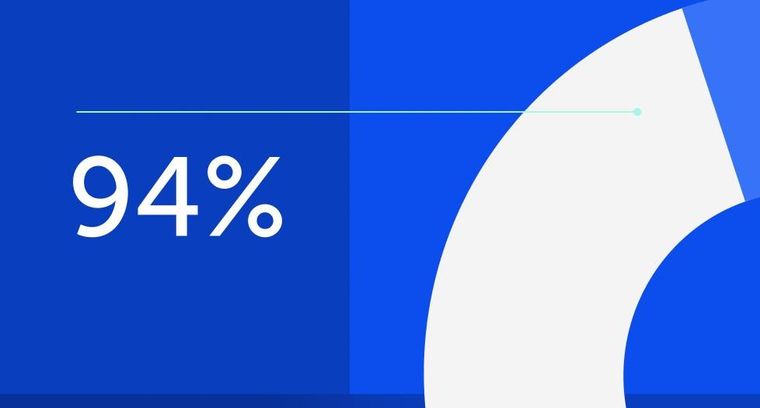
94% of researchers rate our articles as excellent or good
Learn more about the work of our research integrity team to safeguard the quality of each article we publish.
Find out more
REVIEW article
Front. Chem., 14 September 2022
Sec. Organic Chemistry
Volume 10 - 2022 | https://doi.org/10.3389/fchem.2022.991026
This article is part of the Research TopicSix-Membered Heterocycles: Their Synthesis and Bio ApplicationsView all 9 articles
Quinazolinone, a bicyclic compound, comprises a pyrimidine ring fused at 4´ and 8´ positions with a benzene ring and constitutes a substantial class of nitrogen-containing heterocyclic compounds on account of their frequent existence in the key fragments of many natural alkaloids and pharmaceutically active components. Consequently, tremendous efforts have been subjected to the elegant construction of these compounds and have recently received immense interest in synthetic and medicinal chemistry. The domain of synthetic organic chemistry has grown significantly over the past few decades for the construction of highly functionalized therapeutically potential complex molecular structures with the aid of small organic molecules by replacing transition-metal catalysis. The rapid access to this heterocycle by means of organocatalytic strategy has provided new alternatives from the viewpoint of synthetic and green chemistry. In this review article, we have demonstrated a clear presentation of the recent organocatalytic synthesis of quinazolinones of potential therapeutic interests and covered the literature from 2015 to date. In addition to these, a clear presentation and understanding of the mechanistic aspects, features, and limitations of the developed reaction methodologies have been highlighted.
Heterocyclic compounds are among the most versatile organic compounds in natural and biologically active synthetic materials, pharmaceuticals, and synthetic intermediates (Bur and Padwa, 2004; Dwivedi et al., 2020; Borah et al., 2021a; Borah and Chowhan, 2021; Borah and Chowhan, 2022a; Kumar B. et al., 2022). The basic unit of heterocycles comprises a ringed structure with a heteroatom like nitrogen, oxygen, or sulfur, other than carbon, and the properties of the compound depend significantly on the number and types of heteroatoms present in the ring. Owing to their immense importance in a variety of disciplines of medicinal chemistry, drug design, and functional materials, the construction of heterocyclic compounds, especially those containing nitrogen and oxygen, has received enormous interest in organic synthesis (Majumdar et al., 2014; Patrusheva et al., 2018; Chugh et al., 2020; Anantha et al., 2021; Mermer et al., 2021; Xing et al., 2021; Borah et al., 2022a). Among various nitrogen-containing heterocycles, quinazolinone, a bicyclic compound with a pyrimidine system fused at 4´ and 8´ positions with a benzene ring, has recently received a great deal of attention in organic and medicinal chemistry (Auti et al., 2020; Khan et al., 2014; Kshirsagar, 2015; Dherbassy et al., 2021; Hao et al., 2021). Quinazolinone and its derivatives are an essential heterocyclic skeleton that serves a prominent role in several cellular processes and have been established for their remarkable therapeutic significance as antihypertensive, antimicrobial, antihyperlipidemic, anti-inflammatory, and anticonvulsant activities (Mhaske and Argade, 2006; Khan et al., 2016). At the same time, some of their derivatives are also used as antitumor chemotherapeutic agents (Hekal and Abu El-Azm, 2018; Hakim et al., 2022). For instance, some representative examples of therapeutically potential quinazolinone derivatives are described in Figure 1. The natural alkaloid rutaecarpine isolated from the unripe fruit of Evodia rutaecarpa was found to possess anti-inflammatory properties as it inhibits cyclooxygenase 2 (Chen and Chen, 1933; Bergman and Bergman, 1985; Moon et al., 1999). Quinazolinone containing another natural alkaloid, luotonin A, has been derived from Peganum nigellastrum, a Chinese herbal medicinal plant, and demonstrated to exhibit cytotoxic effects against murine leukemia P388 cell line (Ma et al., 1997) and recognized as antiviral and antiphytopathogenic fungus agents (Hao et al., 2020). Raltitrexed (Gunasekara and Faulds, 1998) from the quinazolinone family under the brand name Tomudex®) is constructed as a particular thymidylate synthase inhibitor and used as a chemotherapeutic agent against colorectal cancer (Köhne et al., 1998). Bouchardatine, a β-indoloquinazoline alkaloid isolated from the aerial part of Bouchardatia neurococca, has been recognized as an adipogenesis inhibitor (Rao et al., 2015). The quinazolinone drug idelalisib, commercially available as Zydelig, was used for the treatment of chronic lymphocytic leukemia (Shanafelt et al., 2015; Zirlik and Veelken, 2018). Norquinadoline A isolated from Cladosporium sp. PJX-41, a mangrove-based fungus, was known to have antiviral activity (Peng et al., 2013). In addition to these, a diverse range of natural and non-natural quinazolinone products displayed remarkable biological activities such as anticonvulsant, antitussive, antidiabetic, diuretic, hypnotic, sedative, analgesic, and many more (Gupta et al., 2018).
Recognizing such promising features, elegant synthetic routes for the construction of these heterocycles, starting from the traditional method to the green catalytic one, have been developed over the past decades (Scheme 1). Although the developed methodologies were well established, sometimes they suffer several serious drawbacks such as the utilization of hazardous reagents, transition-metal catalysts, toxic volatile solvents, high temperatures, harsh reaction conditions, long reaction times, and excessive energy inputs that have a negative impact on both the environment and the overall chemical process, making it difficult to create eco-friendly and sustainable nature. Consequently, significant efforts have been dedicated in the last decades to overcoming these limitations by improving the reaction conditions for the synthesis of these heterocycles that are of significant interest to the scientific community.
Over the last decades, the field of organic synthesis has been mainly dominated by metal catalysis (Nakamura and Yamamoto, 2004; D'Souza and Mueller, 2007; Shang and Liu, 2013) and biocatalysis (Sugai et al., 1997; Reetz, 2013). Despite being powerful catalytic systems that have already been successfully employed for the assembly of quinazolinones and have huge applications in numerous organic syntheses to build valuable structural frameworks such as drug molecules and natural product analogs, transition metal catalysts have many limitations and drawbacks from the perspectives of synthetic efficiency and green chemistry (Chatterjee et al., 2011; Zhou 2016). Intriguingly, it is highly desired to develop a chemical process that uses alternative materials for synthetic purposes that are not only environmentally friendly but also easily available in bulk quantities anywhere at a very low price for the synthesis of complex molecules of potential therapeutic significance with high atom- and step-economies by reducing or avoiding the utilization of transition metal catalysts, co-catalyst, or any additives (Bhunia et al., 2012; Sun and Shi, 2014; Poudel et al., 2018; Khan et al., 2020; Borah and Chowhan, 2022b).
In this regard, the use of small organic molecules called organocatalysts has received substantial attention in organic transformation due to their remarkable properties (List, 2007; Bertelsen and Jørgensen, 2009; Borah et al., 2021b). Not only metal catalysts but also organocatalysts are better replacements for the highly substrate-specific and extremely sensitive biocatalyst. The existence of organocatalyst has led to a revolution in the synthesis of molecular diversity and complexity in an asymmetric and non-asymmetric manner via several activation modes and has turned into one of the most important hot topics of current research in terms of synthetic efficiency and from the green chemistry point of view (Dalko and Moisan, 2001; Barbas, 2008; MacMillan, 2008). The distinctive ability to accomplish chemical transformation through different activation modes, avoidance of expensive catalysts and metal catalyst(s), high stability, ready availability, easy recoverability, lower activation energy, high efficiency, and an immediate reduction in the toxicity and reaction costs offers organocatalytic synthetic approaches as efficient routes for the synthesis of a diverse range of quinazolinone scaffolds from the green and sustainable chemistry viewpoints (Renzi and Bella, 2012; Ren and Wang, 2013; Borah et al., 2021d).
Several review articles focus on the synthesis of quinazolinones from non-conventional to conventional processes (Khan et al., 2015; Khan et al., 2014; Rohokale and Kshirsagar, 2016; Faisal and Saeed, 2021). He et al. reviewed the synthesis of quinazolinone derivatives based on different types of reactions used (He et al., 2014) Abbas et al., and demonstrated the utilization of isatoic anhydride in the synthesis of quinazolinones (Abbas et al., 2016). Maiden and Harrity disclosed the metal-catalyzed synthetic approaches to quinazolinones (Maiden and Harrity, 2016). Heravi and Mohammadkhani reviewed the microwave-assisted synthesis of quinazoline and quinazolinones (Mohammadkhani and Heravi, 2020). Kumar et al. summarize the synthesis of quinazolinones by using different nanoparticles as catalytic systems (Kumar P. et al., 2022). Here, we have demonstrated a recent outlook on the synthesis of quinazolinones by using various organocatalytic systems and cover the report from 2015 to 2022. The mechanistic rationalizations, remarkable advantages, limitations, and scopes of future exploration have also been discussed. The review has been organized based on the types of substrates and catalysts employed.
In 2015, Yang et al. devised a highly convenient metal-free approach for the assembly of 2-aryl (alkyl)-quinazolin-4(3H)-ones 3 via selective breaking of the C-C triple bond of ketoalkynes 2. Under oxidant-free conditions, the trifluoroacetic acid (TFA)-promoted reactions of various anthranilamides 1 and ketoalkynes 2 furnished the representative products in 45–98% yields (Scheme 2) (Yang et al., 2015). The reaction was applicable to aryl- and alkyl-substituted ketoalkynes, and the implication of steric and electronic effects of various substituents on the reaction is minimal. Despite having these successful attempts, they further investigated the reaction with various anthranilamides possessing electron-deficient and electron-rich groups, and in all the cases, the products were found to be formed in quantitative yields. The overall process for this transformation starts with the TFA-mediated Michael addition of 1 and 2 to form the enaminone intermediate Int-1. The subsequent intramolecular cyclization of Int-1 afforded Int-3, which is followed by cleavage of the C-C triple bond to generate the final products 3.
The selective C-C bond cleavage strategy under metal-free conditions was also developed by Li et al. at the same time. With the aid of phosphorous acid (H3PO3), the representative quinazolinone products 6 derived from the cyclo-condensation of 2-aminobenzamides 4 and β-ketoesters 5 have been accomplished in 86–95% yields. Introducing various aprotic solvents such as ethanol (EtOH) and methanol (MeOH) offered an excellent yield of products as compared to an aprotic solvent such as dimethylformamide (DMF), toluene, acetonitrile (CH3CN), and dioxane that provided products with lower yields. Furthermore, the yield of the product was increased with reduced reaction time by increasing the temperature from 25 to 50 °C (Scheme 3) (Li et al., 2015). Regardless of the steric hindrance and electronic effect of the substituents, this acid-catalyzed cyclo-condensation could be employed in a variety of β-ketoesters to yield the corresponding quinazolinones in excellent yields. To broaden the scope of the present protocol, the reaction with β-diketones 7 was also explored. Despite demonstrating a broad range of aliphatic diketones, the steric effect plays a crucial role, and bulky substitutions such as aryl-substituted β-diketones were inactive in cyclo-condensation reactions, which marks the limitations of the present approach.
SCHEME 3. H3PO3 catalyzed rapid synthesis of quinazolinones via selective C-C bond cleavage strategy.
Inspired by the elegant advantages of the previous work, Shen et al. devised a green and straightforward technique for the selective C-C bond cleavage of acyclic or cyclic 1,3-diketones 7 or 9 to access a wide variety of 4(3H)-quinazolinones (Scheme 4A) (Shen et al., 2015). By using 10 mol% of camphorsulphonic acid (CSA) as a Brønsted acid catalyst in an aqueous solution of biodegradable ethyl lactate, the corresponding products 8 and 10 derived from the reaction of 2-aminobenzamides 4 and diketones 7 or 9 have been achieved in <1–98% and 35–98% yield, respectively. The execution of the reaction with various substitutions on 4 or 7 or 9 was found to have no greater influence on the yield of the products. However, the sterically hindered bulky substituents such as the tert-Butyl group present on 1,3-diketone 7 significantly decreased the yield of the product (<1%), whereas smaller groups such as methyl yielded 98% of the product. The mechanism for the formation of 10a begins with the generation of Int-4 from the condensation of 4 and 9a, which was further tautomerized to Int-5. Subsequently, the intramolecular cyclization of Int-5 and final C-C bond cleavage occurred to form the product 10a.
SCHEME 4. CSA, p-SAC, and p-TSA catalyzed one-pot synthesis of quinazolinones 8 & 10 (A), dihydro‐quinazolinones 12 (B), and spiro-quinazolinones 14 (C), respectively.
Rahman et al. demonstrated the catalytic activity of p-sulfonic acid calix [4] arene (p-SAC) as an organocatalyst in the efficient construction of 2,3-dihydroquinazolin-4(1H)-ones 12 from the direct cyclo-condensation of anthranilamide 4a with aldehydes 11 (Scheme 4B) (Rahman et al., 2015). With the aid of only 1 mol% of the catalyst, along with water as the green solvent, the representative products 12 were accomplished in moderate to excellent yields at room temperature. The synthetic potentiality of the current strategy was established by recovering and reusing the catalyst for up to five successive sessions with a minimal change in catalytic property. The reaction conditions tolerate an extensive range of aryl-, heteroaryl-, and alkyl-substituted aldehydes. Moreover, the reaction is resistant to steric and electronic effects and provided significant yield regardless of aromatic, electron-withdrawing, or donating group. However, the reaction with acetone or propyl aldehydes required a longer reaction time to complete as compared to other aldehydes. In a similar manner, a very efficient p-toluene sulfonic acid (p-TSA) catalyzed one-pot approach for the assembly of spiro quinazolinones from the reaction of anthranilamide 4a with ketones 13 was developed by Revathy and Lalitha (Scheme 4C) (Revathy and Lalitha, 2015). The reaction worked well with a wide range of ketones, and the representative products 14 were obtained in good to excellent yields within a short reaction duration. With various diketones as the substrate, the reactions with anthranilamide 4a were completed in 1 h, whereas the same reaction with cyclic ketones was completed within 10 min. Furthermore, the utilization of 1,4-cyclohexanedione in a 1:1 ratio resulted in the formation of single spiro compounds on one side of the ketone; while using a 2:1 ratio, the dispiro compounds were formed. The reaction can proceed through the formation of imine intermediate Int-10 via the addition of p-TSA activated Int-7 with 4a. The final cyclization of the -NH2 group on the double bond of imine of Int-10 followed by the H+ shift afforded the product 14.
In 2016, Liu et al. disclosed a facile and straightforward approach for the elegant construction of 2-hetarylquinazolin-4(3H)-ones via metal-free oxidative amination of Csp3–H bonds. With the aid of diphenylphosphinic acid (Ph2PO2H) as the catalyst, tetra-n-Butyl ammonium iodide (TBAI) as the iodine source, and tert-butyl hydroperoxide (TBHP) as the oxidant, the representative products 16 derived from 2-aminobenzamides 4 with diverse electron-withdrawing or electron-donating groups and (2-aza-aryl)methanes 15 have been obtained in good to excellent yields (Scheme 5) (Liu et al., 2016). While substitutions on the R2 position of 4 by groups like methyl smoothly reacted to afford the desired products, the presence of more hindered bulkier groups in the R2 position decreased the yield of the products. On the other hand, diverse (2-aza-aryl) methanes such as 2-methylquinolines, 1-methylisoquinoline, 3-methylbenzo [f]quinolone, 2-methylquinoxaline, and 2-methylbenzo [d]thiazole worked well for this reaction. However, 2-methylnaphthalene and pyridine derivatives failed to yield any products under the standard reaction conditions, which marks the limitations of this chemistry and calls for further developments.
A very simple and practical one-pot approach for the rapid access to enantiomerically pure aryl-quinazolinones catalyzed by chiral phosphoric acids was discovered by Wang et al. (Scheme 6) (Wang et al., 2017). With the aid of 10 mol% of C-1 as the chiral catalyst and 2,3-dichloro-5,6-dicyano-1,4-benzoquinone (DDQ) as the oxidant, the treatment of 2-aminobenzamides 17 and aldehydes 18 was found to proceed at 0°C to furnish the axially chiral aryl quinazolinones 19 in moderate to excellent yields with good to excellent enantioselectivities. In addition to demonstrating the diverse substitutions on different positions of the N-aryl ring of 2-aminobenzamides 17, a broad range of aryl and heteroaryl-substituted aldehydes possessing electron-deficient or electron-donating groups were established to be very efficient for this reaction. Furthermore, they also disclosed the N-triflylphosphoramides (C-2) catalyzed selective C-C bond cleavage strategy for the atroposelective construction of alkyl-substituted aryl quinazolinones 22. Pleasingly, the reaction of t-Bu substituted 2-aminobenzamides 20 and 4-methoxypentenone 21 afforded the representative products 22 in 75–95% yields with good enantioselectivity. The synthetic efficiency of the protocol was further established by transforming the synthesized products into more complex products 23 and 24 without affecting the enantioselectivity.
SCHEME 6. Enantioselective construction of axially chiral quinazolinones by chiral phosphoric acid catalysis.
Another achievement for the synthesis of different types of quinazolinones 25 and 27 has been accomplished by Yashwantrao et al. by employing mechanical activation as an environmentally benign approach (Scheme 7) (Yashwantrao et al., 2019). With the aid of 10 mol% of p-toluene sulfonic acid (p-TSA) as the Brønsted acid catalyst, the solvent-free reaction of anthranilamide 1 and aldehydes 18 under mechanochemical grinding conditions delivers the corresponding quinazolinone products 25 in moderate to excellent yield within 3–15 min. While the reaction of anthranilamide 1 with different carbonyl compounds 26 under the optimal condition delivers the quinazolinones of type 27 in moderate to excellent yield in only 5 min. Wide-range of functional group tolerances, mild reaction conditions, solvent-free, waste-free, and metal-free are some of the key advantages of this protocol. The practicality of the protocol was established by performing Gram scale synthesis in quantitative yield.
Jia et al. disclosed an acid-catalyzed oxidative cyclization strategy for the rapid construction of diverse tetracyclic quinazolinones. By using 30 mol% of trifluoroacetic acid (TFA) as the catalyst and tert-butyl hydroperoxide (TBHP) as the oxidant, the reaction of isatins 28 and 1,2,3,4-tetrahydroisoquinolines 29 in toluene at 120°C afforded the representative quinazolinones 30 in 52–82% yields (Scheme 8) (Jia et al., 2020). Isatins having either electron-withdrawing or donating groups on the aromatic ring smoothly participated in this reaction with no significant effect on the reaction rates. Similarly, 1,2,3,4-tetrahydroisoquinolines possessing electron-rich groups such as methoxy and halogenated groups such as bromo were established to be very efficient for this reaction. However, the reaction with alkyl-substituted benzyl amines and alkyl cyclic amines such as piperidine and pyrrolidine failed to work in this condition which marks the limitations of this approach. The synthetic application of the protocol was established by the Pd-catalyzed cross-coupling reaction of the product 30d to furnish the product 31. Moreover, the authors synthesized the natural alkaloid rutaecarpine 33 from the one-step reaction of isatin 28a and 2,3,4,9-tetrahydro-1H-pyrido [3,4-b]indole 32 under optimal conditions that also contribute the practical effectiveness of the present protocol.
The exploitation of N-formyl imide as a carbon source for the metal-free synthesis of quinazolinones was developed by Huang et al. (Scheme 9) (Huang et al., 2020). With the aid of 0.2 equivalents of pyridinium p-toluene sulfonate (PPTS) as the acid catalyst, the treatments of diverse N-substituted 2-aminobenzamide 34 and N-formyl imide 35 using tetrahydrofuran (THF) as a solvent at 70°C, the representative products 36 were accomplished in 71–97% yields within 8–24 h. The reaction conditions tolerate a wide variety of N-aryl and N-aliphatic substitutions on the 2-aminobenzamide ring. Although the present approach offered a lot of advantages such as mild reactions, easy setup procedure, metal-free, oxidant-free, and good functional group tolerances, the reaction required a prolonged time to complete, which marks the limitations of this approach’s otherwise outstanding developments.
Feng and Wu developed a metal-free procedure for the oxidative cyclization of 2-fluorobenzaldehyde 37 and 2-aminopyridines 38 to access quinazolinones. With the help of 1,4-diazabicyclo [2.2.2]octane (DABCO) as the basic organocatalyst and tert-butyl hydroperoxide (TBHP) as the oxidant, the representative products 39 were obtained in moderate to good yields (Scheme 10) (Feng and Wu, 2017). The presence of different substitutions such as methyl, chloro, and fluoro on the different positions of 2-aminopyridines leads to the corresponding products in sufficient yields, while bromo, cyano, and nitro-substituted 2-aminopyridines were unable to react under this standard condition, and only trace amounts of products were observed. This is most likely due to the reduced electron density on NH–Ar anion, thereby being unable to undergo nucleophilic substitution with electrophile 37. On the other hand, 6-methyl substituted 2-aminopyridines provided the simple non-cyclized amide as the final product rather than the quinazolinone product due to the steric hindrance. The reaction was further investigated for other benzaldehydes, including 2-bromobenzaldehyde and 2-nitrobenzaldehyde with 2-aminopyridines under optimal conditions, and the respective quinazolinone products were found to be formed in 36 and 10% yield, respectively.
Lee et al. demonstrated the exploitation of dimethyl sulfoxide (DMSO) as the carbon source for the assembly of quinazolinones under transition-metal-free conditions. With the aid of 1,4-diazabicyclo [2.2.2]octane (DABCO) as the basic catalyst and potassium persulfate (K2S2O8) as the additive, the microwave-assisted reaction of 2-amino benzamides 4 with DMSO furnished the representative products 43 in 47–72% yields within 2 h (Scheme 11) (Lee et al., 2019). The reaction conditions were well-tolerated for both N-unsubstituted and N-substituted 2-amino benzamides, with a slight change in the yield of the products. With N-substituted amides, all aliphatic and aromatic groups having electron-releasing substituents react smoothly to afford the products in quantitative yields. This is due to an increase in the nucleophilicity of the amide, thereby increasing the yield of the products by favoring annulation, whereas trifluoromethyl substituted amide also provided the product efficiently, albeit with a 47% yield. The strong electron-withdrawing groups such as cyano and nitro on the N-aryl ring of 4 decomposed under this reaction condition which marks the limitations of this approach. In addition to these, a wide variety of dialkyl-substituted sulfoxides was established to be very effective for this reaction to deliver the quinazolinone products 45. The proposed mechanism involves the reaction of DMSO with K2S2O8 to in situ form the sulfenium ion Int-11 which condensed with 4a to form Int-12. The intermediate Int-13 was formed from Int-12 after the elimination of CH3SH, which can then undergo intramolecular annulation to produce Int-14. The final autooxidation of Int-14 yields the final product 43a.
Chen et al. devised a microwave-assisted metal-free one-pot strategy for the synthesis of quinazolin-2,4-diones 48 from 2-aminobenzamides 46 and di-tert-butyl decarbonate (Boc)2O 47 as the precursor which constructed the carbonyl moiety at the 2-position of quinazolinediones. With the aid of 4-dimethylaminopyridine (DMAP) as the base catalyst, the representative product 48 could be accomplished either by microwave irradiation for 30 min or simple stirring at room temperature for 12 h in poor to excellent yields (Scheme 12) (Chen et al., 2020). Diverse substitutions on the nitrogen atom of 2-aminobenzamides by alkyl, benzyl, and aryl groups smoothly participated in this reaction. When R2 was an electron-donating group such as alkyl or benzyl, the yield of the product was increased as compared to aryl substitution with various electron-deficient groups that offered a lower yield, albeit with p-methoxy substitutions that yield 82% of the product. Furthermore, 2-aminobenzamides possessing methoxy or methyl group (R1 = Me, OMe) furnish the products with greater yields due to an increased nucleophilicity of the amino group, whereas electron-deficient groups (R1 = F, CF3) gave very low yields. Conversely, 1-substituted quinazoline-2,4-diones (R3 = Me, Et) have also been synthesized by this reaction but in very low yields.
Lin et al. demonstrated the one-pot construction of various 2,3-dialkyl substituted quinazolinones via the aza-annulation of secondary amides and isocyanates. With the aid of 2-bromopyridine as the base catalyst, the triflic anhydride (Tf2O) activated N-aryl amide 49 and isocyanates 50 afforded the representative quinazolinone products 51 in 41–92% yields. Broad functional group tolerances, mild setup procedure, and good chemoselectivity are some of the salient features of this protocol. The synthetic application of the present protocol was established by the acid-catalyzed reaction of product 51d with 4-formylbenzonitrile to form the biologically active 2-styrylquinazolinones such as product 52 (Scheme 13) (Lin et al., 2021).
SCHEME 13. Metal-free chemoselective one-pot construction of quinazolinones via reaction with isocyanates.
The introduction of thiamine hydrochloride, commonly known as vitamin B1 (VB1), as an efficient organocatalyst for the construction of 2,3-dihydroquinazolinones was developed by Devi et al. (Scheme 14A) (Devi et al., 2017). With water as the green mother nature solvent, the treatments of 2-aminobenzamide 4a and diverse aldehydes and ketones 11 were found to proceed smoothly under reflux conditions to deliver the representative products 12 in 74–86% yields. The reaction required only 10 mol% of the catalyst and was completed within a very short duration of time. To broaden the scopes of the reaction, a diverse range of heteroaryl and aryl-substituted aldehydes with varying electron-donating and electron-deficient substituents were explored and recognized to be very compatible with this reaction. On the other hand, ketones such as cyclopentanone, cycloheptanone, and acetone were well-tolerated, whereas acetophenone failed to deliver any product. The utilization of water as the solvent, reusable catalytic system, and low cost of the catalyst makes this protocol environmentally benign.
SCHEME 14. Vitamin B1 catalyzed water enabled synthesis of quinazolinones 12 (A) and enantioselective construction of quinazolinones 53 by organocatalyst C-3 (B).
Around the same time, a highly convergent and facile catalytic enantioselective construction of 2,3-dihydroquinazolinones was developed by Ayyanar et al. (Scheme 14B) (Ayyanar et al., 2017). With the support of 1 mol% of chiral organocatalyst C-3, the reaction of 2-aminobenzamide 4a and aldehydes 53 in methanol at 30 °C afforded the representative optically active products 54 in 94–99% yields with moderate to excellent enantioselectivity. The reaction conditions were very suitable for a vast array of aryl aldehydes to work efficiently with this reaction, and for ortho, para, or meta substitutions, better stereo control was achieved. In addition to demonstrating cyclohexanone as the reactive partner for this reaction, the reaction was not completed with aliphatic aldehydes (R = Me, C3H7). Interestingly, this reaction does not require any co-catalyst or additives to improve the enantioselectivity of the respective products.
Recently, multi-component reactions (MCRs), which allow the formation of multiple bonds in a single operation, have been demonstrated as a promising tool for the creation of diverse molecular structures with enhanced efficiency, reduced waste, and high atom economy from easily accessible simple and inexpensive starting materials by effortless mixing of the reactant (Rotstein et al., 2014; Borah et al., 2022c). The ability to accomplish the requisite products in “one-pot” by operationally simple workup procedure without using complex purification techniques and avoiding the isolation of the reaction intermediate features multi-component reaction, a powerful strategy for green or sustainable synthesis (Borah et al., 2022b).
Recognizing these features, Fozooni and Firoozi devised a one-pot procedure for the synthesis of 2,3-dihydroquinazolinones by introducing a microwave-assisted three-component reaction of isatoic anhydride 55, aldehydes 53, and 2-(4-aminobenzamido)acetic acid 56 (Scheme 15) (Fozooni and Firoozi, 2015). With the aid of acetic acid (AcOH) in ethanol, the representative products 57 were accomplished in 78–85% yields within 8–10 min. Aryl and heteroaryl substituted aldehydes have been selected to be very compatible for this reaction. Although the reaction proceeded under both microwave irradiation and the conventional method, the low yield of the products was observed by the conventional method and required a longer reaction time as compared to microwave techniques. The exploitation of microwaves not only enhances the yield but also reduces the reaction times and provides a clean pathway toward the products. Notwithstanding these developments, the narrow substrate scopes constitute the limitation of this protocol and call for further developments. The overall process proceeded through the decarboxylative reaction between acid-activated isatoic anhydride Int-15 and amine 56 to form an intermediate Int-16 which on reaction with activated aldehydes produce the imine intermediate Int-17. The subsequent cyclization of Int-17 delivered the products 57.
The introduction of ultrasound irradiation as a highly efficient and environmentally benign activation method for the one-pot assembly of 2,3-dihydroquinazolinones was realized by Chen et al. (Scheme 16A) (Chen et al., 2015). With water as the green solvent system and dodecylbenzene sulfonic acid (DBSA) as the catalyst, the ultrasound-assisted three-component reaction of isatoic anhydride 55, aldehydes 58, and aniline 59 afforded the representative quinazolinone products 60 in 62–76% yields within 1–2 h. In a similar manner, Fahimi and Sardarian synthesized a series of 2,3-substituted quinazolinones 61 from the reaction of isatoic anhydride 55, aldehydes 53, and aniline 59 by using citric acid as the catalyst in ethanol under reflux conditions. A broad variety of electron-deficient or electron-donating substituents have been smoothly worked under the standard conditions, and a total of 18 compounds were isolated in 78–95% yield (Scheme 16B) (Fahimi and Sardarian, 2016). Later, Karhale et al. disclosed the oxalic acid-catalyzed three-component reaction of isatoic anhydride 55, aldehydes 58, and ammonium acetate 62 for the rapid access to quinazolinone derivatives 63 in 72–92% yields (Scheme 16C) (Karhale et al., 2017). The broad functional groups, mild setup procedures, short reaction times, easy isolation, column-free, waste-free, and metal-free were some of the advantages of all the three procedures developed. However, the yield of the products in all the cases needs to be improved, which leaves a gap for further developments.
SCHEME 16. Domino synthesis of quinazolinones in different reaction conditions as developed by Chen et al., (A), Fahimi (B) and Karhale et al., (C).
A highly convenient one-pot strategy for the consecutive synthesis of quinazolinone sulfonamides was designed by Balalaie et al. from the four-component reaction of isatoic anhydride 55, aldehydes 58, saccharin 64, and hydrazine hydrate 65 (Scheme 17) (Balalaie et al., 2017). With the aid of propyl sulfonic acid functionalized SBA-15 (SBA-Pr-SO3H) as a heterogeneous catalyst in solvent-free conditions, the representative products 66 have been isolated in 60–90% yields after 4 h. The avoidance of solvents, utilization of readily accessible starting materials, and easy work-up procedure, are key salient features of this protocol. However, the narrow substrate scopes mark the limitations of this strategy.
A concise organocatalytic one-pot approach for the facile access to spiro-fused quinazolinones by means of acetic acid catalyzed three-component reaction of isatoic anhydride 55, aryl amines 67, and cyclic ketones 26 was demonstrated by Ramesh et al. With the aid of 10 mol% of the catalyst, the desired products 68 have been obtained in 81–97% yields (Scheme 18) (Ramesh et al., 2018). The reaction condition tolerates a broad range of functional groups with a slight decrease in yield in the case of aliphatic amines. This is presumably due to their high nucleophilicity. Overall, it has the advantages of mild reaction conditions, short reaction time, experimental simplicity, and excellent yields.
Taking advantage of the bifunctional donor-acceptor reactivity of 2-aminoethanesulfonic acid (taurine), Chate et al. disclosed a facile and efficient one-pot procedure for the assembly of diverse quinazolinone derivatives 70 under mild conditions (Scheme 19) (Chate et al., 2020). With the aid of 15 mol% of taurine as the organocatalyst, the three-component reaction of isatoic anhydride 55, isoniazid 69, and aldehydes 53 using water as the green solvent system, the corresponding products 70 have been obtained in 85–94% yields within 2–4 h under reflux conditions. Inspired by this result, they further investigated the reaction between isatins 71, isatoic anhydride 55, and amines 67 under standard conditions. Interestingly, the reaction afforded the spiro-oxindole-bearing quinazolinone products 75–90% yields 72 in. A wide variety of aryl and heteroaryl substituted aldehydes and amines were well-tolerable for both reactions. The practical application of the protocol was established by demonstrating the recyclability experiments of the catalyst up to three consecutive cycles with a slight change in catalytic properties. The formation of product 72a can be explained by the initial nucleophilic addition of amine 67a to taurine-activated isatoic anhydride followed by decarboxylation to form the intermediate Int-19. This intermediate on condensation with protonated isatin 71a delivered imine intermediate Int-20, and the subsequent intramolecular cyclization of Int-20 yields the final product 72a.
Dige et al. explored a highly convenient ultrasound-assisted strategy for the Brønsted acid-catalyzed construction of a variety of quinazolinones by a one-pot multi-component reaction of isatoic anhydride 55, 2-furoic hydrazides 74, and various substituted salicylaldehydes 73 (Scheme 20) (Dige et al., 2019). By employing 20 mol% of p-toluene sulfonic acid (p-TSA) in an aqueous ethanolic solution, the representative quinazolinone products 75 have been accomplished in 71–96% yields within 55–70 min of ultrasonication at room temperature. Pleasingly, it was found that salicylaldehydes possessing different electron-rich and electron-poor groups worked suitably in this reaction without having an adverse effect on the product yield. A few of the synthesized compounds were also shown to be tyrosinase enzyme inhibitors. Some of the main advantages of this strategy include the use of sound waves as an effective green way, combined green solvents, quick reaction times, and simple extraction methods.
Phakodee et al. disclosed a highly efficient Ph3P−I2 promoted synthesis of quinazolinones 78 from the treatment of methyl anthranilate 76, carboxylic acid or acid chloride 77, and amine 59. Diverse substitutions on ring 76 and various amines were found to be tolerable to deliver the products in 27–89% yield. Although the synthesis of quinazolinones from acid chlorides has smoothly proceeded, the synthesis directly from carboxylic acids was found to be very difficult and provides a low yield of the products. This might be due to the difficulties in the amide bond formation from aliphatic acids. Broad functional group tolerance, mild reaction conditions, and readily accessible starting materials are some of the advantages of this protocol (Scheme 21) (Phakhodee et al., 2017).
Treatment of isatoic anhydride 55, pyrazole substituted aldehydes 79, and diverse amines 59 proceeded smoothly in the presence of 5 mol% of l-proline to furnish the desired dihydro-quinazolinone products 80 in moderate to good yields, which on oxidation with potassium permanganate (KMnO4) yielded the quinazolinones bearing pyrazole core 81 after 8 h in 68–91% yields (Scheme 22) (Mehta et al., 2014). Most of the synthesized compounds have been established as antimicrobial, antifungal, and antituberculosis agents. The operational simplicity, green solvent system, mild reaction conditions, and biologically active compounds are some key features of this approach. However, the narrow substrate scopes with the low yield of the products point toward the drawback of this procedure.
A facile and eco-friendly domino multi-component strategy for the synthesis of quinazolinone derivatives was developed by Khandebharad et al., using triethanolamine (TEOA) as the catalyst (Scheme 23) (Khandebharad et al., 2020). With the aid of 10 mol% of the catalyst and 5 mol% of NaCl in the aqueous medium, the one-pot reaction of isatoic anhydride 55, aldehydes 53 with various nitrogen sources such as ammonium carbonate 82, amine 59, or phenyl hydrazine 84 under reflux conditions delivered the representative products 61, 83, and 85 in moderate to good yields respectively. The utilization of sodium chloride (NaCl) salt in this process increases the hydrophobic effect in aqueous media and controls the formation of micelles, thereby forming the desired product significantly in a cleaner way. On the other hand, because the reaction is temperature-dependent, a drop in temperature favors the development of micellar structures by reducing the hydration of the hydrophilic head group. Consequently, as the temperature rises, the micellization process occurs at a lower concentration. This process has several key characteristics that make it an effective and promising synthetic approach for the synthesis of quinazolinone derivatives, including straightforward reaction conditions, outstanding yield, a wide substrate range, and an easy work-up procedure.
SCHEME 23. TEOA in combination with NaCl-catalyzed synthesis of quinazolinones in an aqueous medium.
Kawade et al. developed a facile mechanochemical multi-component strategy for the efficient access to quinazolinone derivatives by the one-pot treatments of anthranilic acid 86, triethyl orthoformate 87, and aromatic amines 67 (Scheme 24) (Kawade et al., 2016). Grinding the reactants with the help of a mortar and pestle by using 10 mol% of thiamine hydrochloride (VB1) as the organocatalyst, the representative quinazolinone products 88 have been accomplished in 84–95% yields within 45–60 min. The reaction is not vulnerable to electron-donating and withdrawing groups on aromatic amine, not showing any considerable difference in the yield of the final products. In contrast, the reaction did not proceed with aliphatic amines, which marks the shortcoming of the current approach.
Recognizing the widespread distribution of quinazolinone heterocycle in the key fragments of natural alkaloids and pharmacologically active molecules, the elegant synthesis of these heterocycles has always received tremendous attention over the last decades to the present. However, in this 21st century, to move toward more green and sustainable chemistry, the development of new synthetic pathways by exploring the potentiality of simple easily accessible raw materials to construct highly functionalized quinazolinone molecules that will hold huge pharmaceutical applications is extremely demanding but challenging.
Notwithstanding the tremendous progress accomplished in the transition-metal-catalyzed construction of quinazolinone, most of them require other non-commercial supporting ligands, co-catalyst(s), additives, high energy conditions, and long reaction times. On the other hand, the high cost involved in the preparation of metal catalyst(s) marks the major limitations in quinazolinone chemistry. In addition, they are very sensitive to air and moisture. The removal of transition metal catalysts from a reaction mixture which is particularly crucial to the pharmaceutical industry often becomes a formidable challenge and is very expensive, which points toward the failure of green and more sustainable synthesis. Consequently, the occurrence of transition metal catalysts even at the lowest loading corresponds to disadvantageous effects on the environmentally friendly nature of the chemical process.
In this review article, we have demonstrated the recent organocatalytic approaches for the assembly of diverse quinazolinone derivatives. The ability to accomplish these heterocycles by means of transition-metal-free organocatalytic conditions with an immediate decrease in the cost of the transformations and the utilization of easily accessible starting material and green solvent system in most cases features the advantages of the developed synthetic procedures. From the aforementioned observations, it is clear to conclude that the exploitation of organocatalysts in different solvent systems such as water, ethanol, and methanol and solvent-free conditions offered the desired quinazolinones products in very good to excellent yields. Moreover, the reaction with organocatalysis does not necessitate any other supporting ligands, co-catalysts, oxidants, and other expensive materials in most cases, which demonstrates the sustainability and eco-friendly nature of the transformation. In most of the works, the representative quinazolinones were isolated pure without using any complex purification techniques such as column chromatography, thereby reducing the waste of solvents and time. Other remarkable properties include mild reaction conditions, broad functional group tolerances, and being environmentally benign. On the other hand, some of the existing work still needs to be developed due to the unusual requirements of longer reaction times, limited substrate scopes, and high catalyst loading.
We hope that the information presented here will stimulate and encourage the synthetic community to discover outstanding work in the elegant synthesis of these heterocycles by applying more convenient organocatalytic approaches and other synthetic approaches and to explore the potentiality of the quinazolinones to synthesize much more complex scaffolds for providing promising therapeutic significance and optoelectronic properties.
BB: Design, development, and conceptualization of the work and writing the original draft. SS: Writing–original draft. MP: Writing and resources. LC: Supervision.
BB thanks UGC-India for the fellowship and the Central University of Gujarat for the infrastructure to carry out the work.
The authors declare that the research was conducted in the absence of any commercial or financial relationships that could be construed as a potential conflict of interest.
All claims expressed in this article are solely those of the authors and do not necessarily represent those of their affiliated organizations, or those of the publisher, the editors, and the reviewers. Any product that may be evaluated in this article, or claim that may be made by its manufacturer, is not guaranteed or endorsed by the publisher.
Abbas, S. Y., El-Bayouki, K. A., and Basyouni, W. M. (2016). Utilization of isatoic anhydride in the syntheses of various types of quinazoline and quinazolinone derivatives. Synth. Commun. 46, 993–1035. doi:10.1080/00397911.2016.1177087
Anantha, I. S. S., Kerru, N., Maddila, S., and Jonnalagadda, S. B. (2021). Recent progresses in the multicomponent synthesis of dihydropyridines by applying sustainable catalysts under green conditions. Front. Chem. 9, 800236. doi:10.3389/fchem.2021.800236
Auti, P. S., George, G., and Paul, A. T. (2020). Recent advances in the pharmacological diversification of quinazoline/quinazolinone hybrids. RSC Adv. 10, 41353–41392. doi:10.1039/D0RA06642G
Ayyanar, S., Vijaya, P. K., Mariyappan, M., Ashokkumar, V., Sadhasivam, V., Balakrishnan, S., et al. (2017). Enantioselective synthesis of dihydroquinazolinone derivatives catalyzed by a chiral organocatalyst. New J. Chem. 41, 7980–7986. doi:10.1039/C7NJ00538E
Balalaie, S., Hekmat, S., Ramezanpour, S., Rominger, F., Kabiri-Fard, H., and Vavsari, V. F. (2017). An environmentally friendly approach for the synthesis of quinazolinone sulfonamide. Monatsh. Chem. 148, 1453–1461. doi:10.1007/s00706-017-1924-x
Barbas, C. F. (2008). Organocatalysis lost: modern chemistry, ancient chemistry, and an unseen biosynthetic apparatus. Angew. Chem. Int. Ed. 47, 42–47. doi:10.1002/anie.200702210
Bergman, J., and Bergman, S. (1985). Studies of rutaecarpine and related quinazolinocarboline alkaloids. J. Org. Chem. 50, 1246–1255. doi:10.1021/jo00208a018
Bertelsen, S., and Jørgensen, K. A. (2009). Organocatalysis—after the gold rush. Chem. Soc. Rev. 38, 2178–2189. doi:10.1039/B903816G
Bhunia, A., Yetra, S. R., and Biju, A. T. (2012). Recent advances in transition-metal-free carbon–carbon and carbon–heteroatom bond-forming reactions using arynes. Chem. Soc. Rev. 41, 3140–3152. doi:10.1039/C2CS15310F
Borah, B., and Chowhan, L. R. (2021). Recent advances in the transition-metal-free synthesis of quinoxalines. RSC Adv. 11, 37325–37353. doi:10.1039/D1RA06942J
Borah, B., and Chowhan, L. R. (2022a). Recent updates on the stereoselective synthesis of structurally functionalized spiro-oxindoles mediated by isatin N, N´ cyclic azomethine imine 1, 3-dipoles. Tetrahedron Lett. 104, 154014. doi:10.1016/j.tetlet.2022.154014
Borah, B., and Chowhan, L. R. (2022b). Ultrasound-assisted transition-metal-free catalysis: a sustainable route towards the synthesis of bioactive heterocycles. RSC Adv. 12, 14022–14051. doi:10.1039/D2RA02063G
Borah, B., Dwivedi, K. D., Kumar, B., and Chowhan, L. R. (2021a). Recent advances in the microwave-and ultrasound-assisted green synthesis of coumarin-heterocycles. Arab. J. Chem. 15, 103654. doi:10.1016/j.arabjc.2021.103654
Borah, B., Dwivedi, K. D., and Chowhan, L. R. (2021b). Recent approaches in the organocatalytic synthesis of pyrroles. RSC Adv. 11, 13585–13601. doi:10.1039/D1RA01690C
Borah, B., Dhar Dwivedi, K., and Chowhan, L. R. (2021c). 4‐Hydroxycoumarin: A versatile substrate for transition‐metal‐free multicomponent synthesis of bioactive heterocycles. Asian J. Org. Chem. 10, 3101–3126. doi:10.1002/ajoc.202100550
Borah, B., Dwivedi, K. D., and Chowhan, L. R. (2021d). Recent advances in metal‐and organocatalyzed asymmetric functionalization of pyrroles. Asian J. Org. Chem. 10, 2709–2762. doi:10.1002/ajoc.202100427
Borah, B., Patat, M., Swain, S., and Chowhan, L. R. (2022a). Recent advances and prospects in the transition‐metal‐free synthesis of 1, 4‐dihydropyridines. ChemistrySelect 7, e202202484. doi:10.1002/slct.202202484
Borah, B., Bora, J., Ramesh, P., and Chowhan, L. R. (2022b). Sonochemistry in an organocatalytic domino reaction: an expedient multicomponent access to structurally functionalized dihydropyrano [3, 2-b] pyrans, spiro-pyrano [3, 2-b] pyrans, and spiro-indenoquinoxaline-pyranopyrans under ambient conditions. RSC Adv. 12, 12843–12857. doi:10.1039/D2RA01917E
Bur, S. K., and Padwa, A. (2004). The pummerer reaction: methodology and strategy for the synthesis of heterocyclic compounds. Chem. Rev. 104, 2401–2432. doi:10.1021/cr020090l
Chate, A. V., Rudrawar, P. P., Bondle, G. M., and Sangeshetti, J. N. (2020). 2-Aminoethanesulfonic acid: An efficient organocatalyst for green synthesis of spirooxindole dihydroquinazolinones and novel 1, 2-(dihydroquinazolin-3 (4 H) isonicotinamides in water. Synth. Commun. 50, 226–242. doi:10.1080/00397911.2019.1692868
Chatterjee, T., Bhadra, S., and Ranu, B. C. (2011). Transition metal-free procedure for the synthesis of S-aryl dithiocarbamates using aryl diazonium fluoroborate in water at room temperature. Green Chem. 13, 1837–1842. doi:10.1039/C1GC00001B
Chen, A. L., and Chen, K. K. (1933). The constituents of Wu chü yü (Evodia rutæcarpa)**From the lilly research laboratories, eli lilly and company, indianapolis. J. Am. Pharm. Assoc. 22, 716–719. doi:10.1002/jps.3080220804
Chen, B. H., Li, J. T., and Chen, G. F. (2015). Efficient synthesis of 2, 3-disubstituted-2, 3-dihydroquinazolin-4 (1H)-ones catalyzed by dodecylbenzenesulfonic acid in aqueous media under ultrasound irradiation. Ultrason. Sonochem. 23, 59–65. doi:10.1016/j.ultsonch.2014.08.024
Chen, H., Li, P., Qin, R., Yan, H., Li, G., and Huang, H. (2020). DMAP-catalyzed one-pot synthesis of quinazoline-2, 4-diones from 2-aminobenzamides and di-tert-butyl dicarbonate. ACS Omega 5, 9614–9623. doi:10.1021/acsomega.0c01104
Chugh, A., Kumar, A., Verma, A., Kumar, S., and Kumar, P. (2020). A review of antimalarial activity of two or three nitrogen atoms containing heterocyclic compounds. Med. Chem. Res. 29, 1723–1750. doi:10.1007/s00044-020-02604-6
Dalko, P. I., and Moisan, L. (2001). Enantioselective organocatalysis. Angew. Chem. Int. Ed. 40, 3726–3748. doi:10.1002/1521-3773(20011015)40:20%3C3726::AID-ANIE3726%3E3.0.CO;2-D
Devi, J., Kalita, S. J., and Deka, D. C. (2017). Expeditious synthesis of 2, 3-dihydroquinazolin-4 (1 H)-ones in aqueous medium using thiamine hydrochloride (VB1) as a mild, efficient, and reusable organocatalyst. Synth. Commun. 47, 1601–1609. doi:10.1080/00397911.2017.1337149
Dherbassy, Q., Manna, S., Shi, C., Prasitwatcharakorn, W., Crisenza, G. E., Perry, G. J., et al. (2021). Enantioselective copper‐catalyzed borylative cyclization for the synthesis of quinazolinones. Angew. Chem. 133, 14476–14480. doi:10.1002/ange.202103259
Dige, N. C., Mahajan, P. G., Raza, H., Hassan, M., Vanjare, B. D., Hong, H., et al. (2019). Ultrasound mediated efficient synthesis of new 4-oxoquinazolin-3 (4H)-yl) furan-2-carboxamides as potent tyrosinase inhibitors: Mechanistic approach through chemoinformatics and molecular docking studies. Bioorg. Chem. 92, 103201. doi:10.1016/j.bioorg.2019.103201
D'Souza, D. M., and Mueller, T. J. (2007). Multi-component syntheses of heterocycles by transition-metal catalysis. Chem. Soc. Rev. 36, 1095–1108. doi:10.1039/B608235C
Dwivedi, K. D., Borah, B., and Chowhan, L. R. (2020). Ligand free one-pot synthesis of pyrano [2, 3-c] pyrazoles in water extract of banana peel (WEB): a green chemistry approach. Front. Chem. 7, 944. doi:10.3389/fchem.2019.00944
Fahimi, N., and Sardarian, A. R. (2016). Citric acid: A green bioorganic catalyst for one-pot three-component synthesis of 2, 3-dihydroquinazoline-4 (1H)-ones. Curr. Organocatal. 3, 39–44. Available at: https://www.ingentaconnect.com/content/ben/cocat/2016/00000003/00000001/art00008. doi:10.2174/2213337202666150602221505
Faisal, M., and Saeed, A. (2021). Chemical insights into the synthetic chemistry of quinazolines: Recent advances. Front. Chem. 8, 594717. doi:10.3389/fchem.2020.594717
Feng, J. B., and Wu, X. F. (2017). Oxidative synthesis of quinazolinones under metal‐free conditions. J. Heterocycl. Chem. 54, 794–798. doi:10.1002/jhet.2562
Fozooni, S., and Firoozi, H. (2015). Microwave-assisted synthesis of new quinazolinone and (dihydroquinazolinylphenyl) oxazolone derivatives. Chem. Heterocycl. Compd. (N. Y). 51, 340–345. doi:10.1007/s10593-015-1705-6
Gunasekara, N. S., and Faulds, D. (1998). Raltitrexed. Drugs 55, 423–435. doi:10.2165/00003495-199855030-00012
Gupta, T., Rohilla, A., Pathak, A., Akhtar, M. J., Haider, M. R., and Yar, M. S. (2018). Current perspectives on quinazolines with potent biological activities: A review. Synth. Commun. 48, 1099–1127. doi:10.1080/00397911.2018.1431282
Hakim, F., Salfi, R., Bhikshapathi, D., and Khan, A. (2022). Anticancer evaluation of novel quinazolinone acetamides: Synthesis and characterization. Anticancer. Agents Med. Chem. 22, 926–932. doi:10.2174/1871520621666210524164351
Hao, Y., Wang, K., Wang, Z., Liu, Y., Ma, D., and Wang, Q. (2020). Luotonin A and its derivatives as novel antiviral and antiphytopathogenic fungus agents. J. Agric. Food Chem. 68, 8764–8773. doi:10.1021/acs.jafc.0c04278
Hao, S., Yang, J., Liu, P., Xu, J., Yang, C., and Li, F. (2021). Linear-organic-polymer-supported iridium complex as a recyclable auto-tandem catalyst for the synthesis of quinazolinones via selective hydration/acceptorless dehydrogenative coupling from o-aminobenzonitriles. Org. Lett. 23, 2553–2558. doi:10.1021/acs.orglett.1c00475
He, L., Li, H., Chen, J., and Wu, X. F. (2014). Recent advances in 4 (3 H)-quinazolinone syntheses. RSC Adv. 4, 12065–12077. doi:10.1039/C4RA00351A
Hekal, M. H., and Abu El-Azm, F. S. (2018). New potential antitumor quinazolinones derived from dynamic 2-undecyl benzoxazinone: synthesis and cytotoxic evaluation. Synth. Commun. 48, 2391–2402. doi:10.1080/00397911.2018.1490433
Huang, H. Y., Lin, X. Y., Yen, S. Y., and Liang, C. F. (2020). Facile access to N-formyl imide as an N-formylating agent for the direct synthesis of N-formamides, benzimidazoles and quinazolinones. Org. Biomol. Chem. 18, 5726–5733. doi:10.1039/D0OB01080D
Jia, F. C., Chen, T. Z., and Hu, X. Q. (2020). TFA/TBHP-promoted oxidative cyclisation for the construction of tetracyclic quinazolinones and rutaecarpine. Org. Chem. Front. 7, 1635–1639. doi:10.1039/D0QO00345J
Karhale, S., Survase, D., Bhat, R., Ubale, P., and Helavi, V. (2017). A practical and green protocol for the synthesis of 2, 3-dihydroquinazolin-4 (1H)-ones using oxalic acid as organocatalyst. Res. Chem. Intermed. 43, 3915–3924. doi:10.1007/s11164-016-2855-6
Kawade, D. S., Chaudhari, M. A., Gujar, J. B., and Shingare, M. S. (2016). Thiamine hydrochloride (vitamin B1) as an efficient catalyst for the synthesis of 4-(3H)-Quinazolinone derivatives using grinding method. Iran. J. Catal. 6, 313–318.
Khan, I., Ibrar, A., Abbas, N., and Saeed, A. (2014). Recent advances in the structural library of functionalized quinazoline and quinazolinone scaffolds: Synthetic approaches and multifarious applications. Eur. J. Med. Chem. 76, 193–244. doi:10.1016/j.ejmech.2014.02.005
Khan, I., Ibrar, A., Ahmed, W., and Saeed, A. (2015). Synthetic approaches, functionalization and therapeutic potential of quinazoline and quinazolinone skeletons: the advances continue. Eur. J. Med. Chem. 90, 124–169. doi:10.1016/j.ejmech.2014.10.084
Khan, I., Zaib, S., Batool, S., Abbas, N., Ashraf, Z., Iqbal, J., et al. (2016). Quinazolines and quinazolinones as ubiquitous structural fragments in medicinal chemistry: An update on the development of synthetic methods and pharmacological diversification. Bioorg. Med. Chem. 24, 2361–2381. doi:10.1016/j.bmc.2016.03.031
Khan, I., Zaib, S., and Ibrar, A. (2020). New frontiers in the transition-metal-free synthesis of heterocycles from alkynoates: an overview and current status. Org. Chem. Front. 7, 3734–3791. doi:10.1039/D0QO00698J
Khandebharad, A. U., Sarda, S. R., Gill, C. H., and Agrawal, B. R. (2020). Synthesis of quinazolinone derivatives catalyzed by triethanolamine/NaCl in aqueous media. Polycycl. Aromat. Compd. 40, 437–445. doi:10.1080/10406638.2018.1441884
Köhne, C. H., Thuss-Patience, P., Friedrich, M., Daniel, P. T., Kretzschmar, A., Benter, T., et al. (1998). Raltitrexed (Tomudex): an alternative drug for patients with colorectal cancer and 5-fluorouracil associated cardiotoxicity. Br. J. Cancer 77, 973–977. doi:10.1038/bjc.1998.160
Kshirsagar, U. A. (2015). Recent developments in the chemistry of quinazolinone alkaloids. Org. Biomol. Chem. 13, 9336–9352. doi:10.1039/C5OB01379H
Kumar, B., Babu, N. J., and Chowhan, R. L. (2022). Sustainable synthesis of highly diastereoselective & fluorescent active spirooxindoles catalyzed by copper oxide nanoparticle immobilized on microcrystalline cellulose. Appl. Organomet. Chem. 36, e6742. doi:10.1002/aoc.6742
Kumar, P., Tomar, V., Joshi, R. K., and Nemiwal, M. (2022). Nanocatalyzed synthetic approach for quinazoline and quinazolinone derivatives: A review (2015–present). Synth. Commun. 52, 795–826. doi:10.1080/00397911.2022.2041667
Lee, S., Sim, J., Jo, H., Viji, M., Srinu, L., Lee, K., et al. (2019). Transition metal-free synthesis of quinazolinones using dimethyl sulfoxide as a synthon. Org. Biomol. Chem. 17, 8067–8070. doi:10.1039/C9OB01629E
Li, Z., Dong, J., Chen, X., Li, Q., Zhou, Y., and Yin, S. F. (2015). Metal-and oxidant-free synthesis of quinazolinones from β-ketoesters with o-aminobenzamides via phosphorous acid-catalyzed cyclocondensation and selective C–C bond cleavage. J. Org. Chem. 80, 9392–9400. doi:10.1021/acs.joc.5b00937
Lin, Y., He, S. F., Geng, H., Xiao, Y. C., Ji, K. L., Zheng, J. F., et al. (2021). Chemoselective reactions of isocyanates with secondary amides: One-pot construction of 2, 3-dialkyl-substituted quinazolinones. J. Org. Chem. 86, 5345–5353. doi:10.1021/acs.joc.0c02929
Liu, H., Zhai, T., Ding, S., Hou, Y., Zhang, X., Feng, L., et al. (2016). Direct and metal-free oxidative amination of sp 3 C–H bonds for the construction of 2-hetarylquinazolin-4 (3 H)-ones. Org. Chem. Front. 3, 1096–1099. doi:10.1039/C6QO00231E
Ma, Z. Z., Hano, Y., Nomura, T., and Chen, Y. J. (1997). Two new pyrroloquinazolinoquinoline alkaloids from Peganum nigellastrum. Heterocycles 46, 541–546. doi:10.3987/com-97-s65
MacMillan, D. W. (2008). The advent and development of organocatalysis. Nature 455 (7211), 304–308. doi:10.1038/nature07367
Maiden, T. M. M., and Harrity, J. P. A. (2016). Recent developments in transition metal catalysis for quinazolinone synthesis. Org. Biomol. Chem. 14, 8014–8025. doi:10.1039/C6OB01402J
Majumdar, P., Pati, A., Patra, M., Behera, R. K., and Behera, A. K. (2014). Acid hydrazides, potent reagents for synthesis of oxygen-nitrogen-and/or sulfur-containing heterocyclic rings. Chem. Rev. 114, 2942–2977. doi:10.1021/cr300122t
Mehta, H. B., Dixit, B. C., and Dixit, R. B. (2014). L-Proline catalyzed one-pot multi-component synthesis of 2-(1, 3-diphenyl-1H-pyrazol-4-yl) quinazolin-4 (3H)-one derivatives and their biological studies. Chin. Chem. Lett. 25, 741–744. doi:10.1016/j.cclet.2014.03.015
Mermer, A., Keles, T., and Sirin, Y. (2021). Recent studies of nitrogen containing heterocyclic compounds as novel antiviral agents: A review. Bioorg. Chem. 114, 105076. doi:10.1016/j.bioorg.2021.105076
Mhaske, S. B., and Argade, N. P. (2006). The chemistry of recently isolated naturally occurring quinazolinone alkaloids. Tetrahedron 62, 9787–9826. doi:10.1016/j.tet.2006.07.098
Mohammadkhani, L., and Heravi, M. M. (2020). Microwave-assisted synthesis of quinazolines and quinazolinones: an overview. Front. Chem. 8, 580086. doi:10.3389/fchem.2020.580086
Moon, T. C., Murakami, M., Kudo, I., Son, K. H., Kim, H. P., Kang, S. S., et al. (1999). A new class of COX-2 inhibitor, rutaecarpine from Evodia rutaecarpa. Inflamm. Res. 48, 621–625. doi:10.1007/s000110050512
Nakamura, I., and Yamamoto, Y. (2004). Transition-metal-catalyzed reactions in heterocyclic synthesis. Chem. Rev. 104, 2127–2198. doi:10.1021/cr020095i
Patrusheva, O. S., Volcho, K. P., and Salakhutdinov, N. F. (2018). Synthesis of oxygen-containing heterocyclic compounds based on monoterpenoids. Russ. Chem. Rev. 87, 771–796. doi:10.1070/RCR4810
Peng, J., Lin, T., Wang, W., Xin, Z., Zhu, T., Gu, Q., et al. (2013). Antiviral alkaloids produced by the mangrove-derived fungus Cladosporium sp. PJX-41. J. Nat. Prod. 76, 1133–1140. doi:10.1021/np400200k
Phakhodee, W., Wangngae, S., and Pattarawarapan, M. (2017). Approach to the synthesis of 2, 3-disubstituted-3 H-quinazolin-4-ones mediated by Ph3P–I2. J. Org. Chem. 82, 8058–8066. doi:10.1021/acs.joc.7b01322
Poudel, T. N., Tamargo, R. J. I., Cai, H., and Lee, Y. R. (2018). Recent progress in transition‐metal‐free, base‐mediated benzannulation reactions for the synthesis of a diverse range of aromatic and heteroaromatic compounds. Asian J. Org. Chem. 7, 985–1005. doi:10.1002/ajoc.201800080
Rahman, M., Ling, I., Abdullah, N., Hashim, R., and Hajra, A. (2015). Organocatalysis by p-sulfonic acid calix [4] arene: a convenient and efficient route to 2, 3-dihydroquinazolin-4 (1 H)-ones in water. RSC Adv. 5, 7755–7760. doi:10.1039/C4RA16374E
Ramesh, R., Kalisamy, P., Malecki, J. G., and Lalitha, A. (2018). Metal-free mild synthesis of novel 1′ H-spiro [cycloalkyl-1, 2′-quinazolin]-4′(3′ H)-ones by an organocatalytic cascade reaction. Synlett 29, 203–208. doi:10.1055/s-0036-1590917
Rao, Y., Liu, H., Gao, L., Yu, H., Tan, J. H., Ou, T. M., et al. (2015). Discovery of natural alkaloid bouchardatine as a novel inhibitor of adipogenesis/lipogenesis in 3T3-L1 adipocytes. Bioorg. Med. Chem. 23, 4719–4727. doi:10.1016/j.bmc.2015.05.057
Reetz, M. T. (2013). Biocatalysis in organic chemistry and biotechnology: past, present, and future. J. Am. Chem. Soc. 135, 12480–12496. doi:10.1021/ja405051f
Ren, Q., and Wang, J. (2013). Recent developments in amine‐catalyzed non‐asymmetric transformations. Asian J. Org. Chem. 2, 542–557. doi:10.1002/ajoc.201200191
Renzi, P., and Bella, M. (2012). Non-asymmetric organocatalysis. Chem. Commun. 48, 6881–6896. doi:10.1039/C2CC31599H
Revathy, K., and Lalitha, A. (2015). p-TSA-catalyzed synthesis of spiroquinazolinones. J. Iran. Chem. Soc. 12, 2045–2049. doi:10.1007/s13738-015-0680-2
Rohokale, R. S., and Kshirsagar, U. A. (2016). Advanced synthetic strategies for constructing quinazolinone scaffolds. Synthesis 48, 1253–1268. doi:10.1055/s-0035-1560413
Rotstein, B. H., Zaretsky, S., Rai, V., and Yudin, A. K. (2014). Small heterocycles in multicomponent reactions. Chem. Rev. 114, 8323–8359. doi:10.1021/cr400615v
Shanafelt, T. D., Borah, B. J., Finnes, H. D., Chaffee, K. G., Ding, W., Leis, J. F., et al. (2015). Impact of ibrutinib and idelalisib on the pharmaceutical cost of treating chronic lymphocytic leukemia at the individual and societal levels. J. Oncol. Pract. 11, 252–258. doi:10.1200/JOP.2014.002469
Shang, X., and Liu, Z. Q. (2013). Transition metal-catalyzed C vinyl–C vinyl bond formation via double C vinyl–H bond activation. Chem. Soc. Rev. 42, 3253–3260. doi:10.1039/C2CS35445D
Shen, G., Zhou, H., Du, P., Liu, S., Zou, K., and Uozumi, Y. (2015). Brønsted acid-catalyzed selective C–C bond cleavage of 1, 3-diketones: a facile synthesis of 4 (3 H)-quinazolinones in aqueous ethyl lactate. RSC Adv. 5, 85646–85651. doi:10.1039/C5RA17969F
Sugai, T., Yamazaki, T., Yokoyama, M., and Ohta, H. (1997). Biocatalysis in organic synthesis: the use of nitrile-and amide-hydrolyzing microorganisms. Biosci. Biotechnol. Biochem. 61, 1419–1427. doi:10.1271/bbb.61.1419
Sun, C. L., and Shi, Z. J. (2014). Transition-metal-free coupling reactions. Chem. Rev. 114, 9219–9280. doi:10.1021/cr400274j
Wang, Y. B., Zheng, S. C., Hu, Y. M., and Tan, B. (2017). Brønsted acid-catalysed enantioselective construction of axially chiral arylquinazolinones. Nat. Commun. 8, 15489. doi:10.1038/ncomms15489
Xing, Z., Wu, W., Miao, Y., Tang, Y., Zhou, Y., Zheng, L., et al. (2021). Recent advances in quinazolinones as an emerging molecular platform for luminescent materials and bioimaging. Org. Chem. Front. 8, 1867–1889. doi:10.1039/D0QO01425G
Yang, X., Cheng, G., Shen, J., Kuai, C., and Cui, X. (2015). Cleavage of the C–C triple bond of ketoalkynes: synthesis of 4 (3 H)-quinazolinones. Org. Chem. Front. 2, 366–368. doi:10.1039/C4QO00260A
Yashwantrao, G., Jejurkar, V. P., Kshatriya, R., and Saha, S. (2019). Solvent-free, mechanochemically scalable synthesis of 2, 3-dihydroquinazolin-4 (1H)-one using Brønsted acid catalyst. ACS Sustain. Chem. Eng. 7, 13551–13558. doi:10.1021/acssuschemeng.9b03199
Zhou, Q. L. (2016). Transition‐metal catalysis and organocatalysis: where can progress be expected? Angew. Chem. Int. Ed. 55, 5352–5353. doi:10.1002/anie.201509164
Keywords: quinazolinone, organocatalyst, heterocycles, natural product, therapeutic agents
Citation: Borah B, Swain S, Patat M and Chowhan LR (2022) Recent advances and prospects in the organocatalytic synthesis of quinazolinones. Front. Chem. 10:991026. doi: 10.3389/fchem.2022.991026
Received: 11 July 2022; Accepted: 26 July 2022;
Published: 14 September 2022.
Edited by:
Pezhman Shiri, Shiraz University of Medical Sciences, IranReviewed by:
Seyyed Amir Siadati, Qaemshahr Islamic Azad University, IranCopyright © 2022 Borah, Swain, Patat and Chowhan. This is an open-access article distributed under the terms of the Creative Commons Attribution License (CC BY). The use, distribution or reproduction in other forums is permitted, provided the original author(s) and the copyright owner(s) are credited and that the original publication in this journal is cited, in accordance with accepted academic practice. No use, distribution or reproduction is permitted which does not comply with these terms.
*Correspondence: L. Raju Chowhan, cmNob3doYW5AY3VnLmFjLmlu
Disclaimer: All claims expressed in this article are solely those of the authors and do not necessarily represent those of their affiliated organizations, or those of the publisher, the editors and the reviewers. Any product that may be evaluated in this article or claim that may be made by its manufacturer is not guaranteed or endorsed by the publisher.
Research integrity at Frontiers
Learn more about the work of our research integrity team to safeguard the quality of each article we publish.