- State Key Laboratory of Organic-Inorganic Composites, College of Chemical Engineering, Beijing University of Chemical Technology, Beijing, China
Photocatalytic nitrogen reduction reaction (NRR) to ammonia holds a great promise for substituting the traditional energy-intensive Haber–Bosch process, which entails sunlight as an inexhaustible resource and water as a hydrogen source under mild conditions. Remarkable progress has been achieved regarding the activation and solar conversion of N2 to NH3 with the rapid development of emerging photocatalysts, but it still suffers from low efficiency. A comprehensive review on photocatalysts covering tungsten and related metals as well as their broad ranges of alloys and compounds is lacking. This article aims to summarize recent advances in this regard, focusing on the strategies to enhance the photocatalytic performance of tungsten and related metal semiconductors for the NRR. The fundamentals of solar-to-NH3 photocatalysis, reaction pathways, and NH3 quantification methods are presented, and the concomitant challenges are also revealed. Finally, we cast insights into the future development of sustainable NH3 production, and highlight some potential directions for further research in this vibrant field.
1 Introduction
As the cornerstone of modern civilization, ammonia (NH3) is an incontrovertible raw material for modern industry and agriculture, which plays a crucial role in human survival and economic growth. Moreover, due to its high hydrogen content (17.7 wt%), gravimetric energy density (3 kWh kg−1) and easy liquification (−33°C under atmospheric pressure), NH3 also serves as a useful commodity for chemicals used in industries and as a carbon-free clean energy carrier (Guo and Chen, 2017). NH3 is by far predominantly fabricated via the energy- and capital-intensive Haber-Bosch process requiring extreme reaction conditions of 300–500°C and 15–25 MPa, which gives rise to excessive consumption of feedstocks and consequently high CO2 emissions. Therefore, exploring and developing renewable, environment-friendly, and green routes to yield NH3 is desirable. Photocatalytic N2 fixation is perceived as an alternative sustainable strategy for facile, cost-effective ammonia production using water and nitrogen gas under ambient conditions. There is enormous, acknowledged and untapped potential in this emerging field. Considering that nitrogen reduction reaction (NRR) coupled with water oxidation is a thermodynamically uphill reaction, solar energy is hence crucial to induce incoming photons generating electronic charge carriers to initiate the catalytic reaction. Since the pioneering research by Schrauzer and co-workers embarked on the photocatalytic reduction of N2 to NH3 by employing TiO2-based photocatalysts (Schrauzer and Guth, 1977), explorative efforts in this sector have been exerted to develop novel photocatalysts with high efficiency, especially in recent years (Schrauzer and Guth, 2002). The initial attempts are currently expanded to widen the scope of investigated materials and their modifications, as well as effective strategies of tuning crystalline phase, surface defects, heteroatom doping, surface modification and/or heterostructure construction for enhancing photocatalytic performances. The photochemical process underpins the terms of selectivity, efficiency, and low operational cost for the production of NH3 toward the practical implementation at relatively ambient conditions using solar energy. Despite the huge potentials, the photocatalytic NH3 production still falls far short of the ideal of being commercialized, which results from weak adsorption/activation of the nonpolar N≡N triple bond of N2 (941 kJ mol−1), inefficient light absorption, and poor photo-induced charge separation (Shen et al., 2021).
Design and development of efficient photocatalysts hold the key to achieve improved performance of photocatalytic NRR. To date, several prior articles (Chen et al., 2018; Shi et al., 2019; Shuai Zhang et al., 2019; Huang et al., 2020; Chen-Xuan Xu et al., 2021; Lee et al., 2021; Shen et al., 2021; Tong Xu et al., 2021) have outlined the recent advancements of photoreduction of N2 to NH3 and photocatalysts engineering strategies. Transition metals, especially the early transition metals (e.g., tungsten, molybdenum, vanadium etc.), which possess both empty orbitals and abundant d-orbital electrons as well as suitable bandgap energies, could activate dinitrogen molecules through σ-donation/π-backdonation effects, showing huge potential for applications as photocatalysts for photocatalytic NRR. However, few reviews, to the best of our knowledge, have hitherto summarized tungsten and related metals photocatalysts together with a specific focus on strategies to enhance their performances. This review elaborated the state-of-the-art understanding of the basic principles of NRR photocatalysis, reaction mechanisms, thermodynamic limits, and enforceable protocols involved in the overall photochemical processes. We comprehensively discuss recent progress over semiconductors containing tungsten (W), molybdenum (Mo), cobalt (Co), vanadium(V), tantalum (Ta), niobium (Nb), rhenium (Re), zirconium (Zr), hafnium (Hf), and their major advantages as to photocatalysis activity. Furthermore, we emphasize several strategies to improve the photocatalytic performance and also highlight the challenges and future directions for sustainable NH3 production.
2 Fundamentals for photochemical NRR
2.1 Properties of N2 molecules
Molecular dinitrogen possesses a triple bond between the nitrogen atoms and a non-bonding pair of electrons on each atom. Atomic nitrogen has 5 valence electrons and 4 valence orbitals (2s, 2px, 2py, and 2pz), whereas hybridization of the s-p atomic orbitals of N2 consists of four bonding orbitals (two σ and two π orbitals) and four antibonding orbitals (two σ* and two π* orbitals). The electrons from the π and 2σ orbitals are shared to form N≡N bond leaving these from 1σ* and 1σ orbitals the non-bonding electron pairs (Figure 1A) (Kitano et al., 2012). Hence, the large energy gap of 10.82 eV between the highest occupied molecular orbital (HOMO) and lowest unoccupied molecular orbital (LUMO) seriously hinders electron injection into N2 antibonding orbitals (Jia and Quadrelli, 2014). A strong N≡N bond energy (945 kJ mol−1) and first-bond breaking energy (410 kJ mol−1) render N2 molecules extremely thermodynamically stable (Gambarotta and Scott, 2004), meanwhile, N2 molecules are chemically inert by virtue of high ionization energy of 15.85 eV and negative electron affinity of −1.9 eV (Figure 1B). Therefore, adsorption and dissociation of N2 with weak polarizability and lacking dipole moment are widely regarded to be the rate-determining steps of NRR (Guo et al., 2018; Liang Yang et al., 2020; Yi-Fei Zhang et al., 2020). Both computational and experimental works have demonstrated that transition metal-based materials interact strongly with N2 through the formation of N–metal bonds, thanks to the empty d orbitals in the transition metals (TMs) accepting the lone pair electrons of N2 and back donating d-p electrons into the anti-binding orbitals of N2 based on an “acceptance-donation” protocol, whereby the triple bond can be weakened and activated to facilitate the bond dissociation (Figure 1C). Some advances have demonstrated the incomparable advantages of d block compounds, but this realm remains elusive.
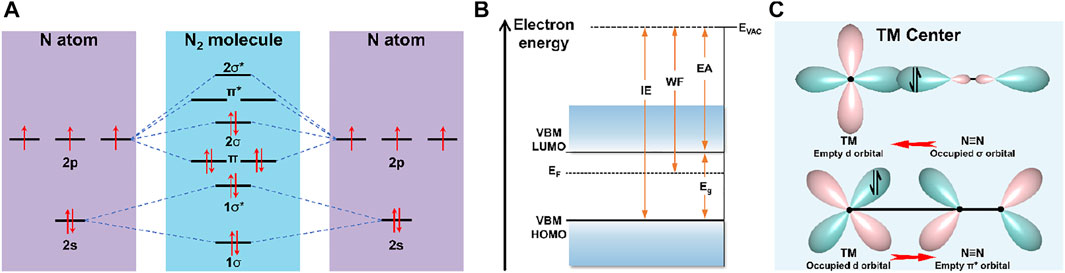
FIGURE 1. (A) Diagram of N atomic orbitals and N2 molecular orbitals. (B) Energy diagram for N2. EVAC, Eg, WF, IE, EA, and EF represent vacuum level, energy gap, work function, ionization energy, electron affinity, and Fermi level, respectively. (C) Schematic illustration of N2 bonded to TMs.
2.2 Principles and mechanisms of photochemical NRR to NH3
Ideally, photocatalytic NRR into NH3 involves transfer of 6 protons and 6electrons, which is a strong endothermic reaction with stoichiometric O2 production formed by water oxidation (overall reaction: N2 (g) + 3H2O (l) ↔ 2NH3 (g) + 3/2O2 (g), ∆G = +7.03 eV) (Medford and Hatzell, 2017). The photochemical NRR requires a potential of at least 1.17 eV per electron (Comer and Medford, 2018), which is initiated by the absorption of photons with an energy higher than the energy bandgap (Eg) of a semiconductor photocatalyst to generate photoexcited electron-hole pairs and an energy >1.17 eV demanded for the overall NH3 production (Figure 2A). Upon photo-irradiation with sufficient energy supply, electrons (e−) leap into the conduction band (CB) with simultaneous generation of holes (h+) at valence band (VB), thus triggering the reduction of N2 to NH3 with photo-excited electrons and water oxidation with created holes (Linsebigler et al., 1995). The photoinduced separation of charge carriers is the prerequisite for all semiconductor photocatalysis. However, the migration and separation of photoexcited electron-hole pairs to the semiconductor surface active sites to participate in the redox reactions competes with their recombination in the bulk and on the surface. Eventually, the reaction products desorb from the photocatalyst surface and are transferred to the medium to close the cycle (Figure 2B). Note that the NRR is an uphill reaction. As a consequence, the CB and VB positions of a photocatalyst must bestride the reduction potential of N2 and the oxidation potential of H2O. Theoretically, the bottom of the CB should be more negative than the reduction potential of N2/N2H (−3.2 V vs. normal hydrogen electrode, NHE), while the top of VB must be beyond the oxidation potential of H2O/O2 (+1.23 V vs. NHE). Satisfying both of the above requirements with a singular conventional semiconductor seems to be incompatible, which is unfavorable for harvesting most of the light across the solar spectrum. Since a considerable number of electron-hole pairs recombine inside or on the surface of the catalyst with a rather fast kinetics rate (Zhang et al., 2012), or dissipate in the form of heat or light energy, thus resulting in a decrease of reaction efficiency. Accordingly, most of the reported catalysts for photocatalytic NH3 production still suffer from low light utilization, fast recombination of photoexcited electron-hole pairs, poor N2 adsorption/activation, and sluggish electron-to-N2 transfer kinetics, hobbling the overall solar-to-ammonia conversion efficiency. Additionally, the insufficient stability of the photocatalysts is another serious issue. Many semiconductors could undergo photo-corrosion upon light irradiation, which can be induced by the photo-generated electrons or holes, thus leading to degradation of NRR performance and inhibiting the long-time photocatalytic ammonia synthesis.
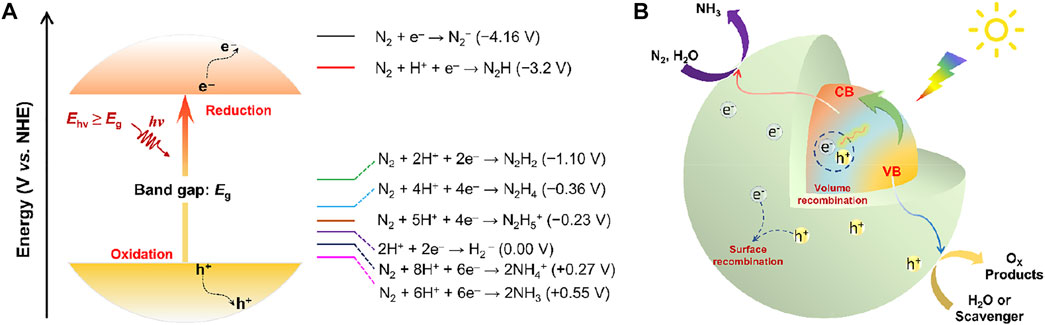
FIGURE 2. (A) Schematic energy diagram related to the reduction of N2 to NH3. (B) Schematic illustration of the complete photocatalytic NH3 synthesis over semiconductor-based photocatalysts.
The current well-established mechanisms for photocatalytic NRR can be roughly divided into the following: dissociative, associative or enzymatic pathways as shown in Figure 3 (van der Ham et al., 2014; Hao Li et al., 2016; Shipman and Symes, 2017). For the dissociative pathway, the breaking of the N≡N bond precedes the hydrogenation process, followed by the stepwise protonation of the adsorbed nitrogen atoms to form NH3, analogous to the reaction mechanism of the industrial Haber-Bosch process (Garden and Skulason, 2015), which is however unfavorable for N2 photoreduction to NH3 resulting from the large external energy input required for the cleavage of the N≡N triple bond (Shipman and Symes, 2017). According to the different hydrogenation sequences, the associative N2 reduction mechanism follows either distal pathway or alternating pathway. In the distal associative pathway, the nitrogen atom far from the adsorption site is protonated successively before generating the first NH3 molecule, leaving another N atom to yield the second NH3 molecule. Conversely, in the alternate pathway, the hydrogenation reaction occurs alternately at two nitrogen atoms, each of which could react with injected electrons and protons, forming key intermediates such as metal-bound diazene (HN = NH) and reaction byproducts such as N2H4 (Bo et al., 2021). As for the enzymatic pathway, the 2 N atoms of the nitrogen molecule are simultaneously adsorbed by the active center of the catalyst, anchored on the catalyst surface via the “side-on” configuration, and then hydrogenated (Hinnemann and Norskov, 2006; Xiao-Fei Li et al., 2016). The proposal that gained the widest support was that photocatalytic NH3 synthesis follows associative pathways, during which the adsorption and activation of N2 and then the transfer of photogenerated electrons from the photocatalyst to N2 provide a lower reaction energy barrier for the dissociation of N≡N triple bonds (Montoya et al., 2015). The activation of N2 involves the formation of a coordinate bond with the active site proceeded on a catalytic surface, and the subsequent electron transfer and protonation are the keys to weakening the N≡N bonding energy (Jacobsen et al., 2001). Different mechanisms have been proposed, but deep mechanistic understanding of NRR that may vary for distinct catalytic systems remains to be further explored.
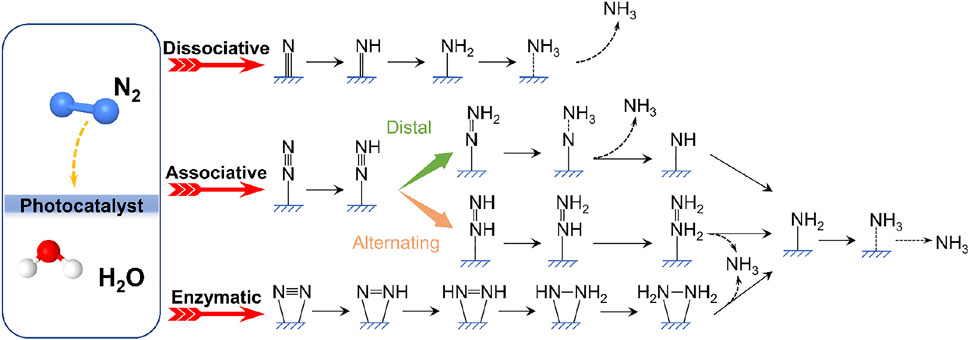
FIGURE 3. Schematic representation of possible pathways for N2 fixation to NH3 on catalyst surfaces.
2.3 Thermodynamic limits of photocatalytic NRR to NH3
Due to the proton-electron transfer of multiple intermediates resulting in sluggish reaction kinetics, the thermodynamic constraints relying on the reaction intermediates and the overall and half-reaction thermodynamics independent of the photocatalysts play the decisive role in the photocatalytic NRR process (Table 1). Despite the overall reaction of NH3 production via N2 reduction in water (N2 (g) + 6H+ + 6e− ↔ 2NH3 (g), E0 = +0.55 V vs. NHE) is more thermodynamically feasible than the competing hydrogen evolution reaction (HER) by water reduction, HER is more likely to occur with fewer electrons, decreasing the selectivity toward NH3. Besides, the more negative potentials for the intermediate reactions, such as −4.2 V (vs. RHE) for one-electron activation to form surface-bound N2 and −3.2 V (vs. RHE) for first proton-coupled one-electron hydrogenation of N2 (Zhu et al., 2013; Shi et al., 2020a; Huang et al., 2020), are beyond the photocatalysis capability of most semiconductors without active sites.
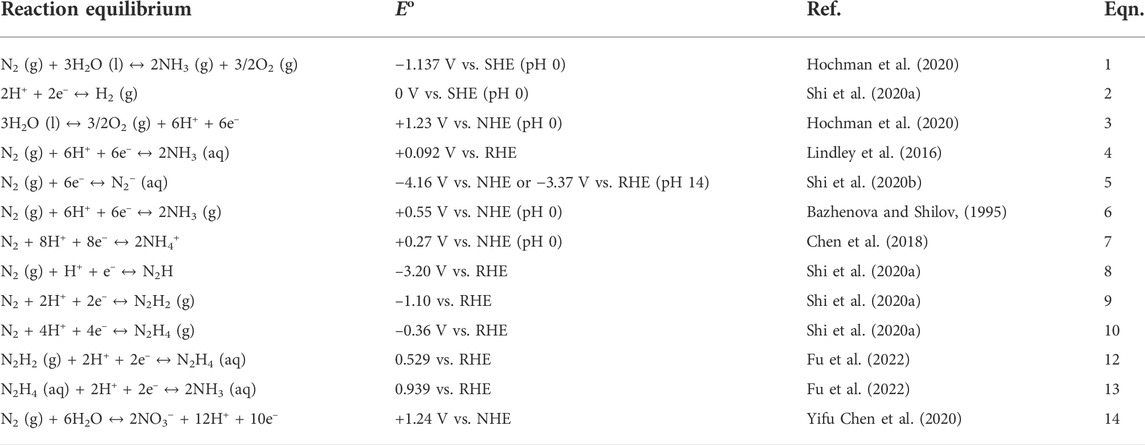
TABLE 1. Thermodynamic potentials of hydrogenation reactions related to the whole nitrogen fixation pathways.
The initial chemisorption and activation of N2 could trigger the formation of different kinds of species in the subsequent reactions owing to multiple complicated electrons transferred hydrogenation procedures and the presence of reactive oxygen species by water oxidation half-reaction, such as oxygen, hydroxyl radicals ·OH (2H2O+ 4h+ → O2 + 4H+, E0 = 0.81 V vs. NHE at pH 7 or H2O+ h+ → OH + H+, E0 = 2.32 V vs. NHE at pH 7) (Sun et al., 2018). Adversely, the formed O2 further captures electrons in the CB to suppress the NH3 photosynthesis (Shiraishi et al., 2018; Sun et al., 2018), and the strong oxidation agents ·OH can also further generate nitrite or nitrate from the generated NH3 by photooxidation, which are detrimental to N2 photoreduction to NH3. Because of its weaker interaction with the surface O atom caused by vacancies or doping, metal is further less firmly bound to O following the release of one H atom from adsorbed H2O* onto the surface O atom, thus two OH* bonded at the neighboring catalytic sites are easily coupled forming peroxide H2O2* preferentially adsorbed at active sites, thereafter poisoning the photocatalyst (Liu et al., 2012). The oxidation half-reaction products also oxidize the photogenerated NH3 to HNO3, leading to a decrease in the NH3 yield. Yang et al. (2021) transformed the N2 disproportionation reaction into a complete reductive nitrogen photofixation by introducing Au nanoparticles into Fe-TiO2 to effectively decompose H2O2. Some neglected N2 fixation products (e.g., N2H4, NO2−, and NO3−, etc.) as essential chemicals should be noted (Table 1, Eqs. 10 and 14), in which N2H4 as a by-product from afore-mentioned associative alternating pathways has also been detected in the same research (Schrauzer and Guth, 2002; Xiao-Fei Li et al., 2016). Li et al. (Hao Li et al., 2016) discovered that N2H4 was quickly generated over the BiOCl nanosheets exposed with (010) facets and then gradually disappeared to produce NH3, which strongly supports the alternative mechanism. In terms of thermodynamic potentials (Table 1, Eqs. 9 and 12), N2H4 is more prone to be transformed to NH3 from surface–N2H4 intermediates due to its much weaker N–N single bond. Similar to the hydrogenation pathway to NH3, dissociated adsorbed N2 molecule is oxidized to the intermediate of metastable NO* by photogenerated h+, followed by further oxidation with O2 and H2O from the reaction media, by which N2 is converted to nitrate and nitrite through continuous photooxidation reactions (Comer and Medford, 2018). The previously reported N2 photoreduction products are either NH4+ or NO3− (Liu et al., 2019; Dong Zhang et al., 2022), simultaneous coproduction of NH4+ and NO3− through simultaneous reduction and oxidation of N2 in pure water, which was demonstrated to occur spontaneously in aqueous solutions (Ren et al., 2020). Another critical aspect in NH3 production via photochemical N2 reduction is that some key reaction intermediates are instrumental in NH3 conversion. Zhao et al. (2022) established a redox pathway with a lower kinetic barrier for NH3 photosynthesis, in which N2 and O2 can be trapped at the oxygen vacancies in ultrathin two-dimensional (2D) CuCo metal-organic frameworks (MOFs) to generate *NO and further be reduced to NH3 by visible light. Although the desired NH3 conversion and selectivity are swayed by these competing reactions, some by-products also play imperative roles in industrial production and living needs.
The addition of sacrificial agents (typically electron donors such as sulfites, amines, humic acid, ascorbic acid, and alcohols) with oxidation potentials lower than water appeases the requirement for oxidizing ability in some SC photocatalysts, further suppressing electron-hole pair recombination (Shen et al., 2020). On the other hand, the overall production rate of NH3 is kinetically balanced by the hole consumption rate on the photocatalyst since electrons and holes are generated in pairs under illumination. Organic alcohols with an α-H adjacent to the OH group(s)-to wit, methanol, ethanol, 2-propanol, ethylene glycol, and so forth, can react with holes in VB to accelerate the production of electrons and liberation of protons (Chen et al., 2015). Among them, methanol is demonstrated to be more appropriate and efficient than other hole sacrificial reagents. Methanol not only loses electrons more easily to consume the accumulated holes due to its lower HOMO (Zhao et al., 2015), but also promotes the solubility of N2, which could act as a proton donor and partial electron donor for subsequent reduction reactions (Li et al., 2018). However, methanol as the sacrificial agent could be oxidized to form carbonyl-containing compounds (e.g., aldehyde or ketone) and finally CO2, which might interfere with product detection and quantification. The N2 fixation reaction can also be facilitated by *CO2− produced from the oxidation process of methanol (Swain et al., 2020). Cao and co-workers (Cao et al., 2018) found that the·CO2− intermediates transformed from methanol or absorbed CO2 affected the nitrogen fixation due to their strongly reducing ability (ECO2/·CO2− = 1.8 V) (5N2 + 2CO2− + 4H2O → 2NH3 + 2CO2 + 2OH−) (Dimitrijevic et al., 2011). It should be noted that the NH3 formation mechanism in such sacrificial systems should be taken with great caution, the target scavenger should be chosen carefully.
2.4 Protocols, evaluation, and detection methods in photocatalytic NRR
Despite the great strides toward photon-driven ammonia production that have been taken, unified rules and standards in this fledgling field should be formulated to ensure the authority and accuracy, by which the impetus imparted forcefully contribute to advance of the sustainable technology. Since the low NH3 yield (ppb/ppm level) and ubiquitous contaminants plague experimental practices (Gao et al., 2018; Tang and Qiao, 2019; Zhao et al., 2019), establishing a uniform protocol for rigorous experiments preceding the detection and quantification of NH3 are noncontroversial. Therefore, to clarify the source of NH3 and ensure data reproducibility, various contamination sources from the environment, latex gloves, human respiration, stable deionized water, photochemical reactor, or the used feed gas, even NH3 or amine groups unintentionally introduced by catalysts, should be ruled out to avoid overestimation of the NH3 concentration (Bai et al., 2017; Zhao et al., 2021). The photocatalysts, photoreaction set-up and all its components as well as sample tubing should be thoroughly rinsed with fresh ultrapure water and properly stored. Particular emphasis should be put on the NOx contaminants derived from N-containing chemicals, protic solvents, or supply gas, which are easily reduced to NH3 (Xue Chen et al., 2020; Li et al., 2022a; Feng Wang et al., 2022; Xu et al., 2022). To account for the reliability and repeatability of photoactivity, it is strongly recommended to use reported rigorous experimental protocols (Andersen et al., 2019; Tang and Qiao, 2019; Choi et al., 2020; Shen et al., 2021), which clearly list experimental methods, gas purification, blank and control experiments, especially the isotopic labeling experiments. The 14N2 and 15N2 feed gases should be pre-purified to remove any possible NOx or NH3 to eliminate uncertainty and even false positives of catalytic data (Zhao et al., 2020; Hui et al., 2022; Yuting Wang et al., 2022).
However, controversy exists as to how to obtain reliable detection and quantification of NH3 and NH4+, which severely hampered the growth of this field. Fortunately, the development of measuring techniques further pushed the advance of NH3 evaluation. Currently, detection and quantification of NH3 could be mainly divided into five methods, including spectrophotometric/colorimetric assays using indophenol blue or Nessler’s reagent, ion chromatography (IC), ion-selective electrode (ISE), fluorescence, and 1H-NMR spectroscopy methods (Gao et al., 2018; Zhao et al., 2019). These methods are methodologically sound and get concordant precise results for NH3 determination in water systems (Mansingh et al., 2021). However, each of these methods has both advantages and limits for measuring NH3. Colorimetric assays are widely available with benefits of good sensitivity, fine accuracy (0–500 µgNH3 L−1), and low cost (Zhao et al., 2019). The pH, solvent used, presence of certain metal ions, sacrificial agents and their oxides, and nitrogen-containing chemicals can all adversely increase the amount of NH3 detected by the coloration methods (Gao et al., 2018). IC is recommended for NH3 quantification given its reproducible, precise results with a wider range of NH3 estimation, superior efficiency and selectivity, and good stability, but it still suffers from the disadvantage that certain sacrificial agents affect the separation efficiency of the cation-exchange column. NMR spectroscopy, mass spectroscopy, and enzyme assays are also employed as supplementary measures to eliminate the possible false-positive results, the first of which, even quantitatively, could not prove the origin of all ammonia generated. For consistency and scientific rigor, the concentration of NH3 detected should be cross-checked with two or more different quantitative methods, even conducted in in-situ and continuous monitoring processes for reliable evidences from an unimpeachable source.
Given the lack of the standard photocatalytic reaction systems used in conventional laboratory tests (Ziegenbalg et al., 2021), it is therefore necessary to pay particular attention to various vital details during the assessment of photocatalytic nitrogen fixation. Figure 4 presents the effect of important experimental parameters on the observed catalytic performance for photo-driven NH3 production. An objective and meaningful comparison of photocatalytic NH3 synthesis performance among different groups is heavily reliant on a set of standard experimental conditions (e.g., light source intensity, irradiation wavelength range, photocatalyst dosage, reaction solution volume, reactor type, reaction temperature and pressure, etc.). Many technical aspects especially the light source are often missing or not stated in sufficient details. Standardization of reactor design is desirable, thereby minimizing the impact of geometry. Reported performance evaluation is primarily based on NH3 yield, apparent quantum yield/efficiency (AQY/AQE) and turnover frequency (TOF). However, mass-based performance metrics (e.g., μmol h−1 g−1) are insufficient because photocatalytic activity is not necessarily proportional to the catalyst mass (Kramm et al., 2019; Huimin Liu et al., 2021). Similarly, AQY/AQE is often used to evaluate the photocatalytic activity under monochromatic light excitation of the same specific wavelength, which is closely related to the wavelength of incident photons and the intrinsic properties of the materials, since photocatalysts generally behave differently on absorption coefficients and photocatalytic activities at each irradiation wavelength (Yuhua Wang et al., 2019). TOF differs as well in terms of the active centers. Solar-to-NH3-yield (SAY)/solar-to-NH3 (STA) efficiency can be universally used as the practical standard for comparison, which is determined by a solar simulator (AM 1.5G) with an irradiance of 100 mW cm−2. With more regard to normalizing evaluation systems, solar-to-NH3 energy conversion (SEC) efficiency is suggested to objectively compare the catalytic activity of different materials and assay the future industrialization opportunities (targeted SEC ≈10%) (Rong Zhang et al., 2019; Ziegenbalg et al., 2021).
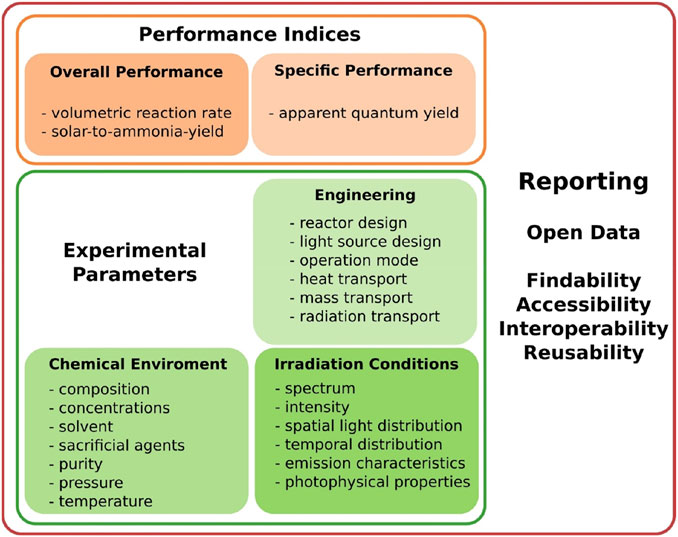
FIGURE 4. Guidelines for experiment normalization to rule out the impact of various parameters that influence the overall and specific performance indices. Reproduced from ref. (Ziegenbalg et al., 2021). With permission from the John Wiley and Sons.
3 Tungsten-based and related photocatalysts for NRR
3.1 Metal oxide-based photocatalysts
3.1.1 Tungsten oxides
Tungsten oxides, commonly denoted as WO3−x∙nH2O (x < 1, n = 0–2), are somewhat distorted in their crystal structure made up of perovskite units, rather not perfect octahedral, with bandgap energies ranging from 2.4 to 2.8 eV depending on their stoichiometries, crystalline structure and density of defects (Zheng et al., 2011; Sun et al., 2019). Tungsten trioxide (WO3) is the most popular semiconductor among all tungsten oxides because of its exceptional chromic properties and mixed polymorphs, which manifest in rich and diverse structures (Girish Kumar and Koteswara Rao, 2015; Yin and Asakura, 2019; Cheng and Zhang, 2020). Among different structures of WO3, the monoclinic phase is a preferred photocatalyst with the most relatively thermodynamically stable configuration than orthorhombic and hexagonal phases, while triclinic and cubic crystal structures rarely get attention (Zheng et al., 2011; Girish Kumar and Koteswara Rao, 2015; Fan et al., 2021). Hou et al. (Hou et al., 2019) developed a facile method to prepare monoclinic WO3 via thermal treatment of nanoporous metals, wherein nanoporous WO3-600 was composed of connected grains rather than a single grain, containing abundant grain boundaries (GBs) (Figures 5A–C). Impressively, WO3-600 showed excellent performance for photocatalytic NRR with an NH3 yield rate as high as 230 µmol gcat.−1 h−1 without any sacrificial agents at room temperature, 17 times higher than that for WO3 nanoparticles (WO3-NPs) without GBs (Figure 5D). Moreover, almost 100% of initial activity was maintained even after ten successive reaction rounds over WO3-600 (Figure 5E). Quasi in situ XPS and in situ electron spin resonance (ESR) measurements have been employed to verify the pivotal role of GBs in inducing a large number of operando OVs under light irradiation (Figures 5F,G). These operando OVs served as highly active sites for efficient adsorption and activation of N2, which have been confirmed by temperature-programmed desorption of N2 (N2-TPD) (Figure 5H), directly contributing to easier delivery of photoexcited electrons to adsorbates through metal-oxygen covalency. Then N2H* intermediates coupled with protons were observed by in situ diffuse reflectance infrared Fourier transform (DRIFT) (Figure 5I). As shown in Figure 5J, sub-5-nm-sized nanowires of hexagonal tungsten oxide (h-W O 3) via a dopant replacement-driven molten salt method also turned out to be excellent photocatalysts with a high NH3 production rate of 370 μmol g−1 h−1, benefiting from unique features of the Mo-doped ultrathin hexagonal structure, thus facilitating carrier separation and dissociation of N2 molecules (Mao et al., 2020). Tailoring the morphology of WO3 is not only a rational route to investigate the relationship between the microstructure and photocatalytic performances, but also a feasible approach for fabricating highly photoactive nanomaterials.
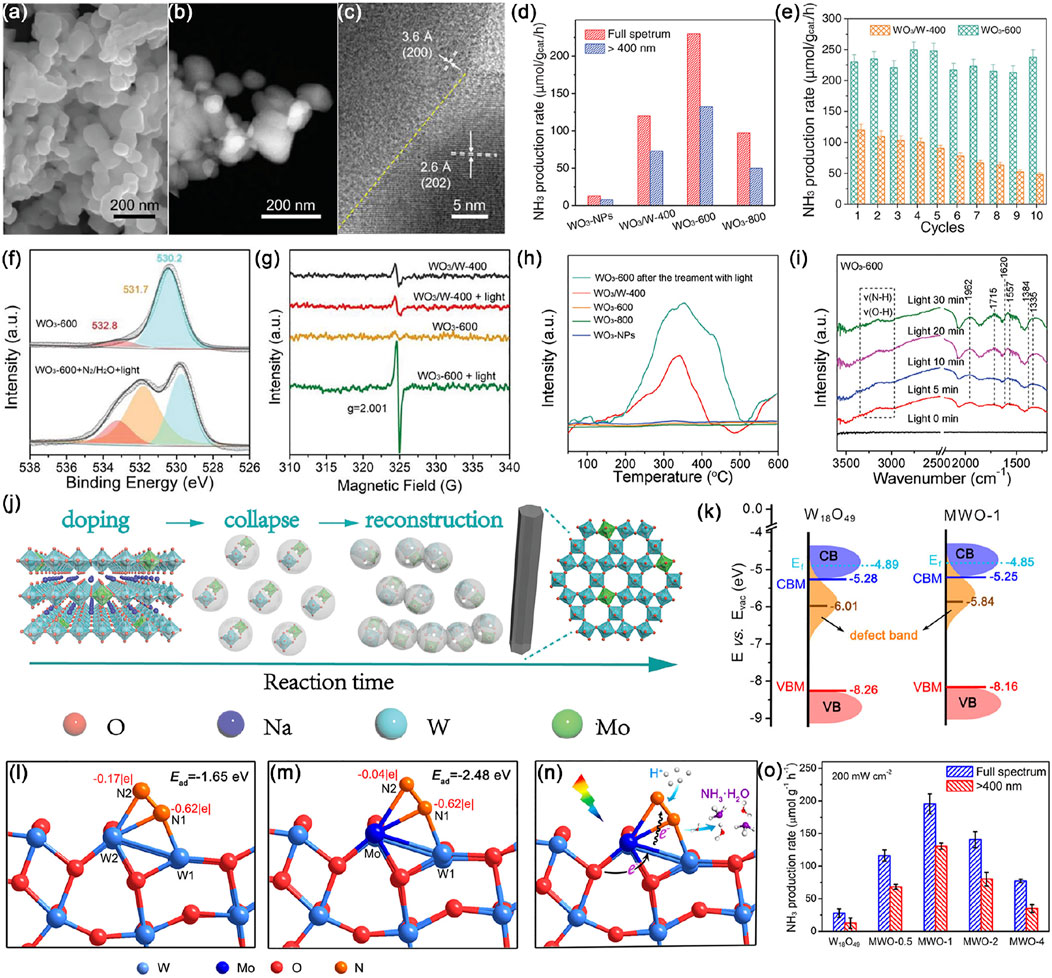
FIGURE 5. (A) Scanning electron microscopy (SEM) image, (B) high-angle annular dark-field scanning transmission electron microscopy (HAADF-STEM) image, and (C) high-resolution TEM (HRTEM) image of WO3-600. (D) Photocatalytic NH3 production rates over WO3-NPs, WO3/W-400, WO3-600, and WO3-800. (E) NH3 production rates for WO3-600 and WO3/W-400 over the course of ten rounds of successive reactions. (F) Quasi in situ XPS spectra of O 1s in WO3-600 before and after treatment. (G) In situ ESR spectra of WO3/W-400 and WO3-600 before and after light irradiation. (H) N2-TPD profiles of WO3/W-400, WO3-600, WO3-800, WO3-NPs, and WO3-600 after the treatment with light. (I) In situ DRIFT spectra recorded during the photocatalytic N2 fixation over WO3-600. Reproduced from Hou et al. (2019) with permission from the John Wiley and Sons. (J) Schematic illustration of the generation of Mo doped h-WO3 crystalline nanowires. Reproduced from Mao et al. (2020) with permission from the John Wiley and Sons. (K) Schematic illustration of the electronic band structures of W18O49 and 1 mol% Mo-doped W18O49 ultrathin nanowires. Optimized adsorption configurations of N2 molecules and their corresponding charge distribution on the surface of (L) W18O49 and (M) Mo-doped W18O49. (N) Scheme for photocatalytic N2 reduction over Mo-doped W18O49. (O) Photocatalytic ammonia production rates by W18O49, MWO-0.5, MWO-1, MWO-2, and MWO-4 UTNWs in the first 2 h. Reproduced from Ning Zhang et al. (2018) with permission from American Chemical Society.
Since the lattice of WO3 withstands a considerable loss of oxygen content, the resulting nonstoichiometric tungsten suboxides (WO3–x) compositions such as W20O58, W18O49 and W24O68, have suitable bandgap energy, and tunable electronic band structure, charge redistribution, as well as existence of mixed-valence W ions benefiting different degrees of oxygen deficiency in their structures (Song et al., 2015; Sun et al., 2019; Yin and Asakura, 2019; Meng Yang et al., 2020). To accommodate the large energy band of N2, Zhang et al. (Congmin Zhang et al., 2018) used a solvothermal method to fabricate defect-rich W18O49 ultrathin nanowires doped with Mo, where Mo dopants shifted defect-band center up toward the Fermi level (EF), thereby harvesting more photon energy to provide adequate energetic electrons for N2 reduction (Figure 5K). Moreover, the Mo–O covalent bond facilitated the separation and transfer of photogenerated charges from coordinatively unsaturated Mo sites to N2 adsorbates, while the formation of the Mo−W bond can effectively enhance the molecular polarization of chemisorbed N2 as a reactive dual-active center, resulting in better activation (Figures 5L, N). The as-prepared 1 mol% Mo-doped W18O49 sample showed enhanced photocatalytic performance with an NH3 generation rate of 195.5 μmol g−1 h−1 and STA efficiency of 0.028% under simulated sunlight (Figure 5O). Analogously, Mn2+ ions were introduced to replace W sites in the W18O49 lattice, which not only acted as chemisorption and activation centers for N2 and H2O molecules, but also facilitated the separation and migration of photogenerated charges (Ying et al., 2019). Tailoring surface oxygen vacancies and doping of tungsten oxides with heteroatoms are effective strategies to increase the number of active sites for N2 chemisorption (Mingli Zhang et al., 2020).
3.1.2 Molybdenum trioxides
Molybdenum in the same transition metal group with tungsten shares some similar chemical properties, where a non-stoichiometric form MoO3–x, analogous to WO3–x, exhibits prodigious potential for solar-driven photocatalysis due to its strong OVs-induced localized surface plasmon resonance (LSPR) absorption in visible-near infrared (vis-NIR) region (Yehuan Li et al., 2019; Zheng Wang et al., 2019; Qiu et al., 2021; Li et al., 2022b). Bai et al. (Bai et al., 2022) synthesized Schottky-barrier-free MoO3−x spheres via a facile aerosol-spray method for plasmon-driven photochemical N2 fixation to NH3, which features metal-like free charge carriers with the Fermi level above the bottom of the defect band and the defect band located closely to the conduction band (Figures 6A,B). The MoO3−x spheres treated at 350 °C delivered an NH3 production rate of 435.57 μmol h−1 g−1 in 20 vol% methanol aqueous solution under full-spectrum Xe lamp illumination, with an AQE of 1.24% at 808 nm and 1.12% at 1,064 nm and a STA efficiency of 0.057% in pure water under simulated sunlight (Figures 6C,D). Both the measured AQEs and the corresponding wavelengths under NIR region are among the highest values to date. Specifically, the OVs enable a perfect functional combination of rich active sites for N2 absorption with broad-spectrum plasmon-induced hot electrons and empty states in the defect band within the MoO3−x spheres, which facilitates the multi-electron reduction-oxidation (red-ox) reactions involved in photocatalytic N2 reduction. With the Schottky-barrier-free characteristic, the hot electrons moved freely in the defect-induced electronic states and the conduction band reduced those adsorbed and activated N2 molecules trapped at the OVs to produce NH3, as illustrated in Figure 6B. The defective MoO3−x has been exploited as matrix support to valorize rare earth La single-atom catalysts (SACs) via simple Lewis acid-base interactions due to their well-defined surface structure and high degree of anisotropy (Liu et al., 2022). The density functional theory (DFT) calculations revealed that a La single atom theoretically tends to occupy terminal OVs and coordinate with 2-coordinated O site (Mo–O–Mo, O2c) to form O2c-La-O2c coordination. Moreover, the role of La atoms on O2c–La–O2c site was further clarified, which pumps energetic electrons from their unsaturated 5d orbitals into the π * 2p orbital of the adsorbed N2, boosting N2 adsorption and activation. Isolated atomically dispersed La atoms anchored on MoO3−x support were observed by the aberration-corrected HAADF-STEM and corresponding rainbow-colored images. The O2c−La−O2c configuration close to two oxygen coordination environment was further verified by X-ray absorption fine structure (XAFS), by which La−La bond cannot be distinctly observed in La/MoO3−x. La/MoO3−x possess an NH3 production rate of 209.0 μmol h−1 g−1 without any hole scavenger under visible light, nearly 10 times that of the support. Enhanced adsorption of nitrogen and the symmetric alternative pathway following a side-on bridging adsorption configuration have been corroborated by in situ FT-IR spectra with the combination of DFT calculations, thus La single atoms substantially amplify the activation of N2 toward successive hydrogenation, while lowering the formation energy barrier for *NNH → *NHNH process.
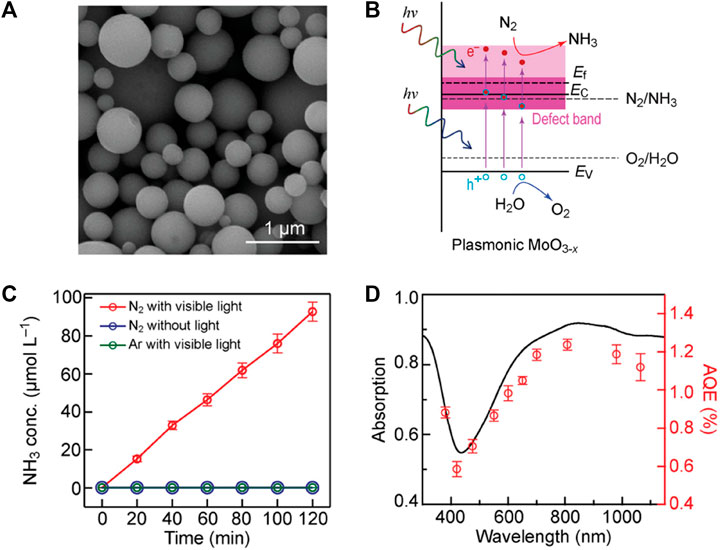
FIGURE 6. (A) SEM image of the MoO3−x spheres prepared at 350°C. (B) Schematics illustrating the band structures of the plasmonic MoO3−x photocatalyst. (C) Photocatalytic NH3 production under different conditions and (D) AQE at different wavelengths over the MoO3−x spheres. The light absorption spectrum is plotted for comparison. Reproduced from Bai et al. (2022) with permission from the John Wiley and Sons.
3.1.3 Mixed valence cobalt oxides
Cobalt, one of the earth-abundant first row transition metals, has gained tremendous attention for photocatalytic N2 reduction and conversion due to its eligibility for N2 dissociation and tunable activity (Chu et al., 2019; Gao et al., 2019), whereas its oxides with mixed-valence states of Co species have poor photostability due to photo-corrosion arising from half oxidation reaction. Combination of CoOx with 2D carbon material supports have been rationally explored to optimize their electronic structures and tailor the active site density (Ahmed et al., 2019; Chu et al., 2019; Zhi-Yuan Wang et al., 2020; Lu et al., 2022). For example, Liu et al. (Yuxin Liu et al., 2021) fabricated CoOx quantum dots anchored on porous graphdiyne (GDY) (GDY@CoOxQD) to construct highly efficient and robust catalysts via a facile in-situ growth strategy for photocatalytic NRR. The composite photocatalysts featured with superlative activity and stability under various conditions as a result of strong quantum effect and a highly compatible synergistic effect. The three-dimensional configurations of the self-supported GDY@CoOxQD nanosheet array and uniformly dispersed CoOxQD on the porous GDY surface were confirmed by SEM and HRTEM, respectively. An average NH3 yield rate of 46 independent experiments over the GDY@CoOxQD in 0.1 M Na2SO4 aqueous solution (pH 7) up to 19,583 μmol g−1 h−1 was attained, exceeding most reported catalysts. Equally importantly, the six different batches exhibited long-term stability of 10 h with nearly constant NH3 yield rates. By comparing the Co XPS results of CoOxQDs on different supported carbon materials and the XANES of GDY@CoOxQD before and after the reaction, it was concluded that the mixed-valence states of Co (Co3+ and Co2+) played a pivotal role in enhancing the reaction activity. As revealed by the DFT calculations, the introduction of GDY and the coexistence of Co2+/Co3+ could facilitate the electron transfer to form a strong d-π* (unocc) antibonding orbital interaction above EF and d-π* (occ) bonding orbital interaction below EF. This is beneficial for weakening the bond order and bond strength of N≡N bond.
Lu et al. (2022) also reported that different cobalt oxide species were responsible for innate active properties, resulting in a synergistic effect on the two half reactions with reduction and oxidation spatially separated at CoO and Co3O4, respectively. The CoO-Co3O4 mixed-oxide (CoO dominated) composites on reduced graphene oxide (RGO) manifested a remarkable NH3 formation efficiency of 89.1 μmol g−1 h−1, over 14 times that of each single component. Furthermore, the photoreaction-induced cation oxidation (CoO to Co3O4) was reduceable/recyclable by photo-reactivating the non-active Co3O4 back to the active CoO at room temperature, thus leading to well-maintained NRR activity after six cycles of operation. As indicated by XANES, XPS, and HRTEM measurements, the compositions were completely transformed into Co3O4 during the 8 h of NRR and converted back to dominant CoO after the reactivation. The component CoO in the composite entailed deep-red-light absorbing defect states, which hindered carrier recombination. The band structure of CoO/Co3O4 formed a direct Z-scheme heterojunction, in which the electrons at the CB of CoO reduced N2 molecules and the holes at the VB and defect energy levels of Co3O4 oxidized H2O molecules.
3.1.4 Other transition metal oxides
Non-noble metal oxides have gained tremendous expectations as promising alternative NRR catalysts primarily owing to their high chemical stability, ease of synthesis, and minimization of noble metals consumption (Liang Yang et al., 2020; Gao et al., 2022; Zhen Zhao et al., 2022). Only a few metal oxides such as VO2 (Haiguang Zhang et al., 2019), Ta2O5 (Fu et al., 2019), Nb2O5 (Han et al., 2018; Kong et al., 2019), NbO2 (Huang et al., 2019), and ZrO2 (Xu et al., 2020) have been reported for electrocatalytic NRR, However, these metal oxides suffer from large band gap, poor light absorption capacity toward visible light, low quantum efficiency, and fast recombination rate of photogenerated excitons, restricting their applications in photocatalytic NRR. The insulating material ZrO2 with a band gap of ∼5.0 eV (Xu and Schoonen, 2000) can absorb ultrahigh UV light (Gaggero et al., 2021). In addition, it possesses high mechanical strength, non-toxicity, and corrosion resistance. Intensive research has been concentrated on engineering and visible photosensitization of high band gap oxides based on Zr elements of the fourth subgroup (Oshikiri et al., 2016; Caiting Feng et al., 2022). Theoretical calculations revealed that the adsorption energy of N (ΔN*) is much lower than that of H on the ZrO2 surface in the aqueous photocatalytic NRR process. This suggests that ZrO2 preferentially adsorbs N atoms and baffles the reduction of H2O to H2 (Skúlason et al., 2012; Tao et al., 2019). Mou et al. (Mou et al., 2019) demonstrated photocatalytic NRR to NH3 based on amorphous ZrO2 in association with g-C3N4 as a visible light harvester. The g-C3N4/ZrO2 lamellar composites were constructed by a simple one-step pyrolysis of the deep eutectic solvent ZrOCl2·8H2O/urea (Figure 7A). The composites imparted an optimal NH3 yield rate of 1,446 μmol L−1 h−1 under visible light illumination, noticeably outperforming each individual counterpart (Figure 7B). The introduction of amorphous ZrO2 restrained the hydrogen generation and facilitated N2 reduction. A synergy between amorphous ZrO2 and g-C3N4 was created contributing to the rapid photoproduced electron–hole pair separation and transfer (Figures 7C–E). 15N isotope analysis verified the contamination-free N2 photofixation in this work. Similarly, Oshikiri and co-workers designed a multi-component photocatalytic system of Au-NPs/Nb-SrTiO3/Zr/ZrOx. The composite was shown to have high affinity to NH3. Plasmonic-induced charge separation happened at the Au/SrTiO3 interface, thereby allowing occurrence of oxidation reactions on gold nanocrystals and N2 reduction on the ZrOx@Zr coating (Figures 7F,G). Figure 7H shows the large interface of ZrOx@Zr and Nb-SrTiO3 affording high selectivity and efficiency for NH3 synthesis owing to the advantage of a stronger binding of ZrOx@Zr to N atoms relative to H atoms compared to Ru.
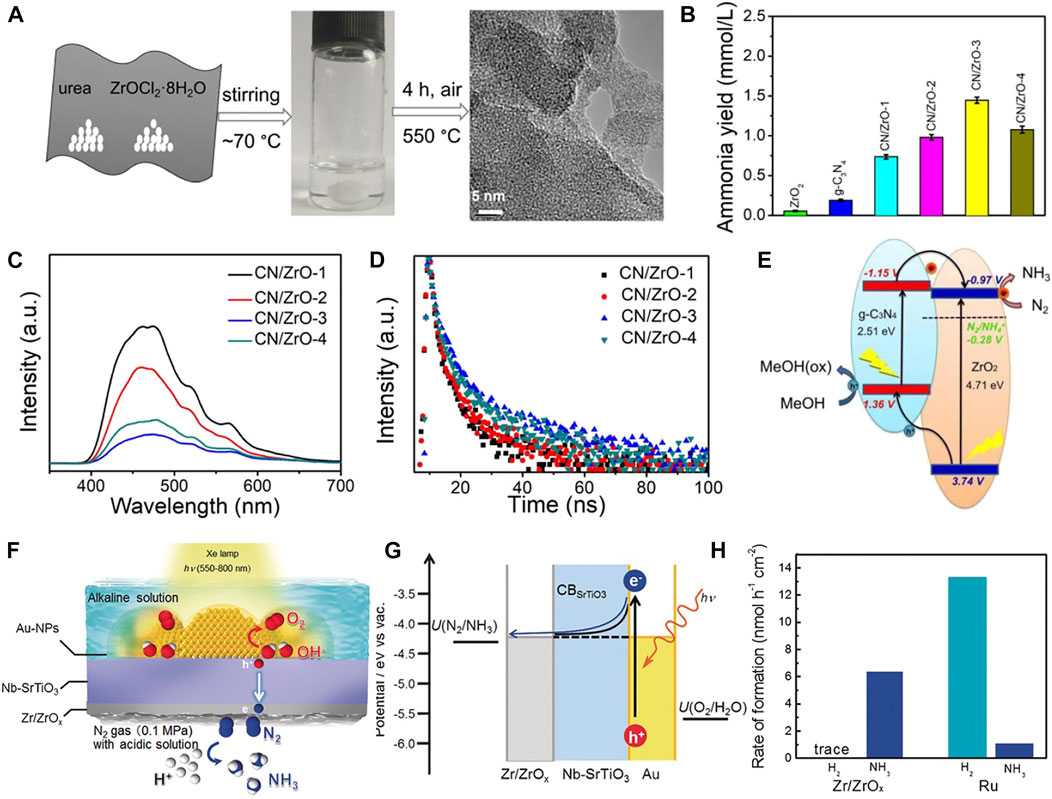
FIGURE 7. (A) Schematic of g-C3N4/ZrO2 composite synthesis. (B) NH3 yields of pure ZrO2, g-C3N4 and g-C3N4/ZrO2 composites with different ZrO2 contents. (C) Photoluminescence (PL) spectroscopy and (D) time-resolved PL (TRPL) decay spectra. (E) Schematic of mechanism for photocatalytic N2 fixation over g-C3N4/ZrO2 composites. Reproduced from Mou et al. (2019) with permission from American Chemical Society. (F) Schematic of NRR on Au-NPs/Nb-SrTiO3/Zr/ZrOx photoelectrode. (G) Energy-level diagram of the plasmon-induced NH3 synthesis device. U: redox potential. (H) NH3 formation rate over Au-NPs/Nb-SrTiO3/Zr/ZrOx and Au-NPs/Nb-SrTiO3/Ru. Reproduced from Oshikiri et al. (2016) with permission from the John Wiley and Sons.
3.2 Oxometallate-based photocatalysts
3.2.1 Bismuth-based oxometallates
Among various polyoxometalates, bismuth-based compounds such as Bi2WO6, Bi2MoO6 of Aurivillius structure, and BiVO4 of Scheelite structure, are another class of oxides of interest because of their considerable chemical stability, up-shifted VB resulting from hybridization between Bi 6s and O 2p states and narrow band gaps, together with their similar layered crystal structures complimentary for charge separation and transfer (Zhang et al., 2011; Hao et al., 2020; Wenjie Liu et al., 2020; Utomo et al., 2022). Besides, the hybridization of Bi 6s and O 2p levels accounts for their largely dispersed VB, benefiting the migration of photoinduced holes and thus improving the oxidative reactions (Huang et al., 2020). Bi2WO6 consists of accumulated layers of discontinuous [Bi2O2]2+ and octahedral [WO4]2− sheets, and Bi2MoO6 is composed of [MoO2]2+ layers and [Bi2O2]2+ layers, while BiVO4 features with a monoclinic crystal system comprising BiO8 and VO4 groups (Cooper et al., 2014; Dai et al., 2016; Zhu et al., 2022). Nevertheless, the potentially high NRR activities are profoundly impeded by their intrinsic shortcomings, such as the photo-corrosion susceptibility for Bi2WO6, the limited light absorption in the ultraviolet region for Bi2MoO6, the poor water oxidation kinetics and slow mobility of photo-excited charge carriers for BiVO4 (Girish Kumar and Koteswara Rao, 2015; Xin Liu et al., 2020). Also, they suffer from limited interaction with nitrogen and weak reducing ability as the CB is not sufficiently negative (Hao et al., 2020). To alleviate these problems, heteroatom doping (Meng et al., 2019; Lin Liu et al., 2021), control of facet exposure (Zhang et al., 2021), defect engineering (Haitao Li et al., 2021; Libo Wang et al., 2021; Cai Feng et al., 2022; Guoan Wang et al., 2022), heterostructure construction (Fei et al., 2019; Shende et al., 2019; Xue et al., 2019; Vesali-Kermani et al., 2020a; Chao Liu et al., 2020; Xuerui Zhang et al., 2022), morphology modification (Ning Zhang et al., 2018; Zhou et al., 2019; Sun et al., 2020; Bao et al., 2021), and regulation of internal electric fields (Lv et al., 2018) have been employed to improve the photocatalytic efficiency of N2-to-NH3 conversion.
Hao et al. (2016) doped Bi2WO6 with different ratios of Mo, combined with exposed edge unsaturated Mo atoms as the active center for N2 adsorption, activation and photocatalytic reduction (Figure 8A). Benefiting from exposed active sites, narrower bandgap, and ultrasmall subunits in Bi2MoO6 system (H-Bi2MoO6), N2 molecules from air were transformed into NH3 with an NH3 evolution rate of 1.3 mmol g−1 h−1 under simulated sunlight illumination, ≈9.5 times higher than bare Bi2MoO6 (Figure 8B). In another work, Wang et al. demonstrated creation of enriched surface OVs via surface-layer Br doping into Bi2MoO6 (BMO) which boosted photocatalytic NRR activity owing to enhanced chemisorption of N2 molecules, enlarged surface area, improved photogenerated charge separation and transfer efficiencies (Lin Wang et al., 2022). The as-made hierarchical BMO microspheres comprised small nanosheets, in which two Br replaced one MoO42− with OH coordination balancing the crystal structure. DFT simulation indicated that Br doping promoted the formation of surface OVs at adjacent Bi, thus inducing the fabrication of the surface/internal homojunction to enhance the photogenerated charge separation. Addition of methanol as an electron donor (eliminating holes) further accelerated the generation of NH3 with a rate of 4.77 μmol h−1. The BMO showed no decrease in photocatalytic activity after five consecutive runs, manifesting its high stability, while the low stability of BMO80 in pure water indicates photogenerated hole oxidation induced by Br loss and OV reduction. Exposing different crystal facets provides enhanced spatial separation of photogenerated electrons and holes between different crystal facets. For instance, Zhang et al. (Zhang et al., 2021) controlled the growth of (040) and (110) facets of single-crystal BiVO4 by adjusting pH, and studied the correlation of facet ratios of BiVO4 crystals with photocatalytic NRR performance. They found that the activity was linearly dependent on the ratio of exposed S(040)/S(110). Separation of space charges was boosted by in-situ photo-deposition of Ag NPs and MnOx selectively loaded on the respective (040) and (110) planes. This enabled creation of a built-in electric field (BIEF) between (040)/(110) facets. Based on the results of active sites and DFT calculations, the cycle of oxygen vacancy-V4+/V5+ in the (040) facets was inferred to be the exact active site for photocatalytic NH3 synthesis. V4+ was proposed to enhance chemisorption of N2 while V5+ behaved as an electron transfer bridge, and the photogenerated electrons trapped in OVs provided driving force for NRR.
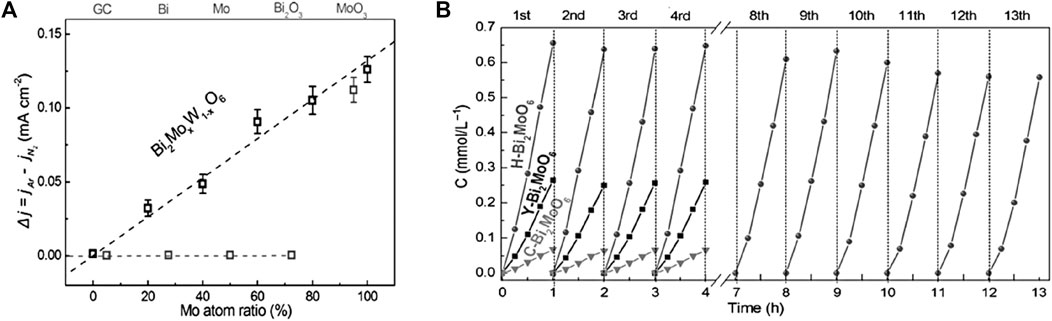
FIGURE 8. (A) The relative current densities (Δj = jAr – jN2) measured at 0.6 V vs. saturated calomel electrode (SCE) of different samples. (B) Multicycle photocatalytic NH3 generation from air of different samples under simulated sunlight irradiation. Reproduced from Zhao et al., 2016 with permission from the John Wiley and Sons.
3.2.2 Polymetallic oxides
Most ternary metal oxides first used for photocatalytic nitrogen fixation are titanate or Bi-based oxide systems (Chen et al., 2018; Huang et al., 2020). Higher conversion efficiency for mingled transition metal composite oxides such as Sb2MoO6 (Mousavi et al., 2022), SrMoO4 (Luo et al., 2019), LaCoO3 (Haiguang Zhang et al., 2019), CoFe2O4 (Zheng et al., 2020), Ni3V2O8 (Vesali-Kermani et al., 2020b), KNbO3 (Xing et al., 2019; Xing et al., 2020; Chamack et al., 2022), LiNbO3 (Xiazhang Li et al., 2020), have been reported compared to corresponding monometallic oxides. Most mixed metal oxides are perovskite oxide compounds with wide bandgaps (Zejian Wang et al., 2021). To overcome the large band gap issue, exotic element doping, noble metal loading, and coupling with other semiconductors can be applied to broaden the light absorption range. For instance, doping of SrMoO4 with Fe simultaneously introduced defect states and Fe–Mo–O active centers acting as the active sites for N2 adsorption, which minimized bandgaps to extend the absorption edge (Luo et al., 2019). As the doping concentration increased, the intrinsic bandgap became narrowed, enabling utilization of visible light. However, the excess doped heteroatoms could act as recombination sites for photoproduced charge carriers, as reflected that the normalized photocurrent transient decreased with that of NH3 yield at higher doping concentrations.
Niobates have emerged as a research hotspot because of their sufficiently negative CB potential endowing photogenerated electrons with strong reducibility and their spontaneous polarization nature promoting surface charge separation (Chen et al., 2019; Chao Liu et al., 2020; Nunes et al., 2020). Chen et al. (2021a) demonstrated the synergy effect between Bi2S3 and KTa0.75Nb0.25O3 (KTN) under simulated sunlight irradiation and the simultaneous action of light and ultrasonic irradiation. The hybrid displayed high piezo-photocatalytic performance with an NH3 production rate reaching 581 μmol L−1 g−1 h−1. All samples depended on KTa0.75Nb0.25O3 to provide the photo/piezogenerated electrons for promoting spatial charge separation, which likely played a dominant role for nitrogen fixation. A pioneering study that coupled conventional KNbO3 with photoactive MOFs was conducted by Chamack et al. (Chamack et al., 2022). Introduction of ([Zn(OBA) (BPDH)0.5]n·1.5DMF (TMU-5) enhanced the photocatalytic performance owing to the porosity, high surface area, and higher density of negative charges on Nb sites observed by N2 adsorption/desorption isotherms and XPS scans, respectively (Figures 9A,B). In addition, KNbO3@TMU-5 also exhibited good stability during the five-cycle test (Figure 9C).
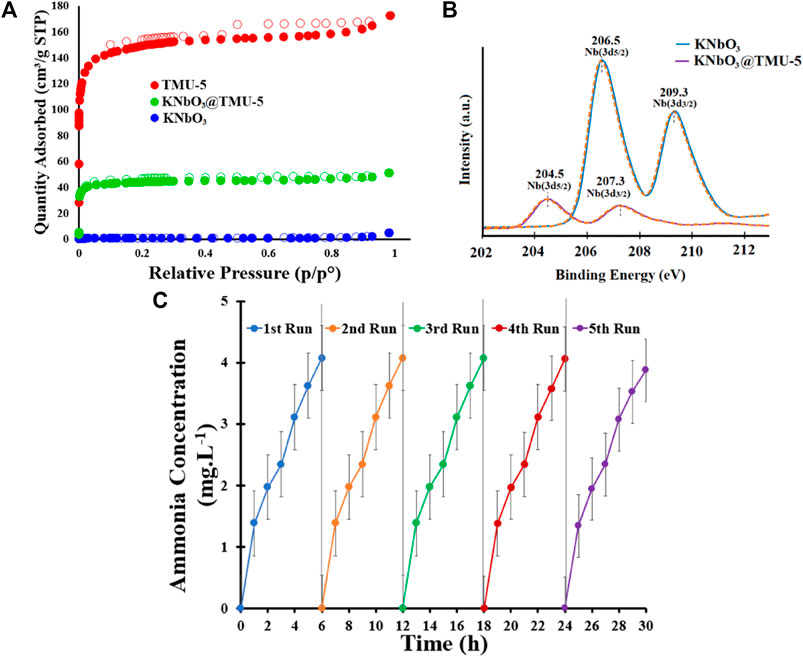
FIGURE 9. (A) N2 adsorption/desorption isotherms for the KNbO3@TMU-5 composite and its components. (B) Core-level XPS scans of Nb atoms of KNbO3 and KNbO3@TMU-5. (C) NH3 concentration versus cycling test on KNbO3@TMU-5. Reproduced from Chamack et al. (2022) with permission from American Chemical Society.
3.2.3 Polyoxometalates
Polyoxometalates (POMs) are a class of discrete inorganic polynuclear anionic molecular metal-oxo clusters composed of cations and polyoxometalate polyanions linked together by shared oxygen atoms to form closed 3-dimensional frameworks. Corner-sharing metal oxide polyhedra (MOx, x = 4, 5, and 6) are the basic building blocks, where M usually represents V, Nb, Mo, W, and Ta in high oxidation states (Yin et al., 2018; Gu et al., 2021; Horn et al., 2021). Semiconductor-like POMs or their constituent hybrids are considered as prodigious photocatalytic materials for NRR due to the following reasons: 1) the reversible gain or loss of a specific number of electrons and diverse active sites furnishing the reversible redox capability and modifiable stability; 2) the preponderance of POMs as an electron “reservoir”; 3) the well-defined HOMO–LUMO gap contributing to oxygen (ligand)-to-metal charge transfer; 4) definite particle sizes and dimensions together with the maintenance of structural intactness (Xiao-Hong Li et al., 2019; Xin Wang et al., 2020; Gu et al., 2021; Horn et al., 2021; Yuan Feng et al., 2022). Xiao et al. (Xiao et al., 2018) successfully covalently bonded the polyacid cluster [H4SiO40W12] (SiW12) to KOH-modified carbonitride graphite nanosheets through a phosphate bridging strategy. This allowed a rapid transfer of photogenerated electrons. The polyacid anion in POMs was suggested to function as an effective binder and electron transport chain, enhancing the interaction with the carrier and electron transport. Cyclic voltammograms of 30-SiW12/K-C3N4 showed that a reversible two-electron redox reaction occurred at −0.06 V and −0.36 V, implying that 30-SiW12/K-C3N4 could act as good containers of electrons and protons to provide protons for the N2 reduction. Integration of porous zeolitic imidazolate framework-67 (ZIF-67) (to enhance N2 adsorption) with various TM-substituted POMs (PMo12−xVx, x = 1, 2, 3, 8) (to supply multiple electrons), was investigated by Li et al. (Xiao-Hong Li et al., 2020). The light absorption of POMs was intensified to red shift by introducing V into POMs caused by the difference in the number of V hyperchromic effects (Figure 10A). The photocatalytic N2 reduction activity increased with increasing the number of V atoms with stronger redox ability than Mo (Figure 10B). The ZIF-67/POM composites attained higher catalytic performance than that of ZIF and POMs alone with the highest N2 fixation efficiency reaching 149.0 μmol L−1 h−1 and a STA efficiency of up to 0.032% for ZIF-67@PMo4V8. POMs not only improved the utilization of light energy but also easily excited electrons under light conditions to participate in the catalytic process (Figure 10C). Reduced POMs could be regenerated to form oxidized POMs in the presence of oxidants (such as O2), enabling a complete self-healing and circulatory system (Figure 10D).
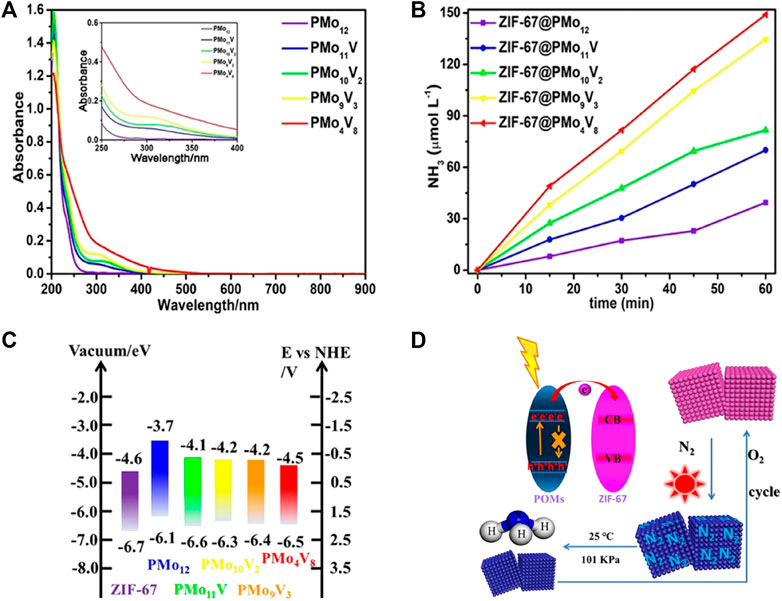
FIGURE 10. (A) UV/Vis absorption spectra of various V-substituted POMs. (B) NH3 yield as a function of reaction time on various ZIF-67/POMs hybrids. (C) Energy levels and (D) electron-transfer mechanism for ZIF-67/POMs hybrids. Reproduced from Hongda Li et al. (2020) with permission from the John Wiley and Sons.
Given the solubility of POM, there are recycling problems with environmental pollution. To overcome this issue, some strategies have been developed to reinforce its stability during NRR, including adhesive (Tianyu Wang et al., 2020), coupling with MOFs (Tianyu Wang et al., 2021), and interfacial interaction (Yuan Feng et al., 2022). For example, Su et al. (2022) fabricated SiW12 encapsulated Cr-MOFs (MIL-101(Cr)) hybrids, which were applied to N2 photocatalysis affording an NH3 yield rate of 75.56 μmol h−1 g−1, about 10 times that of Na4SiW12O40. SiW12 of polyoxometalates was supposed to be the active center, which could be regulated to locate in different cavities of MIL-101(Cr) by controlling the synthesis method. This enhanced the separation efficiency of photoexcited electron-hole pairs. The N2 reduction activity and photocatalyst structure did not change much after 5 cycles. The cooperative effect between the porous MIL-101(Cr) and SiW12 was hypothesized to boost the nitrogen fixation efficiency.
3.3 Transition metal chalcogenides
Transition metal chalcogenides (TMDs) have attracted heightened research interest for photocatalytic NRR primarily owing to their outstanding optical properties (wide spectral response range), relative nontoxicity, liquid media stability, superior electronic mobility, and intrinsic catalytic activity (Sun et al., 2018; Lei et al., 2020; Shen et al., 2020; Shen et al., 2021). Sun et al. (2017) presented the trion-induced NRR on ultrathin sulfur-vacancies (SVs)-rich 2D MoS2, where photoexcited electron-hole pairs combined the doping-induced charges to form trions, bound multiple electrons and located around the Mo sites in MoS2, which lowered thermodynamic barriers and favored the simultaneous six-electron transfer to produce NH3. N2 molecules were prone to be absorbed at the SV sites and activated by the electron-rich species to form NH3 over the SV-tuned ultrathin MoS2. A quasi-stable NH3 evolution rate of 325 μmol g−1 h−1 was attained without using any sacrificial agent or cocatalyst. Qin and coworkers employed a simple one-step hydrothermal method to prepare ultrathin alloyed Mo1–xWxS2 nanosheets with tunable hexagonal (2H)/trigonal (1T) phase ratios using Na2MoO4·2H2O, Na2WO4·2H2O, and thiourea as Mo, W, and S precursors, respectively. Phase engineering and appropriate W doping markedly boosted the N2 photoreduction efficiency. As shown in Figures 11A, the alloys maintained a layer structure during the hydrothermal process, and the 1T, 2H, and polymorph structural domains were also observed in the alloyed Mo1–xWxS2 nanosheets. The alloyed Mo1–xWxS2 nanosheets with a 1T phase concentration of 33.6% and Mo/W of 0.68:0.32 (MWS-2) were found to reach the maximal N2 fixation rate of about 111 μmol g−1 h−1 under visible light, 3.7 (or 3)-fold higher than that of pristine MoS2 (or WS2). DFT calculations revealed that N2 had the highest negative adsorption energy on 1T Mo1–xWxS2 compared to that on 1T MoS2, 2H MoS2, and 2H Mo1–xWxS2 (Figure 11B). Meanwhile, in situ N2 absorption XANES techniques interpreted the energy shift on the peaks of the Mo K-edge as the ascending valence states of Mo, and that of W L-edge as a higher electron density state in W 5d orbitals, resulting in the migration of many electrons from Mo to W. Based on theoretical calculations and photochemical experiments, W doping and the 2H/1T structure were supposed to synergistically enhance the N2 adsorption. Binary and ternary metal sulfide-based composites were shown to facilitate photocatalytic NH3 synthesis via intimate heterointerfaces to diminish charge recombination (Dong Liu et al., 2021). Reported systems in this regard include MoS2/C-ZnO (Xing et al., 2018), C3N4/MoS2/Mn3O4 (Gui Li et al., 2021), WS2@TiO2 (Shi et al., 2020b), and MoS2/MgIn2S4 (Swain et al., 2020).
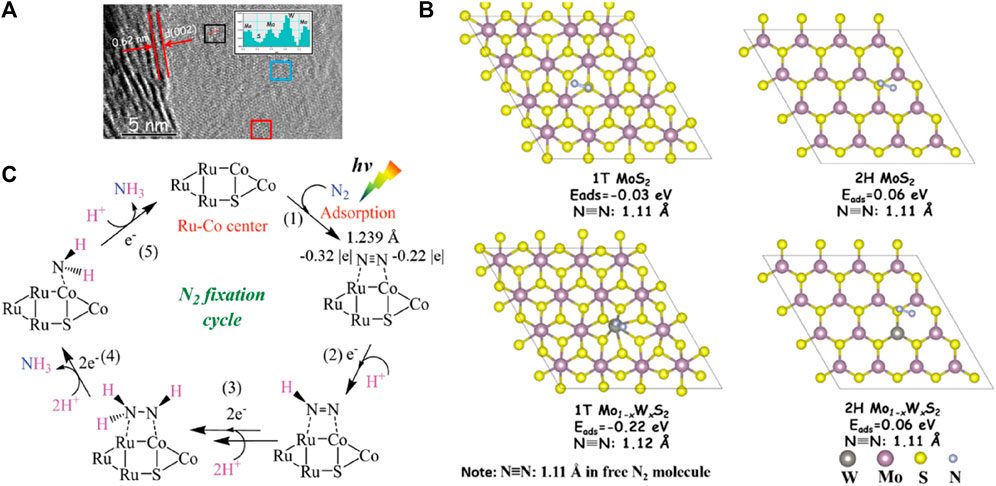
FIGURE 11. (A) HRTEM image of MWS-2 nanosheets. The red and blue squares represent the 2H and 1T structures, respectively; (B) Different adsorption energies of N2 molecules on 1T MoS2, 1T Mo1−xWxS2, 2H MoS2, and 2H Mo1−xWxS2. Reproduced from Qin et al. (2021) with permission from American Chemical Society. (C) Proposed NRR reaction schemes on Ru-SV-CoS/CN. Reproduced from Yuan et al. (2020) with permission from the John Wiley and Sons.
Sparked by the nitrogenase MoFe-based protein, the reduction of N2 on Fe single-atom-modified MoS2 nanosheet photocatalyst was first theoretically predicted by Azofra et al. (Azofra et al., 2017). Following this, various photocatalyst systems entailing Fe supported on MoS2 have emerged. As an example, Zheng et al. (Zheng et al., 2021) developed Fe-decorated 2D MoS2 photocatalysts [Fe-S2-Mo] mimicking FeMoco in nature for NH3 synthesis. A solar-to-NH3 energy-conversion efficiency of 0.24% at 270°C was achieved, representing the highest efficiency among all reported photocatalytic systems thus far. The HAADF-STEM images and simulation results jointly supported that Fe atoms were favorably situated on the atop sites of Mo rather than substituted Mo sites to form inorganic Fe-S2-Mo motifs analogous [Fe–S2–Mo] unit in FeMoco. Theoretical calculations suggested that the HOMO and LUMO orbitals were concentrated on the edge of single-layered MoS2 (sMoS2) with relatively low electron delocalization, indicating the active edge sites of sMoS2. Fe doping distinctly improved their LUMO orbital delocalization degree. Further in situ attenuated total reflection FTIR and the energy plots revealed that the NRR on Fe1 over [Fe–S2–Mo] followed an alternating pathway, showing similarity for both non-biological and biological processes regarding NRR mechanism. Excited electrons could be transferred from the VB to the CB of sMoS2 via the conductive Fe–S2–Mo motifs and reside on the Fe atom during the photoexcitation process to enter into the anti-bonding orbital of an adsorbed N2 molecule, which thus facilitated the hydrogenation reaction of N2 for ammonia production. Similarly, a biomimetic “MoFe cofactor” (the Fe3+/Fe2+ and Mo6+/Mo4+ redox couples) was introduced in MoTe2 nanosheets to facilitate the transport and separation of photo-generated charge carriers by one-electron and two-electron redox reactions with 15 times longer photocarrier lifetime after Fe doping and about 11 times higher NH3 production rate of Fe-doped MoTe2 than that of pure MoTe2 (Hongda Li et al., 2020). An enzymatic-analogous N2-fixation mechanism was also demonstrated on a bimetallic Ru–Co center at Ru/CoSx interface on g-C3N4 sheets by Ru deposition near CoSx induced by S vacancies (Figure 11C). The side-on bridging of N2 on under-coordinated Ru–Co center at Ru/CoSx interface led to high polarization and strong activation of N2, resulting in an AQE of 1.28% at 400 nm and a STA efficiency of 0.042% for NH3 production in pure water.
3.4 Biomimetic photocatalysts
Biological nitrogen fixation has advantages of low energy consumption and high NH3 yield, while multiple adenosine 5′-triphosphate (ATP) hydrolysis electron transfer steps are required per reduced N2 molecule, resulting in modest overall reaction kinetics and slow NH3 synthesis rate. Under such circumstance, exploration of biomimetic systems of molecular analogs to simulate and optimize this process has gained a tremendous interest in scientific community (Meng et al., 2021). Taking advantage of iron molybdenum sulfide chalcogels, Kanatzidis et al. (Banerjee et al., 2015) proposed a nitrogen-fixing biomimetic system by replacing MoFe-based proteins, which is the active site of nitrogenase, with Fe2Mo6S8 chalcogel interconnected through [Sn2S6]4− ligands (Figure 12A). Featuring strong visible-light-absorbing, high spatial density of active sites, and multielectron transformations, the chalcogel-based amorphous framework could effectively convert N2 to NH3 in aqueous media under light illumination. Although its turnover number (TON) was not appealing, this study proved that cluster compounds analogous to nitrogenase can confer cogent catalysis and better stability than nitrogenase. Brown et al. (Brown et al., 2016) employed CdS to photosensitize MoFe proteins by harvesting light energy to replace ATP hydrolysis to drive the enzymatic N2 fixation, with peak NH3 production rates of 315 ± 55 nmol NH3 mg(MoFe protein)−1 min−1 at a TOF of 75 min−1 (Figures 12B,C). N2 reduction persisted for up to 5 h under constant illumination with a TON of 1.1 × 104 mol NH3 mol(MoFe protein)−1. This study indicates that bio-nanocomposites can function as photocatalysts for solar-powered generation of NH3 with TOF comparable to nitrogenase. Inspired by the above works, Liu et al. (Liu et al., 2016) designed a redox-active FeMoS–FeS–SnS chalcogel system consisting of Fe2Mo6S8(SPh)3 and Fe3S4 biomimetic clusters linked by Sn2S6 to reduce N2 to NH3 (Figure 12D). All FeMoS–M–SnS chalcogels constructed by replacing Fe4S4 clusters with redox-inert ions Sb3+, Sn4+, Zn2+, exhibited effective NRR performance. FeMoS with FeS clusters was observed to strengthen NH3 production over FeMoS alone and Fe4S4-only chalcogel (FeS–SnS) and provided higher efficiency than that of [Mo2Fe6S8(SPh)3]-containing chalcogels. Therefore, Fe was believed to be more conducive than Mo for N2 binding (Figure 12E). The active sites in the Fe-containing sulfide clusters were considered to differ (i.e., based mainly on Fe) from that in the nitrogenase enzymes (based on both Mo and Fe). Quantitative isotope labeling and in situ DRIFTS corroborated the origin of detected NH3 from N2 (Figure 12F).
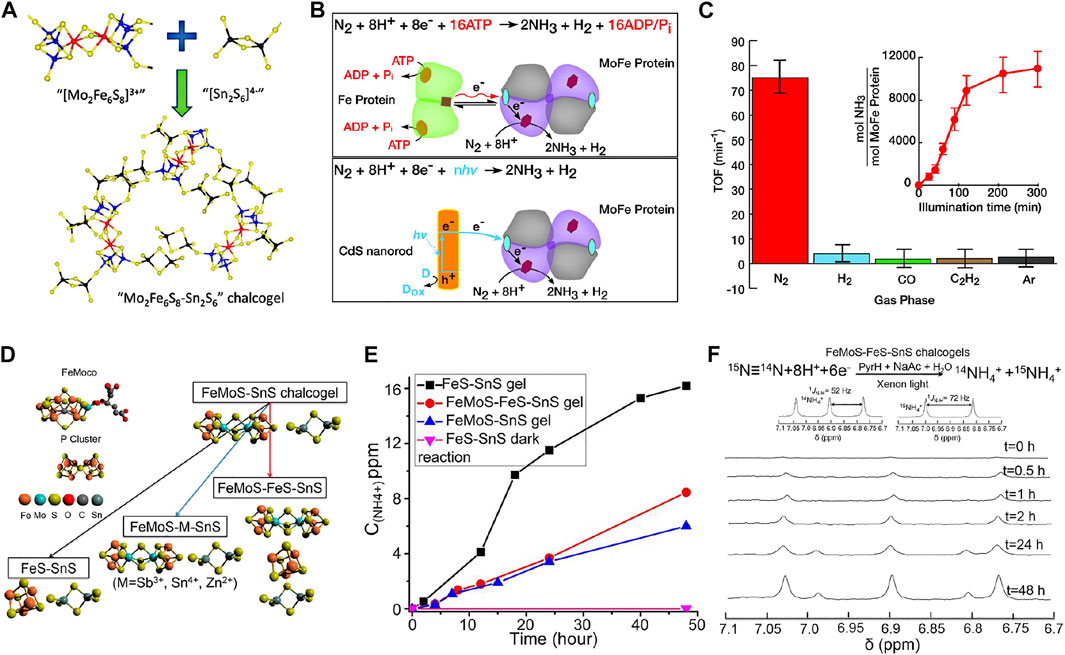
FIGURE 12. (A) Schematic of synthesis of Mo2Fe6S8–Sn2S6 biomimetic chalcogel (FeMoS-chalcogel) (Mo in blue; Fe in red; S in yellow; Sn in black). Reproduced from Banerjee et al. (2015) with permission from American Chemical Society. (B) Reaction scheme for photocatalytic NRR to NH3 by nitrogenase (top) and the CdS:MoFe protein biohybrids (below). (C) Photocatalytic NRR to NH3 on CdS:MoFe protein biohybrids under a 100% N2 atmosphere, 10% of either H2, CO, or C2H2 in a bulk phase of 90% N2. Reproduced from Brown et al. (2016) with permission from American Association for the Advancement of Science. (D) Model structures of the FeMo cofactor and P cluster, and the synthetic routes towards the assembly of different chalcogels. (E) Comparisons of photocatalytic NRR performance for different biomimetic chalcogels. (F) Isotope labeling experiment results detected by NMR. Reproduced from Liu et al. (2016) with permission from National Academy of Sciences of the United States of America.
3.5 Metal-organic frameworks (MOFs)
MOFs are micro-mesoporous hybrid materials composed of metal ion nodes connected with organic linkers together or clusters and organic frameworks. Similar excitation characteristics of electron-hole pairs endow MOFs and their derivatives with intriguing semiconductor-like properties in various photochemical reactions (Hu et al., 2022). High microporosity and diverse functionalities enable the introduction of defined and highly exposed metal nodes onto the larger surfaces to promote or catalyze targeted reactions (Hao et al., 2021; Lin Wang et al., 2022). Especially, these exposed coordinatively unsaturated metal sites not only contribute to higher catalytic activities, but also behave as Lewis acid sites to withdraw p electrons from N2 molecules and weaken the N≡N triple-bonds, accounting for photochemical N2 reduction (Fu et al., 2022). Chen et al. (2021b) exploited gas-permeable MOF substrates (i.e., UiO-66) to not only serve as a stable matrix to confine the surface-clean gold nanoparticles (AuNPs) with high dispersity, but also ensure the accessibility of these AuNPs to both N2 molecules and (hydrated) protons (Figure 13A), enabling direct plasmonic NRR with high efficiency. The porosity of the MOF matrix facilitated mass transport of reactants and products, promoting the total reaction rate (Chen et al., 2022; Guanhua Zhang et al., 2022). Accordingly, the NH3 evolution rates on porous Au@MOFs particles were superior to those of nonporous particles at the specially designed gas–membrane–solution (GMS) reaction interface, and the GMS system was better than the powder-in-solution (PiS) system (Figure 13B). In another work, Guo et al. (Guo et al., 2022) investigated the [Zr6O6] cluster effect and the leading role of photoelectrons over the protonation of nitrogen by using an N-free dehydrated Zr-based MOF, UiO-66(SH)2 (Figure 13C). The UV/Vis diffuse reflectance spectrum combined with theory studies suggested that the introduction of thiol groups (–SH) caused an absorption edge of UiO-66(SH)2 deep into the visible region. The hopping process of the photoelectron from VB to the unoccupied Zr-4days is dominated by the ligand-to-metal charge transfer (LMCT) (Figure 13D). The dehydration opened a “gate” for the entry of N2 molecules into the [Zr6O6] cluster, of which three active cage modes strongly bound with N2 molecules and drive the cleavage of N≡N bond by the photoelectrons (Figure 13E). However, most MOF-related photocatalysts focused either on the coordination environment around the metal nodes, or the role of photosensitive ligands or single transition metals (Hu et al., 2022). Gao and co-authors presented photo-excited cluster defects and linker defects to revamp the photocatalytic NRR performance of UiO-66 (Gao et al., 2021). They shows the performance under alternate UV-Vis and visible light irradiation and after subsequent post-synthetic ligand exchange (PSE) process. Compared to UV-Vis light, the performance of the second test under visible light did not improve. After the PSE process that repaired linker defects instead of cluster defects, activity was restored under UV-Vis light. This demonstrated that photo-induced defects can only be created by UV light, where linker defects played a critical role in improving the performance due to the Zr nodes with unsaturated coordination induced by linker defects being more conducive to photo-driven NH3 synthesis.
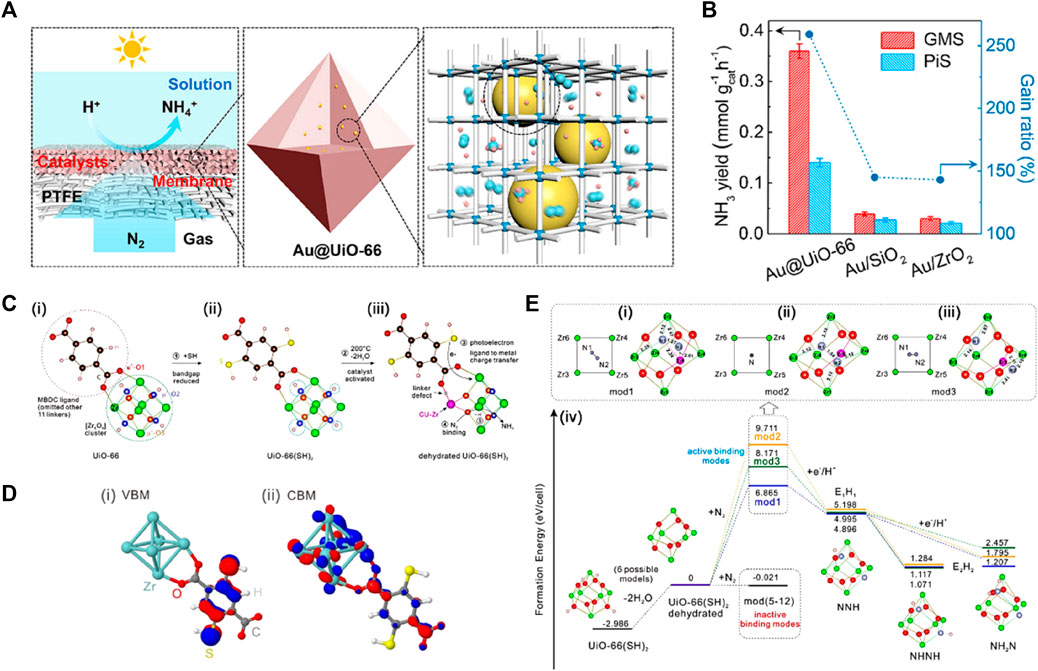
FIGURE 13. (A) Schematic illustration for direct NRR on Au encapsulated in UiO-66 matrix. (B) NH3 yield on powder (in the PiS system) and membrane (in the GMS system) catalysts consisting of Au@UiO-66, Au/SiO2, and Au/ZrO2. Reproduced from Chen et al. (2021b) with permission from American Chemical Society. (C) Schematic evolution of the partial structure of UiO66, UiO-66(SH)2 and the dehydrated UiO-66(SH)2. (D) The calculated band orbitals for VBM and CBM of UiO-66(SH)2 at Γ point. (E) The three active modes (mod 1–3) with each centerpiece configuration of the [Zr6O6] cluster encaging N2 molecule and their corresponding formation energy diagrams. Reproduced from Guo et al. (2022) with permission from the John Wiley and Sons.
Designing and constructing photocatalysts with bimetallic active centers is an effective way for nitrogen photofixation (Zhen Zhao et al., 2022). An et al. (An et al., 2021) designed a series of modularized UiO-66-based MOFs, U(Zr-Hf)-X, with bimetallic Zr-Hf nodes and functionalized ligands, and demonstrated a tandem ligand-to-metal-to-metal electron transfer (LMMET) pathway. By independently manipulating the Zr/Hf molar ratio and substituent group of TPA, the optimal U (0.5Hf)-2SH (metal nodes: 0.5Zr: 0.5Hf; linkers: TPA-2SH) yielded an NH3 production rate up to 116.1 μmol h−1 g−1 under visible light. This was attributed to the broadening of the absorption spectrum to visible light by –2SH modification according to combined experiment-theory results. Although these results suggested that the synergistic effect of bimetallic Zr-Hf nodes was favorable for NRR performance, it is uncertain whether the TPA-2SH ligand contributed to charger transfer mechanism. The proposed NRR pathway of U (0.5Hf)-2SH was explored. The one-step two-photon excitation path strides across the uphill process of the electron transfer from TPA-2SH ligands to metal nodes in UiO-66. The Hf species served as an electron buffer tank to optimize the electron transfer, while the Zr species acted as the catalytic active site due to the difference in electrode potentials between Zr–O and Hf–O clusters. Meanwhile, Zr-dominated π-backbonding mechanism weakened the N≡N bond in absorbed N2 molecules via the electron transfer synergy to generate NH3. Nonetheless, research on photocatalytic NRR using MOFs is relatively rare, the existing MOF-based photocatalysts have still an exemplary significance regarding constructing other visible-light-driven MOF-based NRR photocatalysts.
4 Conclusions and future remarks
The photocatalytic NRR is an appealing alternative to the current industrial thermocatalytic Haber-Bosch process for NH3 production. It only requires solar energy and water, rendering the process environmentally friendly and highly sustainable. With a general awareness of emphasizing on green chemistry and sustainable development, this field is bound to have a significant and far-reaching impact on how humans understand and engage in the nitrogen cycle. Despite recent progresses that have been achieved in the field of photocatalytic NH3 synthesis, it is still in its infancy. A comparable conversion efficiency to the century-old Haber-Bosch process remains unsolved, especially on how to accentuate N2 activation and accelerate the kinetics of electron transfer to N2. Continuous efforts in the design and development of nontoxic, efficient, stable and low-cost catalysts minimizing the consumption of noble metals have obtained some positive advancements. Tungsten and related metal-based materials have shown enormous accolades as photocatalysts for NRR. However, there still remain many challenges to be addressed as follows:
1) The intrinsic mechanism and kinetic control of nitrogen fixation are still ambiguous and complex, warranting further investigations. Because of the complex multi-electron reactions involved and the presence of various intermediate species, combination of multiple accurate analytical methods and advanced characterization techniques are required. It is essential to develop in-situ techniques to study the adsorption structure of N2 and key intermediates on the surface of catalytic materials, providing useful information on the catalytic active center and its dynamic evolution during the reaction process.
2) Various environmental factors (such as cation effect, electric field effect, pH, hydrophobicity, and actual active site, etc.) (Zhou et al., 2022) can profoundly affect the photocatalytic NRR process. It is thus necessary to explore and clarify the synergistic effect among the semiconductor photocatalysts and mixed solvent systems to further reveal the multi-effects of collaborative catalytic mechanism.
3) Equally importantly, the cooperative mechanisms among heterostructure, element doping and interaction between components and role of each individual strategy in N2 photocatalysis remain to be elucidated. It is necessary to comprehensively use various design and control strategies of catalysts to combine element doping, defect construction, and structural design to increase active sites, suppress the recombination of photogenerated carriers, and enhance the adsorption and activation of nitrogen molecules in the NRR process.
4) Efficient utilization of solar energy is the focus of interest in photocatalysis. However, the utilization of light in the NIR and the far-infrared region above 1,000 nm for NRR photocatalysis is poor. To facilitate the practical utilization of NRR photocatalysis, the design of suitable photocatalysts for wide-range light harvest from ultraviolet to near-infrared regions is important. This may be realized by integration with NIR and even far-infrared responsive materials such as dye molecules and black phosphorus (Zhang et al., 2017), narrow band gap NIR harvesters as well as materials having surface plasmon resonance effect.
5) The extensive use of sacrificial agents leads to additional costs, which deteriorate the overall affordability of the environment. Therefore, some cheap sacrificial agents at this stage, such as starch, biomass, plastics, wastewater, etc., could also be developed as sacrificial agents to further reduce the cost of large-scale application of N2 photocatalysis.
6) The attained SCE of N2 photoreduction is still less than 1%, far below the minimum standard of 10% needed to realize industrialization, and the stability during the reaction process is however still much below the requirements for long-term practical applications.
7) The photostability of semiconductor catalysts is another adverse issue that deserves much attention. The surfaces of many n-type and p-type semiconductors (such as sulphides) are susceptible to decomposition by photogenerated holes or electrons, decreasing the lifetimes of the photocatalysts. To address this issue, strategies such as coating with a second phase, surface passivation and functionalization and incorporation with cocatalysts can be employed (Sun et al., 2018).
To date, major endeavors in materials engineering toward N2 photocatalysis have been made on traditional semiconductors. Some emerging photocatalytic materials such as MOFs and covalent organic frameworks (COFs) have not been fully explored for photocatalytic N2 reduction. Meanwhile, the design of cation defects and further combined defects and investigation of their roles in MOFs in N2 reduction are rarely conducted, which deserves further studies. To synergistically promote N2 photocatalysis, integration of multiple design strategies (e.g., defect engineering and other modification strategies such as creation of Z-scheme heterostructures to separate ammonia production and water oxidation sites in space) is preferred. Additionally, combination of material engineering and external fields (e.g., microwaves, mechanical stress, temperature gradient, electric field, magnetic field, and coupled fields) is another promising strategy to further boost photocatalytic N2 reduction reactions.
Current research focuses mainly on the microstructure design of materials and the improvement of photocatalytic performance, while less attention was paid on the development of photocatalytic devices suitable for future applications and reliable cost accounting. The bridge between laboratory research and practical application development is crucial. To better understand the conditions required to reach practical industrial applications, we should compare photocatalytic NRR with current industrial Haber-Bosch reactions from various perspectives, such as reaction temperature and pressure, ammonia/nitrate output, pollution, etc. In an actual photoreactor, N2 would be separated from the air using standard cryogenic separation or membrane processes and would need to be recovered and recycled for cost reasons. Current Photocatalytic NRR is more carried out in aqueous solutions, which means that liquid-phase products can be directly obtained to produce liquid fertilizers that can be directly used and sold, but the subsequent separation of catalysts will further increase the energy cost of the entire process. Even if the final product is anhydrous ammonia for fuel feedstock, the purification of low concentrations of NH3 from the N2 stream adds to the energy-consuming step. Therefore, how to achieve higher product concentrations in flowing gas setups is an important target of this particular approach. From another point of view, this idea guides us to no longer pay attention to the NH3 yield as an indicator, but to focus on the integration of upstream synthesis and downstream applications to reduce energy consumption and pollution in the entire production process, to achieve the final goal of “carbon neutrality” faster.
Author contributions
All authors listed have made a substantial, direct, and intellectual contribution to the work and approved it for publication.
Funding
This work was supported by the National Natural Science Foundation of China (No. 21972010) and the Beijing Natural Science Foundation (No. 2192039).
Conflict of interest
The authors declare that the research was conducted in the absence of any commercial or financial relationships that could be construed as a potential conflict of interest.
Publisher’s note
All claims expressed in this article are solely those of the authors and do not necessarily represent those of their affiliated organizations, or those of the publisher, the editors and the reviewers. Any product that may be evaluated in this article, or claim that may be made by its manufacturer, is not guaranteed or endorsed by the publisher.
Supplementary material
The Supplementary Material for this article can be found online at: https://www.frontiersin.org/articles/10.3389/fchem.2022.978078/full#supplementary-material
References
Ahmed, M. I., Chen, S., Ren, W., Chen, X., and Zhao, C. (2019). Synergistic bimetallic CoFe2O4 clusters supported on graphene for ambient electrocatalytic reduction of nitrogen to ammonia. Chem. Commun. 55 (81), 12184–12187. doi:10.1039/C9CC05684J
An, K., Ren, H., Yang, D., Zhao, Z., Gao, Y., Chen, Y., et al. (2021). Nitrogenase-inspired bimetallic metal organic frameworks for visible-light-driven nitrogen fixation. Appl. Catal. B Environ. 292, 120167. doi:10.1016/j.apcatb.2021.120167
Andersen, S. Z., Colic, V., Yang, S., Schwalbe, J. A., Nielander, A. C., McEnaney, J. M., et al. (2019). A rigorous electrochemical ammonia synthesis protocol with quantitative isotope measurements. Nature 570 (7762), 504–508. doi:10.1038/s41586-019-1260-x
Azofra, L. M., Sun, C., Cavallo, L., and MacFarlane, D. R. (2017). Feasibility of N2 binding and reduction to ammonia on Fe-deposited MoS2 2D sheets: A DFT study. Chem. Eur. J. 23 (34), 8275–8279. doi:10.1002/chem.201701113
Bai, J., Chen, X., Xi, Z. Y., Wang, X., Li, Q., Hu, S. Z., et al. (2017). Influence of solvothermal post-treatment on photochemical nitrogen conversion to ammonia with g-C3N4 Catalyst. Acta Phys. -Chim. Sin. 33 (3), 611–619. doi:10.3866/pku.Whxb201611102
Bai, H., Lam, S. H., Yang, J., Cheng, X., Li, S., Jiang, R., et al. (2022). A Schottky-barrier-free plasmonic semiconductor photocatalyst for nitrogen fixation in a “one-stone-two-birds” manner. Adv. Mat. 34 (2), 2104226. doi:10.1002/adma.202104226
Banerjee, A., Yuhas, B. D., Margulies, E. A., Zhang, Y., Shim, Y., Wasielewski, M. R., et al. (2015). Photochemical nitrogen conversion to ammonia in ambient conditions with FeMoS-chalcogels. J. Am. Chem. Soc. 137 (5), 2030–2034. doi:10.1021/ja512491v
Bao, L., Yuan, Y.-j., Zhang, H., Zhang, X., and Xu, G. (2021). Understanding the hierarchical behavior of Bi2WO6 with enhanced photocatalytic nitrogen fixation activity. Dalton Trans. 50 (21), 7427–7432. doi:10.1039/D1DT00762A
Bazhenova, T. A., and Shilov, A. E. (1995). Nitrogen fixation in solution. Coord. Chem. Rev. 144, 69–145. doi:10.1016/0010-8545(95)01139-G
Bo, Y., Wang, H., Lin, Y., Yang, T., Ye, R., Li, Y., et al. (2021). Altering hydrogenation pathways in photocatalytic nitrogen fixation by tuning local electronic structure of oxygen vacancy with dopant. Angew. Chem. Int. Ed. 60 (29), 16085–16092. doi:10.1002/anie.202104001
Brown, K. A., Harris, D. F., Wilker, M. B., Rasmussen, A., Khadka, N., Hamby, H., et al. (2016). Light-driven dinitrogen reduction catalyzed by a CdS: Nitrogenase MoFe protein biohybrid. Science 352 (6284), 448–450. doi:10.1126/science.aaf2091
Cai Feng, C., Wu, P., Li, Q., Liu, J., Wang, D., Liu, B., et al. (2022). Amorphization and defect engineering in constructing ternary composite Ag/PW10V2/am-TiO2−x for enhanced photocatalytic nitrogen fixation. New J. Chem. 46 (4), 1731–1740. doi:10.1039/D1NJ05917C
Caiting Feng, C., Liu, J., Li, Q., Ji, L., Wu, P., Yuan, X., et al. (2022). Fabricating Ag/PW12/Zr-mTiO2 composite via doping and interface engineering: An efficient catalyst with bifunctionality in photo- and electro-driven nitrogen reduction reactions. Adv. Sustain. Syst. 6 (1), 2100307. doi:10.1002/adsu.202100307
Cao, S., Chen, H., Jiang, F., and Wang, X. (2018). Nitrogen photofixation by ultrathin amine-functionalized graphitic carbon nitride nanosheets as a gaseous product from thermal polymerization of urea. Appl. Catal. B Environ. 224, 222–229. doi:10.1016/j.apcatb.2017.10.028
Chamack, M., Ifires, M., Akbar Razavi, S. A., Morsali, A., Addad, A., Larimi, A., et al. (2022). Photocatalytic performance of perovskite and metal-organic framework hybrid material for the reduction of N2 to ammonia. Inorg. Chem. 61 (3), 1735–1744. doi:10.1021/acs.inorgchem.1c03622
Chao Liu, C., Zhang, Q., Hou, W., and Zou, Z. (2020). 2D titanium/niobium metal oxide-based materials for photocatalytic application. Sol. RRL 4 (9), 2000070. doi:10.1002/solr.202000070
Chen, W. T., Chan, A., Al-Azri, Z. H. N., Dosado, A. G., Nadeem, M. A., Sun-Waterhouse, D., et al. (2015). Effect of TiO2 polymorph and alcohol sacrificial agent on the activity of Au/TiO2 photocatalysts for H2 production in alcohol–water mixtures. J. Catal. 329, 499–513. doi:10.1016/j.jcat.2015.06.014
Chen, X., Li, N., Kong, Z., Ong, W.-J., and Zhao, X. (2018). Photocatalytic fixation of nitrogen to ammonia: State-of-the-art advancements and future prospects. Mat. Horiz. 5 (1), 9–27. doi:10.1039/c7mh00557a
Chen, F., Huang, H., Guo, L., Zhang, Y., and Ma, T. (2019). The role of polarization in photocatalysis. Angew. Chem. Int. Ed. 58 (30), 10061–10073. doi:10.1002/anie.201901361
Chen, L., Dai, X., Li, X., Wang, J., Chen, H., Hu, X., et al. (2021a). A novel Bi2S3/KTa0.75Nb0.25O3 nanocomposite with high efficiency for photocatalytic and piezocatalytic N2 fixation. J. Mat. Chem. A 9 (22), 13344–13354. doi:10.1039/D1TA02270A
Chen, L., Hao, Y.-C., Guo, Y., Zhang, Q., Li, J., Gao, W.-Y., et al. (2021b). Metal-organic framework membranes encapsulating gold nanoparticles for direct plasmonic photocatalytic nitrogen fixation. J. Am. Chem. Soc. 143 (15), 5727–5736. doi:10.1021/jacs.0c13342
Chen, C., Sun, M., Wang, K., and Li, Y. (2022). Dual-metal single-atomic catalyst: The challenge in synthesis, characterization, and mechanistic investigation for electrocatalysis. SmartMat. doi:10.1002/smm2.1085
Chen-Xuan Xu, C. X., Huang, J. D., and Ma, J. M. (2021). Green, cheap and rechargeable Al–N2 battery with efficient N2 fixation. Rare Met. 40 (1), 1–2. doi:10.1007/s12598-020-01626-8
Cheng, Q., and Zhang, G.-K. (2020). Enhanced photocatalytic performance of tungsten-based photocatalysts for degradation of volatile organic compounds: A review. Tungsten 2 (3), 240–250. doi:10.1007/s42864-020-00055-5
Choi, J., Suryanto, B. H. R., Wang, D., Du, H. L., Hodgetts, R. Y., Ferrero Vallana, F. M., et al. (2020). Identification and elimination of false positives in electrochemical nitrogen reduction studies. Nat. Commun. 11 (1), 5546. doi:10.1038/s41467-020-19130-z
Chu, K., Liu, Y.-p., Li, Y.-b., Zhang, H., and Tian, Y. (2019). Efficient electrocatalytic N2 reduction on CoO quantum dots. J. Mat. Chem. A 7 (9), 4389–4394. doi:10.1039/C9TA00016J
Comer, B. M., and Medford, A. J. (2018). Analysis of photocatalytic nitrogen fixation on rutile TiO2 (110). ACS Sustain. Chem. Eng. 6 (4), 4648–4660. doi:10.1021/acssuschemeng.7b03652
Congmin Zhang, C., Chen, G., Lv, C., Yao, Y., Xu, Y., Jin, X., et al. (2018). Enabling nitrogen fixation on Bi2WO6 photocatalyst by c-PAN surface decoration. ACS Sustain. Chem. Eng. 6 (9), 11190–11195. doi:10.1021/acssuschemeng.8b02236
Cooper, J. K., Gul, S., Toma, F. M., Chen, L., Glans, P.-A., Guo, J., et al. (2014). Electronic structure of monoclinic BiVO4. Chem. Mat. 26 (18), 5365–5373. doi:10.1021/cm5025074
Dai, Z., Qin, F., Zhao, H., Ding, J., Liu, Y., Chen, R., et al. (2016). Crystal defect engineering of Aurivillius Bi2MoO6 by Ce doping for increased reactive species production in photocatalysis. ACS Catal. 6 (5), 3180–3192. doi:10.1021/acscatal.6b00490
Dimitrijevic, N. M., Vijayan, B. K., Poluektov, O. G., Rajh, T., Gray, K. A., He, H., et al. (2011). Role of water and carbonates in photocatalytic transformation of CO2 to CH4 on titania. J. Am. Chem. Soc. 133 (11), 3964–3971. doi:10.1021/ja108791u
Dong Liu, D., Chen, S., Li, R., and Peng, T. (2021). Review of Z-scheme heterojunctions for photocatalytic energy conversion. Acta Phys. -Chim. Sin. 37 (6), 2010017. doi:10.3866/pku.Whxb202010017
Dong Zhang, D., Li, Y., Li, Y., and Zhan, S. (2022). Towards single-atom photocatalysts for future carbon-neutral application. SmartMat. doi:10.1002/smm2.1095
Fan, Y. S., Xi, X. L., Liu, Y. S., Nie, Z.-R., Zhao, L. Y., Zhang, Q. H., et al. (2021). Regulation of morphology and visible light-driven photocatalysis of WO3 nanostructures by changing pH. Rare Met. 40 (7), 1738–1745. doi:10.1007/s12598-020-01490-6
Fei, T., Yu, L., Liu, Z., Song, Y., Xu, F., Mo, Z., et al. (2019). Graphene quantum dots modified flower like Bi2WO6 for enhanced photocatalytic nitrogen fixation. J. Colloid Interface Sci. 557, 498–505. doi:10.1016/j.jcis.2019.09.011
Feng Wang, F., Ding, Q., Bai, Y., Bai, H., Wang, S., Fan, W., et al. (2022). Fabrication of an amorphous metal oxide/p-BiVO4 photocathode: Understanding the role of entropy for reducing nitrate to ammonia. Inorg. Chem. Front. 9 (4), 805–813. doi:10.1039/D1QI01472B
Fu, W., Zhuang, P., OliverLam Chee, M., Dong, P., Ye, M., Shen, J., et al. (2019). Oxygen vacancies in Ta2O5 nanorods for highly efficient electrocatalytic N2 reduction to NH3 under ambient conditions. ACS Sustain. Chem. Eng. 7 (10), 9622–9628. doi:10.1021/acssuschemeng.9b01178
Fu, Y., Liao, Y., Li, P., Li, H., Jiang, S., Huang, H., et al. (2022). Layer structured materials for ambient nitrogen fixation. Coord. Chem. Rev. 460, 214468. doi:10.1016/j.ccr.2022.214468
Gaggero, E., Calza, P., Cerrato, E., and Paganini, M. C. (2021). Cerium-europium- and erbium-modified ZnO and ZrO2 for photocatalytic water treatment applications: A review. Catalysts 11 (12), 1520. doi:10.3390/catal11121520
Gambarotta, S., and Scott, J. (2004). Multimetallic cooperative activation of N2. Angew. Chem. Int. Ed. 43 (40), 5298–5308. doi:10.1002/anie.200301669
Gao, X., Wen, Y., Qu, D., An, L., Luan, S., Jiang, W., et al. (2018). Interference effect of alcohol on Nessler’s reagent in photocatalytic nitrogen fixation. ACS Sustain. Chem. Eng. 6 (4), 5342–5348. doi:10.1021/acssuschemeng.8b00110
Gao, Y., Han, Z., Hong, S., Wu, T., Li, X., Qiu, J., et al. (2019). ZIF-67-derived cobalt/nitrogen-doped carbon composites for efficient electrocatalytic N2 reduction. ACS Appl. Energy Mat. 2 (8), 6071–6077. doi:10.1021/acsaem.9b01135
Gao, W., Li, X., Zhang, X., Su, S., Luo, S., Huang, R., et al. (2021). Photocatalytic nitrogen fixation of metal–organic frameworks (MOFs) excited by ultraviolet light: Insights into the nitrogen fixation mechanism of missing metal cluster or linker defects. Nanoscale 13 (16), 7801–7809. doi:10.1039/D1NR00697E
Gao, Y., Xia, Q., Hao, L., Robertson, A. W., and Sun, Z. (2022). Design of porous core–shell manganese oxides to boost electrocatalytic dinitrogen reduction. ACS Sustain. Chem. Eng. 10 (3), 1316–1322. doi:10.1021/acssuschemeng.1c07824
Garden, A. L., and Skúlason, E. (2015). The mechanism of industrial ammonia synthesis revisited: Calculations of the role of the associative mechanism. J. Phys. Chem. C 119 (47), 26554–26559. doi:10.1021/acs.jpcc.5b08508
Girish Kumar, S., and Koteswara Rao, K. S. R. (2015). Tungsten-based nanomaterials (WO3 & Bi2WO6): Modifications related to charge carrier transfer mechanisms and photocatalytic applications. Appl. Surf. Sci. 355, 939–958. doi:10.1016/j.apsusc.2015.07.003
Gu, J., Chen, W., Shan, G. G., Li, G., Sun, C., Wang, X. L., et al. (2021). The roles of polyoxometalates in photocatalytic reduction of carbon dioxide. Mat. Today Energy 21, 100760. doi:10.1016/j.mtener.2021.100760
Guanhua Zhang, G., Yuan, X., Xie, B., Meng, Y., Ni, Z., Xia, S., et al. (2022). S vacancies act as a bridge to promote electron injection from Z-scheme heterojunction to nitrogen molecule for photocatalytic ammonia synthesis. Chem. Eng. J. 433, 133670. doi:10.1016/j.cej.2021.133670
Gui Li, G., Yang, W., Gao, S., Shen, Q., Xue, J., Chen, K., et al. (2021). Creation of rich oxygen vacancies in bismuth molybdate nanosheets to boost the photocatalytic nitrogen fixation performance under visible light illumination. Chem. Eng. J. 404, 127115. doi:10.1016/j.cej.2020.127115
Guo, B., Cheng, X., Tang, Y., Guo, W., Deng, S., Wu, L., et al. (2022). Dehydrated UiO-66(SH)2: The Zr−O Cluster and its photocatalytic role mimicking the biological nitrogen fixation. Angew. Chem. Int. Ed. Engl. 61 (13), e202117244. doi:10.1002/anie.202117244
Guo, C. X., Ran, J., Vasileff, A., and Qiao, S. Z. (2018). Rational design of electrocatalysts and photo(electro)catalysts for nitrogen reduction to ammonia (NH3) under ambient conditions. Energy Environ. Sci. 11 (1), 45–56. doi:10.1039/C7EE02220D
Guo, J., and Chen, P. (2017). Catalyst: NH3 as an energy carrier. Chem 3 (5), 709–712. doi:10.1016/j.chempr.2017.10.004
Guoan Wang, G., Huo, T., Deng, Q., Yu, F., Xia, Y., Li, H., et al. (2022). Surface-layer bromine doping enhanced generation of surface oxygen vacancies in bismuth molybdate for efficient photocatalytic nitrogen fixation. Appl. Catal. B Environ. 310, 121319. doi:10.1016/j.apcatb.2022.121319
Haiguang Zhang, H., Li, X., Su, H., Chen, X., Zuo, S., Yan, X., et al. (2019). Sol–gel synthesis of upconversion perovskite/attapulgite heterostructures for photocatalytic fixation of nitrogen. J. Sol-Gel Sci. Technol. 92 (1), 154–162. doi:10.1007/s10971-019-05071-7
Haitao Li, H., Liu, Y., Liu, Y., Wang, L., Tang, R., Deng, P., et al. (2021). Efficient visible light driven ammonia synthesis on sandwich structured C3N4/MoS2/Mn3O4 catalyst. Appl. Catal. B Environ. 281, 119476. doi:10.1016/j.apcatb.2020.119476
Han, J., Liu, Z., Ma, Y., Cui, G., Xie, F., Wang, F., et al. (2018). Ambient N2 fixation to NH3 at ambient conditions: Using Nb2O5 nanofiber as a high-performance electrocatalyst. Nano Energy 52, 264–270. doi:10.1016/j.nanoen.2018.07.045
Hao Li, H., Shang, J., Shi, J., Zhao, K., and Zhang, L. (2016). Facet-dependent solar ammonia synthesis of BiOCl nanosheets via a proton-assisted electron transfer pathway. Nanoscale 8 (4), 1986–1993. doi:10.1039/C5NR07380D
Hao, Y., Dong, X., Zhai, S., Ma, H., Wang, X., Zhang, X., et al. (2016). Hydrogenated bismuth molybdate nanoframe for efficient sunlight‐driven nitrogen fixation from air. Chem. Eur. J. 22 (52), 18722–18728. doi:10.1002/chem.201604510
Hao, Q., Liu, C., Jia, G., Wang, Y., Arandiyan, H., Wei, W., et al. (2020). Catalytic reduction of nitrogen to produce ammonia by bismuth-based catalysts: State of the art and future prospects. Mat. Horiz. 7 (4), 1014–1029. doi:10.1039/C9MH01668F
Hao, L., Xia, Q., Zhang, Q., Masa, J., and Sun, Z. (2021). Improving the performance of metal-organic frameworks for thermo-catalytic CO2 conversion: Strategies and perspectives. Chin. J. Catal. 42 (11), 1903–1920. doi:10.1016/S1872-2067(21)63841-X
Hinnemann, B., and Nørskov, J. K. (2006). Catalysis by enzymes: The biological ammonia synthesis. Top. Catal. 37 (1), 55–70. doi:10.1007/s11244-006-0002-0
Hochman, G., Goldman, A. S., Felder, F. A., Mayer, J. M., Miller, A. J. M., Holland, P. L., et al. (2020). Potential economic feasibility of direct electrochemical nitrogen reduction as a route to ammonia. ACS Sustain. Chem. Eng. 8 (24), 8938–8948. doi:10.1021/acssuschemeng.0c01206
Hongda Li, H., Gu, S., Sun, Z., Guo, F., Xie, Y., Tao, B., et al. (2020). The in-built bionic “MoFe cofactor” in Fe-doped two-dimensional MoTe2 nanosheets for boosting the photocatalytic nitrogen reduction performance. J. Mat. Chem. A 8 (26), 13038–13048. doi:10.1039/d0ta04251j
Horn, M. R., Singh, A., Alomari, S., Goberna-Ferrón, S., Benages-Vilau, R., Chodankar, N., et al. (2021). Polyoxometalates (POMs): From electroactive clusters to energy materials. Energy Environ. Sci. 14 (4), 1652–1700. doi:10.1039/D0EE03407J
Hou, T. T., Xiao, Y., Cui, P. X., Huang, Y., Tan, X. P., Zheng, X. S., et al. (2019). Operando oxygen vacancies for enhanced activity and stability toward nitrogen photofixation. Adv. Energy Mat. 9 (43), 1902319. doi:10.1002/aenm.201902319
Hu, K., Huang, Z., Zeng, L., Zhang, Z., Mei, L., Chai, Z., et al. (2022). Recent advances in MOF-based materials for photocatalytic nitrogen fixation. Eur. J. Inorg. Chem. 2022 (3), e202100748. doi:10.1002/ejic.202100748
Huang, L., Wu, J., Han, P., Al-Enizi, A. M., Almutairi, T. M., Zhang, L., et al. (2019). NbO2 electrocatalyst toward 32% faradaic efficiency for N2 fixation. Small Methods 3 (6), 1800386. doi:10.1002/smtd.201800386
Huang, Y., Zhang, N., Wu, Z., and Xie, X. (2020). Artificial nitrogen fixation over bismuth-based photocatalysts: Fundamentals and future perspectives. J. Mat. Chem. A 8 (10), 4978–4995. doi:10.1039/C9TA13589H
Hui, X., Li, L., Xia, Q., Hong, S., Hao, L., Robertson, A. W., et al. (2022). Interface engineered Sb2O3/W18O49 heterostructure for enhanced visible-light-driven photocatalytic N2 reduction. Chem. Eng. J. 438, 135485. doi:10.1016/j.cej.2022.135485
Huimin Liu, H., Guijarro, N., and Luo, J. (2021). The pitfalls in electrocatalytic nitrogen reduction for ammonia synthesis. J. Energy Chem. 61, 149–154. doi:10.1016/j.jechem.2021.01.039
Jacobsen, C. J., Dahl, S., Clausen, B. S., Bahn, S., Logadottir, A., Nørskov, J. K., et al. (2001). Catalyst design by interpolation in the periodic table: Bimetallic ammonia synthesis catalysts. J. Am. Chem. Soc. 123 (34), 8404–8405. doi:10.1021/ja010963d
Jia, H. P., and Quadrelli, E. A. (2014). Mechanistic aspects of dinitrogen cleavage and hydrogenation to produce ammonia in catalysis and organometallic chemistry: Relevance of metal hydride bonds and dihydrogen. Chem. Soc. Rev. 43 (2), 547–564. doi:10.1039/C3CS60206K
Kitano, M., Inoue, Y., Yamazaki, Y., Hayashi, F., Kanbara, S., Matsuishi, S., et al. (2012). Ammonia synthesis using a stable electride as an electron donor and reversible hydrogen store. Nat. Chem. 4 (11), 934–940. doi:10.1038/nchem.1476
Kong, W., Liu, Z., Han, J., Xia, L., Wang, Y., Liu, Q., et al. (2019). Ambient electrochemical N2-to-NH3 fixation enabled by Nb2O5 nanowire array. Inorg. Chem. Front. 6 (2), 423–427. doi:10.1039/C8QI01049H
Kramm, U. I., Marschall, R., and Rose, M. (2019). Pitfalls in heterogeneous thermal, electro‐and photocatalysis. ChemCatChem 11 (11), 2563–2574. doi:10.1002/cctc.201900137
Lee, J., Tan, L. L., and Chai, S. P. (2021). Heterojunction photocatalysts for artificial nitrogen fixation: Fundamentals, latest advances and future perspectives. Nanoscale 13 (15), 7011–7033. doi:10.1039/d1nr00783a
Lei, W., Xiao, J.-L., Liu, H.-P., Jia, Q.-L., and Zhang, H.-J. (2020). Tungsten disulfide: Synthesis and applications in electrochemical energy storage and conversion. Tungsten 2 (3), 217–239. doi:10.1007/s42864-020-00054-6
Li, X., Sun, X., Zhang, L., Sun, S., and Wang, W. (2018). Efficient photocatalytic fixation of N2 by KOH-treated g-C3N4. J. Mat. Chem. A 6 (7), 3005–3011. doi:10.1039/C7TA09762J
Li, J., Chen, R., Wang, J., Zhou, Y., Yang, G., Dong, F., et al. (2022a). Subnanometric alkaline-earth oxide clusters for sustainable nitrate to ammonia photosynthesis. Nat. Commun. 13 (1), 1098. doi:10.1038/s41467-022-28740-8
Li, J., Lou, Z., and Li, B. (2022b). Nanostructured materials with localized surface plasmon resonance for photocatalysis. Chin. Chem. Lett. 33 (3), 1154–1168. doi:10.1016/j.cclet.2021.07.059
Liang Yang, L., Choi, C., Hong, S., Liu, Z., Zhao, Z., Yang, M., et al. (2020). Single yttrium sites on carbon-coated TiO2 for efficient electrocatalytic N2 reduction. Chem. Commun. 56 (74), 10910–10913. doi:10.1039/D0CC01136C
Libo Wang, L., Zhang, Q., Wei, T., Li, F., Sun, Z., Xu, L., et al. (2021). WC and cobalt nanoparticles embedded in nitrogen-doped carbon 3D nanocage derived from H3PW12O40@ZIF-67 for photocatalytic nitrogen fixation. J. Mat. Chem. A 9 (5), 2912–2918. doi:10.1039/D0TA10303A
Lin Liu, L., Liu, J., Sun, K., Wan, J., Fu, F., Fan, J., et al. (2021). Novel phosphorus-doped Bi2WO6 monolayer with oxygen vacancies for superior photocatalytic water detoxication and nitrogen fixation performance. Chem. Eng. J. 411, 128629. doi:10.1016/j.cej.2021.128629
Lin Wang, L., Li, X., Hao, L., Hong, S., Robertson, A. W., Sun, Z., et al. (2022). Integration of ultrafine CuO nanoparticles with two-dimensional MOFs for enhanced electrochemicgal CO2 reduction to ethylene. Chin. J. Catal. 43 (4), 1049–1057. doi:10.1016/S1872-2067(21)63947-5
Lindley, B. M., Appel, A. M., Krogh-Jespersen, K., Mayer, J. M., and Miller, A. J. (2016). Evaluating the thermodynamics of electrocatalytic N2 reduction in acetonitrile. ACS Energy Lett. 1 (4), 698–704. doi:10.1021/acsenergylett.6b00319
Linsebigler, A. L., Lu, G., and Yates, J. T. (1995). Photocatalysis on TiO2 surfaces: Principles, mechanisms, and selected results. Chem. Rev. 95 (3), 735–758. doi:10.1021/cr00035a013
Liu, J., Zhang, Y., Lu, L., Wu, G., and Chen, W. (2012). Self-regenerated solar-driven photocatalytic water-splitting by urea derived graphitic carbon nitride with platinum nanoparticles. Chem. Commun. 48 (70), 8826. doi:10.1039/C2CC33644H
Liu, J., Kelley, M. S., Wu, W., Banerjee, A., Douvalis, A. P., Wu, J., et al. (2016). Nitrogenase-mimic iron-containing chalcogels for photochemical reduction of dinitrogen to ammonia. Proc. Natl. Acad. Sci. U. S. A. 113(20), 5530–5535. doi:10.1073/pnas.1605512113
Liu, Y., Cheng, M., He, Z., Gu, B., Xiao, C., Zhou, T., et al. (2019). Pothole‐rich ultrathin WO3 nanosheets that trigger N≡N bond activation of nitrogen for direct nitrate photosynthesis. Angew. Chem. Int. Ed. 58 (3), 731–735. doi:10.1002/anie.201808177
Liu, X., Luo, Y., Ling, C., Shi, Y., Zhan, G., Li, H., et al. (2022). Rare Earth La single atoms supported MoO3−x for efficient photocatalytic nitrogen fixation. Appl. Catal. B Environ. 301, 120766. doi:10.1016/j.apcatb.2021.120766
Lu, H., Zhao, Y.-M., Saji, S. E., Yin, X., Wibowo, A., Tang, C. S., et al. (2022). All room-temperature synthesis, N2 photofixation and reactivation over 2D cobalt oxides. Appl. Catal. B Environ. 304, 121001. doi:10.1016/j.apcatb.2021.121001
Luo, J., Bai, X., Li, Q., Yu, X., Li, C., Wang, Z., et al. (2019). Band structure engineering of bioinspired Fe doped SrMoO4 for enhanced photocatalytic nitrogen reduction performance. Nano Energy 66, 104187. doi:10.1016/j.nanoen.2019.104187
Lv, C., Yan, C., Chen, G., Ding, Y., Sun, J., Zhou, Y., et al. (2018). An amorphous noble-metal-free electrocatalyst that enables nitrogen fixation under ambient conditions. Angew. Chem. Int. Ed. 57 (21), 6073–6076. doi:10.1002/anie.201801538
Mansingh, S., Das, K. K., Sultana, S., and Parida, K. (2021). Recent advances in wireless photofixation of dinitrogen to ammonia under the ambient condition: A review. J. Photochem. Photobiol. C Photochem. Rev. 47, 100402. doi:10.1016/j.jphotochemrev.2021.100402
Mao, Y., Yang, X., Gong, W., Zhang, J., Pan, T., Sun, H., et al. (2020). A dopant replacement-driven molten salt method toward the synthesis of sub-5-nm-sized ultrathin nanowires. Small 16 (23), 2001098. doi:10.1002/smll.202001098
Medford, A. J., and Hatzell, M. C. (2017). Photon-driven nitrogen fixation: Current progress, thermodynamic considerations, and future outlook. ACS Catal. 7 (4), 2624–2643. doi:10.1021/acscatal.7b00439
Meng, Q., Lv, C., Sun, J., Hong, W., Xing, W., Qiang, L., et al. (2019). High-efficiency Fe-mediated Bi2MoO6 nitrogen-fixing photocatalyst: Reduced surface work function and ameliorated surface reaction. Appl. Catal. B Environ. 256, 117781. doi:10.1016/j.apcatb.2019.117781
Meng, S.-L., Li, X.-B., Tung, C.-H., and Wu, L.-Z. (2021). Nitrogenase inspired artificial photosynthetic nitrogen fixation. Chem 7 (6), 1431–1450. doi:10.1016/j.chempr.2020.11.002
Meng Yang, M., Huo, R. P., Shen, H. D., Xia, Q. N., Qiu, J. S., Robertson, A. W., et al. (2020). Metal-tuned W18O49 for efficient electrocatalytic N2 reduction. ACS Sustain. Chem. Eng. 8 (7), 2957–2963. doi:10.1021/acssuschemeng.9b07526
Mingli Zhang, M., Choi, C., Huo, R., Gu, G. H., Hong, S., Yan, C., et al. (2020). Reduced graphene oxides with engineered defects enable efficient electrochemical reduction of dinitrogen to ammonia in wide pH range. Nano Energy 68, 104323. doi:10.1016/j.nanoen.2019.104323
Montoya, J. H., Tsai, C., Vojvodic, A., and Nørskov, J. K. (2015). The challenge of electrochemical ammonia synthesis: A new perspective on the role of nitrogen scaling relations. ChemSusChem 8 (13), 2180–2186. doi:10.1002/cssc.201500322
Mou, H., Wang, J., Yu, D., Zhang, D., Chen, W., Wang, Y., et al. (2019). Fabricating amorphous g-C3N4/ZrO2 photocatalysts by one-step pyrolysis for solar-driven ambient ammonia synthesis. ACS Appl. Mat. Interfaces 11 (47), 44360–44365. doi:10.1021/acsami.9b16432
Mousavi, M., Habibi, M. M., Zhang, G., Pourhakkak, P., moradian, S., Ghasemi, J. B., et al. (2022). In-situ construction of ZnO/Sb2MoO6 nano-heterostructure for efficient visible-light photocatalytic conversion of N2 to NH3. Surf. Interfaces 30, 101844. doi:10.1016/j.surfin.2022.101844
Ning Zhang, N., Jalil, A., Wu, D. X., Chen, S. M., Liu, Y. F., Gao, C., et al. (2018). Refining defect states in W18O49 by Mo doping: A strategy for tuning N2 activation towards solar-driven nitrogen fixation. J. Am. Chem. Soc. 140 (30), 9434–9443. doi:10.1021/jacs.8b02076
Nunes, B. N., Lopes, O. F., Patrocinio, A. O. T., and Bahnemann, D. W. (2020). Recent advances in niobium-based materials for photocatalytic solar fuel production. Catalysts 10 (1), 126. doi:10.3390/catal10010126
Oshikiri, T., Ueno, K., and Misawa, H. (2016). Selective dinitrogen conversion to ammonia using water and visible light through plasmon‐induced charge separation. Angew. Chem. Int. Ed. 55 (12), 3942–3946. doi:10.1002/anie.201511189
Qin, J., Zhao, W., Hu, X., Li, J., Ndokoye, P., and Liu, B. (2021). Exploring the N2 adsorption and activation mechanisms over the 2H/1T Mixed-phase ultrathin Mo1−xWxS2 nanosheets for boosting N2 photosynthesis. ACS Appl. Mater. Interfaces. 13 (6), 7127–7134. doi:10.1021/acsami.0c19282
Qiu, P., Huang, C., Dong, G., Chen, F., Zhao, F., Yu, Y., et al. (2021). Plasmonic gold nanocrystals simulated efficient photocatalytic nitrogen fixation over Mo doped W18O49 nanowires. J. Mat. Chem. A 9 (25), 14459–14465. doi:10.1039/D1TA03339E
Ren, W., Mei, Z., Zheng, S., Li, S., Zhu, Y., Zheng, J., et al. (2020). Wavelength-dependent solar N2 fixation into ammonia and nitrate in pure water. Research 2020, 1–12. doi:10.34133/2020/3750314
Rong Zhang, R., Guo, H., Yang, L., Wang, Y., Niu, Z., Huang, H., et al. (2019). Electrocatalytic N2 fixation over hollow VO2 microspheres at ambient conditions. ChemElectroChem 6 (4), 1014–1018. doi:10.1002/celc.201801484
Schrauzer, G. N., and Guth, T. D. (1977). Photolysis of water and photoreduction of nitrogen on titanium dioxide. J. Am. Chem. Soc. 99 (22), 7189–7193. doi:10.1021/ja00464a015
Schrauzer, G., and Guth, T. (2002). Photolysis of water and photoreduction of nitrogen on titanium dioxide. J. Am. Chem. Soc. 99 (22), 7189–7193. doi:10.1021/ja00464a015
Shen, H., Peppel, T., Strunk, J., and Sun, Z. (2020). Photocatalytic reduction of CO2 by metal-free-based materials: Recent advances and future perspective. Sol. RRL 4 (8), 1900546. doi:10.1002/solr.201900546
Shen, H., Yang, M., Hao, L., Wang, J., Strunk, J., Sun, Z., et al. (2021). Photocatalytic nitrogen reduction to ammonia: Insights into the role of defect engineering in photocatalysts. Nano Res. 15, 2773–2809. doi:10.1007/s12274-021-3725-0
Shende, A. G., Tiwari, C. S., Bhoyar, T. H., Vidyasagar, D., and Umare, S. S. (2019). BWO nano-octahedron coupled with layered g-C3N4: An efficient visible light active photocatalyst for degradation of cationic/anionic dyes, and N2 reduction. J. Mol. Liq. 296, 111771. doi:10.1016/j.molliq.2019.111771
Shi, R., Zhao, Y., Waterhouse, G. I. N., Zhang, S., and Zhang, T. (2019). Defect engineering in photocatalytic nitrogen fixation. ACS Catal. 9 (11), 9739–9750. doi:10.1021/acscatal.9b03246
Shi, L., Yin, Y., Wang, S., and Sun, H. (2020a). Rational catalyst design for N2 reduction under ambient conditions: Strategies toward enhanced conversion efficiency. ACS Catal. 10 (12), 6870–6899. doi:10.1021/acscatal.0c01081
Shi, L., Li, Z., Ju, L., Carrasco-Pena, A., Orlovskaya, N., Zhou, H., et al. (2020b). Promoting nitrogen photofixation over a periodic WS2@TiO2 nanoporous film. J. Mat. Chem. A 8 (3), 1059–1065. doi:10.1039/C9TA12743G
Shipman, M. A., and Symes, M. D. (2017). Recent progress towards the electrosynthesis of ammonia from sustainable resources. Catal. Today 286, 57–68. doi:10.1016/j.cattod.2016.05.008
Shiraishi, Y., Shiota, S., Kofuji, Y., Hashimoto, M., Chishiro, K., Hirakawa, H., et al. (2018). Nitrogen fixation with water on carbon-nitride-based metal-free photocatalysts with 0.1% solar-to-ammonia energy conversion efficiency. ACS Appl. Energy Mat. 1 (8), 4169–4177. doi:10.1021/acsaem.8b00829
Shuai Zhang, S., Zhao, Y., Shi, R., Waterhouse, G. I., and Zhang, T. (2019). Photocatalytic ammonia synthesis: Recent progress and future. EnergyChem 1 (2), 100013. doi:10.1016/j.enchem.2019.100013
Skúlason, E., Bligaard, T., Gudmundsdóttir, S., Studt, F., Rossmeisl, J., Abild-Pedersen, F., et al. (2012). A theoretical evaluation of possible transition metal electro-catalysts for N2 reduction. Phys. Chem. Chem. Phys. 14 (3), 1235–1245. doi:10.1039/C1CP22271F
Song, J., Huang, Z.-F., Pan, L., Zou, J.-J., Zhang, X., Wang, L., et al. (2015). Oxygen-deficient tungsten oxide as versatile and efficient hydrogenation catalyst. ACS Catal. 5 (11), 6594–6599. doi:10.1021/acscatal.5b01522
Su, S., Li, X., Zhang, X., Zhu, J., Liu, G., Tan, M., et al. (2022). Keggin-type SiW12 encapsulated in MIL-101(Cr) as efficient heterogeneous photocatalysts for nitrogen fixation reaction. J. Colloid Interface Sci. 621, 406–415. doi:10.1016/j.jcis.2022.04.006
Sun, S., Li, X., Wang, W., Zhang, L., and Sun, X. (2017). Photocatalytic robust solar energy reduction of dinitrogen to ammonia on ultrathin MoS2. Appl. Catal. B Environ. 200, 323–329. doi:10.1016/j.apcatb.2016.07.025
Sun, Z., Talreja, N., Tao, H., Texter, J., Muhler, M., Strunk, J., et al. (2018). Catalysis of carbon dioxide photoreduction on nanosheets: Fundamentals and challenges. Angew. Chem. Int. Ed. 57 (26), 7610–7627. doi:10.1002/anie.201710509
Sun, Z., Huo, R., Choi, C., Hong, S., Wu, T.-S., Qiu, J., et al. (2019). Oxygen vacancy enables electrochemical N2 fixation over WO3 with tailored structure. Nano Energy 62, 869–875. doi:10.1016/j.nanoen.2019.06.019
Sun, D., Bai, H., Zhao, Y., Zhang, Q., Bai, Y., Liu, Y., et al. (2020). Amorphous MnCO3/C double layers decorated on BiVO4 photoelectrodes to boost nitrogen reduction. ACS Appl. Mat. Interfaces 12 (47), 52763–52770. doi:10.1021/acsami.0c16337
Swain, G., Sultana, S., and Parida, K. (2020). Constructing a novel surfactant-free MoS2 nanosheet modified MgIn2S4 marigold microflower: An efficient visible-light driven 2D-2D p-n heterojunction photocatalyst toward HER and pH regulated NRR. ACS Sustain. Chem. Eng. 8 (12), 4848–4862. doi:10.1021/acssuschemeng.9b07821
Tang, C., and Qiao, S.-Z. (2019). How to explore ambient electrocatalytic nitrogen reduction reliably and insightfully. Chem. Soc. Rev. 48 (12), 3166–3180. doi:10.1039/C9CS00280D
Tao, H., Choi, C., Ding, L.-X., Jiang, Z., Han, Z., Jia, M., et al. (2019). Nitrogen fixation by Ru single-atom electrocatalytic reduction. Chem 5 (1), 204–214. doi:10.1016/j.chempr.2018.10.007
Tianyu Wang, T., Feng, C., Liu, J., Wang, D., Hu, H., Hu, J., et al. (2021). Bi2WO6 hollow microspheres with high specific surface area and oxygen vacancies for efficient photocatalysis N2 fixation. Chem. Eng. J. 414, 128827. doi:10.1016/j.cej.2021.128827
Tianyu Wang, T., Liu, J., Wu, P., Feng, C., Wang, D., Hu, H., et al. (2020). Direct utilization of air and water as feedstocks in the photo-driven nitrogen reduction reaction over a ternary Z-scheme SiW9Co3/PDA/BWO hetero-junction. J. Mat. Chem. A 8 (32), 16590–16598. doi:10.1039/C9TA13902H
Tong Xu, T., Ma, B., Liang, J., Yue, L., Liu, Q., Li, T., et al. (2021). Recent progress in metal-free electrocatalysts toward ambient N2 reduction reaction. Acta Phys. -Chim. Sin. 37 (7), 2009043. doi:10.3866/pku.Whxb202009043
Utomo, W. P., Leung, M. K. H., Yin, Z., Wu, H., and Ng, Y. H. (2022). Advancement of bismuth-based materials for electrocatalytic and photo(electro)catalytic ammonia synthesis. Adv. Funct. Mat. 32 (4), 2106713. doi:10.1002/adfm.202106713
van der Ham, C. J. M., Koper, M. T. M., and Hetterscheid, D. G. H. (2014). Challenges in reduction of dinitrogen by proton and electron transfer. Chem. Soc. Rev. 43 (15), 5183–5191. doi:10.1039/C4CS00085D
Vesali-Kermani, E., Habibi-Yangjeh, A., Diarmand-Khalilabad, H., and Ghosh, S. (2020a). Nitrogen photofixation ability of g-C3N4 nanosheets/Bi2MoO6 heterojunction photocatalyst under visible-light illumination. J. Colloid Interface Sci. 563, 81–91. doi:10.1016/j.jcis.2019.12.057
Vesali-Kermani, E., Habibi-Yangjeh, A., and Ghosh, S. (2020b). Efficiently enhanced nitrogen fixation performance of g-C3N4 nanosheets by decorating Ni3V2O8 nanoparticles under visible-light irradiation. Ceram. Int. 46 (15), 24472–24482. doi:10.1016/j.ceramint.2020.06.232
Wen Zhao, W., Qin, J., Teng, W., Mu, J., Chen, C., Ke, J., et al. (2022). Catalytic photo-redox of simulated air into ammonia over bimetallic MOFs nanosheets with oxygen vacancies. Appl. Catal. B Environ. 305, 121046. doi:10.1016/j.apcatb.2021.121046
Wenjie Liu, W., Yin, K., Yuan, K., Zuo, S., Yang, S., Yao, C., et al. (2020). In situ synthesis of Bi2MoO6@C@attapulgite photocatalyst for enhanced photocatalytic nitrogen fixation ability under simulated solar irradiation. Colloids Surfaces A Physicochem. Eng. Aspects 591, 124488. doi:10.1016/j.colsurfa.2020.124488
Xiao, C., Zhang, L., Wang, K., Wang, H., Zhou, Y., Wang, W., et al. (2018). A new approach to enhance photocatalytic nitrogen fixation performance via phosphate-bridge: A case study of SiW12/K-C3N4. Appl. Catal. B Environ. 239, 260–267. doi:10.1016/j.apcatb.2018.08.012
Xiao-Fei Li, X.-F., Li, Q.-K., Cheng, J., Liu, L., Yan, Q., Wu, Y., et al. (2016). Conversion of dinitrogen to ammonia by FeN3-embedded graphene. J. Am. Chem. Soc. 138 (28), 8706–8709. doi:10.1021/jacs.6b04778
Xiao-Hong Li, X.-H., Chen, W.-L., Tan, H.-Q., Li, F.-R., Li, J.-P., Li, Y.-G., et al. (2019). Reduced state of the graphene oxide@polyoxometalate nanocatalyst achieving high-efficiency nitrogen fixation under light driving conditions. ACS Appl. Mat. Interfaces 11 (41), 37927–37938. doi:10.1021/acsami.9b12328
Xiao-Hong Li, X.-H., He, P., Wang, T., Zhang, X.-W., Chen, W.-L., Li, Y.-G., et al. (2020). Keggin-type polyoxometalate-based ZIF-67 for enhanced photocatalytic nitrogen fixation. ChemSusChem 13 (10), 2769–2778. doi:10.1002/cssc.202000328
Xiazhang Li, X., He, C., Dai, D., Zuo, S., Yan, X., Yao, C., et al. (2020). Nano-mineral induced nonlinear optical LiNbO3 with abundant oxygen vacancies for photocatalytic nitrogen fixation: Boosting effect of polarization. Appl. Nanosci. 10 (9), 3477–3490. doi:10.1007/s13204-020-01443-6
Xin Liu, X., Gu, S., Zhao, Y., Zhou, G., and Li, W. (2020). BiVO4, Bi2WO6 and Bi2MoO6 photocatalysis: A brief review. J. Mat. Sci. Technol. 56, 45–68. doi:10.1016/j.jmst.2020.04.023
Xin Wang, X., Feng, Z., Xiao, B., Zhao, J., Ma, H., Tian, Y., et al. (2020). Polyoxometalate-based metal-organic framework-derived bimetallic hybrid materials for upgraded electrochemical reduction of nitrogen. Green Chem. 22 (18), 6157–6169. doi:10.1039/D0GC01149E
Xing, P., Chen, P., Chen, Z., Hu, X., Lin, H., Wu, Y., et al. (2018). Novel ternary MoS2/C-ZnO composite with efficient performance in photocatalytic NH3 synthesis under simulated sunlight. ACS Sustain. Chem. Eng. 6 (11), 14866–14879. doi:10.1021/acssuschemeng.8b03388
Xing, P., Wu, S., Chen, Y., Chen, P., Hu, X., Lin, H., et al. (2019). New application and excellent performance of Ag/KNbO3 nanocomposite in photocatalytic NH3 synthesis. ACS Sustain. Chem. Eng. 7 (14), acssuschemeng.9b01938. doi:10.1021/acssuschemeng.9b01938
Xing, P., Zhang, W., Chen, L., Dai, X., Zhang, J., Zhao, L., et al. (2020). Preparation of a NiO/KNbO3 nanocomposite via a photodeposition method and its superior performance in photocatalytic N2 fixation. Sustain. Energy Fuels 4 (3), 1112–1117. doi:10.1039/C9SE01003C
Xu, T., Ma, D., Li, C., Liu, Q., Lu, S., Asiri, A. M., et al. (2020). Ambient electrochemical NH3 synthesis from N2 and water enabled by ZrO2 nanoparticles. Chem. Commun. 56 (25), 3673–3676. doi:10.1039/C9CC10087C
Xu, H., Ma, Y., Chen, J., Zhang, W., and Yang, J. (2022). Electrocatalytic reduction of nitrate – A step towards a sustainable nitrogen cycle. Chem. Soc. Rev. 51, 2710–2758. doi:10.1039/D1CS00857A
Xu, Y., and Schoonen, M. A. (2000). The absolute energy positions of conduction and valence bands of selected semiconducting minerals. Am. Mineral. 85 (3-4), 543–556. doi:10.2138/am-2000-0416
Xue Chen, X., Li, J.-Y., Tang, Z.-R., and Xu, Y.-J. (2020). Surface-defect-engineered photocatalyst for nitrogen fixation into value-added chemical feedstocks. Catal. Sci. Technol. 10 (18), 6098–6110. doi:10.1039/d0cy01227k
Xue, X., Chen, R., Yan, C., Hu, Y., Zhang, W., Yang, S., et al. (2019). Efficient photocatalytic nitrogen fixation under ambient conditions enabled by the heterojunctions of n-type Bi2MoO6 and oxygen-vacancy-rich p-type BiOBr. Nanoscale 11 (21), 10439–10445. doi:10.1039/C9NR02279A
Xuerui Zhang, X., Shi, R., Li, Z., Zhao, J., Huang, H., Zhou, C., et al. (2022). Photothermal-assisted photocatalytic nitrogen oxidation to nitric acid on palladium-decorated titanium oxide. Adv. Energy Mat. 12, 2103740. doi:10.1002/aenm.202103740
Yang, J., Bai, H., Guo, Y., Zhang, H., Jiang, R., Yang, B., et al. (2021). Photodriven disproportionation of nitrogen and its change to reductive nitrogen photofixation. Angew. Chem. Int. Ed. 60 (2), 927–936. doi:10.1002/anie.202010192
Yehuan Li, Y., Chen, X., Zhang, M., Zhu, Y., Ren, W., Mei, Z., et al. (2019). Oxygen vacancy-rich MoO3−x nanobelts for photocatalytic N2 reduction to NH3 in pure water. Catal. Sci. Technol. 9 (3), 803–810. doi:10.1039/C8CY02357C
Yi-Fei Zhang, Y.-F., Zhu, Y.-K., Lv, C.-X., Lai, S.-J., Xu, W.-J., Sun, J., et al. (2020). Enhanced visible-light photoelectrochemical performance via chemical vapor deposition of Fe2O3 on a WO3 film to form a heterojunction. Rare Met. 39 (7), 841–849. doi:10.1007/s12598-019-01311-5
Yifu Chen, Y., Liu, H., Ha, N., Licht, S., Gu, S., Li, W., et al. (2020). Revealing nitrogen-containing species in commercial catalysts used for ammonia electrosynthesis. Nat. Catal. 3 (12), 1055–1061. doi:10.1038/s41929-020-00527-4
Yin, S., and Asakura, Y. (2019). Recent research progress on mixed valence state tungsten based materials. Tungsten 1 (1), 5–18. doi:10.1007/s42864-019-00001-0
Yin, Y., Jiang, M., and Liu, C. (2018). DFT study of POM-supported single atom catalyst (M1/POM, M = Ni, Pd, Pt, Cu, Ag, Au, POM = [PW12O40]3-) for activation of nitrogen molecules. Acta Phys. -Chim. Sin. 34 (3), 270–277. doi:10.3866/pku.Whxb201707071
Ying, Z., Chen, S., Zhang, S., Peng, T., and Li, R. (2019). Efficiently enhanced N2 photofixation performance of sea-urchin-like W18O49 microspheres with Mn-doping. Appl. Catal. B Environ. 254, 351–359. doi:10.1016/j.apcatb.2019.05.005
Yuan Feng, Y., zhao, Z., Wang, T., Li, J., Xu, M., Jiao, H., et al. (2022). Magnetic field-enhanced photocatalytic nitrogen fixation over defect-rich ferroelectric Bi2WO6. Ceram. Int. 48, 20062–20069. doi:10.1016/j.ceramint.2022.03.282
Yuan, J., Yi, X., Tang, Y., Liu, M., and Liu, C. (2020). Efficient photocatalytic nitrogen fixation: Enhanced polarization, activation, and cleavage by asymmetrical electron donation to N≡N bond. Adv. Funct. Mater. 30 (4), 1906983. doi:10.1002/adfm.201906983
Yuhua Wang, Y. H., Liu, T. Y., Li, H. H., Liu, B., and Yang, L. F. (2019). Tungsten-based photocatalysts with UV–Vis–NIR photocatalytic capacity: Progress and opportunity. Tungsten 1 (4), 247–257. doi:10.1007/s42864-020-00031-z
Yuting Wang, Y., Li, H., Zhou, W., Zhang, X., Zhang, B., Yu, Y., et al. (2022). Structurally disordered RuO2 nanosheets with rich oxygen vacancies for enhanced nitrate electroreduction to ammonia. Angew. Chem. Int. Ed. Engl. 61, e202202604. doi:10.1002/anie.202202604
Yuxin Liu, Y., Xue, Y., Hui, L., Yu, H., Fang, Y., He, F., et al. (2021). Porous graphdiyne loading CoOx quantum dots for fixation nitrogen reaction. Nano Energy 89, 106333. doi:10.1016/j.nanoen.2021.106333
Zejian Wang, J. H., Ng, Sue-Faye, Wen, Liu, Huang, Junjie, Chen, Pengfei, and Ong, Wee-Jun (2021). Recent progress of perovskite oxide in emerging photocatalysis landscape: Water splitting, CO2 reduction, and N2 fixation. Acta Phys. -Chim. Sin. 37 (6), 2011033. doi:10.3866/pku.Whxb202011033
Zhang, Y., Yu, J., Yu, D., Zhou, X., and Lu, W. (2011). Enhancement in the photocatalytic and photoelectrochemical properties of visible-light driven BiVO4 photocatalyst. Rare Met. 30 (1), 192–198. doi:10.1007/s12598-011-0267-8
Zhang, L., Mohamed, H. H., Dillert, R., and Bahnemann, D. (2012). Kinetics and mechanisms of charge transfer processes in photocatalytic systems: A review. J. Photochem. Photobiol. C Photochem. Rev. 13 (4), 263–276. doi:10.1016/j.jphotochemrev.2012.07.002
Zhang, Y., Dong, N., Tao, H., Yan, C., Huang, J., Liu, T., et al. (2017). Exfoliation of stable 2D black phosphorus for device fabrication. Chem. Mat. 229, 66445–66456. doi:10.1021/acs.chemmater.7b01991
Zhang, G., Meng, Y., Xie, B., Ni, Z., Lu, H., Xia, S., et al. (2021). Precise location and regulation of active sites for highly efficient photocatalytic synthesis of ammonia by facet-dependent BiVO4 single crystals. Appl. Catal. B Environ. 296, 120379. doi:10.1016/j.apcatb.2021.120379
Zhao, W., Xi, H., Zhang, M., Li, Y., Chen, J., Zhang, J., et al. (2015). Enhanced quantum yield of nitrogen fixation for hydrogen storage with in situ-formed carbonaceous radicals. Chem. Commun. 51 (23), 4785–4788. doi:10.1039/C5CC00589B
Zhao, Y., Shi, R., Bian, X., Zhou, C., Zhao, Y., Zhang, S., et al. (2019). Ammonia detection methods in photocatalytic and electrocatalytic experiments: How to improve the reliability of NH3 production rates? Adv. Sci. 6 (8), 1802109. doi:10.1002/advs.201802109
Zhao, Z. Q., Choi, C., Hong, S., Shen, H. D., Yan, C., Masa, J., et al. (2020). Surface-engineered oxidized two-dimensional Sb for efficient visible light-driven N2 fixation. Nano Energy 78, 105368. doi:10.1016/j.nanoen.2020.105368
Zhao, Y., Wu, F., Miao, Y., Zhou, C., Xu, N., Shi, R., et al. (2021). Revealing ammonia quantification minefield in photo/electrocatalysis. Angew. Chem. Int. Ed. 60 (40), 21728–21731. doi:10.1002/anie.202108769
Zhen Zhao, Z., Park, J., Choi, C., Hong, S., Hui, X., Zhang, H., et al. (2022). Engineering vacancy and hydrophobicity of two-dimensional TaTe2 for efficient and stable electrocatalytic N2 reduction. Innovation 3 (1), 100190. doi:10.1016/j.xinn.2021.100190
Zheng, H., Ou, J. Z., Strano, M. S., Kaner, R. B., Mitchell, A., Kalantar‐zadeh, K., et al. (2011). Nanostructured tungsten oxide–properties, synthesis, and applications. Adv. Funct. Mat. 21 (12), 2175–2196. doi:10.1002/adfm.201002477
Zheng, Q., Xu, Y., Wan, Y., Wu, J., Hu, X., Yao, X., et al. (2020). Synthesis of CoFe2O4-modified g-C3N4 with enhanced photocatalytic performance for nitrogen fixation. J. Nanopart. Res. 22 (9), 301. doi:10.1007/s11051-020-05028-w
Zheng, J., Lu, L., Lebedev, K., Wu, S., Zhao, P., McPherson, I. J., et al. (2021). Fe on molecular-layer MoS2 as inorganic Fe-S2-Mo motifs for light-driven nitrogen fixation to ammonia at elevated temperatures. Chem. Catal. 1 (1), 162–182. doi:10.1016/j.checat.2021.03.002
Zheng Wang, Z., Li, C., and Domen, K. (2019). Recent developments in heterogeneous photocatalysts for solar-driven overall water splitting. Chem. Soc. Rev. 48 (7), 2109–2125. doi:10.1039/C8CS00542G
Zhi-Yuan Wang, Z.-Y., Jiang, S.-D., Duan, C.-Q., Wang, D., Luo, S.-H., Liu, Y.-G., et al. (2020). In situ synthesis of Co3O4 nanoparticles confined in 3D nitrogen-doped porous carbon as an efficient bifunctional oxygen electrocatalyst. Rare Met. 39 (12), 1383–1394. doi:10.1007/s12598-020-01581-4
Zhou, S., Zhang, C., Liu, J., Liao, J., Kong, Y., Xu, Y., et al. (2019). Formation of an oriented Bi2WO6 photocatalyst induced by in situ Bi reduction and its use for efficient nitrogen fixation. Catal. Sci. Technol. 9 (20), 5562–5566. doi:10.1039/C9CY00972H
Zhou, X., Liu, H., Xia, B. Y., Ostrikov, K., Zheng, Y., Qiao, S.-Z., et al. (2022). Customizing the microenvironment of CO2 electrocatalysis via three-phase interface engineering. SmartMat 3 (1), 111–129. doi:10.1002/smm2.1109
Zhu, D., Zhang, L., Ruther, R. E., and Hamers, R. J. (2013). Photo-illuminated diamond as a solid-state source of solvated electrons in water for nitrogen reduction. Nat. Mat. 12 (9), 836–841. doi:10.1038/nmat3696
Zhu, J., Chen, X., Thang, A. Q., Li, F.-L., Chen, D., Geng, H., et al. (2022). Vanadium-based metal-organic frameworks and their derivatives for electrochemical energy conversion and storage. SmartMat. doi:10.1002/smm2.1091
Keywords: photocatalysis, nitrogen reduction, ammonia synthesis, tungsten, semiconductor
Citation: Hui X, Wang L, Yao Z, Hao L and Sun Z (2022) Recent progress of photocatalysts based on tungsten and related metals for nitrogen reduction to ammonia. Front. Chem. 10:978078. doi: 10.3389/fchem.2022.978078
Received: 25 June 2022; Accepted: 15 July 2022;
Published: 22 August 2022.
Edited by:
Tiancheng Mu, Renmin University of China, ChinaReviewed by:
Baowen Zhou, Shanghai Jiao Tong University, ChinaTianbin Wu, Institute of Chemistry (CAS), China
Copyright © 2022 Hui, Wang, Yao, Hao and Sun. This is an open-access article distributed under the terms of the Creative Commons Attribution License (CC BY). The use, distribution or reproduction in other forums is permitted, provided the original author(s) and the copyright owner(s) are credited and that the original publication in this journal is cited, in accordance with accepted academic practice. No use, distribution or reproduction is permitted which does not comply with these terms.
*Correspondence: Leiduan Hao, aGFvbGRAbWFpbC5idWN0LmVkdS5jbg==; Zhenyu Sun, c3VuenlAbWFpbC5idWN0LmVkdS5jbg==