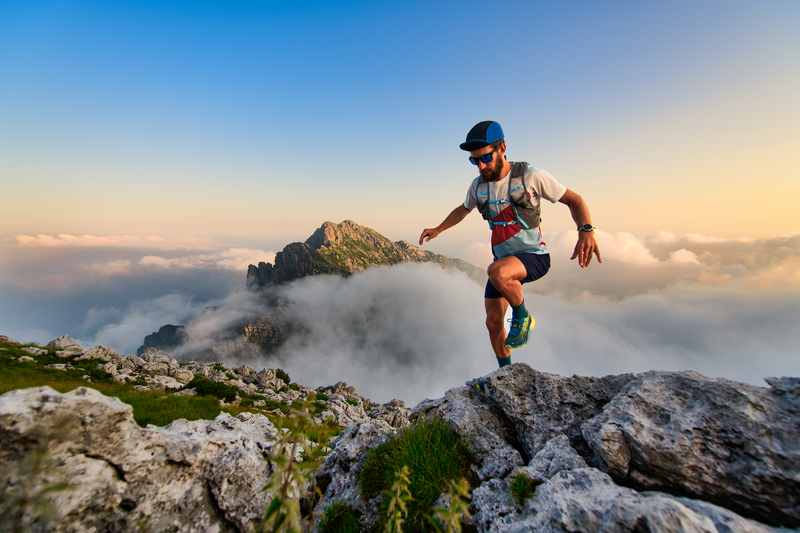
95% of researchers rate our articles as excellent or good
Learn more about the work of our research integrity team to safeguard the quality of each article we publish.
Find out more
ORIGINAL RESEARCH article
Front. Chem. , 23 August 2022
Sec. Catalytic Reactions and Chemistry
Volume 10 - 2022 | https://doi.org/10.3389/fchem.2022.978698
A correction has been applied to this article in:
Corrigendum: Influence of carrier effect on Pd/Al2O3 for methane complete catalytic oxidation
Pd/Al2O3 catalysts modified by different chemical elements (Mg, Si, Ce, and Zr) were tested for methane (CH4) catalytic combustion, and PdO nanoparticles loaded on modified Al2O3 were systematically studied. These conditions assess the carrier effects of Pd/Al2O3 and acid strength influences on CH4 combustion. We observed carrier effects on activation energy through tuning Pd 3d binding energies (BEs) and on pre-exponential factors (A) through Pd dispersion and acidity on supports. When the BE of Pd 3d5/2 is 337.3 eV, PdO nanoparticles loaded on modified Al2O3 have excellent activity in cracking the C−H bond of CH4, which leads to the lowest activation energy (Ea), regardless of the size effect of the PdO nanoparticle. Furthermore, a theoretical construction that acid sites on catalysts promote the reversible elementary step (2Pd−OH ↔ Pd−O* + Pd* + H2O) right shifts improving the A dependency on the quantity of exposed Pd* and Pd−O*. As a result, Al2O3, as the carrier, not only modifies the electronic characteristics and size of supported PdO nanoparticles but also participates in the reaction process via acid sites on the surface of Al2O3.
CH4, being one of the most significant greenhouse gases, has a global warming potential 20 times greater than carbon dioxide (CO2) (Zimmerle et al., 2020). Anthropogenic activities are to blame for the rise in atmospheric methane (CH4) throughout the industrial era, according to the Intergovernmental Panel on Climate Change (IPCC) Fifth Assessment Report in 2013. Anthropogenic sources account for over 60% of worldwide methane emissions, with fossil fuel production and usage accounting for roughly one-third of anthropogenic methane emissions (Alden et al., 2020). The majority of emissions from fossil fuels come from natural gas supply networks (Anthony 2008; Yang et al., 2022a). Recently, greater scientific, governmental, social, and industry focus has been placed on CH4 emissions (Cardoso-Saldana and Allen, 2020). The catalytic oxidation of CH4 has been widely investigated (Li et al., 2020; Jang et al., 2021; He et al., 2019). So far, researchers in this field have utilized a broad range of catalysts, including those based on noble metals (Pt and Pd) (Gao et al., 2021; Jang et al., 2021), transition metal oxides (Singh et al., 2020), and perovskite- and spinel-type materials (Lim et al., 2017a; Chu et al., 2022). Pd is thought to be a promising active ingredient in the oxidation of CH4 (Ding et al., 2020). Oxide materials, which are commonly utilized as supports for heterogeneous catalysts’ active metal nanoparticles, are known to impact catalytic activity via electronic metal-support interaction (Campbell 2012). The catalytic activity of supported Pd has been rationalized using aluminum oxides (Al2O3) (Gao et al., 2008; Satsuma et al., 2015a; Price et al., 2016; Shi et al., 2022), molecular sieves (Lei et al., 2020; Zhang et al., 2020), transition metal oxides (Khan et al., 2020; Li et al., 2020), and hydrotalcite oxides (Zhan et al., 2019). Al2O3 is a significant class of materials that are widely employed as catalyst supports in a variety of industries due to their large surface area and structural durability at high temperatures (Xu et al., 2021).
Extensive research has been conducted to determine how to improve the Pd/Al2O3 activity by modifying the Al2O3 surface. Chen et al. (2020a) developed uniform regular mesopore distribution and high-specific surface area Pd/Al2O3 to limit active palladium migration and sintering. Jeong et al. (2020) and Cargnello et al. (2012) create Pd@CeO2 structures that are uniformly anchored on the alumina support, resulting in unusually rapid methane oxidation due to improved metal-support interactions. Mg (Zhan et al., 2019), Ni (Zou et al., 2020), Co (Satsuma et al., 2015b; Lin et al., 2020), and La (Liu et al., 2013) were also used to modify the Pd/Al2O3 surface, resulting in perovskite- and spinel-type MgAlO2, NiAlO2, CoAlO2, and LaAlO3 with the maximum Pd↔PdO transformation capacity and hence high catalytic activity towards CH4 (Zhan et al., 2019). Notably, Yazawa et al. (1999) and Yazawa et al. (2000) found that the activity of supported Pd was influenced by the acid strength of the modified Al2O3, owing to the acidic support materials impeding the oxidation of supported palladium. However, Zhang et al. (2020) found that while alumina supports modified with and without phosphate have similar oxidation states of supported Pd, the Pd/Al2O3 without phosphate demonstrated better catalytic performance than with phosphate, indicating that formed cristobalite-type aluminum phosphate (AlPO4) on the Al2O3 surface decreases amounts of acid strength. Furthermore, the acid strength of the Al2O3 impacts not just the oxidation of supported Pd but may also engage in methane reaction pathways. Wang et al. (2010) verified that the unpaired electron on an O atom bonded with Al can draw a hydrogen atom from CH4 to produce CH3*. As a result, identifying the active species of Pd/Al2O3 catalyst and clarifying the carrier effect of Pd/Al2O3 is necessary to improve the catalytic activities.
To understand the Al2O3 carrier effects properly, we must first understand the mechanics of supported Pd catalyzing CH4. For decades, researchers have devoted substantial efforts to rationalizing the catalytic activity of supported Pd catalysts, and many mechanisms have been proposed (Xiong et al., 2018; Chen et al., 2020b). Monteiro et al. (2001a) found that PdO is the active phase in the reaction of methane degradation, which proved that the increase in the PdO surface area on metallic Pd foils leads to the activation rate constant of CH4 increasing monotonically. C−H bond activation of CH4 is the limit step. In PdO phase, C−H bonds can be activated by Pd2+-O2− ion pairs, in which four-center (HC3δ−−Pd2+−O2−−Hδ+)⧧ transition states formation via σ-bond metathesis pathways and stabilize the C−H bond (Fujimoto et al., 1998; Chin et al., 2016). Also, Pd2+−CH3δ−, Pd2+−Hδ+, and HC3δ−−Hδ+ interactions are stronger than with metallic Pd. Chin et al. (2016) concluded that because of the disparities in C–H bond activation transition states, activation barriers and pre-exponential variables are significantly different. Noted here, the catalytic activity varied depending on the type of support materials used. The interactions between Pd active phases and support are still not fully understood, although such information is crucial for the real application of Pd/Al2O3 in effective CH4 catalytic oxidation. The activity of CH4 catalytic oxidation of Pd/Al2O3 cannot be attributed solely to high surface area and structural stability at elevated temperature (Wang et al., 2019; Cao et al., 2023), but crystal defects of alumina, as reactive sponges (Karl et al., 1999), construct acid sites, and nanostructures affect the catalytic system (Chen et al., 2020a). For instance, Lim et al. (2017b) reported that the acidity of zeolite (Pd-SSZ-13, Pd-LTA, Pd-PST-7, and Pd-RTH) was positively correlated with the activity of CH4 combustion.
Herein, the carrier effect on catalytic oxidation of CH4 over PdOx catalyst at the range of 200–500°C was studied by using a series of Al2O3 as support materials modified by Si, Mg, Ce, and Zr. In detail, 1 wt% Pd-loaded catalysts were subjected to catalytic testing under the same condition (1,000 ppm CH4, 10% O2, and N2 as balance gas). These data show that modified Al2O3 influences the size distribution of PdO nanoparticles, binding energy of Pd 3d, and acid strength of the catalysts. The catalytic activities of samples do not exhibit size effects consistent with the literature (Chin et al., 2016) that the smaller the PdO particles, the higher activities, which are kinetic restrictions under 700°C. The relationship between palladium oxidation state and catalytic activity in diverse combinations revealed that the activation energy for each catalyst is dependent on the palladium oxidation state. When the binding energy of Pd 3d5/2 is 337.3 eV, cracking the C−H bond of CH4, it has the lowest activation energy (Ea). To understand the effect of Lewis acids of catalysts on pre-exponential of C–H active rates, methane oxidation of Pd-loaded pure Al2O3 has been measured. Moreover, Lewis acid sites could positively shift the reversible reaction (2Pd−OH ↔ Pd−O* + Pd* + H2O) into the right side, which accelerates Pd–OH species to form water and then transfers into water and surface vacancy. These processes increase the relative abundance of exposed Pd atoms (Pd*) to chemisorb oxygen molecules forming activated oxygen atoms (O*) and methane forming activated CH4 (CH4*).
Pd(NO3)2·2H2O (15% as Pd) was purchased from Shanghai Jiuling Chemical CO., Ltd. Modified Al2O3 with 5 wt% Mg, 5 wt% Si, 20 wt% Ce, 20 wt% Zr, and 40 wt% Si were supported by Sasol and calcined at 600°C for 5 h. The sample names are PURAL Mg 5, SIRAL 5, PURALOX SCFa-160/Ce20, PURALOX SCFa-190 Zr20, and SIRAL 40 HPV. Al2O3 was supported by Aluminum Corporation of China and calcined at 600°C for 5 h.
200 mg of Pd(NO3)2 solution (15 wt % as Pd) is added into 5 ml distilled water (solution A). Solution A is mixed with 2.97 g of support to create a viscous slurry, which is then evaporated to near-dryness on a hot plate (while being constantly stirred) and dried for 12 h at 120°C. The final products are ground to a particle size below 100 μm and calcined in air at 550°C for 5 h using a heating ramp of 5°C/min. All Pd-loaded samples are named as 5Mg, 5Si, 20Ce, 20Zr, 40Si, and SDLY.
As comparison samples, pure Al2O3, purchased from Shanghai Aladdin Biochemical Technology Co., Ltd., was used. In addition, 1 wt% Pd was loaded on it as per the abovementioned method. Then 0.1 wt% K element (K2CO3) was impregnated on the Pd/Al2O3. The unloading K sample (named 0K) and loading sample (named 0.1K) were calcinated in air at 550°C for 5 h using a heating ramp of 5°C/min.
A pulsed CO chemical sorption technique, which is modified from the method used by Jeong et al. (2020), is applied to determine the dispersion of palladium in the catalyst. The sample was exposed to gas flows in the following sequence: 100 mg of catalyst is heated in a 5% H2 flow (30 ml/min, Ar is balanced gas.) to 200°C. After cooling to 50°C, 10% CO/He is pulsed until CO chemisorption onto the catalyst is saturated. CO might be adsorbed on the ceria or zirconium surface, creating carbonates, and causing the dispersion to be exaggerated. The order in which the sample is exposed to gas flows is as follows: 100 mg of catalyst is heated to 200°C in a 5% H2/Ar flow with 30 ml/min. After cooling to 50°C, the sample is exposed to the following gas flows: 1) Ar (5 min); 2) CO2 (30 min); 3) He (60 min); and 4) 10% CO/He. Then, the mixture is pulsed until CO chemisorption onto the catalyst is saturated.
The electronic properties of Pd are studied by XPS (Al K-Alpha, Thermo Scientific, ESCALAB 250Xi). The maximum intensity of the advantageous C 1s signal at 284.8 eV is used as the reference to estimate the binding energies of the Pd element.
To get the NH3-TPD experiment data, 100 mg sample is heated in He flow with 30 ml/min at 200°C and extracted ab/adsorption gases and then cooled to 50°C. The sample is placed under NH3 atmosphere for 90 min, and then desorption at the range of 50–600°C in He flow (30 ml/min) with a ramp rate of 10°C/min. The transmission electron microscopy (TEM) apparatus JEM-ARM200F (JEOL) is used to obtain pictures of materials at an accelerating voltage of 200 kV. A small number of samples is dispersed in ethanol evenly. Then, the solution is dropped on a carbon-coated Cu grid and dried for characterization.
The performance of the catalysts for methane oxidation was carried out in a continuous micro-reactor with an internal diameter of 13 mm at 0.1 atm. In brief,100 mg sieved catalyst grains (40–60 mesh) diluted with 400 mg quartz sand (40–60 mesh) were loaded into the reactor. The catalyst bed was held between two layers of quartz wool located in the middle of the reactor. The reactor was heated using a ceramic tubular furnace, and the temperature of the catalyst bed was controlled and monitored using a K-type thermocouple put within the reactor and close to the catalytic bed. The blank experiment loaded with quartz or catalyst supports without loading active metals was tested at different temperatures. No discernible CH4 conversions were observed at 300°C below.
The composition of the reactant mixture was adjusted by controlling the flow rates of CH4, O2, and N2, using BROOK® MFC while keeping the total flow rate at a constant of 1,000 ml/min. The conditions were consistent with a gas hourly space velocity of 600,000 ml gcat.−1 h−1. The composition of the effluent gases was analyzed by an online Fourier-transform infrared (FT-IR) gas analyzer (MKS, MultiGas™ analyzer, model 6030) equipped with a liquid-nitrogen cooled mercury-cadmium-telluride (MCT) detector installed with a ZnSe window. The detector size is 0.25 mm, and the path length is 5.11 m. The wavelength cutoff is 16 μm.
In this study, employing modified Al2O3 loaded with 1 wt% Pd, we investigated CH4 conversion on the different catalysts in the temperature range of 200–500°C at GHSV of 600,000 ml gcat.−1 h−1with with a gas composition of 0.1% CH4 and 10% O2 balanced by N2, as shown in Figure 1. Keeping at the same level of Pd loading on the Pd/PURALOX SCFa-190 Zr20 (20Zr), Pd/PURALOX SCFa-160/Ce20 (20Ce), Pd/PURAL MG 5 (5Mg), Pd/SIRAL 5 (5Si), Pd/SIRAL 40 HPV (40Si), and Pd/Al2O3 (SDLY) catalysts, the derived catalysts showed very different activities, indicating that the support materials have a significant influence on the catalytic activity. The dependence of activity on the reaction temperature showed that the CH4 c conversion increased with increasing temperature, especially in the range above 350°C, but exhibited as two types of trendlines: 5Si and 40Si and SDLY showed “S” type trendlines; while 5Mg, 20Ce and 20Zr showed continuous increasing trendlines until 500°C. Among these catalysts, 5Si exhibited the best catalytic activity, whose temperatures at 10%, 50%, and 90% conversion (T10, T50, and T90) are 322, 350, and 412°C. 20Ce, as the most inactive catalyst, reaches 10% conversion of CH4 at 411°C (T10), 29% even at 500°C. In the temperature window of 325–400°C, the catalyst activity decreased in the sequence of 5Si > 40Si > SDLY > 20Zr > 5Mg > 20Ce which directly affects the reaction rate of methane combustion, on methane combustion.
FIGURE 1. CH4 combustion over a series of Pd (1 wt%) catalysts at temperatures from 200 to 500°C; Reaction conditions: 0.1% CH4, 10% O2, N2 balance, GHSV = 600,000 ml gcat.−1 h−1.
A typical Arrhenius plot from which activation energies and pre-exponential factors were calculated is shown in Figure 2A. In comparison with other catalysts, the kinetic rate data on the 5Si catalyst is significantly higher than that of others, i.e., the reaction rate on the 5Si catalyst is about 20 times higher than that on 20Ce at 320°C. The apparent activation energy for each catalyst ranges from 63 to 129 kJ mol−1. In order to further explore the influence of the carrier effects on the intrinsic reaction rate, the TOF of a single active site as a function of the reciprocal temperature is shown in Figure 2B and Table 1. Notably, 20Zr and SDLY prepared at the same Pd loadings (1 wt%) showed very different pre-exponential factors (Ln(A)) when normalized by the number of exposed Pd atoms (Figure 2B and Table 1) and exhibited similar activation energies (105–108 kJ mol−1). Furthermore, the rates of 5Si are 4.5 times higher than that of 5Mg catalysts that are both exhibited the lowest activation energies (63–72 kJ mol−1). The data presented here demonstrate that supports play an important role in influencing the reaction kinetics, especially pre-exponential factors.
FIGURE 2. (A) Kinetic rate data for CH4 combustion on a series of Pd (1 wt%) catalysts. (B) Turnover frequency plot of different catalysts for the CH4 combustion.
Chin et al. (2016) and Fujimoto et al. (1998) concluded that the initial H-abstraction of methane on unsaturated Pd2+ sites (Pd*) on the surface of PdO particles is the rate-limiting step depending on the apparent activation energies of catalysts. The thermodynamic and kinetic barriers for the elementary steps for methane catalytic oxidation sequences depend on thermodynamic and kinetic Pd*−PdO interconversions. Monteiro et al. (2001b) studied the combustion of methane in excess oxygen over Pd and confirmed that the turnover rate and reaction orders are consistent with the equation r = k[CH4]0.7[O2]0.2[H2O]−0.9. In this study, [O2]/[CH4] is 100, and it is reasonable to assume that [O2]0.2 is a constant, which means that the chemical equilibrium of oxygen is achieved during the conversion of Pd into PdO, irrespective of the amount of methane. Because of the thermodynamical barrier with CH4 under a lower temperature (<350°C), the reversible reaction equilibrium of Pd→PdO shifts to the right, resulting in retardation of the rate of PdO decomposition and the nucleation of Pd* assemblies on the PdO surface continuously. Such thermodynamic barriers are overcome, which is either limited by temperature or influenced by metal-support interactions, as carrier effects tune chemical states of the surficial Pd (Pd*) on nanoparticles affecting C–H bonds activation rates in an energetically favorable path.
The intrinsic endowment of Pd can be qualitative and quantified through binding energy (BE) of Pd 3d, determining the thermodynamics of Pd*−PdO interconversions in the reaction system (Chin et al., 2016; Chen et al., 2021). Price et al. (2016) reported that BE of Pd 3d on supported Pd catalysts will not change, especially the peaks values, either in an excess oxygen atmosphere or in an ultra-high vacuum without CH4 (Yang et al., 2022b), meaning the rationality of constructing the relationship between BE of Pd 3d in ultra-high vacuum and activation energies (Ea), as shown in Supplementary Figure S1. First, modified Al2O3 with different species or amounts of metallic oxide leads to the change of PdO nanoparticle size and BE of Pd 3d on supports, as shown in Table 1. The Pd dispersion decreased in the sequence of SDLY ≈ 5Si ≈ 5Mg > 40Si > 20Zr > 20Ce, and consequently the PdO nanoparticle size decreased in the order of 20Ce > 20Zr > 40Si > 5Mg ≈ 5Si ≈ SDLY. It is of note that smaller PdO nanoparticles of 5Mg (5.3 nm) have a lower C–H activation rate than 40Si with larger PdO nanoparticles (13.3 nm), which is contrary to the size effects that the associated thermodynamic tendency for Pd*−PdO interconversions (turnover frequency, TOF) is lower for big Pd clusters than for smaller size structures. It is possible that Chin et al. (2016) proposed the theory that C–H activation rates are kinetically prohibitive with CH4 if the temperature is 700°C. Such thermodynamic restrictions of C–H bond activation under 350°C cannot follow the law of size effects. In this study, we analyzed the binding energy of Pd on different supports. It is observed that 5Si and 5Mg, having the lowest Ea in Figure 3B, showed prominent peaks at 337.3 eV. The prominent binding energy peaks, as shown in Supplementary Table S1, are either higher or lower than 337.3 eV, corresponding to the increased Ea, which is a coincidence with that Pd4+ (Wang et al., 2010), like Pd on 20Ce with 338.3 eV, and Pd0 (Xiong et al., 2018; Chen et al., 2020b), like Pd on SDLY with 336.7 eV. They show inactive or lower activity for CH4 oxidation.
FIGURE 3. (A) Pd 3d XPS spectra on various supports. (B) Relationship between binding energy (BE) of Pd 3d5/2 and activation energy (Ea).
As Chin et al. (2016) and Fujimoto et al. (1998) proposed, in the sequence of elementary steps, unsaturated Pd sites (Pd*) and neighboring Pd–O surface species jointly and simultaneously accomplish the cleavage of C–H. Their studies show that the presence of oxygen vacancies on PdO surfaces chemisorb CH4 (CH4*) and H atoms are successively extracted from nearby Pd–O to create hydroxyl groups (Pd–OH). The recombination of surface hydroxyl groups created during C–H bond activation regenerates these vacancies in the catalytic cycle. However, the correlations between the binding energy of Pd 3d and the thermodynamics and kinetics of the Pd*−PdO interconversion remain controversial. Here we propose a mechanism to rationalize the above observation. The CH4 might dissociatively adsorb on metallic Pd and Pd–O sites, as shown in Scheme 1, the coordination of unsaturated Pd sites with positive charges (Pdδ+) may capture gas phase or physisorbed methane to form a four-center [H3C-Pd2+-H-O2−] transition states, consequently dissociate to [H3C-Pd2+] and Pd–OH]. On the catalyst with a binding energy of Pd 3d5/2 lower than 337.3 eV, the coordination unsaturated Pd sites (Pdδ+) have weak abstraction power to electron. As a result, it is hard to activate CH4 molecular and form four-center [H3C-Pd2+-H-O2−] transition states. When the binding energy of Pd 3d5/2 on the catalyst is above 337.3 eV (Pdδ+++), although the coordination of unsaturated Pd sites (Pdδ+++) has a strong capability to capture CH4 molecular, the four-center [H3C-Pd2+-H-O2−] transition states cannot be easily reached because of the higher barrier of formation of Pd–O–H. When the binding energy of Pd 3d5/2 on the catalysts is around 337.3 eV (Pdδ++), H atoms on dissociative absorbed CH4 (CH4*) can be abstracted immediately by neighboring Pd–O, because of the formation of the lowest activation barrier four-center [H3C-Pd2+-H-O2−]. Finally, the thermodynamics of Pd*−PdO interconversions can be tuned by the chemical states of Pd via interactions between Pd and modified Al2O3, as shown in Figure 3A, and the imperfect coordination of Pd2+ ions and nearby oxygen atoms has better activity in shattering the C−H bond of CH4, resulting in reduced methane oxidation Ea when the binding energy of Pd 3d5/2 is 337.3 eV (Pdδ++).
The acidity of catalysts is confirmed from coincided profiles of CH4 combustion pre-exponential factor of rate coefficient, when the Ea of catalysts is similar, the pre-exponential factors increase with the acid content of the catalyst. Parravano et al. (1948) reported that the exchange of C–H bonds occurs freely in a methane molecule in contact with silica–alumina catalyst at 345°C far below the cracking temperatures (700–1,000°C). But few researchers reported that modified Al2O3 without loading the active phase can degrade methane or produce another molecule (like C2H6). The contradiction can be ascribed that modified Al2O3 supports are not able to activate O2 in the system as O* species, and the C–C bond formation need a much higher temperature (Parravano et al., 1948). These reveal that the reaction of CH4 ↔ CH3* + H* on Al2O3 supports without an active phase is reversible. Haldeman and Emmett (1956) studied that most of the active sites of silica–alumina are Lewis acids rather than Bronsted acids for the exchange of H2O at a higher temperature, indicating that Lewis acids could be good for forming OH groups. To evaluate the amount of Lewis acid sites, NH3-TPD were chosen to measure the medium acid sites at 100–400°C (Lewis acid sites) (Gao et al., 2021), as shown in Supplementary Figure S1.
To understand the effect of Lewis acids of catalysts on pre-exponential of C–H active rates, methane oxidation of Pd-loaded pure Al2O3 has been tested at the temperature range of 200–500°C as a function of the presence or absence of K2CO3 as shown in Figure 4. The samples (Figure 4) with alkali metal ions presence of 0 wt%, 0.1 wt%, and 0.5 wt% are named 0K, 0.1K, and 0.5K. As shown in Figure 4A, the catalytic activity of supported Pd (1 wt%) as a function of reaction temperature strongly depends on the support materials, in the range of 200–500°C. The catalytic activity with respect to methane conversion decreases with increasing K ions loading. Among these catalysts, 0K catalyst exhibited the best catalytic activity, corresponding to the T10, T50, and T90, at 318, 350, and 499°C, respectively. Compared with 0K, the SDLY catalyst (as shown in Figure 1), the T10, T50, and T90, are 333, 367, and 471°C, respectively, which showed a similar performance with that of 0K catalyst. The kinetic rate measurements indicate the 0K catalyst’s strong intrinsic activity when compared to the reference catalyst (Figure 4B). For each of the catalysts, the apparent activation energies are also similar (101–104 kJ mol−1) and so close to the Ea of 20Zr (105 kJ mol−1), in which the coincidence that the BEs of Pd 3d5/2 on 0K, 0.1K, 0.5K, and 20Zr are all same (Table 1 and Figure 5E) fitting for the curve in Figure 3B, further proved that BEs of Pd 3d5/2 on catalysts determine the Ea of methane combustion in an excess oxygen atmosphere.
FIGURE 4. (A) CH4 combustion over a series of Pd (1 wt%) catalysts at temperatures from 200 to 500°C; Reaction conditions: 0.1% CH4, 10% O2, N2 balance, GHSV = 600,000 ml gcat.−1 h−1. (B) Kinetic rate data for CH4 combustion on a series of Pd (1 wt%) catalysts. 0K means that 0 wt% K was loaded on catalysts (same as 0.1K and 0.5K).
FIGURE 5. High-angle annular dark field (HAADF) micrographs of 0K (A) and 0.5K (B) catalysts; (E) PdO nanoparticles size distributions of 0K (C) and 0.5K (D). (E) Pd 3d XPS spectra of 0K, 0.1K, and 0.5K.
The higher negative Gibbs free energy for PdO production, in which the transition of Pd↔PdO* changes O*−O* adatom sites into Pd2+−O2− ion pairs, the smaller Pd cluster. More effective Pd2+−O2− ion pairs for the kinetically significant C−H bond activation in CH4 (Chin et al., 2016). To study the influence of PdO nanoparticle size effects and dispersion on pre-exponential factors, PdO nanoparticle size distributions in 0K (Figures 5A and C) and 0.5K (Figures 5B and D) are measured, which correspond to the mean diameter of 5.8 and 5.1 nm. The 0 and 0.1K catalysts shown in Figure 5 have similar nanoparticle sizes and dispersions, indicating that 0K, 0.1K, and 0.5K have similar size effects and dispersions. Moreover, the similar specific surface area (131, 133, and 144 m2 g−1), as shown in Table 1, indicates indifferent heat and mass transfer rates because introduced K did not change the pore structure of supports. In the kinetics rate plot in Figure 4B, it is found that the pre-exponential factors A of the model catalysts (0K, 0.1K, and 0.5K) are variable as the Lewis acid contents on the catalysts are different. The pre-exponential factors increase with the increase of Lewis acid contents. In other words, the Lewis acid on the catalysts can promote the C–H activation rates by affecting the pre-exponential factor.
The interplay between carbon atoms in the gaseous or adsorbed CH4 and coordinatively unsaturated Pd sites (Pd*) on the surface of PdO particles form absorbed CH4 (CH4*), according to suggested chemical pathways for the oxidation of methane on PdO (Fujimoto et al., 1998; Chin et al., 2016). Al2O3, as supports, cannot catalyze the oxidation of methane without active phases. Then the forming water molecular due to quasi-equilibrated condensation of Pd–OH species, desorption, and regenerate surface vacancies (Pd*) required for methane activation (Fujimoto et al., 1998; M. 1972). The reversible step (2Pd−OH ↔ Pd−O* + Pd* + H2O) determines the abundance of exposed Pd* and Pd−O*, the H-abstraction step will be blocked kinetically when Pd−OH is the most abundant surface species. Haldeman and Emmett (1956) found that hydrogen diffuses as H2O (OH groups or H+) through the Lewis acids on the support, indicating Lewis acid sites are good for the exchange of H2O. By assuming the inclusion of a Lewis acid site in the reaction sequence, researchers suggested the presence of Lewis acid sites near the PdO nanoparticles; these acid sites increase the abundance of exposed Pd* and Pd−O*, which induce the transfer of surface hydroxyl groups on Pd−OH to Lewis acid sites or condensation of Pd–OH and Al–OH to form water (Scheme 2). In the sequence of elementary steps of this scheme, surface vacancies (Pd*) are regenerated by the migration of hydroxyl from Pd–OH to Al because the exchange of hydroxyl between water and alumina can be measured at 100°C (Mills and Hindin, 1950), indicating the possibility of surface migration of hydroxyl between Pd–OH and Al. Parker et al. (2010) characterized the hydrous palladium oxide and found that the length of the Pd−O bond in hydrous PdO is the same as in anhydrous PdO, meaning the weakness of O−H bonds in Pd−OH and differences with OH−, so that O−H bonds in the Pd−OH can be easily broken. Thus, surface oxygen species (Pd–O*) can be regenerated by condensation of Pd–OH and Al–OH species to form water because of the hydrogen bond. When the amount of Pd−OH species reaches a certain constant, the quasi-equilibrated step (2Pd−OH ↔ Pd−O* + Pd* + H2O) results in the active sites (Pd* and Pd−O*) being continuously occupied. Al–OH/Al sites promote the positive shift in the quasi-equilibrated step so that pre-exponential factors are improved.
The carrier effects on palladium oxide for methane combustion were studied in this work. The effects on activation energy were adjusted through tuning Pd 3d binding energies, and pre-exponential factors (A) were tuned by Pd dispersion and acidity on supports. First, the modification of Al2O3 by different metallic oxides can affect the interaction between metal and support, which results in a significant change in Pd 3d binding energies. The coordination of unsaturated Pd2+ ions (Pd*) and nearby oxygen atoms synergetically plays as the active sites for dissociation of the C−H bond of CH4=. When the binding energy of Pd 3d5/2 is at about 337.3 eV, the catalyst shows the lowest activation energy (Ea); while increased or decreased binding energies of Pd 3d on the Pd supported different metallic oxide modified Al2O3 support. Finally, the shifts of the reversible elementary step (2Pd−OH ↔ Pd−O* + Pd* + H2O) depend on the density of acid sites on catalysts. The abundance of exposed Pd* and Pd−O* (improving the pre-exponential factors) appears to increase with the increase of acid strength.
The original contributions presented in the study are included in the article/Supplementary Material; further inquiries can be directed to the corresponding authors.
SP: methodology, data curation, and writing—original draft. ZM, JM, and HYW: formal analysis and validation. JC and HW: XPS and TPD testing and management. YL: grammar checking and editing. ZA and BW: writing—review and editing validation.
This work was supported by the Science and Technology Project of China Energy Investment, which is the development and engineering demonstration of key materials for VOCs oxidation catalysis in coal chemical industry (ST930021005C).
The authors declare that the research was conducted in the absence of any commercial or financial relationships that could be construed as a potential conflict of interest.
The handling editor TZ declared a past co-authorship with the author BW.
All claims expressed in this article are solely those of the authors and do not necessarily represent those of their affiliated organizations, or those of the publisher, the editors, and the reviewers. Any product that may be evaluated in this article, or claim that may be made by its manufacturer, is not guaranteed or endorsed by the publisher.
The Supplementary Material for this article can be found online at: https://www.frontiersin.org/articles/10.3389/fchem.2022.978698/full#supplementary-material
Alden, C. B., Wright, R. J., Coburn, S. C., Caputi, D., Wendland, G., Rybchuk, A., et al. (2020). Temporal variability of emissions revealed by continuous, long-term monitoring of an underground natural gas storage facility. Environ. Sci. Technol. 54, 14589–14597. doi:10.1021/acs.est.0c03175
Anthony, D. B. (2008). Megafauna biomass tradeoff as a driver of quaternary and future extinctions. Proc. Natl. Acad. Sci. U. S. A. 105, 11543–11548. doi:10.1073/pnas.0801918105
Campbell, C. T. (2012). Catalyst-support interactions: Electronic perturbations. Nat. Chem. 4, 597–598. doi:10.1038/nchem.1412
Cao, Y., Ran, R., Wu, X. D., Si, Z. C., Kang, F. Y., Weng, D., et al. (2023). Progress on metal-support interactions in Pd-based catalysts for automobile emission control. J. Environ. Sci. 125, 401–426. doi:10.1016/j.jes.2022.01.011
Cardoso-Saldana, F. J., and Allen, D. T. (2020). Projecting the temporal evolution of methane emissions from oil and gas production sites. Environ. Sci. Technol. 54, 14172–14181. doi:10.1021/acs.est.0c03049
Cargnello, M., Jaen, J. J. D., Garrido, J. C. H., Bakhmutsky, K., Montini, T., Gamez, J. J. C., et al. (2012). Exceptional activity for methane combustion over modular Pd@CeO2 subunits on functionalized Al2O3. Science 337, 713–717. doi:10.1126/science.1222887
Chen, B., Lin, J., Chen, X., Zheng, Y., Zhang, H. W., Huang, F., et al. (2020a). Controllable synthesis of mesoporous alumina as support for palladium catalysts and reconstruction of active sites during methane combustion. Int. J. Hydrogen Energy 45, 15142–15156. doi:10.1016/j.ijhydene.2020.03.233
Chen, J., Wu, Y., Hu, W., Qu, P. F., Liu, X., Yuan, R., et al. (2020b). Insights into the role of Pt on Pd catalyst stabilized by magnesia-alumina spinel on gama-alumina for lean methane combustion: Enhancement of hydrothermal stability. Mol. Catal. 496, 111185. doi:10.1016/j.mcat.2020.111185
Chen, S., Li, S., You, R., Guo, Z., Wang, F., Li, G., et al. (2021). Elucidation of active sites for CH4 catalytic oxidation over Pd/CeO2 via tailoring metal-support interactions. ACS Catal. 11, 5666–5677. doi:10.1021/acscatal.1c00839
Chin, Y, H., García-Diéguez, M., and Iglesia, E. (2016). Dynamics and thermodynamics of Pd–PdO phase transitions: Effects of Pd cluster size and kinetic implications for catalytic methane combustion. J. Phys. Chem. C 120, 1446–1460. doi:10.1021/acs.jpcc.5b06677
Chu, P., Wang, S., Zhang, Y., Zhao, S., Wang, Y., Deng, J., et al. (2022). Component regulation in novel La-Co-O-C composite catalyst for boosted redox reactions and enhanced thermal stability in methane combustion. J. Environ. Sci. 126, 459–469. doi:10.1016/j.jes.2022.04.002
Ding, Y., Wu, Q., Lin, B., Guo, Y. L., Guo, Y., Wang, Y. S., et al. (2020). Superior catalytic activity of a Pd catalyst in methane combustion by fine-tuning the phase of ceria-zirconia support. Appl. Catal. B Environ. 266, 118631. doi:10.1016/j.apcatb.2020.118631
Fujimoto, K., Ribeiro, F. H., Avalos-Borja, M., and Iglesia, E. (1998). Structure and reactivity of PdOx/ZrO2 catalysts for methane oxidation at low temperatures. J. Catal. 179, 431–442. doi:10.1006/jcat.1998.2178
Gao, D., Zhang, C., Wang, S., Yuan, Z. S., and Wang, S. D. (2008). Catalytic activity of Pd/Al2O3 toward the combustion of methane. Catal. Commun. 9, 2583–2587. doi:10.1016/j.catcom.2008.07.014
Gao, M., Gong, Z., Weng, X., Shang, W. X., Chai, Y. C., Dai, W. L., et al. (2021). Methane combustion over palladium catalyst within the confined space of MFI zeolite. Chin. J. Catal. 42, 1689–1699. doi:10.1016/s1872-2067(20)63775-5
Haldeman, R. G., and Emmett, P. H. (1956). A study of the water content of A silica-alumina catalyst and its component oxides. J. Am. Chem. Soc. 78, 2917–2922. doi:10.1021/ja01594a001
He, C., Cheng, J., Zhang, X., Douthwaite, M., Pattisson, S., Hao, Z. P., et al. (2019). Recent advances in the catalytic oxidation of volatile organic compounds: A review based on pollutant sorts and sources. Chem. Rev. 119, 4471–4568. doi:10.1021/acs.chemrev.8b00408
Jang, E. J., Lee, J., Oh, D. G., and Kwak, J. H. (2021). CH4 oxidation activity in Pd and Pt-Pd bimetallic catalysts: Correlation with surface PdOx quantified from the drifts study. ACS Catal. 11, 5894–5905. doi:10.1021/acscatal.1c00156
Jeong, H., Kwon, O., Kim, B. S., Bae, J., Shin, S., Kim, H. E., et al. (2020). Highly durable metal ensemble catalysts with full dispersion for automotive applications beyond single-atom catalysts. Nat. Catal. 3 (4), 368–375. doi:10.1038/s41929-020-0427-z
Karl, S., Stephen, J. P., and Sokrates, T. P. (1999). Hydrogen and the structure of the transition aluminas. J. Am. Chem. Soc. 121, 7493–7499. doi:10.1021/ja991098o
Khan, H. A., Hao, J. Y., and Farooq, A. (2020). Catalytic performance of Pd catalyst supported on Zr:Ce modified mesoporous silica for methane oxidation. Chem. Eng. J. 397, 125489. doi:10.1016/j.cej.2020.125489
Lei, J., Niu, R., Wang, S., and Li, J. P. (2020). The Pd/Na-ZSM-5 catalysts with different Si/Al ratios on low concentration methane oxidation. Solid State Sci. 101, 106097. doi:10.1016/j.solidstatesciences.2019.106097
Li, C., Li, W., Chen, K., Ogunbiyi, A. T., Zhou, Z., Xue, F. Y., et al. (2020). Palladium nanoparticles supported on surface-modified metal oxides for catalytic oxidation of lean methane. ACS Appl. Nano Mat. 3 (12), 12130–12138. doi:10.1021/acsanm.0c02614
Lim, J. B., Jo, D., and Hong, S. B. (2017a). Palladium-exchanged small-pore zeolites with different cage systems as methane combustion catalysts. Appl. Catal. B Environ. 219, 155–162. doi:10.1016/j.apcatb.2017.07.032
Lim, T. H., Park, S. B., Kim, J. M., and Kim, D. H. (2017b). Ordered mesoporous MCo2O4 (m = Cu, Zn and Ni) spinel catalysts with high catalytic performance for methane combustion. J. Mol. Catal. A Chem. 426, 68–74. doi:10.1016/j.molcata.2016.11.002
Lin, J., Chen, Y. L., Liu, X. J., Chen, X. H., Zheng, Y., Huang, F., et al. (2020). Microstructural property regulation and performance in methane combustion reaction of ordered mesoporous alumina supported palladium-cobalt bimetallic catalysts. Appl. Catal. B Environ. 263, 118269. doi:10.1016/j.apcatb.2019.118269
Liu, Y., Wang, S., Gao, D., Sun, T. J., Zhang, C. X., Wang, S. D., et al. (2013). Influence of metal oxides on the performance of Pd/Al2O3 catalysts for methane combustion under lean-fuel conditions. Fuel Process. Technol. 111, 55–61. doi:10.1016/j.fuproc.2013.01.013
Mills, G. A., and Hindin, S. G. (1950). Chemical characterization of catalysts. II. Oxygen exchange between water and cracking catalysts. J. Am. Chem. Soc. 72, 5549–5554. doi:10.1021/ja01168a051
Monteiro, R. S., Zemlyanov, D., Storey, J. M., and Ribeiro, F. H. (2001a). Surface area increase on Pd foils after oxidation in excess methane. J. Catal. 201, 37–45. doi:10.1006/jcat.2001.3235
Monteiro, R. S., Zemlyanov, D., Storey, J. M., and Ribeiro, F. H. (2001b). Turnover rate and reaction orders for the complete oxidation of methane on A palladium foil in excess dioxygen. J. Catal. 199, 291–301. doi:10.1006/jcat.2001.3176
Parker, S. F., Refson, K., Hannon, A. C., Albers, P., and Robertson, S. J. (2010). Characterization of hydrous palladium oxide: Implications for low-temperature carbon monoxide oxidation. J. Phys. Chem. C 114, 14164–14172. doi:10.1021/jp103847d
Parravano, G., Hammel, E. F., and Taylor, H. S. (1948). The exchange reaction between methane and deuteromethanes on silica—alumina cracking catalysts. J. Am. Chem. Soc. 70, 2269–2270. doi:10.1021/ja01186a083
Price, R., Eralp-Erden, T., Crumlin, E., Rani, S., Garcia, S., Smith, R., et al. (2016). The partial oxidation of methane over Pd/Al2O3 catalyst nanoparticles studied in-situ by near ambient-pressure X-ray photoelectron spectroscopy. Top. Catal. 59, 516–525. doi:10.1007/s11244-015-0520-8
Satsuma, A., Tojo, T., Okuda, K., Yamamoto, Y., Arai, S., and Oyama, J. (2015b). Effect of preparation method of Co-promoted Pd/alumina for methane combustion. Catal. Today 242, 308–314. doi:10.1016/j.cattod.2014.05.046
Satsuma, A., Osaki, K., Yanagihara, M., Ohyama, J., and Shimizu, K. (2015a). Low temperature combustion over supported Pd catalysts- strategy for catalyst design. Catal. Today 258, 83–89. doi:10.1016/j.cattod.2015.03.047
Shi, W., Xu, G., Han, X., Wang, Y., Liu, Z., Xue, S., et al. (2022). Nano-sized alumina supported palladium catalysts for methane combustion with excellent thermal stability. J. Environ. Sci. 126, 333–347. doi:10.1016/j.jes.2022.04.030
Singh, S. A., Madras, G., and Sreedhar, I. (2020). Transition metal (Ni, Cu and Fe) substituted Co3O4-ZrO2 catalysts for lean methane combustion. Top. Catal. 64, 243–255. doi:10.1007/s11244-020-01382-0
Wang, Z. C., Wu, X. N., Zhao, Y. X., Ma, J. B., Ding, X. L., and He, S. G. (2010). Room-temperature methane activation by a bimetallic oxide cluster AlVO4. Chem. Phys. Lett. 489, 25–29.
Wang, Z., Jiang, Y., Jin, F., Catherine, S., Michael, H., Alfons, B., et al. (2019). Strongly enhanced acidity and activity of amorphous silica-alumina by formation of pentacoordinated alv species. J. Catal. 372, 1–7. doi:10.1016/j.jcat.2019.02.007
Xiong, H. F., Wiebenga, M. H., Carrillo, C., Gaudet, J. R., Pham, H. N., Kunwar, D., et al. (2018). Design considerations for low-temperature hydrocarbon oxidation reactions on Pd based catalysts. Appl. Catal. B Environ. 236, 436–444. doi:10.1016/j.apcatb.2018.05.049
Xu, S., Jaegers, N. R., Hu, W., Kwak, J. H., Bao, X., Sun, J., et al. (2021). High-field one-dimensional and two-dimensional 27Al magic-angle spinning nuclear magnetic resonance study of theta-delta-and gamma-Al2O3 dominated aluminum oxides: Toward understanding the Al sites in gamma- Al2O3. ACS Omega 6, 4090–4099. doi:10.1021/acsomega.0c06163
Yang, F., Zhong, J., Wang, S., Hu, X., Wang, H., Tang, M., et al. (2022a). Patterns and drivers of CH4 concentration and diffusive flux from a temperate river–reservoir system in north China. J. Environ. Sci. 116, 184–197. doi:10.1016/j.jes.2021.12.004
Yang, Y., Lee, J., Dorakhan, R., Nie, H., Fu, G., Quarantotto, A., et al. (2022b). Active site structure and methane oxidation reactivity of bimetallic Pd and Pt nanoparticles. Appl. Catal. A General 629, 118290. doi:10.1016/j.apcata.2021.118290
Yazawa, Y., Yoshida, H., Takagi, N., Komai, S., Satsuma, A., Hattori, T., et al. (1999). Acid strength of support materials as a factor controlling oxidation state of palladium catalyst for propane combustion. J. Catal. 187, 15–23. doi:10.1006/jcat.1999.2583
Yazawa, Y., Yoshida, H., Takagi, N., Komai, S., Satsuma, A., Murakami, Y., et al. (2000). Acid strength of support materials as a factor controlling catalytic activity of noble metal catalysts for catalytic combustion. Stud. Surf. Sci. Catal. 130, 2189–2194.
Zhan, Y., Kang, L., Zhou, Y., Cai, G. H., Chen, C. Q., Jiang, L. L., et al. (2019). Pd/Al2O3 catalysts modified with Mg for catalytic combustion of methane:effect of Mg/Al mole ratios on the supports and active PdOx formation. J. Fuel Chem. Technol. 47, 1235–1244. doi:10.1016/s1872-5813(19)30050-7
Zhang, G., Chen, J., Wu, Y., Liu, X., Qu, P. F., Shen, P. Q., et al. (2020). Pd supported on alumina modified by phosphate: Highly phosphorus-resistant three-way catalyst for natural gas vehicles. J. Taiwan Inst. Chem. Eng. 115, 108–116. doi:10.1016/j.jtice.2020.09.032
Zhang, L., Chen, J., Guo, X., Yin, S., Zhang, M., Rui, Z., et al. (2021). Combination of reduction-deposition Pd loading and zeolite dealumination as an effective route for promoting methane combustion over Pd/beta. Catal. Today 376, 119–125. doi:10.1016/j.cattod.2020.07.005
Zimmerle, D., Vaughn, T., Luck, B., Lauderdale, T., Keen, K., Harrison, M., et al. (2020). Methane emissions from gathering compressor stations in the US. Environ. Sci. Technol. 54, 7552–7561. doi:10.1021/acs.est.0c00516
Keywords: methane combustion, palladium, alumina, acid sites, carrier effect
Citation: Peng S, Ma Z, Ma J, Wang H, Chen J, Wei H, Li Y, Ao Z and Wang B (2022) Influence of carrier effect on Pd/Al2O3 for methane complete catalytic oxidation. Front. Chem. 10:978698. doi: 10.3389/fchem.2022.978698
Received: 26 June 2022; Accepted: 18 July 2022;
Published: 23 August 2022.
Edited by:
Tao Zhu, China University of Mining and Technology, ChinaReviewed by:
Wenxiang Tang, Sichuan University, ChinaCopyright © 2022 Peng, Ma, Ma, Wang, Chen, Wei, Li, Ao and Wang. This is an open-access article distributed under the terms of the Creative Commons Attribution License (CC BY). The use, distribution or reproduction in other forums is permitted, provided the original author(s) and the copyright owner(s) are credited and that the original publication in this journal is cited, in accordance with accepted academic practice. No use, distribution or reproduction is permitted which does not comply with these terms.
*Correspondence: Zhimin Ao, YW96aGltQGhvdG1haWwuY29t; Baodong Wang, YmFvZG9uZy53YW5nLmRAY2huZW5lcmd5LmNvbS5jbg==
Disclaimer: All claims expressed in this article are solely those of the authors and do not necessarily represent those of their affiliated organizations, or those of the publisher, the editors and the reviewers. Any product that may be evaluated in this article or claim that may be made by its manufacturer is not guaranteed or endorsed by the publisher.
Research integrity at Frontiers
Learn more about the work of our research integrity team to safeguard the quality of each article we publish.