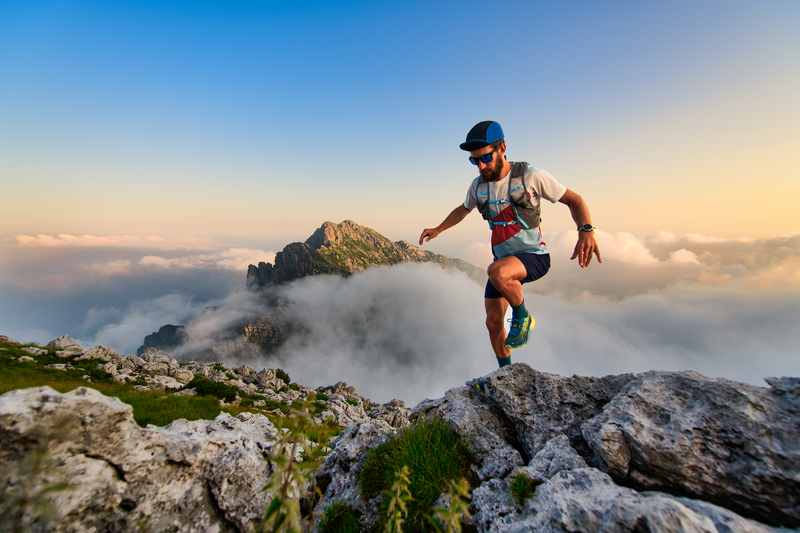
95% of researchers rate our articles as excellent or good
Learn more about the work of our research integrity team to safeguard the quality of each article we publish.
Find out more
MINI REVIEW article
Front. Chem. , 13 January 2023
Sec. Electrochemistry
Volume 11 - 2023 | https://doi.org/10.3389/fchem.2023.1098460
This article is part of the Research Topic Photo/Electrocatalysis for Energy Storage and Conversion View all 9 articles
Cation-disordered rock-salt cathodes (DRX) are promising materials that could deliver high capacities (>250 mAh g−1) with Earth abundant elements and materials. However, their electrochemical performances, other than the capacity, should be improved to be competitive cathodes, and many strategies have been introduced to enhance DRXs. Fluorination has been shown to inhibit oxygen loss and increase power density. Nevertheless, fluorinated cation-disordered rock-salts still suffer from rapid material deterioration and low scalability which limit their practical applications. This mini-review highlights the key challenges for the commercialization of fluorinated cation-disordered rock-salts, discusses the underlying reasons behind material failure and proposes future development directions.
Lithium-ion batteries (LIBs) are presently the go-to energy storage technology for electric vehicles and mobile devices. Performance enhancements over the past two decades, such as increases specific capacity and cycle life, have enabled wider use of applications. Goodenough and Park (2013); Yabuuchi (2018); Jin et al. (2020); Kobayashi et al. (2020); Lee et al. (2020b); Tian et al. (2021) However, the conventional layered intercalation materials are reaching their practical limits (∼250 mAh g−1). Thereby cathode structures with a high Li concentration are being actively sought. One such class of materials is the cation-disordered rock-salts (DRXs).
In LiTMO2 rock-salt type compounds, there are four types of crystal structures according to their cation ordering—DRXs, layered, spinel-like, and γ-LiFeO2. Other than the DRX structure, transition metal (TM) ions have preferred octahedral sites due to the crystal field stabilization energy, and it determines the ordering of the cations (Clément et al., 2018). As the TMO6 octahedra in rock-salts shares their edge, cation disorder inducing octahedral distortion is not energetically favored. On the other hand, an environment with low crystal field stabilization energy such as d0-centered octahedra, octahedral distortion is allowed so that cation disorder could happen (Urban et al., 2017).
Conventionally, the rock-salt-based crystal structure has not been considered as reversible ion hosts because of the absence of required Li-ion migration channels. However, an exception happens when the chemical composition is Li-excess, and the arrangement of cations is disordered (Lee et al., 2014). In this environment, a type of local structure is observed, which is named “0-TM” (Lee et al., 2014). Then, the percolation of Li-ion is enabled by the continuous connection of the 0-TM local structures. The discovery of the Li-ion percolation mechanism has led to significant attention to the DRXs (Yabuuchi et al., 2016; Cai et al., 2021; Ahn et al., 2022; Pei et al., 2022; Zhou et al., 2022). However, many thresholds must be overcome for the commercialization of DRXs, such as low cyclic retention, wide operation voltage, and unsuitable synthetic procedures.
Fluorination for DRXs requires the substitution of lattice oxygen by fluorine, and it is the most representative method to enhance the electrochemical performance of DRXs (Takeda et al., 2017; Campéon and Yabuuchi, 2021). As previously introduced by Ji et al., the intense amount of fluorine introduction significantly changes the physical properties of DRXs, and it leads to electrochemical behavior changes such as redox center rebalancing, redox potential modulation. Ji et al. (2019b), Clément et al. (2020) In general, when F is substituted for metal oxide materials, it is difficult to form a phase due to distortion of the coordination structure, and it limits a substitution concentration of F in oxides (Ménétrier et al., 2008). However, most of DRXs incorporate d0 transition metals (TM) to maintain a cation-disorder state as referred above. Urban et al. (2017) Thereby d0-centered octahedra eases the fluorination barrier so that relatively more significant amounts of fluorine can be substituted (Kitchaev et al., 2018). However, some aspects inherit or even worsen the negative aspects that DRX has for commercialization after fluorination. For example, synthetic difficulties for F substitution, side effects created additionally by F—F-loss, and contribution to the formation of Li-F short-range ordering (Ji et al., 2019a; Ji et al., 2019b; Crafton et al., 2020; Chen et al., 2021a).
Understanding the cause of failure mechanism enables determination of the stable operation limits of DRX whilst limiting material deterioration. For instance, the TM-dependent degradation process of F-DRXs suggests an essential criterion for designing the chemical composition of F-DRXs. Thus, this mini-review summarizes the thresholds for commercializing F-DRXs based on the latest reports and suggests a future research direction. The early part of the review summarizes the degradation process of F-DRXs, inherited from DRXs, and highlights the changes from the fluorination. The pros and cons of fluorination are discussed followed by an assessment of research directions to enable commercialization of F-DRXs.
Most crystalline degradation of DRXs depend on their unstable surface character as shown in Figure 1, which illustrates the overall degradation process of F-DRXs. The deteriorated surface incorporates oxygen loss and TM migration (Lee et al., 2017; Crafton et al., 2020; Zhou et al., 2020; Huang et al., 2021; Schweidler et al., 2022). Although the causality between the oxygen loss and TM migration is not perfectly unveiled, the reduced TMs in the deteriorated surface phase suggest that the two phenomena are closely related. Therefore, it means that the instability of either the chemical stability of the lattice oxygen or the structural stability of the transition metal migration can contribute to the degradation.
FIGURE 1. A schematic diagram illustrating the degradation pathways of fluorinated cation-disordered rock-salts (F-DRXs).
First, the destabilization pathway through the oxidation of lattice oxygen in DRXs could be considered. Li-excess DRXs are known to have oxygen redox at the high state of charge, which means that the lattice oxygen is oxidized during the charging process (Seo et al., 2016; Zhou et al., 2020). The oxidized oxygen species in bulk could be stabilized by a specific coordination structure or chemical composition, and it retains partial reversibility by the reduction of the species (House et al., 2018; Eum et al., 2022). However, unlike bulk lattice oxygen, oxidized surface oxygen produces high reactivity by forming radicals due to the deficiency of coordination number. The oxidized surface oxygen reacts with the electrolyte to form CO2 or O2 gases, which are irreversibly lost (Lee et al., 2017; Crafton et al., 2020; Schweidler et al., 2022). Although the electrolyte decomposition at the cathode-electrolyte interface by the electron transport from highest occupied molecular orbital (HOMO) of electrolyte to cathode is reported in the Li-stoichiometric environment as well, the oxidized oxygen catalyzes the side reaction (Delmas et al., 1997). Also, recently reported oxygen redox in Li-stoichiometric layered oxide cathodes suggests the necessity to revisit the existing electrolyte decomposition mechanism from the perspective of the oxygen redox (Li et al., 2019; Lee et al., 2020a; Hu et al., 2021; Lee et al., 2021).
TM evolution with oxygen loss in DRXs is different from other layered oxide cathodes that are known to show oxygen redox. The degradation of the layered oxide surface proceeds from layered structure (TM:O = 1:2) to spinel structure (TM:O = 3:4) and finally transforms to rock-salt structure (TM:O = 1:1). Jung et al. (2014) The trend indicates the decrease in TM coordination number with oxygen, it has been interpreted that the transformation happens with the loss of oxygen (Boulineau et al., 2013; Shin et al., 2018; Cui et al., 2020). This phenomenon is called “densification” as the concentration of the surface increases. However, the crystalline structure of DRXs has rock-salt symmetry; most DRXs retain rock-salt structure after deterioration. It is noteworthy that the phase transition has transition metal dependence, Kwon et al. (2020).
The site previously occupied by Li+ gets emptied after the charging process, and surface transition metals migrate from the surface fill the site. Although the surface phase maintains the original symmetry, TM migration is a crucial structural degradation mechanism from the perspective of percolation, which is the fundamental Li-ion transport mechanism of DRXs. As also referred to in the introduction, Lee et al. suggested a “0-TM” local structure, an oxygen-centered tetrahedral coordinated structure surrounded by the Li-ion (Lee et al., 2014). The percolation happens through the continuous connection of the 0-TM. The Li-excess composition is necessary to percolate the Li-ion, however, filling the Li site with TM destroys the Li-excess environment at the surface. It blocks the percolation pathway and deters Li-ion transport.
In terms of preventing the loss of oxygen, fluorination has the advantage of minimizing oxygen oxidation by reducing the transition metal in DRXs (Lee et al., 2017; Crafton et al., 2020; Zhou et al., 2020; Huang et al., 2021; Schweidler et al., 2022). While the oxidation state of lattice oxygen is 2-, fluorine’s oxidation state is 1-, so fluorination allows TM to have more d electrons by reduction. In general, the charge compensation for the Li-ion extraction preferentially happens through d orbitals because the d orbitals of TMs are closer to the Fermi level than oxygen (Manthiram, 2020). As a result, TM reduction prevents the deterioration from oxygen oxidation. However, it is noteworthy that the reduced transition metals seem to not reach their original limits of the oxidation state after the charging process. In Ni-Ti-Mo-O-F DRXs compounds, soft X-ray absorption spectroscopy (sXAS) revealed that Ni2+/4+ redox reservoir is incompletely used (Lee et al., 2017). The Ni L3-edge spectra collected by the bulk-sensitive total fluorescence yield (TFY) mode showed that a significant amount of Ni2+ still exists at the fully charged state. Based on the nuclear magnetic resonance (NMR) spectroscopy and calculation results, Clement et al. claimed that Ni directly bonded with F is reduced to 2+, and F-neighboring Li is partially inaccessible due to the strong Li-F bonding, which is detail explained in the later part (Clément et al., 2018; Kitchaev et al., 2018). Nevertheless, the oxidation state of Ni evaluated by sXAS is much lower than other cathodes having Ni4+ and is still remained ambiguous. Hence, it requires a systematic sXAS study to elucidate the effect of fluorination on the TM redox centers, including Ni.
The stabilization of surface oxygen has been observed via diverse characterization. Operando online electrochemical mass spectroscopy (OEMS) shows a significant decrease in gas evolution like O2 or CO2 (Crafton et al., 2020). OEMS results of DRX with oxygen isotope substituted DRXs revealed that the oxygen contained in the gas came from the electrolyte instead of the material, suggesting the effect of fluorination prevented oxygen loss. In terms of spectroscopy, various techniques like resonant inelastic X-ray scattering (RIXS), XAS, X-ray photoelectron spectroscopy (XPS), electron paramagnetic resonance (EPR), and Raman spectroscopy have been used to detect oxidized oxygen species (Yamamoto et al., 2019; Chang et al., 2020; Mozhzhukhina et al., 2020; Naylor et al., 2020; Zhou et al., 2020). RIXS can detect a unique peak for the oxidized lattice oxygen; the intensity comparison of the unique peak can be used to check the reversibility of the oxygen redox (Yang and Devereaux, 2018). The fluorinated group showed higher peak intensity than the control group, and the reversibility at the extended cycles was also better than the control group. Besides, spectroscopies providing surface information like XPS and XAS with electron yield mode suggested that the surface TM reduction of the long-term cycling was enhanced after the proper amount of fluorination. The electrochemical performances correspond to the observations above, supporting that surface stabilization by fluorination prevents material degradation.
The reactive surface of DRXs also causes the TM dissolution issue, and it has been known that DRXs have relatively low surface stability while the surface of other cathode materials is passivated by CEI formation. Moghadam et al. had a report about the effect of fluorination to prevent TM dissolution in DRX with Li-Mn-Ti composition (Shirazi Moghadam et al., 2021; Shirazi Moghadam et al., 2022). Conversely, the V-containing surface layer of Li2VO2F was not passivated, and vanadium was steadily lost from the material by repeating the (de)formation during the cycling (Källquist et al., 2019; Källquist et al., 2020). Therefore it remains undetermined whether or not fluorination has an effect of TM dissolution in DRXs.
From the overall viewpoint, a significant degradation pathway of DRXs is particle cracking. As the cracking exposes the unreacted fresh surface to the electrolyte, it should be carefully understood to prevent surface degradation. Unlike the cracking of layered oxide cathodes, including Li-stoichiometric or Li/Mn-rich oxides, which gradually happens during the long-term cycling, immediate cracking happens in DRXs during their first cycle. Recently, Wang et al. suggested that DRX is a set of randomly oriented O3-packing blocks based on layered-like anisotropy (Wang et al., 2022). Although the study does not include any mechanical insights, the discovery of random anisotropy suggests that DRXs may weaker against the internal stress (Ortiz and Suresh, 1993; Lim et al., 2017). Interestingly, fluorination seems to affect the cracking behavior of the DRXs. Chen et al. reported that the cracking of DRXs is non-directional, fluorination leads to the aligned cracking by increasing the concentration of Li on (001) plane (Chen et al., 2021b). However, it is difficult to rule out the possibility that the improved electrochemical performance is due to other effects of fluorination. Even though the cracking pattern is significantly changed, the exposure of the new surface is resultantly the same. However, considering the reports from other cathodes, the reactivity between the newly formed surface and electrolyte might be different due to the facet preference, and reassembling behavior of pulverized particles could be different. Thus, further understanding of the relationship between directional cracking and its electrochemical performance is required.
Fluorination is used as a method to minimize the failure of DRXs, and it provides electrochemical enhancements. However, the physicochemical property changes along with the substitution by fluorine leads to alternative side effects, which have to be understood and managed. Especially, the high thermodynamic stability of LiF lowers the solubility of F in DRXs, making LiF easily segregated during the fluorination, and it affects the overall degradation process of F-DRXs (Ménétrier et al., 2008; Szymanski et al., 2022).
F-DRXs show different densification behavior from DRXs. The densification of DRX includes the cationic arrangement with oxygen loss. Chen et al. reported that the additional anion sublattice rearrangement was named “anionic densification” for F-DRXs (Chen et al., 2021a). The F K-edge XAS with surface-detective total electron yield (TEY) mode was used for the characterization. The change of spectral shape indicated the change of F-containing species on the surface of F-DRXs, which means that the LiF-like domain is dominant at the surface as the cycle proceeds. Anionic densification in F-DRXs results in additional electrochemical performance degradation by forming an insulating LiF-like surface component.
Fluorine concentration increase at the surface by anionic densification means the total decrease of F concentration of the material. Besides the LiF-like domain formation, F-loss by dissolution has been reported (Crafton et al., 2020). OEMS helps quantify the dissolved fluorine by detecting gaseous trimethylsilyl fluoride. The addition of fluorine scavenger additives like tris(trimethylsilyl) phosphate (TMSPa) makes gaseous trimethylsilyl fluoride by reacting with dissolved F−. Crafton et al. reported the loss of F via dissolution at the high redox potential region based on the OEMS method.
Likewise, fluorine-loss through several pathways makes it difficult to retain the intended concentration of fluorine. The decrease in concentration may remove the benefits of fluorination. The continuous LiF formation, along with the cycle repetition, suggests that the degradation is not passivated at the early cycles. In addition, exposing a new surface from the particle pulverization implies that the degradation from fluorine could be accelerated.
The high energetic stability of LiF raises concerns not only for the surface, but also for the bulk. As demonstrated by Kitchaev et al., the strong Li-F binding limits the number of accessible Li to 0.4–0.8 per F (Kitchaev et al., 2018). In addition, a tetrahedrally coordinated Li-F short-range order is formed during the charging process. Clément et al. (2018) Li-F short-range order partially blocks the percolation pathway by forming a locally isolated Li cluster. On the other hand, interestingly, Ouyang et al. reported that Li-F short-range ordering is linked when the F content is increased above a certain amount, creating a new percolation pathway based on their theoretical calculation (Ouyang et al., 2020). Although it is experimentally validated, however, the demonstration was indirectly conducted by comparison of electrochemical profiles along with the F contents in the sample. Therefore, it is hard to confirm that continuous Li-F short-range order is critical for enhancing Li-ion transport. Therefore, the Li transport property per change of F contents requires a deeper understanding of theoretical and experimental studies.
Fluorination prevents the deterioration of materials by reducing the amount of oxygen loss, as summarized in part 2. However, it does not mean a complete removal of degradation factors, and introducing fluorine in DRXs also brings new degradation factors. Phenomenologically, the degradation that remains after fluorination of DRXs can be summarized as follows: 1) Blocking the Li percolation pathway by a concerted densification mechanism on the surface; 2) Decreased electric conductivity by LiF-like domain formation; 3) Increased CO2 evolution instead of decreased O2 evolution; 4) TM and fluorine loss.
Unsurprisingly, most degradation except Li-F short-range ordering has arisen from their surface. Current commercial cathodes have introduced an interphase, which can prevent the material surface from direct contact with the electrolytes, to overcome the issues from unstable surfaces, and this has been shown to be a successful strategy. There are many ways to form the interphases, including ex-situ coating, in-situ interphase formation using electrolyte additives, surface doping, and chemical treatment as illustrated in Figure 2A (Choi et al., 2019; Huang et al., 2019; Lim et al., 2019; Naylor et al., 2020; Kim et al., 2021). The methods are not limited by their scalability and have an advantage from the perspective of commercialization. However, the existence of F at the surface of F-DRX differs their chemical properties from the other oxide materials. Thus, optimization of precursors and synthetic environments is required for the conformal interphase layer. In terms of process, the use of processes that can stimulate unstable F, such as heat treatment, is limited (Takeda et al., 2017). Thus, processes that can realize newly developed precursors within a lower synthetic temperature are needed.
FIGURE 2. A schematic diagram suggesting the future research direction of fluorinated cation-disordered rock-salts (F-DRXs) to enable commercialization. (A) Methodologies for artificial interphase formation on the surface. (B) Requirements for the scale-up of F-DRXs including F-precursor diversification and designation of large-scale high-energy milling.
The synthetic procedure of F-DRX has been limited due to the low solubility of fluorine in oxides, and the mechanochemical synthesis is the only successful method so far. Although the mechanochemical synthesis is industry-friendly and scaleable, F-DRXs require high-energy ball milling like planetary ball-mill (Yabuuchi, 2022). Unfortunately, the scale of the planetary ball mill is structurally limited to hundreds of grams, which is a threshold for commercialization (Zhang et al., 2021). Therefore, the scalability enhancement of mechanochemical synthesis has the highest priority among the targets for commercialization until the emergence of the alternative synthetic procedure.
There are various issues to be addressed to overcome the synthetic issue of F-DRXs. First, a deeper understanding of the mechanochemical substitution of F through ball-milling (Chen et al., 2015; Cai et al., 2022). High-energy milling synthesis methods with higher production capacity include Simoloyer®, eccentric miller, and extruder, but the working principle, such as mixing and pressing method, is different depending on the design difference as shown in Figure 2B (Amrute et al., 2021; Colacino et al., 2021). Therefore, a correct understanding of the F-substitution enables the correct designation of the milling process. For example if, the instantaneous high pressure generated from the ball’s kinetic energy inside the planetary ball milling is the most important factor for F substitution. Then, the instrumentation used for scale-up must also have a similar pressure-generating mechanism. Next, the diversification of the F precursors is needed. Currently, LiF is the only successful precursor for F-DRXs (Szymanski et al., 2022). Although LiF is an excellent precursor, simultaneously providing Li and F without further impurity, the flexibility of the procedure can be limited. In addition, an additional variable, such as Li contents, happens if the targeting F contents change. If another phase of F precursor is developed, it will enhance the flexibility of the procedure. For example, discovering a gaseous F precursor could enable a gas-solid mechanochemical reaction. Of course, a careful experimental design should be followed, considering the environmental effects of fluorine precursor and safety.
The application of fluorination to DRXs has a high potential due to its high specific capacity. As summarized in this paper, fluorination seems beneficial to suppress the previously unstable surface reactions of DRXs, which may be a major origin of cycle life enhancement. However, there are still many hurdles to overcome in order to improve the material to a level for commercialization. For example, problems caused by the introduction of new elements such as LiF-like domain formation, difficult synthesis process, and surface side reactions that are not perfectly controlled. Therefore, additional research on new processes, the development of precursors, and methods applicable to industrial-scale processes such as surface coating are required. Ultimately, research on improving compatibility is needed when fluorination is applied with other strategies that improve the performance of DRXs itself.
G-HL, conceptualization and writing–original draft. JL, conceptualization and writing–original draft. JS, investigation. LH, funding acquisition, supervision, writing–review and editing. WY, supervision, funding acquisition, and writing–review and editing.
This work is supported by the Assistant Secretary for Energy Efficiency and Renewable Energy, Vehicle Technologies Office of the US Department of Energy (DOE) under contract no. DEAC02-05CH11231, under the Advanced Battery Materials Research (BMR) Program. The Advanced Light Source is supported by the Director, Office of Science, Office of Basic Energy Sciences, of the U.S. Department of Energy under contract no. DE-AC02-05CH11231. JL and LJH thanks to CATMAT project (EP/S003053/1, FIRG016) from the Faraday Institution for the support.
Author JS was employed by Umicore USA.
The remaining authors declare that the research was conducted in the absence of any commercial or financial relationships that could be construed as a potential conflict of interest.
All claims expressed in this article are solely those of the authors and do not necessarily represent those of their affiliated organizations, or those of the publisher, the editors and the reviewers. Any product that may be evaluated in this article, or claim that may be made by its manufacturer, is not guaranteed or endorsed by the publisher.
Ahn, J., Ha, Y., Satish, R., Giovine, R., Li, L., Liu, J., et al. (2022). Exceptional cycling performance enabled by local structural rearrangements in disordered rocksalt cathodes. Adv. Energy Mat. 12 (27), 2200426. doi:10.1002/aenm.202200426
Amrute, A. P., De Bellis, J., Felderhoff, M., and Schuth, F. (2021). Mechanochemical synthesis of catalytic materials. Chemistry 27 (23), 6819–6847. doi:10.1002/chem.202004583
Boulineau, A., Simonin, L., Colin, J. F., Bourbon, C., and Patoux, S. (2013). First evidence of manganese-nickel segregation and densification upon cycling in Li-rich layered oxides for lithium batteries. Nano Lett. 13 (8), 3857–3863. doi:10.1021/nl4019275
Cai, H., Chen, R., Bahri, M., Hawkins, C., Sonni, M., Daniels, L., et al. (2022). Fluorine-rich oxyfluoride spinel Li1.25Ni0.625Mn1.125O3F utilizing redox-active Ni and Mn for high capacity and improved cyclability. ChemRxiv. doi:10.26434/chemrxiv-2022-8f3fk
Cai, Z., Ji, H., Ha, Y., Liu, J., Kwon, D.-H., Zhang, Y., et al. (2021). Realizing continuous cation order-to-disorder tuning in a class of high-energy spinel-type Li-ion cathodes. Matter 4 (12), 3897–3916. doi:10.1016/j.matt.2021.10.013
Campéon, B. D. L., and Yabuuchi, N. (2021). Fundamentals of metal oxide/oxyfluoride electrodes for Li-/Na-ion batteries. Chem. Phys. Rev. 2 (4), 041306. doi:10.1063/5.0052741
Chang, J. H., Baur, C., Ateba Mba, J.-M., Arčon, D., Mali, G., Alwast, D., et al. (2020). Superoxide formation in Li2VO2F cathode material – A combined computational and experimental investigation of anionic redox activity. J. Mat. Chem. A 8 (32), 16551–16559. doi:10.1039/d0ta06119k
Chen, D., Ahn, J., Self, E., Nanda, J., and Chen, G. (2021a). Understanding cation-disordered rocksalt oxyfluoride cathodes. J. Mat. Chem. A 9 (12), 7826–7837. doi:10.1039/d0ta12179g
Chen, D., Zhang, J., Jiang, Z., Wei, C., Burns, J., Li, L., et al. (2021b). Role of fluorine in chemomechanics of cation-disordered rocksalt cathodes. Chem. Mat. 33 (17), 7028–7038. doi:10.1021/acs.chemmater.1c02118
Chen, R., Ren, S., Yavuz, M., Guda, A. A., Shapovalov, V., Witter, R., et al. (2015). Li+ intercalation in isostructural Li2VO3 and Li2VO2F with O2- and mixed O2-/F- anions. Phys. Chem. Chem. Phys. 17 (26), 17288–17295. doi:10.1039/c5cp02505b
Choi, A., Lim, J., Kim, H., Doo, S. W., and Lee, K. T. (2019). In situ electrochemical Zn2+-doping for Mn-rich layered oxides in Li-ion batteries. ACS Appl. Energy Mat. 2 (5), 3427–3434. doi:10.1021/acsaem.9b00241
Clément, R. J., Kitchaev, D., Lee, J., and Gerbrand, C. (2018). Short-range order and unusual modes of nickel redox in a fluorine-substituted disordered rocksalt oxide lithium-ion cathode. Chem. Mat. 30 (19), 6945–6956. doi:10.1021/acs.chemmater.8b03794
Clément, R. J., Lun, Z., and Ceder, G. (2020). Cation-disordered rocksalt transition metal oxides and oxyfluorides for high energy lithium-ion cathodes. Energy & Environ. Sci. 13 (2), 345–373. doi:10.1039/c9ee02803j
Colacino, E., Isoni, V., Crawford, D., and García, F. (2021). Upscaling mechanochemistry: Challenges and opportunities for sustainable industry. Trends Chem. 3 (5), 335–339. doi:10.1016/j.trechm.2021.02.008
Crafton, M. J., Yue, Y., Huang, T. Y., Tong, W., and McCloskey, B. D. (2020). Anion reactivity in cation-disordered rocksalt cathode materials: The influence of fluorine substitution. Adv. Energy Mat. 10 (35), 2001500–2001511. doi:10.1002/aenm.202001500
Cui, C., Fan, X., Zhou, X., Chen, J., Wang, Q., Ma, L., et al. (2020). Structure and interface design enable stable Li-rich cathode. J. Am. Chem. Soc. 142 (19), 8918–8927. doi:10.1021/jacs.0c02302
Delmas, C., Pérès, J. P., Rougier, A., Demourgues, A., Weill, F., Chadwick, A., et al. (1997). On the behavior of the LixNiO2 system: An electrochemical and structural overview. J. Power Sources 68 (1), 120–125. doi:10.1016/S0378-7753(97)02664-5
Eum, D., Kim, B., Song, J. H., Park, H., Jang, H. Y., Kim, S. J., et al. (2022). Coupling structural evolution and oxygen-redox electrochemistry in layered transition metal oxides. Nat. Mat. 21 (6), 664–672. doi:10.1038/s41563-022-01209-1
Goodenough, J. B., and Park, K. S. (2013). The Li-ion rechargeable battery: A perspective. J. Am. Chem. Soc. 135 (4), 1167–1176. doi:10.1021/ja3091438
House, R. A., Jin, L., Maitra, U., Tsuruta, K., Somerville, J. W., Förstermann, D. P., et al. (2018). Lithium manganese oxyfluoride as a new cathode material exhibiting oxygen redox. Energy & Environ. Sci. 11 (4), 926–932. doi:10.1039/c7ee03195e
Hu, E., Li, Q., Wang, X., Meng, F., Liu, J., Zhang, J.-N., et al. (2021). Oxygen-redox reactions in LiCoO2 cathode without O–O bonding during charge-discharge. Joule 5 (3), 720–736. doi:10.1016/j.joule.2021.01.006
Huang, B., Wang, R., Gong, Y., He, B., and Wang, H. (2019). Enhanced cycling stability of cation disordered rock-salt Li1.2Ti0.4Mn0.4O2 material by surface modification with Al2O3. Front. Chem. 7, 107. doi:10.3389/fchem.2019.00107
Huang, T.-Y., Crafton, M. J., Yue, Y., Tong, W., and McCloskey, B. D. (2021). Deconvolution of intermixed redox processes in Ni-based cation-disordered Li-excess cathodes. Energy & Environ. Sci. 14 (3), 1553–1562. doi:10.1039/d0ee03526b
Ji, H., Kitchaev, D. A., Lun, Z., Kim, H., Foley, E., Kwon, D.-H., et al. (2019a). Computational investigation and experimental realization of disordered high-capacity Li-ion cathodes based on Ni redox. Chem. Mat. 31 (7), 2431–2442. doi:10.1021/acs.chemmater.8b05096
Ji, H., Urban, A., Kitchaev, D. A., Kwon, D. H., Artrith, N., Ophus, C., et al. (2019b). Hidden structural and chemical order controls lithium transport in cation-disordered oxides for rechargeable batteries. Nat. Commun. 10 (1), 592. doi:10.1038/s41467-019-08490-w
Jin, D., Park, J., Ryou, M. H., and Lee, Y. M. (2020). Structure-controlled Li metal electrodes for post-Li-ion batteries: Recent progress and perspectives. Adv. Mat. Interfaces 7 (8), 1902113. doi:10.1002/admi.201902113
Jung, S.-K., Gwon, H., Hong, J., Park, K.-Y., Seo, D.-H., Kim, H., et al. (2014). Understanding the degradation mechanisms of LiNi0.5Co0.2Mn0.3O2 cathode material in lithium ion batteries. Adv. Energy Mat. 4 (1), 1300787. doi:10.1002/aenm.201300787
Källquist, I., Martin, J.-F., Naylor, A. J., Baur, C., Fichtner, M., Colin, J.-F., et al. (2020). Influence of electrolyte additives on the degradation of Li2VO2F Li-rich cathodes. J. Phys. Chem. C 124 (24), 12956–12967. doi:10.1021/acs.jpcc.0c02840
Källquist, I., Naylor, A. J., Baur, C., Chable, J., Kullgren, J., Fichtner, M., et al. (2019). Degradation mechanisms in Li2VO2F Li-rich disordered rock-salt cathodes. Chem. Mat. 31 (16), 6084–6096. doi:10.1021/acs.chemmater.9b00829
Kim, Y., Park, H., Dolocan, A., Warner, J. H., and Manthiram, A. (2021). Wet-CO2 pretreatment process for reducing residual lithium in high-nickel layered oxides for lithium-ion batteries. ACS Appl. Mat. Interfaces 13 (23), 27096–27105. doi:10.1021/acsami.1c06277
Kitchaev, D. A., Lun, Z., Richards, W. D., Ji, H., Clément, R. J., Balasubramanian, M., et al. (2018). Design principles for high transition metal capacity in disordered rocksalt Li-ion cathodes. Energy & Environ. Sci. 11 (8), 2159–2171. doi:10.1039/c8ee00816g
Kobayashi, T., Zhao, W., Rajendra, H. B., Yamanaka, K., Ohta, T., and Yabuuchi, N. (2020). Nanosize cation-disordered rocksalt oxides: Na2TiO3-NaMnO2 binary system. Small 16 (12), e1902462. doi:10.1002/smll.201902462
Kwon, D.-H., Lee, J., Artrith, N., Kim, H., Wu, L., Lun, Z., et al. (2020). The impact of surface structure transformations on the performance of Li-excess cation-disordered rocksalt cathodes. Cell Rep. Phys. Sci. 1 (9), 100187–100209. doi:10.1016/j.xcrp.2020.100187
Lee, G. H., Lau, V. W. h., Yang, W., and Kang, Y. M. (2021). Utilizing oxygen redox in layered cathode materials from multiscale perspective. Adv. Energy Mat. 11 (27), 2003227. doi:10.1002/aenm.202003227
Lee, G. H., Muhammad, S., Sergey, C., Lee, H., Yoon, J., Kang, Y. M., et al. (2020b). Advances in the cathode materials for lithium rechargeable batteries. Angew. Chem. Int. Ed. 59 (7), 2578–2605. doi:10.1002/anie.201902359
Lee, G. H., Wu, J., Kim, D., Cho, K., Cho, M., Yang, W., et al. (2020a). Reversible anionic redox activities in conventional LiNi1/3 Co1/3 Mn1/3 O2 cathodes. Angew. Chem. Int. Ed. 59 (22), 8681–8688. doi:10.1002/anie.202001349
Lee, J., Papp, J. K., Clement, R. J., Sallis, S., Kwon, D. H., Shi, T., et al. (2017). Mitigating oxygen loss to improve the cycling performance of high capacity cation-disordered cathode materials. Nat. Commun. 8 (1), 981–990. doi:10.1038/s41467-017-01115-0
Lee, J., Urban, A., Li, X., Su, D., Hautier, G., and Ceder, G. (2014). Unlocking the potential of cation-disordered oxides for rechargeable lithium batteries. Science 343 (6170), 519–522. doi:10.1126/science.1246432
Li, N., Sallis, S., Papp, J. K., Wei, J., McCloskey, B. D., Yang, W., et al. (2019). Unraveling the cationic and anionic redox reactions in a conventional layered oxide cathode. ACS Energy Lett. 4 (12), 2836–2842. doi:10.1021/acsenergylett.9b02147
Lim, J., Choi, A., Kim, H., Doo, S. W., Park, Y., and Lee, K. T. (2019). In situ electrochemical surface modification for high-voltage LiCoO2 in lithium ion batteries. J. Power Sources 426, 162–168. doi:10.1016/j.jpowsour.2019.04.011
Lim, J. M., Hwang, T., Kim, D., Park, M. S., Cho, K., and Cho, M. (2017). Intrinsic origins of crack generation in Ni-rich LiNi0.8Co0.1Mn0.1O2 layered oxide cathode. Mater. Sci. Rep. 7, 39669. doi:10.1038/srep39669
Manthiram, A. (2020). A reflection on lithium-ion battery cathode chemistry. Nat. Commun. 11 (1), 1550. doi:10.1038/s41467-020-15355-0
Ménétrier, M., Bains, J., Croguennec, L., Flambard, A., Bekaert, E., Jordy, C., et al. (2008). NMR evidence of LiF coating rather than fluorine substitution in Li(Ni0.425Mn0.425Co0.15)O2. J. Solid State Chem. 181 (12), 3303–3307. doi:10.1016/j.jssc.2008.09.002
Mozhzhukhina, N., Kullgren, J., Baur, C., Gustafsson, O., Brant, W. R., Fichtner, M., et al. (2020). Short-range ordering in the Li-rich disordered rock salt cathode material Li2VO2F revealed by Raman spectroscopy. J. Raman Spectrosc. 51 (10), 2095–2101. doi:10.1002/jrs.5953
Naylor, A. J., Källquist, I., Peralta, D., Martin, J.-F., Boulineau, A., Colin, J.-F., et al. (2020). Stabilization of Li-rich disordered rocksalt oxyfluoride cathodes by particle surface modification. ACS Appl. Energy Mat. 3 (6), 5937–5948. doi:10.1021/acsaem.0c00839
Ortiz, M., and Suresh, S. (1993). Statistical properties of residual stresses and intergranular fracture in ceramic materials. J. Appl. Mech. 60 (1), 77–84. doi:10.1115/1.2900782
Ouyang, B., Artrith, N., Lun, Z., Jadidi, Z., Kitchaev, D. A., Ji, H., et al. (2020). Effect of fluorination on lithium transport and short-range order in disordered-rocksalt-type lithium-ion battery cathodes. Adv. Energy Mat. 10 (10), 1903240. doi:10.1002/aenm.201903240
Pei, Y., Chen, Q., Ha, Y., Su, D., Zhou, H., Li, S., et al. (2022). Fluorinated rocksalt cathode with ultra-high active Li content for lithium-ion batteries. Angew. Chem. Int. Ed. 61, e202212471. doi:10.1002/anie.202212471
Schweidler, S., Dreyer, S. L., Breitung, B., and Brezesinski, T. (2022). Acoustic emission monitoring of high-entropy oxyfluoride rock-salt cathodes during battery operation. Coatings 12 (3), 402. doi:10.3390/coatings12030402
Seo, D. H., Lee, J., Urban, A., Malik, R., Kang, S., and Ceder, G. (2016). The structural and chemical origin of the oxygen redox activity in layered and cation-disordered Li-excess cathode materials. Nat. Chem. 8 (7), 692–697. doi:10.1038/nchem.2524
Shin, Y., Kan, W. H., Aykol, M., Papp, J. K., McCloskey, B. D., Chen, G., et al. (2018). Alleviating oxygen evolution from Li-excess oxide materials through theory-guided surface protection. Nat. Commun. 9 (1), 4597. doi:10.1038/s41467-018-07080-6
Shirazi Moghadam, Y., Dinda, S., El Kharbachi, A., Melinte, G., Kübel, C., and Fichtner, M. (2022). Structural and electrochemical insights from the fluorination of disordered Mn-based rock salt cathode materials. Chem. Mat. 34 (5), 2268–2281. doi:10.1021/acs.chemmater.1c04059
Shirazi Moghadam, Y., El Kharbachi, A., Diemant, T., Melinte, G., Hu, Y., and Fichtner, M. (2021). Toward better stability and reversibility of the Mn4+/Mn2+ double redox activity in disordered rocksalt oxyfluoride cathode materials. Chem. Mat. 33 (21), 8235–8247. doi:10.1021/acs.chemmater.1c02334
Szymanski, N. J., Zeng, Y., Bennett, T., Patil, S., Keum, J. K., Self, E. C., et al. (2022). Understanding the fluorination of disordered rocksalt cathodes through rational exploration of synthesis pathways. Chem. Mat. 34, 7015–7028. doi:10.1021/acs.chemmater.2c01474
Takeda, N., Hoshino, S., Xie, L., Chen, S., Ikeuchi, I., Natsui, R., et al. (2017). Reversible Li storage for nanosize cation/anion-disordered rocksalt-type oxyfluorides: LiMoO2–xLiF (0 ≤ x ≤ 2) binary system. J. Power Sources 367, 122–129. doi:10.1016/j.jpowsour.2017.09.060
Tian, Y., Zeng, G., Rutt, A., Shi, T., Kim, H., Wang, J., et al. (2021). Promises and challenges of next-generation "beyond Li-ion" batteries for electric vehicles and grid decarbonization. Chem. Rev. 121 (3), 1623–1669. doi:10.1021/acs.chemrev.0c00767
Urban, A., Abdellahi, A., Dacek, S., Artrith, N., and Ceder, G. (2017). Electronic-structure origin of cation disorder in transition-metal oxides. Phys. Rev. Lett. 119 (17), 176402. doi:10.1103/PhysRevLett.119.176402
Wang, Y., Huang, S., Raji-Adefila, B., Outka, A., Wang, J. H., and Chen, D. (2022). Unraveling the nature and role of layered cation ordering in cation-disordered rock-salt cathodes. J. Am. Chem. Soc. 144 (43), 19838–19848. doi:10.1021/jacs.2c07473
Yabuuchi, N. (2018). Material design concept of lithium-excess electrode materials with rocksalt-related structures for rechargeable non-aqueous batteries. Chem. Rec. 19, 690–707. doi:10.1002/tcr.201800089
Yabuuchi, N., Nakayama, M., Takeuchi, M., Komaba, S., Hashimoto, Y., Mukai, T., et al. (2016). Origin of stabilization and destabilization in solid-state redox reaction of oxide ions for lithium-ion batteries. Nat. Commun. 7, 13814. doi:10.1038/ncomms13814
Yabuuchi, N. (2022). Rational material design of Li-excess metal oxides with disordered rock salt structure. Curr. Opin. Electrochem. 34, 100978. doi:10.1016/j.coelec.2022.100978
Yamamoto, K., Zhou, Y., Yabuuchi, N., Nakanishi, K., Yoshinari, T., Kobayashi, T., et al. (2019). Charge compensation mechanism of lithium-excess metal oxides with different covalent and ionic characters revealed by operando soft and hard X-ray absorption spectroscopy. Chem. Mat. 32 (1), 139–147. doi:10.1021/acs.chemmater.9b02838
Yang, W., and Devereaux, T. P. (2018). Anionic and cationic redox and interfaces in batteries: Advances from soft X-ray absorption spectroscopy to resonant inelastic scattering. J. Power Sources 389, 188–197. doi:10.1016/j.jpowsour.2018.04.018
Zhang, Y., Self, E. C., Thapaliya, B. P., Giovine, R., Meyer, H. M., Li, L., et al. (2021). formation of LiF surface layer during direct fluorination of high-capacity Co-free disordered rocksalt cathodes. ACS Appl. Mat. Interfaces 13 (32), 38221–38228. doi:10.1021/acsami.1c07882
Zhou, K., Li, Y., Ha, Y., Zhang, M., Dachraoui, W., Liu, H., et al. (2022). A nearly zero-strain Li-rich rock-salt oxide with multielectron redox reactions as a cathode for Li-ion batteries. Chem. Mat. 34 (21), 9711–9721. doi:10.1021/acs.chemmater.2c02519
Keywords: Li-ion batteries, cathode, cation-disordered rock-salt, commercialization, degradation pathway, surface coating
Citation: Lee G-H, Lim J, Shin J, Hardwick LJ and Yang W (2023) Towards commercialization of fluorinated cation-disordered rock-salt Li-ion cathodes. Front. Chem. 11:1098460. doi: 10.3389/fchem.2023.1098460
Received: 15 November 2022; Accepted: 02 January 2023;
Published: 13 January 2023.
Edited by:
Yujing Bi, Pacific Northwest National Laboratory (DOE), United StatesReviewed by:
Jiangtao Hu, Shenzhen University, ChinaCopyright © 2023 Lee, Lim, Shin, Hardwick and Yang. This is an open-access article distributed under the terms of the Creative Commons Attribution License (CC BY). The use, distribution or reproduction in other forums is permitted, provided the original author(s) and the copyright owner(s) are credited and that the original publication in this journal is cited, in accordance with accepted academic practice. No use, distribution or reproduction is permitted which does not comply with these terms.
*Correspondence: Gi-Hyeok Lee, R0hMZWVAbGJsLmdvdg==; Wanli Yang, d2x5YW5nQGxibC5nb3Y=
†These authors have contributed equally to this work and share first authorship
Disclaimer: All claims expressed in this article are solely those of the authors and do not necessarily represent those of their affiliated organizations, or those of the publisher, the editors and the reviewers. Any product that may be evaluated in this article or claim that may be made by its manufacturer is not guaranteed or endorsed by the publisher.
Research integrity at Frontiers
Learn more about the work of our research integrity team to safeguard the quality of each article we publish.