO4)2.8H2O, CNF, and Pt/C to be 48 mV/dec1, 87 mV/dec1, 106 mV/dec1, and 36 mV/dec1 where, the composite Co3(PO4)2.8H2O/CNF showed the fastest HER kinetics among all the catalysts prepared (Figure 7B). The long-term cyclic stability of the Co3(PO4)2.8H2O/CNF electrocatalyst toward HER activity was also tested, using 0.5 M H2SO4 solution as the electrolyte for 3000 continuous LSV cycles (Figure 7C). After the stability test, it was found that the overpotential rise was only 8 mV at a current density of 10 mA/cm2, proving the superior long-term stability of the Co3(PO4)2.8H2O/CNF catalyst to HER activity in the acidic medium. Furthermore, long-term stability was tested by chronoamperometry using 0.5 M H2SO4 solution as the electrolyte at a constant overvoltage of 133 mV for 24 h (Figure 7D), and the result shows a very high durability with negligible loss in current density of the catalyst.
FIGURE 7. (A) Polarization curve (LSV) plot and (B) corresponding Tafel plot of Co3(PO4)2.8H2O/CNF, Co3(PO4)2.8H2O, and CNF, and Pt/C. (C) Chronoamperometry method of Co3(PO4)2.8H2O/CNF at an overpotential of 133 mV for 24 h. (D) Polarization curves of Co3(PO4)2.8H2O/CNF of 1st and 3000th cycles of continuous operation.
TABLE 1. Summary of the electrochemical HER activity of Co3(PO4)2.8H2O/CNF, Co3(PO4)2.8H2O, CNF, and Pt/C catalysts in the acidic medium.
Figure 8A represents electrochemical impedance spectroscopy (EIS), where Co3(PO4)2.8H2O/CNF is characterized by the smallest Nyquist plot radius, followed by Co3(PO4)2.8H2O and CNF, indicating a lower charge transfer resistance for indicating these three electrocatalysts. The charge transfer resistances of Co3(PO4)2.8H2O/CNF composite catalysts, Co3(PO4)2.8H2O, and CNF were 43.04 Ω, 84.12 Ω, and 136.19 Ω, respectively, with the Co3(PO4)2.8H2O/CNF composite having the lowest value compared to the other catalysts, indicating its fast kinetic process in HER activity. Mass loading of 0.707 mg/cm2 was introduced onto the prepared Co3(PO4)2.8H2O/CNF electrode to catalyze the HER under acidic conditions in a three-electrode configuration. For deep understanding of the influence of cobalt phosphate nano fibers (Co3(PO4)2.8H2O/CNF) we have also determine the mass activity (MA) of catalysts by mass loading (presented in Figure 8B) at an overvoltage of 133 mV. Co3(PO4)2.8H2O/CNF has the highest mass activity of 169.30 A g−1, compared to its counterparts Co3(PO4)2.8H2O (119.34 A g−1) and CNF (42.00 A g-1). Mass activity was calculated using the following equation:
FIGURE 8. (A) Electrochemical impedance spectroscopy (EIS) plots and (B) mass activity of the catalysts.
The HER process proceeds through three principal steps, called the Volmer, Heyrovsky, and Tafel steps, in the acidic medium (Pentland et al., 1957; Conway and Tilak, 2002; Xie et al., 2013; Basu et al., 2017). The Volmer reaction is associated with proton absorption, which is a primary discharge step (Step 1). The Heyrovsky step is the electrochemical desorption stage (i.e., the combination of a second proton with an absorbed H atom of H2 gas) (Step 2). The Tafel step is a recombination step (i.e., the combination of two nearby absorbed H atoms to produce H2 gas) (Step 3).
where Hads represents a hydrogen atom chemically adsorbed on an active site of the catalyst surface (M). If the Volmer reaction is the rate-determining step, then the Tafel slope should be 120 mV dec−1, and for the Heyrovsky process and Tafel process, Tafel slopes of 40 and 30 mV dec−1 should be obtained, respectively (Xie et al., 2013; Hu et al., 2016). Therefore, combinations of steps (i.e., the Volmer–Heyrovsky or Volmer–Tafel pathways) are required to produce molecular hydrogen in a complete HER process.
In addition, the electrochemical double layer capacitance (Cdl) and the electrochemically active surface area (ECSA) were investigated by cyclic voltammetry (CV) performed at different sampling rates, from 10–50 mV s−1 (Figure 9A). Cdl was estimated by measuring voltammograms in a non-Faradic region, and Cdl was measured to determine the origin of the high HER activity of Co3(PO4)2.8H2O/CNF composite nanostructures. Both the anodic and cathodic double-layer charging currents (Ja and Jc, respectively) were calculated, and the values were plotted against the corresponding sample rates. Thus, the calculated Cdl for the Co3(PO4)2.8H2O/CNF composite is shown in Figure 9B and is 3.072 mF cm−2; the corresponding ECSA is 76.97 cm2, and 1.66/41.7 and 0.83/20.96 for the Co3(PO4)2.8H2O and CNF catalysts Cdl/ECSA, respectively (Supplementary Figures S1C, D and Table 1). The Brunauer–Emmett–Teller (BET) study shows that Co3(PO4)2.8H2O/CNF has the highest surface area of 37.6 m2 g−1 compared to other constituents Co3(PO4)2.8H2O (28.2 m2 g−1) and CNF (21.4 m2 g−1); the results for these constituents are displayed in Supplementary Figure S2. The catalyst possesses excellent durability and stability after 20 h, without apparent chemical or structural deformation; XRD and FE-SEM after stability measurements are presented (Supplementary Figure S3).
FIGURE 9. (A) Cyclic voltammetry curves and (B) the corresponding plots of Ja and Jc against the scan rate for the determination of double-layer capacitance (Cdl) of the Co3(PO4)2.8H2O/CNF composite catalysts.
Co3(PO4)2.8H2O/CNF and Co3(PO4)2.8H2O were synthesized by a simple hydrothermal procedure. The structural characterizations confirmed the formation of Co3(PO4)2.8H2O/CNF with a flower-like structure attached over carbon nanofibers. The synthesized catalyst Co3(PO4)2.8H2O/CNF shows excellent performance for HER with the low overpotential (133 mV) required to generate current densities of 10 mA cm−2, a small Tafel slope (48 mV decade−1), and good stability at 24 h. The composite helps increase the high electroactive surface area, high conductivity, and vertical growth over conductive CNFs, exposing a high density of edge phosphate. This newly developed [Co3(PO4)2.8H2O/CNF] can be considered a promising electrocatalyst for HER in acidic media because of its straightforward synthetic procedure and low cost.
The original contributions presented in the study are included in the article/Supplementary Material; further inquiries can be directed to the corresponding author.
IA: conceptualization, investigation, methodology, writing, review, and editing. RB: investigation, and review and editing. RS: writing and editing. VB: writing and editing. KH: supervision, conceptualization, methodology, writing, review, and editing.
IA is thankful to CSIR for awarding fellowship (File No. 09/1051(0036)/2019-EMR-I). RB is thankful to the DST for providing the INSPIRE Ph.D. fellowship program (DST/INSPIRE Fellowship/[IF190052]).
The authors declare that the research was conducted in the absence of any commercial or financial relationships that could be construed as a potential conflict of interest.
All claims expressed in this article are solely those of the authors and do not necessarily represent those of their affiliated organizations, or those of the publisher, the editors, and the reviewers. Any product that may be evaluated in this article, or claim that may be made by its manufacturer, is not guaranteed or endorsed by the publisher.
The Supplementary Material for this article can be found online at: https://www.frontiersin.org/articles/10.3389/fchem.2023.1129133/full#supplementary-material
Ahmed, I., Biswas, R., Patil, R. A., Halder, K. K., Singh, H., Banerjee, B., et al. (2021). Graphitic carbon nitride composites with MoO3-decorated Co3O4 nanorods as catalysts for oxygen and hydrogen evolution. ACS Appl. Nano Mater. 4, 12672–12681. doi:10.1021/acsanm.1c03238
Ahmed, I., Biswas, R., Singh, H., Patil, R. A., Varshney, R., Patra, D., et al. (2022). Green synthesis of hybrid papain/Ni3(PO4)2 rods electrocatalyst for enhanced oxygen evolution reaction. New J. Chem. 46, 22237–22245. doi:10.1039/d2nj03700a
Anjum, M. A. R., Bhatt, M. D., Lee, M. H., and Lee, J. S. (2018). Sulfur-doped dicobalt phosphide outperforming precious metals as a bifunctional electrocatalyst for alkaline water electrolysis. Chem. Mater. 30, 8861–8870. doi:10.1021/acs.chemmater.8b03908
Basu, M., Nazir, R., Mahala, C., Fageria, P., Chaudhary, S., Gangopadhyay, S., et al. (2017). Ag2S/Ag heterostructure: A promising electrocatalyst for the hydrogen evolution reaction. Langmuir 33, 3178–3186. doi:10.1021/acs.langmuir.7b00029
Biswas, R., Thakur, P., Ahmed, I., Rom, T., Ali, M. S., Patil, R. A., et al. H(2022). Coupling nonstoichiometric Zn0.76Co0.24S with NiCo2S4 composite nanoflowers for efficient synergistic electrocatalytic oxygen and hydrogen evolution reactions. Energy & Fuels 37, 604–613. doi:10.1021/acs.energyfuels.2c03384
Biswas, R., Thakur, P., Kaur, G., Som, S., Saha, M., Jhajhria, V., et al. (2021). Interfacial engineering of CuCo2S4/g-C3N4 hybrid nanorods for efficient oxygen evolution reaction. Inorg. Chem. 60, 12355–12366. doi:10.1021/acs.inorgchem.1c01566
Bui, V. Q., Kumar, A., Bui, H. T., Lee, J., Hwang, Y., Le, H. M., et al. (2020). Boosting electrocatalytic HER activity of 3D interconnected CoSP via metal doping: Active and stable electrocatalysts for pH-universal hydrogen generation. Chem. Mater. 32, 9591–9601. doi:10.1021/acs.chemmater.0c03052
Conway, B., and Tilak, B. (2002). Interfacial processes involving electrocatalytic evolution and oxidation of H2, and the role of chemisorbed H. Electrochimica Acta 47, 3571–3594. doi:10.1016/s0013-4686(02)00329-8
Gao, M.-R., Liang, J.-X., Zheng, Y.-R., Xu, Y.-F., Jiang, J., Gao, Q., et al. (2015). An efficient molybdenum disulfide/cobalt diselenide hybrid catalyst for electrochemical hydrogen generation. Nat. Commun. 6, 5982–5987. doi:10.1038/ncomms6982
Hu, E., Yao, Y., Chen, Y., Cui, Y., Wang, Z., and Qian, G. (2021). Cu2+-Guided construction of the amorphous CoMoO3/Cu nanocomposite for highly efficient water electrolysis. ACS Appl. Energy Mater. 4, 6740–6748. doi:10.1021/acsaem.1c00812
Hu, F. X., Hu, T., Chen, S., Wang, D., Rao, Q., Liu, Y., et al. (2021). Single-atom cobalt-based electrochemical biomimetic uric acid sensor with wide linear range and ultralow detection limit. Nano-micro Lett. 13, 7–13. doi:10.1007/s40820-020-00536-9
Hu, T., Bian, K., Tai, G., Zeng, T., Wang, X., Huang, X., et al. (2016). Oxidation-sulfidation approach for vertically growing MoS2 nanofilms catalysts on molybdenum foils as efficient HER catalysts. J. Phys. Chem. C 120, 25843–25850. doi:10.1021/acs.jpcc.6b08120
Khan, I., Nasim, F., Choucair, M., Ullah, S., Badshah, A., and Nadeem, M. (2016). Cobalt oxide nanoparticle embedded N-CNTs: Lithium ion battery applications. RSC Adv. 6, 1129–1135. doi:10.1039/c5ra23222h
Kubba, D., Ahmed, I., Kour, P., Biswas, R., Kaur, H., Yadav, K., et al. (2022). LaCoO3 perovskite nanoparticles embedded in NiCo2O4 nanoflowers as electrocatalysts for oxygen evolution. ACS Appl. Nano Mater. 11, 16344–16353. doi:10.1021/acsanm.2c03395
Li, X., Xiao, X., Li, Q., Wei, J., Xue, H., and Pang, H. (2018). Metal (M= Co, Ni) phosphate based materials for high-performance supercapacitors. Inorg. Chem. Front. 5, 11–28. doi:10.1039/c7qi00434f
Majhi, K. C., and Yadav, M. (2021). Bimetallic chalcogenide nanocrystallites as efficient electrocatalyst for overall water splitting. J. Alloys Compd. 852, 156736. doi:10.1016/j.jallcom.2020.156736
Majhi, K. C., and Yadav, M. (2022). Facile hydrothermal synthesis of rare Earth phosphate for boosting hydrogen evolution reaction. Int. J. Hydrogen Energy 47, 14092–14103. doi:10.1016/j.ijhydene.2022.02.168
Majhi, K. C., and Yadav, M. (2022). Neodymium oxide doped neodymium phosphate as efficient electrocatalyst towards hydrogen evolution reaction in acidic medium. J. Environ. Chem. Eng. 10, 107416. doi:10.1016/j.jece.2022.107416
Majhi, K. C., and Yadav, M. (2021). Palladium oxide decorated transition metal nitride as efficient electrocatalyst for hydrogen evolution reaction. J. Alloys Compd. 855, 157511. doi:10.1016/j.jallcom.2020.157511
Majhi, K. C., and Yadav, M. (2021). Sphere-shaped bimetallic sulphoselenide: An efficient electrocatalyst for hydrogen evolution reaction. Energy & Fuels 35, 12473–12481. doi:10.1021/acs.energyfuels.1c01079
Pentland, N., Bockris, J. M., and Sheldon, E. (1957). Hydrogen evolution reaction on copper, gold, molybdenum, palladium, rhodium, and iron: Mechanism and measurement technique under high purity conditions. J. Electrochem. Soc. 104, 182. doi:10.1149/1.2428530
Rovetta, A., Browne, M., Harvey, A., Godwin, I., Coleman, J., and Lyons, M. (2017). Cobalt hydroxide nanoflakes and their application as supercapacitors and oxygen evolution catalysts. Nanotechnology 28, 375401. doi:10.1088/1361-6528/aa7f1b
Samal, A., Swain, S., Satpati, B., Das, D. P., and Mishra, B. K. (2016). 3D Co3(PO4)2–reduced graphene oxide flowers for photocatalytic water splitting: A type II staggered heterojunction system. ChemSusChem 9, 3150–3160. doi:10.1002/cssc.201601214
Sari, S. R., Tsushida, M., Sato, T., and Tominaga, M. (2022). Highly sensitive detection of phosphate using well-ordered crystalline cobalt oxide nanoparticles supported by multi-walled carbon nanotubes. Mater. Adv. 3, 2018–2025. doi:10.1039/d1ma01097b
Shu, Y., Li, B., Chen, J., Xu, Q., Pang, H., and Hu, X. (2018). Facile synthesis of ultrathin nickel–cobalt phosphate 2D nanosheets with enhanced electrocatalytic activity for glucose oxidation. ACS Appl. Mater. interfaces 10, 2360–2367. doi:10.1021/acsami.7b17005
Singh, H., Ahmed, I., Biswas, R., Mete, S., Halder, K. K., Banerjee, B., et al. (2022). Genomic DNA-mediated formation of a porous Cu2(OH)PO4/Co3(PO4)2·8H2O rolling pin shape bifunctional electrocatalyst for water splitting reactions. RSC Adv. 12, 3738–3744. doi:10.1039/d1ra09098d
Singh, H., Biswas, R., Ahmed, I., Thakur, P., Kundu, A., Panigrahi, A. R., et al. (2022). Dumbbell-shaped ternary transition-metal (Cu, Ni, Co) phosphate bundles: A promising catalyst for the oxygen evolution reaction. ACS Appl. Mater. Interfaces 14, 6570–6581. doi:10.1021/acsami.1c20356
Song, Z., Zhu, X., Zeng, Y., Wang, A., Li, S., Fan, Y., et al. (2020). Cobalt phosphate cocatalyst loaded-CdS nanorod photoanode with well-defined junctions for highly efficient photoelectrochemical water splitting. Catal. Lett. 150, 1878–1889. doi:10.1007/s10562-019-03084-z
Sun, Y., Li, C., Jiang, S., Xia, R., Wang, X., Bao, H., et al. (2021). Comparative study on supercapacitive and oxygen evolution reaction applications of hollow nanostructured cobalt sulfides. Nanotechnology 32, 385401. doi:10.1088/1361-6528/ac09aa
Woo, S., Lee, J., Lee, D. S., Kim, J. K., and Lim, B. (2020). Electrospun carbon nanofibers with embedded co-ceria nanoparticles for efficient hydrogen evolution and overall water splitting. Materials 13, 856. doi:10.3390/ma13040856
Xie, J., Zhang, J., Li, S., Grote, F., Zhang, X., Zhang, H., et al. (2013). Controllable disorder engineering in oxygen-incorporated MoS2 ultrathin nanosheets for efficient hydrogen evolution. J. Am. Chem. Soc. 135, 17881–17888. doi:10.1021/ja408329q
Yuan, C.-Z., Jiang, Y.-F., Wang, Z., Xie, X., Yang, Z.-K., Yousaf, A. B., et al. (2016). Cobalt phosphate nanoparticles decorated with nitrogen-doped carbon layers as highly active and stable electrocatalysts for the oxygen evolution reaction. J. Mater. Chem. A 4, 8155–8160. doi:10.1039/c6ta01929c
Zhao, W., Wang, S., Feng, C., Wu, H., Zhang, L., and Zhang, J. (2018). Novel cobalt-doped Ni0.85Se chalcogenides (Co<i>x</i>Ni0.85–<i>x</i>Se) as high active and stable electrocatalysts for hydrogen evolution reaction in electrolysis water splitting. ACS Appl. Mater. Interfaces 10, 40491–40499. doi:10.1021/acsami.8b12797
Zhu, M., Yu, S., Ge, R., Feng, L., Yu, Y., Li, Y., et al. (2019). Cobalt oxide supported on phosphorus-doped g-C3N4 as an efficient electrocatalyst for oxygen evolution reaction. ACS Appl. Energy Mater. 2, 4718–4729. doi:10.1021/acsaem.9b00273
Keywords: water splitting, composite, carbon nanofibers, cobalt phosphate, HER
Citation: Ahmed I, Biswas R, Sharma R, Burman V and Haldar KK (2023) Access to carbon nanofiber composite hydrated cobalt phosphate nanostructure as an efficient catalyst for the hydrogen evolution reaction. Front. Chem. 11:1129133. doi: 10.3389/fchem.2023.1129133
Received: 21 December 2022; Accepted: 30 January 2023;
Published: 23 February 2023.
Edited by:
Milutin Smiljanic, National Institute of Chemistry, SloveniaReviewed by:
Ivana Perović, University of Belgrade, SerbiaCopyright © 2023 Ahmed, Biswas, Sharma, Burman and Haldar. This is an open-access article distributed under the terms of the Creative Commons Attribution License (CC BY). The use, distribution or reproduction in other forums is permitted, provided the original author(s) and the copyright owner(s) are credited and that the original publication in this journal is cited, in accordance with accepted academic practice. No use, distribution or reproduction is permitted which does not comply with these terms.
*Correspondence: Krishna Kanta Haldar, a3Jpc2hhbmthbnQuaGFsZGFyQGN1cC5lZHUuaW4=
Disclaimer: All claims expressed in this article are solely those of the authors and do not necessarily represent those of their affiliated organizations, or those of the publisher, the editors and the reviewers. Any product that may be evaluated in this article or claim that may be made by its manufacturer is not guaranteed or endorsed by the publisher.
Research integrity at Frontiers
Learn more about the work of our research integrity team to safeguard the quality of each article we publish.