- 1RIKEN Cluster for Pioneering Research, Saitama, Japan
- 2School of Materials and Chemical Technology, Tokyo Institute of Technology, Tokyo, Japan
- 3School of Pharmaceutical Sciences (Shenzhen), Sun Yat-Sen University, Shenzhen, China
- 4Graduate School of Science, Osaka University, Osaka, Japan
For the stereoselective assembly of bioactive glycans with various functions, 1,2-cis-O-glycosylation is one of the most essential issues in synthetic carbohydrate chemistry. The cis-configured O-glycosidic linkages to the substituents at two positions of the non-reducing side residue of the glycosides such as α-glucopyranoside, α-galactopyranoside, β-mannopyranoside, β-arabinofuranoside, and other rather rare glycosides are found in natural glycans, including glycoconjugate (glycoproteins, glycolipids, proteoglycans, and microbial polysaccharides) and glycoside natural products. The way to 1,2-trans isomers is well sophisticated by using the effect of neighboring group participation from the most effective and kinetically favored C-2 substituent such as an acyl group, although high stereoselective synthesis of 1,2-cis glycosides without formation of 1,2-trans isomers is far less straightforward. Although the key factors that control the stereoselectivity of glycosylation are largely understood since chemical glycosylation was considered to be one of the useful methods to obtain glycosidic linkages as the alternative way of isolation from natural sources, strictly controlled formation of these 1,2-cis glycosides is generally difficult. This minireview introduces some of the recent advances in the development of 1,2-cis selective glycosylations, including the quite recent developments in glycosyl donor modification, reaction conditions, and methods for activation of intermolecular glycosylation, including the bimodal glycosylation strategy for 1,2-cis and 1,2-trans glycosides, as well as intramolecular glycosylations, including recent applications of NAP-ether-mediated intramolecular aglycon delivery.
Introduction
Stereoselective O-glycosylation is essential for achieving the facile assembly of biologically relevant oligosaccharides (Figure 1A). The cis-configured O-glycosidic linkages to the substituents at two positions of the non-reducing side residue of the glycosides such as α-glucopyranoside, α-galactopyranoside, β-mannopyranoside, β-arabinofuranoside, and other rather rare glycosides are found in natural glycans including glycoconjugates (glycoproteins, glycolipids, proteoglycans, and microbial polysaccharides) and glycoside natural products (Figure 1B). The way to 1,2-trans isomers is well sophisticated due to the effect of neighboring group participation from the most effective and kinetically favored C-2 substituent such as an acyl group, although high stereoselective synthesis of 1,2-cis glycosides without formation of the 1,2-trans isomer is far less straightforward. Although the key factors that control the stereoselectivity of glycosylation are largely understood since chemical glycosylation was considered to be one of the useful methods to obtain glycosidic linkages as an alternative way of isolation from natural sources, controlling the stereoselectivity of the formation of 1,2-cis glycoside is extremely challenging in synthetic chemistry, as in the case of α-gluco (2-equatrial)- and β-manno (2-axial)-type glycoside formations (Figures 1Aa,b). To overcome this problem, various methods have been developed for the stereoselective synthesis of more difficult equatorial glycosides such as β-mannoside found in the core structure of the N-glycans (recent review; Ding et al., 2022a). This mini review enclosed the recent advances in stereoselective 1,2-cis-O-glycosylation (recent reviews; Nigudkar and Demchenko, 2015; Takahashi and Toshima, 2021; Manabe, 2021; Mukherjee et al., 2022) for the synthesis of various naturally occurring glycan structures. Donor structures are mainly focused on versatile glycosylation with various acceptor molecules (recent review; Leng et al., 2018) although the acceptor reactivity is also well-known as the important factor in controlling the selectivity of glycosylations (recent review; van der Vorm et al., 2019).
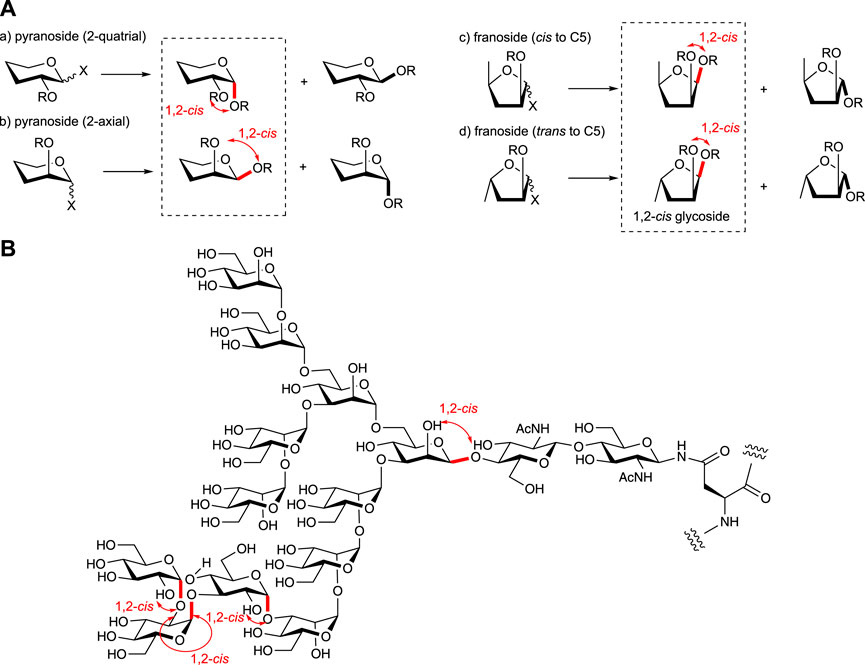
FIGURE 1. (A) Various types of glycosylations and (B) 1,2-cis glycosides in nature (high mannose-type N-glycan is shown as the example).
Recent advances on 1,2-cis glycosylations by intermolecular coupling
Recent development based on glycosyl donor modifications and reaction conditions for 1,2-cis glycosylation
For controlling the stereoselectivity of glycosylation, the protective groups on the donor moiety are well studied as one of the main factors (recent review, Csávás et al., 2021), including chiral auxiliary at 2-position (recent review, Mensink and Boltje, 2017). The cyclic protective groups on diols for the conformationally constrained donors (Jeanneret et al., 2020) could also be used for various glycosyl donors as one of the key stereocontrolling factors for glycosylation. The di-t-butylsilylene (DTBS) group, which was used to construct 1,2-cis glycoside as the protective group of the 4,6-O-galactosyl type donor (Imamura et al., 2003; Imamura et al., 2008a) and also used for GalNAc derivatives (Imamura et al., 2008b), was applied to the synthesis of the all-1,2-cis-linked repeating unit from the Acinetobacter baumannii D78 capsular polysaccharide (Njeri and Ragains, 2022). The cyclic protective groups are also effective in the case of well-studied arabinofuranosylations (review, Imamura and Lowary, 2011) by using 3,5-O- (Ishiwata et al., 2006; Zhu et al., 2006; Crich et al., 2007) and 2,3-O- (Ishiwata et al., 2006; Imamura and Lowary, 2010) cyclic protective groups such as DTBS, benzylidene, tetraisopropyldisiloxanilidene (TIPDS), and xylylene groups. These protective groups were used for conformational fixation of the flexible five-membered furanoside structure (Figure 1Ac) by the formation of bicyclic fused rings to control the approach of acceptor molecules. As an alternative use of the cyclic protective group on the furanoside ring, the 1,4-O-TIPDS-protected xylurofuranosyl donor has been developed for specific glycosylation to obtain 1,2-cis glycosides with various acceptors (Figure 2A1) (Huang and Lowary, 2020a; Huang and Lowary, 2020b) as in the case of a similarly constrained fructofuranosyl donor whose protective group is blocking one side of the approach of the acceptor (Oscarson and Sehgelmeble, 2000).
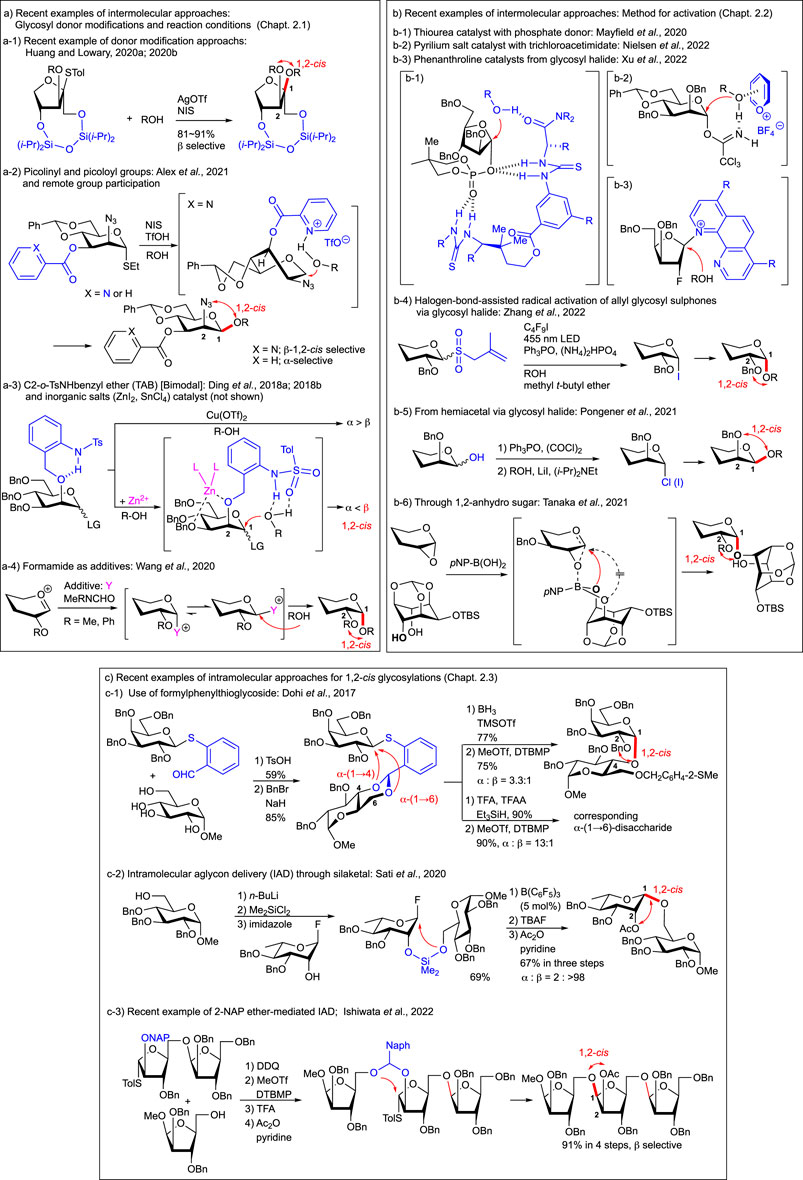
FIGURE 2. Recent advances in 1,2-cis glycosylations: some examples. (A) Recent examples of intermolecular approaches: Glycosyl donor modifications and reaction conditions. a-1) Recent example of donor modification approachs; a-2) Picolinyl and picoloyl groups and remote group participation; a-3) C2-o-TsNHbenzyl ether (TAB) [Bimodal] and inorganic salts (ZnI2, SnCl4) catalyst (not shown); a-4) Formamide as additives. (B) Recent examples of intermolecular approaches: Method for activation. b-1) Thiourea catalyst with phosphate donor; b-2) Pyrilium salt catalyst with trichloroacetimidate; b-3) Phenanthroline catalysts from glycosyl halide; b-4) halogen-bond-assisted radical activation of allyl glycosyl sulphones via glycosyl halide: b-5) From hemiacetal via glycosyl halide; b-6) Through 1,2-anhydro sugar. (C) Recent examples of intramolecular approaches for 1,2-cis glycosylations. c-1) Use of formylphenylthioglycoside; c-2) Intramolecular aglycon delivery (IAD) through silaketal; c-3) Recent example of 2-NAP ether-mediated IAD.
The protective group with hydrogen-bonding property such as picolinyl and picoloyl groups (Figure 2A2) (Pistorio et al., 2014; Alex et al., 2020) acts as the stereo-directing group for 1,2-cis glycosylation (Loh, 2021; recent review, Khanam and Mandal, 2022), which can also be applied to selective β-arabinofuranosylation (Li S et al., 2018) and synthesis of natual prodiuct such as Tiacumicin B (Norsikian et al., 2020; Tresse et al., 2021). The direct intramolecular neighboring and remote group participation of these groups to glycosyl cation led to the trans-glycosylation of the substituents as well (Yasomanee and Demchenko, 2012; McMillan and Crich, 2022).
Bimodal donors (recent review, Ding et al., 2022b) equipped with C2-o-TsNHbenzyl ether (TAB) not only for gluco-type glycosylation but also for manno-type glycosylation can be transformed to both anomers simply by switching reaction conditions (Figure 2A3) (Ding et al., 2018a; Ding et al., 2018b) whose optimizations have been carried out, including on the solvent (Ishiwata et al., 2008a; recent review, Mong et al., 2017) and on the concentrations (some examples: Chao et al., 2009; 2011; Ishiwata et al., 2010a; Kononov et al., 2012) of the O-glycosylation. In the case of gluco-type glycosylation of the trichloroacetimidate donor, screening of the reaction conditions revealed that TfOH was the most effective to afford 1,2-cis α-selective glycosylation at a lower concentration at room temperature and that triflimide (Tf2NH) (Kowalska and Pedersen, 2017) resulted in nearly complete 1,2-trans β-selectivity in EtCN at −78°C. On the other hand in the case of manno-type glycosylation, further screening of the catalyst (recent review, Nielsen and Pedersen, 2018) and the leaving group and thermodynamic conditions (Adamo and Kovác, 2007; Hou and Kovác, 2010) revealed that glycosyl diphenylphosphite (Kondo et al., 1994) was the best among all tested and catalytic amounts of Cu(OTf)2 (Mukaiyama et al., 1979; Sato et al., 1986) at 80°C or two equivalents of ZnI2 at −10°C afforded 1,2-trans α- or 1,2-cis β-selective glycosylations, respectively. The experimental results suggest that ZnI2 breaks the internal hydrogen bonding of the C2-o-TsNH benzyl group between C2-O and NH by coordination of one equivalent of ZnI2 with ether oxygen at cis-configured 2- and 3-positions of the mannosyl donor. The applications of this bimodal methodology to the stereocontrolled assembly of naturally occurring glucans having α/β-linkages to various positions of acceptors and branches have been shown recently (Ding et al., 2020).
As shown in many cases with donor modifications, reagent-controlled glycosylation (Yao et al., 2019) is also an important way for 1,2-cis glycosides. Recently, some practical methodologies have been reported. Additives such as DMF (Koto et al., 1984; Sato et al., 1986) and Ph3P=O were effectively used for the stereoselective construction of α-glucosyl linkages to secondary alcohols with TMSOTf and primary alcohols with TMSI, respectively (Wang et al., 2018; Njeri et al., 2021). The alternative nucleophilic additive for α-glycosylation methyl (phenyl) formamide (MPF) was found and applied to the synthesis of α-(1,4)-glucosamine and α-(1,4)-galactosamine linkages (Figure 2A4) (Wang et al., 2020; Zhang C et al., 2022). A simple ZnI2-directed strategy for 1,2-cis glycosylation bearing 4,6-O-tethered (Crich and Chandrasekera, 2004) glucosyl and mannosyl trichloroacetimidate donors has been developed with excellent stereoselectivity (Ding et al., 2021; Zhong et al., 2021). This simple strategy by the direction of SnCl4 instead of ZnI2 at −40°C afforded 1,2-cis glycoside when 0.1 equivalent was used, and by using three equivalents of SnCl4 at room temperature, we obtained 1,2-trans glycoside via product isomerization through plausible endo-cleavage supported by DFT calculations (Zhong et al., 2022). This also provides a more simple, mild, and effective bimodal glycosylation method. On the other hand, the recent examples for remote group participation (Hansen et al., 2020; Hettikankanamalage et al., 2020; Upadhyaya et al., 2021) introduced the 1,2-cis glycosylations selectively and practically from 6- [2,2-dimethyl-2-(ortho-nitrophenyl)acetyl: Liu et al., 2019; benzoyl: Shadrick et al., 2020; -C(=NPh)CF3: Liu et al., 2022a], 4- (levulinoyl: Zhang et al., 2021), and 3- [2-(diphenylphosphinoyl) acetyl: Liu et al., 2022a; Liu et al., 2022b] positions.
Recent advances in the method for activation of 1,2-cis glycosylation
In addition to various furanosyl phosphate donors (Figures 1Ac,d, 2B1) (Mayfield et al., 2020), the suitably protected mannosyl and rhamnosyl diphenylphosphate donors have been reported to be activated with the bis-thiourea catalyst as one of the organocatalytic approaches (Bradshaw et al., 2018; Xu and Loh, 2018 recent reviews, Mayfield et al., 2020; Park et al., 2017; ) afforded 1,2-cis glycoside in a highly selective manner (Li et al., 2020). It is noteworthy that a Schreiner thiourea catalyst with a halogen bond donor such as 2-iodoimidazolium salt has been developed to afford 1,2-cis N-glycoside from glycosyl trichloroacetimidate and amide of protected Asn (Li G et al., 2018). Pyrilium salt as an alternative organocatalyst effectively promotes the glycosylation of α and β-glycosyl trichloroacetimidate via SN2-type inversion to afford β- (1,2-cis manno-) and α- (1,2-cis gluco-) glycosides, respectively (Figure 2B2) (Nielsen et al., 2022).
Glycosyl halides are still considered one of the most useful and reactive intermediates for glycosidic bond formation as follows. First of all, promoted by phenanthroline catalysts as a recent alternative organocatalytic approach, various glycosyl bromide glycosides including both pyrano- and furanosides (as well as 2-fluoro sugars ) afforded 1,2-cis glycoside through glycosyl phenanthrolinium ion intermediates (Figure 2B3) (Yu et al., 2019; DeMent et al., 2021; Li and Nguyen, 2021; Xu et al., 2022). Second, halogen bond-assisted radical activation of allyl glycosyl sulphones was employed by forming halogen bond (review, Cavallo et al., 2016) complexes with perfluoroalkyl iodides under visible light irradiation conditions (Wan et al., 2021) via glycosyl radical intermediates (recent review, Chen et al., 2021) to give glycosyl iodides as the intermediate for glycosylation. Subsequent glycosylation in one pot afforded 1,2-cis glycoside by the effect of the ether solvent (Figure 2B4) (Zhang Y et al., 2022). Third, from the hemiacetal of mannose and rhamnose, 1,2-cis-β glycosides were obtained via dehydrative halogenation, followed by α-iodide formation mediated by lithium iodide (Figure 2B5) (Pongener et al., 2021). Since halide was used as the key intermediate in the latter two cases, the well-studied chemistry of halides for stereoselective glycosylation could be simply applied.
1,2-Anhydro sugar (Halcomb and Danishefsky., 1989; recent review; Li H et al., 2018), one of the activated forms of the 2-hydroxy-hemiacetal as an ultimately participated epoxide structure to give stereoelectronically favored 1,2-trans isomer, reacted from the opposite side of oxygen of epoxide, applied recently to regioselective (Tomita et al., 2020) and diastereoselective desymmetric 1,2-cis glycoside formations (Figure 2B6) (Tanaka et al., 2020) by the action of tetrahydroxydiboron with trans-diol and p-nitrophenylboronic acid with meso-diol, respectively (recent review, Takahashi et al., 2022). This SNi-type approach to 1,2-cis products supported by mechanistic studies was applied to the synthesis of core structures of phosphatidylinositolmannosides (PIMs) and glycosylphosphatidylinositol (GPI) anchors, as well as the common β-mannoside structure of the LLBM-782 series of antibiotics from meso-diol of m-inositol derivatives.
Recent examples using intramolecular coupling for 1,2-cis glycosylation
In order to get the 1,2-cis glycoside, stereospecifically, the procedure based on intramolecular aglycon delivery (IAD) (Barresi and Hindsgaul, 1991) (recent reviews, Ishiwata and Ito, 2017; Ishiwata, 2019; Fairbanks, 2021) is still one of the most promising methodologies despite the initial tethering between the glycosyl donor and acceptor residues before the intramolecular glycosidic bond formation reaction. However, a two-step procedure can precisely control the approach of the oxygen atom of the hydroxy group in the acceptor residue which was linked as the mixed acetal to the donor residue. IAD had been applied, especially to the β-mannoside linkage found in the core structure of the N-glycans, as one of the most difficult and attractive synthetic targets on stereoselective glycosylation. Although the effort on various intramolecular glycosylations via tethering using two non-reacting functional groups of both donors and acceptors has also been carried out for both 1,2-cis and 1,2-trans selective glycosylations (Jia and Demchenko, 2017), it has been shown as one of the alternative intramolecular methods that the leaving group functionalization in the donor moiety as in the case of o-formylphenyl thioglycoside obtained from non-malodurous calicyl-type thioglycoside (Dohi et al., 2021) can be used for the tethering with the diol acceptor and regioselective ring opening of S-donor-substituted benzylidene acetal, followed by intramolecular glycosylation with the resultant exposed hydroxy group to afford 1,2-cis glycosides over three steps (Figure 2C1) (Dohi et al., 2017). Improvements for IAD have also been achieved by tethering to hydroxy groups on both residues through the silaketal (Stork and Kim, 1992) from sugar silanes (Figure 2C2) (Walk et al., 2015; Sati et al., 2020). In the case of the IAD, the 2-O-mixed acetal linkage and the axial O-mixed acetal substituent as the precursor for intramolecular transfer seem to be kinetically and stereoelectronically favored as in the case of neighboring group participation of acyl groups. p-Methoxybenzyl (PMB) ether-mediated IAD was well known as the most practical method to be applied for the synthesis of β-mannoside in N-glycan (Ito and Ogawa, 1994). The effective oxidative one-step linking of axially configured 2-O-PMB ether to produce a corresponding p-methoxybenzylidene mixed acetal at the 2-O-position of the mannosy donor with the acceptor as an aglycone. For this method to be more versatile, suitable stabilization of the mixed acetal intermediate by introducing the 2-naphthylidene acetal (Ishiwata et al., 2008b; reviews, Ishiwata et al., 2010b; Ishiwata and Ito, 2012) has been developed for various 1,2-cis linkages for application to the synthesis of plant β-L-arabinofuranosides (Figure 2C3) (Kaeothip et al., 2013a; Kaeothip et al., 2013b; Ishiwata et al., 2014; Ishiwata et al., 2022) and various other types of glycosides (Ishiwata et al., 2011; Ishiwata and Ito, 2011; Tamigney et al., 2014; Robinson et al., 2020) including β-L-rhamnosyl linkage (Lee et al., 2008; Yu et al., 2016; recent review, Rai and Kulkarni, 2021).
Summary
This minireview introduced some of the recent advances in the development of stereoselective 1,2-cis-O-glycosylation, for the synthesis of various naturally occurring glycan structures possessing α-glucopyranoside, α-galactopyranoside, β-mannopyranoside, β-arabinofuranoside, and other rare glycosides. Donor structures that mainly focused on versatile glycosylation with various acceptor molecules were shown from recent examples and from further matured ones, such as controlling by glycosyl donor modification and reaction conditions and novel methods for activation of intermolecular glycosylation including the bimodal glycosylation strategy for 1,2-cis and 1,2-trans glycosides, as well as intramolecular glycosylations, including recent applications of NAP-ether-mediated intramolecular aglycon delivery. As in the case of novel methods for activation through glycosyl halides shown in this minireview, it was strongly suggested that the previously studied chemistry of known glycosyl donors for stereoselective glycosylation, including 1,2-cis glycosylation, has similar potential to be simply applied in combination with the novel activation methodology, although it must be well optimized as demonstrated previously for practical use. In many recent cases, the results of stereoselectivity and the pathways of glycosylations have been explained by mechanistic studies using highly optimized density functional theory (DFT) calculations and other organic and enzymatic reactions for our better understanding. In the case of many examples shown in this minireview, hybrid functionals such as B3LYP and M06, as well as double hybrid, ωB97X-D were selected to be used as various basis sets [6-31 + G(d,p), 6-31G(d), 6-31G*, 6-31 + G**, Def2SVPP and Def2TZVP] with/without D3BJ dispersion corrections and in combination with a polarizable continuum model (PCM) or implicit solvation model based on density (SMD) for each solvent. DFT calculations could discuss the evidence of glycosyl cation species as well (review, Merino et al., 2021). However, it is still difficult to compare with other experimental results in different studies as we always had a difficult time understanding the outcome of glycosylation and predicting the reactivity, especially stereoselectivity (Chang et al., 2020; Chang et al., 2021). Only through our continuous effort to gain a clear understanding of it from a multidirectional point of view, 1,2-cis glycosylations would be finally controlled to be well-predictable as 1,2-trans glycosylation.
Author contributions
AI, FD, and YI wrote sections of the manuscript. All authors contributed to manuscript revision, read, and approved the submitted version.
Funding
This work was partly supported by a grant-in-aid for Specially Promoted Research (No. 16H06290 to AI, YI) from the Ministry of Education, Culture, Sports, Science, and Technology, and for Scientific Research (Nos 18K05345 and 19H00929 to AI; No. 22H02196 to YI) from the Japan Society for the Promotion of Science, the Fundamental Research Funds for the Central Universities (No. 31610011 to FD) from China, the Sun Yat-Sen University Startup fund (No. 18841224 to FD), and the Natural Science Foundation of Guangdong Province (No. 42030015 to FD).
Acknowledgments
The authors thank Ms. Masayo Ohara for her technical assistance.
Conflict of interest
The authors declare that the research was conducted in the absence of any commercial or financial relationships that could be construed as a potential conflict of interest.
Publisher’s note
All claims expressed in this article are solely those of the authors and do not necessarily represent those of their affiliated organizations, or those of the publisher, the editors, and the reviewers. Any product that may be evaluated in this article, or claim that may be made by its manufacturer, is not guaranteed or endorsed by the publisher.
References
Adamo, R., and Kovác, P. (2007). Glycosylation under thermodynamic control: Synthesis of the di- and the hexasaccharide fragments of the O-sp of Vibrio cholerae O:1 serotype Ogawa from fully functionalized building blocks. Eur. J. Org. Chem. 2007, 988–1000. doi:10.1002/ejoc.200600851
Alex, C., Visamsirikul, S., and Demchenko, A. V. (2020). A versatile approach to the synthesis of mannosamine glycosides. Org. Biomol. Chem. 18, 6682–6695. doi:10.1039/D0OB01640C
Andrew, B., Metternich, J. B., Trotta, A. H., and Jacobsen, E. N. (2020). Stereospecific furanosylations catalyzed by bis-thiourea hydrogen-bond donors. J. Am. Chem. Soc. 142, 4061–4069. doi:10.1021/jacs.0c00335
Barresi, F., and Hindsgaul, O. (1991). Synthesis of .beta.-mannopyranosides by intramolecular aglycon delivery. J. Am. Chem. Soc. 113, 9376–9377. doi:10.1021/ja00024a057
Bradshaw, G. A., Colgan, A. C., Allen, N. P., Pongener, I., Boland, M. B., Ortin, Y., et al. (2018). Stereoselective organocatalyzed glycosylations – thiouracil, thioureas and monothiophthalimide act as Brønsted acid catalysts at low loadings. Chem. Sci. 10, 508–514. doi:10.1039/c8sc02788a
Cavallo, G., Metrangolo, P., Milani, R., Pilati, T., Priimagi, A., Resnati, G., et al. (2016). The halogen bond. Chem. Rev. 116, 2478–2601. doi:10.1021/acs.chemrev.5b00484
Chang, C.-W., Lin, M.-H., Chan, C.-K., Su, K.-Y., Wu, C.-H., Lo, W.-C., et al. (2021). Automated quantification of hydroxyl reactivities: Prediction of glycosylation reactions. Angew. Chem. Int. Ed. 60, 12413–12423. doi:10.1002/anie.202013909
Chang, C.-W., Lin, M.-H., and Wang, C.-C. (2020). Statistical analysis of glycosylation reactions. Chem. Eur. J. 27, 2556–2568. doi:10.1002/chem.202003105
Chao, C.-S., Li, C.-W., Chen, M.-C., Chang, S.-S., and Mong, K.-K. T. (2009). Low-concentration 1, 2-transβ-Selective glycosylation strategy and its applications in oligosaccharide synthesis. Chem. Eur. J. 15, 10972–10982. doi:10.1002/chem.200901119
Chao, C.-S., Lin, C.-Y., Mulani, S., Hung, W.-C., and Mong, K.-K. T. (2011). Neighboring-group participation by C-2 ether functions in glycosylations directed by nitrile solvents. Chem. Eur. J. 17, 12193–12202. doi:10.1002/chem.201100732
Chen, A., Xu, L., Zhou, Z., Zhao, S., Yang, T., Zhu, F., et al. (2021). Recent advances in glycosylation involving novel anomeric radical precursors. J. Carbohydr. Chem. 40, 361–400. doi:10.1080/07328303.2022.2031207
Crich, D., and Chandrasekera, N. S. (2004). Mechanism of 4, 6-O-Benzylidene-Directed β-mannosylation as determined by α-deuterium kinetic isotope effects. Angew. Chem. Int. Ed. 43, 5386–5389. doi:10.1002/anie.200453688
Crich, D., Pedersen, C. M., Bowers, A. A., and Wink, D. J. (2007). On the use of 3, 5-O-benzylidene and 3, 5-O-(Di-tert-butylsilylene)-2-O-benzylarabinothiofuranosides and their sulfoxides as glycosyl donors for the synthesis of β-arabinofuranosides: Importance of the activation method. J. Org. Chem. 72, 1553–1565. doi:10.1021/jo061440x
Csávás, M., Herczeg, M., Bajza, I., and Borbás, A. (2021). Protecting group manipulations in carbohydrate synthesis. Compr. Glycosci. 2, 464–524. doi:10.1016/B978-0-12-819475-1.00087-0
DeMent, P. M., Liu, C. L., Wakpal, J., Schaugaard, R. N., Schlegel, H. B., Nguyen, H. M., et al. (2021). Phenanthroline-Catalyzed stereoselective formation of alpha-1, 2-cis 2-deoxy-2-fluoro glycosides. ACS Catal. 11, 2108–2120. doi:10.1021/acscatal.0c04381
Ding, F., Ishiwata, A., and Ito, Y. (2018a). Bimodal glycosyl donors protected by 2-O-(ortho-Tosylamido)benzyl group. Org. Lett. 20, 4384–4388. doi:10.1021/acs.orglett.8b01922
Ding, F., Ishiwata, A., and Ito, Y. (2022b). Recent advances of the stereoselective bimodal glycosylations for the synthesis of various glucans. Stud. Nat. Prod. Chem. 74, 1–40. doi:10.1016/B978-0-323-91099-6.00001-3
Ding, F., Ishiwata, A., and Ito, Y. (2018b). Stereodivergent mannosylation using 2-O-(ortho-Tosylamido)benzyl group. Org. Lett. 20, 4833–4837. doi:10.1021/acs.orglett.8b01979
Ding, F., Ishiwata, A., Zhou, S., Zhong, X., and Ito, Y. (2020). Unified strategy toward stereocontrolled assembly of various glucans based on bimodal glycosyl donors. J. Org. Chem. 85, 5536–5558. doi:10.1021/acs.joc.0c00292
Ding, F., Zhao, X., Huang, Y., Zhou, S., Ao, J., Cai, H., et al. (2022a). Recent chemical and chemoenzymatic strategies to complex-type N-glycans. Front. Chem. 10, 880128. doi:10.3389/fchem.2022.880128
Ding, F., Zhou, S., Zhong, X., Guo, A., Xiao, Q., Ao, J., et al. (2021). ZnI2-directed stereocontrolled α-glucosylation. Org. Lett. 23, 6841–6845. doi:10.1021/acs.orglett.1c02405
Dohi, H., Komai, R., Sakai, H., Komuro, H., and Nishida, Y. (2017). Convenient use of o-formylphenyl thioglycoside for regioselective conjugation with glycosyl acceptors towards development of regioselective 1, 2-cis glycosylation. J. Carbohydr. Chem. 36, 307–324. doi:10.1080/07328303.2017.1403612
Dohi, H., Sakurai, R., Tamura, M., Komai, R., and Nishida, Y. (2021). Evaluating the reactivity and stereoselectivity of salicyl-type thioglycosides as non-malodorous thioglycoside alternatives for oligosaccharide synthesis. J. Carbohydr. Chem. 40, 45–65. doi:10.1080/07328303.2021.1921787
Fairbanks, A. J. (2021). “Glycosylation through intramolecular aglycon delivery,”. Editor J. Barchi, Vol. 2, 413–434. doi:10.1016/B978-0-12-409547-2.14950-9Compr. Glycosci.
Halcomb, R. L., and Danishefsky, S. J. (1989). On the direct epoxidation of glycals: Application of a reiterative strategy for the synthesis of .beta.-linked oligosaccharides. J. Am. Chem. Soc. 111, 6661–6666. doi:10.1021/ja00199a028
Hansen, T., Elferink, H., van Hengst, J. M. A., Houthuijs, K. J., Remmerswaal, W. A., Kromm, A., et al. (2020). Characterization of glycosyl dioxolenium ions and their role in glycosylation reactions. Nat. Commun. 11, 2664. doi:10.1038/s41467-020-16362-x
Hettikankanamalage, A. A., Lassfolk, R., Ekholm, F. S., Leino, R., and Crich, D. (2020). Mechanisms of stereodirecting participation and ester migration from near and far in glycosylation and related reactions. Chem. Rev. 120, 7104–7151. doi:10.1021/acs.chemrev.0c00243
Hou, S., and Kovác, P. (2010). Enhanced stereoselectivity of α-mannosylation under thermodynamic control using trichloroacetimidates. Carbohydr. Res. 345, 999–1007. doi:10.1016/j.carres.2010.03.025
Huang, B.-S., and Lowary, T. L. (2020a). A siloxane-bridged glycosyl donor enables highly stereoselective β‐xylulofuranosylation. J. Org. Chem. 85, 15895–15907. doi:10.1021/acs.joc.0c01008
Huang, B.-S., and Lowary, T. L. (2020b). β‐Selective xylulofuranosylation via a conformationally-restricted glycosyl donor. Org. Biomol. Chem. 18, 2264–2273. doi:10.1039/d0ob00260g
Imamura, A., Ando, H., Ishida, H., and Kiso, M. (2008a). DTBS (di-tert-butylsilylene)-directed α-galactosylation for the synthesis of biologically relevant glycans. Curr. Org. Chem. 12, 675–689. doi:10.2174/138527208784577358
Imamura, A., Ando, H., Ishida, H., and Kiso, M. (2008b). DTBS effect: The unique sterically driven director for a-galactosylation. Heterocycles 76, 883. doi:10.3987/REV-08-SR(N)4
Imamura, A., Ando, H., Korogi, S., Tanabe, G., Muraoka, O., Ishida, H., et al. (2003). Di-tert-butylsilylene (DTBS) group-directed α-selective galactosylation unaffected by C-2 participating functionalities. Tetrahedron Lett. 44, 6725–6728. doi:10.1016/S0040-4039(03)01647-2
Imamura, A., and Lowary, T. L. (2011). Chemical synthesis of furanose glycosides. Trends Glycosci. Glycotechnol. 23, 134–152. doi:10.4052/tigg.23.134
Imamura, A., and Lowary, T. L. (2010). β-Selective arabinofuranosylation using a 2, 3-O-Xylylene-Protected donor. Org. Lett. 12, 3686–3689. doi:10.1021/ol101520q
Ishiwata, A., Akao, H., and Ito, Y. (2006). Stereoselective synthesis of a fragment of mycobacterial arabinan. Org. Lett. 8, 5525–5528. doi:10.1021/ol062198j
Ishiwata, A., Fujita, K., Fushinobu, S., Tanaka, K., and Ito, Y. (2022). Synthesis of naturally occurring β-L-arabinofuranosyl-L-arabinofuranoside structures towards the substrate specificity evaluation of β-L-arabinofuranosidase. Bioorg. Med. Chem. 67, 116849. doi:10.1016/j.bmc.2022.116849
Ishiwata, A., and Ito, Y. (2012). Intramolecular aglycon delivery and its application to stereoselective synthesis of glycans. J. Synth. Org. Chem. Jpn. 70, 382–394. doi:10.5059/yukigoseikyokaishi.70.382
Ishiwata, A., and Ito, Y. (2017). “Intramolecular aglycon delivery toward 1,2-cis selective glycosylation,” in Selective glycosylations: Synthetic methods and catalysts. Editor S. Bennett Clay (Weinheim, Germany: Wiley VCH), 79–96. doi:10.1002/9783527696239.ch4
Ishiwata, A., and Ito, Y. (2011). Synthesis of docosasaccharide arabinan motif of mycobacterial cell wall. J. Am. Chem. Soc. 133, 2275–2291. doi:10.1021/ja109932t
Ishiwata, A., Kaeothip, S., Takeda, Y., and Ito, Y. (2014). Synthesis of the highly glycosylated hydrophilic motif of extensins. Angew. Chem. Int. Ed. 53, 9812–9816. doi:10.1002/anie.201404904
Ishiwata, A., Lee, Y. J., and Ito, Y. (2010b). Recent advances in stereoselective glycosylation through intramolecular aglycon delivery. Org. Biomol. Chem. 8, 3596. doi:10.1039/C004281A
Ishiwata, A., Munemura, Y., and Ito, Y. (2008a). NAP ether mediated intramolecular aglycon delivery: A unified strategy for 1, 2-cis-Glycosylation. Eur. J. Org. Chem. 2008, 4250–4263. doi:10.1002/ejoc.200800249
Ishiwata, A., Munemura, Y., and Ito, Y. (2008b). Synergistic solvent effect in 1, 2-cis-glycoside formation. Tetrahedron 64, 92–102. doi:10.1016/j.tet.2007.10.087
Ishiwata, A., Sakurai, A., Dürr, K., and Ito, Y. (2010a). Effects of frozen conditions on stereoselectivity and velocity of O-glycosylation reactions. Bioorg. Med. Chem. 18, 3687–3695. doi:10.1016/j.bmc.2010.04.013
Ishiwata, A., Sakurai, A., Nishimiya, Y., Tsuda, S., and Ito, Y. (2011). Synthetic study and structural analysis of the antifreeze agent xylomannan from upis ceramboides. J. Am. Chem. Soc. 113, 19524–19535. doi:10.1021/ja208528c
Ishiwata, A. (2019). Synthetic study on glycoconjugates containing 1, 2-cis glycoside and their application. Trends Glycosci. Glycotechnol. 31, SE53–SE54. doi:10.4052/tigg.1925.2SE
Ito, Y., and Ogawa, T. (1994). A novel approach to the stereoselective synthesis of β-mannosides. Angew. Chem. Int. Ed. Engl. 33, 1765–1767. doi:10.1002/anie.199417651
Jeanneret, R. A., Johnson, S. E., and Galan, M. C. (2020). Conformationally constrained glycosyl donors as tools to control glycosylation outcomes. J. Org. Chem. 85, 15801–15826. doi:10.1021/acs.joc.0c02045
Jia, X. G., and Demchenko, A. V. (2017). Intramolecular glycosylation. Beilstein J. Org. Chem. 13, 2028–2048. doi:10.3762/bjoc.13.201
Kaeothip, S., Ishiwata, A., Ito, T., Fushinobu, S., Fujita, K., Ito, Y., et al. (2013b). Preparation of p-nitrophenyl β-L-arabinofuranoside as the substrate of β-L-arabinofuranosidase. Carbohydr. Res. 382, 95–100. doi:10.1016/j.carres.2013.10.005
Kaeothip, S., Ishiwata, A., and Ito, Y. (2013a). Stereoselective Synthesis of Arabidopsis CLAVATA3 (CLV-3) glycopeptide, unique protein post-translational modifications of secreted peptide hormone in plant. Org. Biomol. Chem. 11, 5892. doi:10.1039/C3OB41212A
Khanam, A., and Mandal, P. K. (2022). Influence of remote picolinyl and picoloyl stereodirecting groups for the stereoselective glycosylation. Asian J. Org. Chem. 10, 296–314. doi:10.1002/ajoc.202000558
Kondo, H., Aoki, S., Ichikawa, Y., Halcomb, R. L., Ritzen, H., Wong, C.-H., et al. (1994). Glycosyl phosphites as glycosylation reagents: Scope and mechanism. J. Org. Chem. 59, 864–877. doi:10.1021/jo00083a032
Kononov, L. O., Malysheva, N. N., Orlova, A. V., Zinin, A. I., Laptinskaya, T. V., Kononova, E. G., et al. (2012). Concentration dependence of glycosylation outcome: A clue to reproducibility and understanding the reasons behind. Eur. J. Org. Chem. 2012, 1926–1934. doi:10.1002/ejoc.201101613
Koto, S., Morishima, N., and Zen, S. A. (1984). Stereoselective α-glucosylation by use of a mixture of 4-nitrobenzenesulfonyl chloride, silver trifluoromethane sulfonate, N, N-dimethylacetamide and trietheylamine. Carbohydr. Res. 130, 73–83. doi:10.1016/0008-6215(84)85271-4
Kowalska, K., and Pedersen, C. M. (2017). Catalytic stereospecific O-glycosylation. Chem. Commun. 53, 2040–2043. doi:10.1039/C6CC10076G
Lee, Y. J., Ishiwata, A., and Ito, Y. (2008). Stereoselective synthesis of β-L-rhamnopyranosides. J. Am. Chem. Soc. 130, 6330–6331. doi:10.1021/ja801574q
Leng, W.-L., Yao, H., He, J.-X., and Liu, X.-W. (2018). Venturing beyond donor-controlled glycosylation: New perspectives toward anomeric selectivity. Acc. Chem. Res. 51, 628–639. doi:10.1021/acs.accounts.7b00449
Li, G., Noguchi, M., Serizawa, K., and Shoda, S.-I. (2018). Chemistry of 1, 2-anhydro sugars. Chimia 72, 874. doi:10.2533/chimia.2018.874
Li, H. Z., Ding, J., Cheng, C.-R., Chen, Y., and Liang, X.-Y. (2018). β‐L-Arabinofuranosylation conducted by 5-O-(2-pyridinecarbonyl)-L-arabinofuranosyl trichloroacetimidate. Carbohydr. Res. 460, 1–7. doi:10.1016/j.carres.2018.02.006
Li, J. Y., and Nguyen, H. M. A. (2021). Mechanistic probe into 1, 2-cis glycoside formation catalyzed by phenanthroline and further expansion of scope. Adv. Synth. Catal. 363, 4054–4066. doi:10.1002/adsc.202100639
Li, Q., Levi, S. M., and Jacobsen, E. N. J. (2020). Highly selective β-mannosylations and β-rhamnosylations catalyzed by bis-thiourea. J. Am. Chem. Soc. 142 (27), 11865–11872. doi:10.1021/jacs.0c04255
Li, S., Kobayashi, Y., and Takemoto, Y. (2018). Organocatalytic direct α-selective N-glycosylation of amide with glycosyl trichloroacetimidate. Chem. Pharm. Bull. 66, 768–770. doi:10.1248/cpb.c18-00255
Liu, H., Hansen, T., Zhou, S. Y., Wen, G. E., Liu, X. X., Zhang, Q. J., et al. (2019). Dual-participation protecting group solves the anomeric stereocontrol problems in glycosylation reactions. Org. Lett. 21, 8713–8717. doi:10.1021/acs.orglett.9b03321
Liu, X., Lin, Y., Liu, A., Sun, Q., Sun, H., Xu, P., et al. (2022b). 2-Diphenylphosphinoyl-acetyl as a remote directing group for the highly stereoselective synthesis of β-glycosides. Chin. J. Chem. 40, 443–452. doi:10.1002/cjoc.202100865
Liu, X., Song, Y., Liu, A., Zhou, Y., Zhu, Q., Lin, Y., et al. (2022a). More than a leaving group: N-phenyltrifluoroacetimidate as a remote directing group for highly α-selective 1, 2-cis glycosylation. Angew. Chem. Int. Ed. Engl. 61, e202201510. doi:10.1002/anie.202201510
Loh, C. C. J. (2021). Exploiting non-covalent interactions in selective carbohydrate synthesis. Nat. Rev. Chem. 5, 792–815. doi:10.1038/s41570-021-00324-y
Manabe, S. (2021). Recent development of stereoselective glycosylation reactions. Heterocycles 102, 177–210. doi:10.3987/REV-20-933
Mayfield, A. B., Metternich, J. B., Trotta, A. H., and Jacobsen, E. N. (2020). Stereospecific furanosylations catalyzed by bis-thiourea hydrogen-bond donors. J. Am. Chem. Soc. 142, 4061–4069. doi:10.1021/jacs.0c00335
McMillan, T. F., and Crich, D. (2022). Influence of 3-thio substituents on benzylidene-directed mannosylation. Isolation of a bridged pyridinium ion and effects of 3-O-picolyl and 3-S-picolyl esters. Eur. J. Org. Chem. 2022, e202200320. doi:10.1002/ejoc.202200320
Mensink, R. A., and Boltje, T. J. (2017). Advances in stereoselective 1, 2-cis glycosylation using C-2 auxiliaries. Chem. Eur. J. 23, 17637–17653. doi:10.1002/chem.201700908
Merino, P., Delso, I., Pereira, S., Orta, S., Pedrón, M., Tejero, T., et al. (2021). Computational evidence of glycosyl cations. Org. Biomol. Chem. 19, 2350–2365. doi:10.1039/D0OB02373F
Mong, K.-K. T., Nokami, T., Thi, N., Tran, T., and Nhi, P. B. (2017). “Solvent effect on glycosylation,” in Selective glycosylations: Synthetic methods and catalysts. Editor S. Bennett Clay (Weinheim, Germany: Wiley VCH), 55–77. doi:10.1002/9783527696239.ch3
Mukaiyama, T., Nakatsuka, T., and Shoda, S.-i. (1979). An efficient glucosylation of alcohol using 1-thioglucoside derivative. Chem. Lett. 8, 487–490. doi:10.1246/cl.1979.487
Mukherjee, M. M., Ghosh, R., and Hanover, J. A. (2022). Recent advances in stereoselective chemical O-glycosylation reactions. Front. Mol. Biosci. 9, 896187. doi:10.3389/fmolb.2022.896187
Nielsen, M. M., Holmstrøm, T., and Pedersen, C. M. (2022). Stereoselective O‐glycosylations by pyrylium salt organocatalysis. Angew. Chem. Int. Ed. Engl. 61, e202115394. doi:10.1002/anie.202115394
Nielsen, M. M., and Pedersen, C. M. (2018). Catalytic glycosylations in oligosaccharide synthesis. Chem. Rev. 118 (17), 8285–8358. doi:10.1021/acs.chemrev.8b00144
Nigudkar, S. S., and Demchenko, A. V. (2015). Stereocontrolled 1, 2-cis glycosylation as the driving force of progress in synthetic carbohydrate chemistry. Chem. Sci. 6, 2687–2704. doi:10.1039/C5SC00280J
Njeri, D. K., and Ragains, J. R. (2022). Total synthesis of an all-1, 2-cis-Linked repeating unit from the Acinetobacter baumannii D78 capsular polysaccharide. Org. Lett. 24, 3461–3465. doi:10.1021/acs.orglett.2c01034
Njeri, D. K., Valenzuela, E. A., and Ragains, J. R. (2021). Leveraging trifluoromethylated benzyl groups toward the highly 1, 2-cis-selective glucosylation of reactive alcohols. Org. Lett. 23, 8214–8218. doi:10.1021/acs.orglett.1c02947
Norsikian, S., Tresse, C., François-Eude, M., Jeanne-Julien, L., Masson, G., Servajean, V., et al. (2020). Total synthesis of tiacumicin B: Implementing hydrogen bond directed acceptor delivery for highly selective β-glycosylations. Angew. Chem. Int. Ed. 59, 6612–6616. doi:10.1002/anie.202000231
Oscarson, S., and Sehgelmeble, F. W. (2000). A novel β-directing fructofuranosyl donor concept. Stereospecific synthesis of sucrose. J. Am. Chem. Soc. 122 (37), 8869–8872. doi:10.1021/ja001439u
Park, Y., Harper, K. C., Kuhl, N., Kwan, E. E., Liu, R. Y., Jacobsen, E. N., et al. (2017). Macrocyclic bis-thioureas catalyze stereospecific glycosylation reactions. Science 355, 162–166. doi:10.1126/science.aal1875
Pistorio, S. G., Yasomanee, J. P., and Demchenko, A. V. (2014). Hydrogen-bond-mediated aglycone delivery: Focus on β-mannosylation. Org. Lett. 16, 716–719. doi:10.1021/ol403396j
Pongener, I., Pepe, D. A., Ruddy, J. J., and McGarrigle, E. M. (2021). Stereoselective β-mannosylations and β-rhamnosylations from glycosyl hemiacetals mediated by lithium iodide. Chem. Sci. 12, 10070–10075. doi:10.1039/D1SC01300A
Rai, D., and Kulkarni, S, S. (2021). Recent advances in β-L-rhamnosylation. Org. Biomol. Chem. 18, 3216–3228. doi:10.1039/D0OB00297F
Robinson, S. A., Yau, J., Terabe, M., Berzofsky, J. A., Painter, G. F., Compton, B. J., et al. (2020). Synthetic preparation and immunological evaluation of β-mannosylceramide and related N-acyl analogues. Org. Biomol. Chem. 18, 2739–2746. doi:10.1039/D0OB00223B
Sati, G. C., Martin, J. L., Xu, Y., Malakar, T., Zimmerman, P. M., Montgomery, J., et al. (2020). Fluoride migration catalysis enables simple, stereoselective, and iterative glycosylation. J. Am. Chem. Soc. 142, 7235–7242. doi:10.1021/jacs.0c03165
Sato, S., Mori, M., Ito, Y., and Ogawa, T. (1986). An efficient approach to O-glycosides through CuBr2-Bu4NBr mediated activation of glycosides. Carbohydr. Res. 155, C6–C10. doi:10.1016/s0008-6215(00)90163-0
Shadrick, M., Singh, Y., and Demchenko, A. V. (2020). Stereocontrolled α‐galactosylation under cooperative catalysis. J. Org. Chem. 85, 15936–15944. doi:10.1021/acs.joc.0c01279
Stork, G., and Kim, G. (1992). Stereocontrolled synthesis of disaccharides via the temporary silicon connection. J. Am. Chem. Soc. 114, 1087–1088. doi:10.1021/ja00029a047
Takahashi, D., Inaba, K., and Toshima, K. (2022). Recent advances in boron-mediated aglycon delivery (BMAD) for the efficient synthesis of 1, 2-cis glycosides. Carbohydr. Res. 518, 108579. doi:10.1016/j.carres.2022.108579
Takahashi, D., and Toshima, K. (2021). 1,2-cis O-glycosylation methods. Compr. Glycosci. 2, 365–412. doi:10.1016/B978-0-12-819475-1.00016-X
Tamigney, M., Blériot, K. Y., and Gauthier, C. (2014). Intramolecular aglycon delivery enables the synthesis of 6-Deoxy-β-D-manno-heptosides as fragments of burkholderia pseudomallei and Burkholderia mallei capsular polysaccharide. J. Org. Chem. 79, 4615–4634. doi:10.1021/jo500640n
Tanaka, M., Sato, K., Yoshida, R., Nishi, N., Oyamada, R., Inaba, K., et al. (2020). Diastereoselective desymmetric 1, 2-cis-glycosylation of meso-diols via chirality transfer from a glycosyl donor. Nat. Commun. 11, 2431. doi:10.1038/s41467-020-16365-8
Tomita, S., Tanaka, M., Inoue, M., Inaba, K., Takahashi, D., Toshima, K., et al. (2020). Diboron-Catalyzed regio- and 1, 2-cis-α-Stereoselective glycosylation of trans-1, 2-diols. J. Org. Chem. 85, 16254–16262. doi:10.1021/acs.joc.0c02093
Tresse, C., François-Heude, M., Servajean, V., Ravinder, R., Lesieur, C., Geiben, L., et al. (2021). Total synthesis of tiacumicin B: study of the challenging β-selective glycosylations. Chem. Eur. J. 27, 5230–5239. doi:10.1002/chem.202005102
Upadhyaya, K., Subedi, Y. P., and Crich, D. (2021). Direct experimental characterization of a bridged bicyclic glycosyl dioxacarbenium ion by 1 H and 13 C NMR spectroscopy: Importance of conformation on participation by distal esters. Angew. Chem. Int. Ed. 22, 25397–25403. doi:10.1002/anie.202110212
van der Vorm, S., Hansen, T., van Hengst, J. M. A., Overkleeft, H. S., van der Marel, G. A., Codée, J. D. C., et al. (2019). Acceptor reactivity in glycosylation reactions. Chem. Soc. Rev. 48, 4688–4706. doi:10.1039/C8CS00369F
Walk, J. T., Buchan, Z. A., and Montgomery, J. (2015). Sugar silanes: Versatile reagents for stereocontrolled glycosylation via intramolecular aglycone delivery. Chem. Sci. 6, 3448–3453. doi:10.1039/C5SC00810G
Wan, L.-Q., Zhang, X., Zou, Y., Shi, R., Cao, J.-G., Xu, S.-Y., et al. (2021). Nonenzymatic stereoselective S-glycosylation of polypeptides and proteins. J. Am. Chem. Soc. 143, 11919–11926. doi:10.1021/jacs.1c05156
Wang, L., Overkleeft, H. S., van der Marel, G. A., and Codée, J. D. C. (2018). Reagent controlled stereoselective synthesis of α-glucans. J. Am. Chem. Soc. 140 (140), 4632–4638. doi:10.1021/jacs.8b00669
Wang, L., Zhang, Y., Overkleeft, H. S., van der Marel, G. A., and Codée, J. D. C. (2020). Reagent controlled glycosylations for the assembly of well-defined pel oligosaccharides. J. Org. Chem. 85, 15872–15884. doi:10.1021/acs.joc.0c00703
Xu, C., and Loh, C. C. J. (2018). An ultra-low thiourea catalyzed strain-release glycosylation and a multicatalytic diversification strategy. Nat. Commun. 9, 4057. doi:10.1038/s41467-018-06329-4
Xu, H., Schaugaard, R. N., Li, J., Schlegel, H. B., and Nguyen, H. M. (2022). Stereoselective 1, 2-cis furanosylations catalyzed by phenanthroline. J. Am. Chem. Soc. 144, 7441–7456. doi:10.1021/jacs.2c02063
Yadav, R. N., Hossain, Md. F., Das, A., Srivastava, A. K., and Banik, B. K. (2022). Organocatalysis: A recent development on stereoselective synthesis of o-glycosides. Catal. Rev., 1–118. doi:10.1080/01614940.2022.2041303
Yao, H., Vu, M. D., and Liu, X.-W. (2019). Recent advances in reagent-controlled stereoselective/stereospecific glycosylation. Carbohydr. Res. 473, 72–81. doi:10.1016/j.carres.2018.10.006
Yasomanee, J. P., and Demchenko, A. V. (2012). Effect of remote picolinyl and picoloyl substituents on the stereoselectivity of chemical glycosylation. J. Am. Chem. Soc. 134, 20097–20102. doi:10.1021/ja307355n
Yu, F., Li, J. Y., DeMent, P. M., Tu, Y. J., Schlegel, H. B., Nguyen, H. M., et al. (2019). Phenanthroline-Catalyzed stereoretentive glycosylations. Angew. Chem. Int. Ed. 58, 6957–6961. doi:10.1002/anie.201901346
Yu, K., Qiao, Y., Gu, G., Gao, J., Cai, S., Long, Z., et al. (2016). Synthesis of the biological repeating unit of Streptococcus pneumoniae serotype 23F capsular polysaccharide. Org. Biomol. Chem. 14, 11462–11472. doi:10.1039/C6OB02363K
Zhang, C., Zuo, H., Lee, G. Y., Zou, Y., Dang, Q.-D., Houk, K. N., et al. (2022). Halogen-bond-assisted radical activation of glycosyl donors enables mild and stereoconvergent 1, 2-cis-glycosylation. Nat. Chem. 14, 686–694. doi:10.1038/s41557-022-00918-z
Zhang, Y., Wang, L., Overkleeft, H. S., van der Marel, G. A., and Codée, J. D. C. (2022). Assembly of a library of pel-oligosaccharides featuring α-glucosamine and α-galactosamine linkages. Front. Chem. 10, 842238. doi:10.3389/fchem.2022.842238
Zhang, Y., He, H., Chen, Z., Huang, Y., Xiang, G., Li, P., et al. (2021). Merging reagent modulation and remote anchimeric assistance for glycosylation: Highly stereoselective synthesis of α-glycans up to a 30-mer. Angew. Chem. Int. Ed. 60, 12597–12606. doi:10.1002/anie.202103826
Zhong, X., Zhao, X., Ao, J., Huang, Y., Liu, Y., Zhou, S., et al. (2022). An experimental and theoretical study on stereocontrolled glycosylations by a “one-pot” procedure. Org. Chem. Front. doi:10.1039/D2QO00727D
Zhong, X., Zhou, S., Ao, J., Guo, A., Xiao, Q., Huang, Y., et al. (2021). Zinc(II) iodide-directed β-mannosylation: Reaction selectivity, mode and application. J. Org. Chem. 86, 16901–16915. doi:10.1021/acs.joc.1c02091
Keywords: 1,2-cis glycosylation, stereoselective assembly, method for activation of glycosylation, bimodal glycosylation, intramolecular aglycon delivery (IAD)
Citation: Ishiwata A, Tanaka K, Ao J, Ding F and Ito Y (2022) Recent advances in stereoselective 1,2-cis-O-glycosylations. Front. Chem. 10:972429. doi: 10.3389/fchem.2022.972429
Received: 18 June 2022; Accepted: 08 July 2022;
Published: 19 August 2022.
Edited by:
Leonid O. Kononov, N.D. Zelinsky Institute of Organic Chemistry (RAS), RussiaReviewed by:
Todd Lowary, University of Alberta, CanadaCopyright © 2022 Ishiwata, Tanaka, Ao, Ding and Ito. This is an open-access article distributed under the terms of the Creative Commons Attribution License (CC BY). The use, distribution or reproduction in other forums is permitted, provided the original author(s) and the copyright owner(s) are credited and that the original publication in this journal is cited, in accordance with accepted academic practice. No use, distribution or reproduction is permitted which does not comply with these terms.
*Correspondence: Akihiro Ishiwata, YWlzaGl3YUByaWtlbi5qcA==; Feiqing Ding, ZGluZ2ZxM0BtYWlsLnN5c3UuZWR1LmNu; Yukishige Ito, eXVraXRvQGNoZW0uc2NpLm9zYWthLXUuYWMuanA=
†These authors have contributed equally to this work